- 1New Technologies Research Center (NTRC), Amirkabir University of Technology, Tehran, Iran
- 2Department of Biomedical Engineering, Amirkabir University of Technology (Tehran Polytechnic), Tehran, Iran
- 3Burn Research Center, Iran University of Medical Sciences, Tehran, Iran
- 4Department of Biomaterials, Faculty of Interdisciplinary Science and Technology, Tarbiat Modares University, Tehran, Iran
- 5School of Mechanical Engineering, College of Engineering, University of Tehran, Tehran, Iran
- 6Institute of Orthopaedic and Musculoskeletal Science, University College London, Royal National Orthopaedic Hospital, Stanmore, United Kingdom
- 7Institute of Biomaterials, Department of Materials Science and Engineering, University of Erlangen-Nuremberg, Erlangen, Germany
Increasing concern about age-related diseases, particularly musculoskeletal injuries and orthopedic conditions, highlights the need for strategies such as tissue engineering to address them. Surface modification has been developed to create pro-healing interfaces, personalize scaffolds and provide novel medicines. Polydopamine, a mussel-inspired adhesive polymer with highly reactive functional groups that adhere to nearly all substrates, has gained attention in surface modification strategies for biomaterials. Polydopamine was primarily developed to modify surfaces, but its effectiveness has opened up promising approaches for further applications in bioengineering as carriers and nanoparticles. This review focuses on the recent discoveries of the role of polydopamine as a surface coating material, with focus on the properties that make it suitable for tackling musculoskeletal disorders. We report the evolution of using it in research, and discuss papers involving the progress of this field. The current research on the role of polydopamine in bone, cartilage, muscle, nerve, and tendon regeneration is discussed, thus giving comprehensive overview about the function of polydopamine both in-vitro and in-vivo. Finally, the report concludes presenting the critical challenges that must be addressed for the clinical translation of this biomaterial while exploring future perspectives and research opportunities in this area.
Introduction
Musculoskeletal injuries may affect any of the following tissue types: bone, cartilage, nerve, tendon, and muscle, and treatment will depend on the severity of the injury (Zhao et al., 2019; Naghieh et al., 2021). Over 1.71 billion people worldwide have musculoskeletal disorders (WHO, 2021), leading to an exponential demand for tissue engineering (TE) studies to help regenerate injured tissues. The treatment outcome of musculoskeletal disorders is affected by mechanical properties, bioactivity, immune response, and cytotoxicity of scaffolds, which immensely act tissue reconstruction and the success of the implantation process (Bakhtiary et al., 2021; Heid et al., 2022).
Biomaterials-tissue contact interface of scaffolds which are an integral part of a regeneration process induces immune reactions in the patient’s body (Arab-Ahmadi et al., 2021). Moreover, tissue regeneration is affected by the secretion of immune cells during tissue formation, and in case of any abnormal reaction, scaffolds are prone to failure. However, tissue repair can be induced by the immune system and significantly enhanced according to the condition (Yang et al., 2019). For instance, by altering the implant surface, tissue integration and antimicrobial properties can be highly enhanced (Gao et al., 2017a). Hence, the surface properties of scaffolds are critical factors that must be considered since they affect cell attachment and proliferation (Gao et al., 2017b; Alfieri et al., 2022).
Over the last decade, polydopamine (PDA), a mussel-inspired adhesive polymer with a highly reactive functional group including amine, imine, and catechol that adheres to nearly all different substrates and immobilizes biomolecules, has been increasingly investigated. A variety of cellular responses, such as cell spreading, proliferation, migration, and differentiation, can also be influenced by it in the body, enhancing in-vivo performance of scaffolds (Jia et al., 2019; Rezaei et al., 2021). PDA may properly functionalize a broader range of surfaces than other surface modification materials (Ghorbani et al., 2019a; Cheng et al., 2019). PDA presented high biocompatibility by simulating cell interaction as a newly found surface modification material. As a result, it has gained attention and has been widely used in various areas over the last decades (Wang et al., 2015; Ryu et al., 2018; Ghorbani et al., 2020a). This review aims to investigate PDA influence as a coating material in TE, study the evolution of using PDA in scaffold coating and report the recent developments of PDA surface-modified scaffolds in musculoskeletal TE.
Polydopamine as a coating material for enhancing surface functionality of biomedical devices
PDA, as a synthetic eumelanin polymer derived from dopamine (DA), has a molecular structure similar to 3,4-dihydroxy-l-phenylalanine, consists of oligomers that differ in chain lengths (trimers and tetramers are abundant) and different monomers compositions (Liebscher, 2019; Davari et al., 2022). PDA is characteristically exceptional, extremely biocompatible, and has a distinctive chemical structure of a different functional group that includes catechol, amine, and imine, helping to immobilize molecules and absorb metal ions. Hence has been widely used in regulating tissue and cellular response to materials (Shokrollahi et al., 2020; Ghorbani et al., 2021). PDA’s adhesive intensity is attributed to its catechol and aminoethyl groups (Gao et al., 2019; Sarkari et al., 2022). A reduction in aminoethyl groups through extensive 5,6-dihydroxyindole (DHI) synthesis reduces adhesiveness. Therefore, PDA coatings endow the surface with hydrophilic properties (Figure 1) (Liebscher, 2019). PDA has a high level of cellular affinity and the capability to reduce inflammation, immunological response, and cytotoxicity (Hong et al., 2011; Han et al., 2017; Singh et al., 2021).
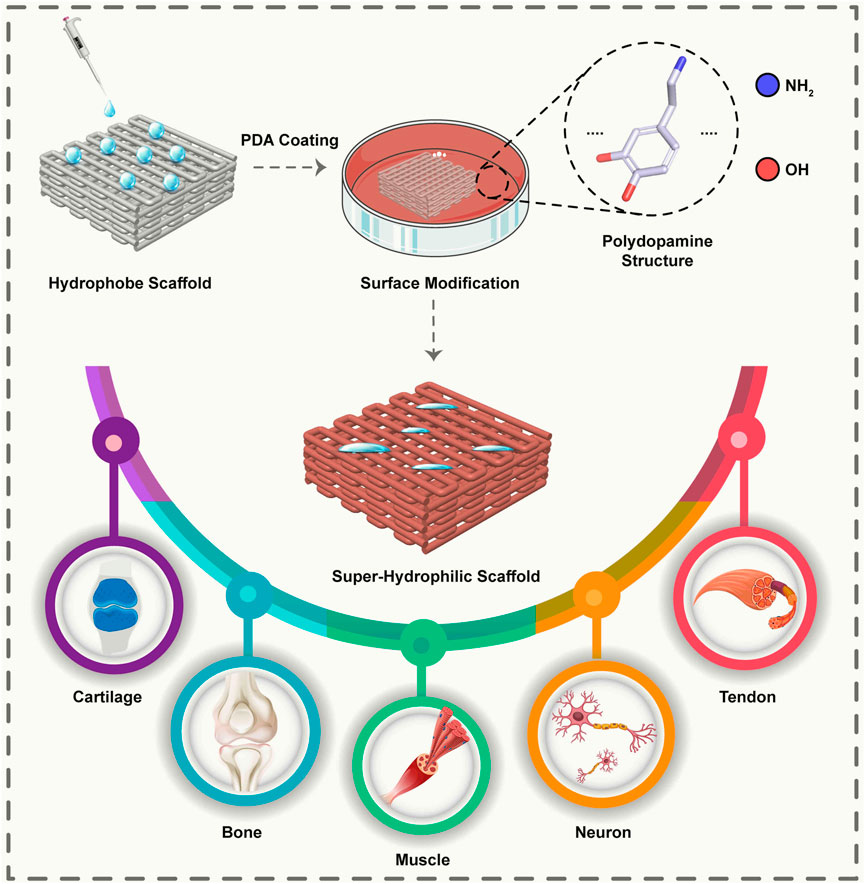
FIGURE 1. Schematic representation of a surface modified with PDA leads to a super hydrophilic surface and enables the adhesion of cells to the surface. It is possible to use scaffolds modified with PDA for various applications in various musculoskeletal tissues.
PDA coatings possess many advantages compared to conventional surface modification strategies, and recently PDA coating mechanisms, chemical structure, and potential applications have been extensively discussed (Ryu et al., 2018; Barros et al., 2021). The PDA coating procedure consists of two steps. The first phase includes the DA monomers autooxidation in which DA quinone is formed in the existence of dissolved oxygen, alkaline buffer, and a sufficient amount of DA hydrochloride monomers, leading to the formation of PDA. The crucial step in PDA constitution is the DA oxidation to dopaquinone in aerobic conditions (van der Leeden, 2005; Jia et al., 2019). Despite the existence of two models: “covalent bonding” or “noncovalent bonding”, the second phase of PDA formation remains elusive. Although the adhesion processes are unknown yet, they are linked to chemical compositions and activity. Since covalent and non-covalent bindings are the two basic types of adhesion mechanisms, an increase in non-covalent bonds will result in stronger adhesion (Gao et al., 2019).
The polymerization process and the mechanism of dopamine formation on different surfaces are similar. Still, some parameters of PDA coating formation, such as immersion time and growth rate and PDA layer formation on different surfaces, are different, as well as some results, such as the thickness of the formed layer and the homogeneity of the layer can be varied depend on the surface (Kang et al., 2009; Lynge et al., 2011; Cui et al., 2018; Ryu et al., 2018).
PDA coatings exhibit several desirable characteristics, including non-specific deposition, processing under mild and controlled conditions to prevent damage during the irradiation process, and preserving scaffolds’ permeability (Miller et al., 2017). In this process, PDA acts as either an oxidant or a reductant which is possible due to its oxidizing quinonyl and reductive catechol. Whatever the surface’s hydrophilicity or hydrophobicity, PDA forms on organic or inorganic surfaces, affecting the surface characteristic of composite materials (Ryu et al., 2018). In addition, because PDA rarely binds to chemicals other than water and solutions containing metal ions, it is helpful in the deposition (Miller et al., 2017). During the coating process, surfaces are immersed in an aqueous alkaline DA hydrochloride solution over a period of time, and following bonding, PDA serves as a secondary platform for functional materials to bind to the surface. (Miller et al., 2017; Ryu et al., 2018). The thickness of PDA coating depends on many factors, including polymerization time, pH, solvents, dopamine concentration, and most importantly, the type of substrate and the temperature. For instance, as a result of using Gompertz’ equation and data reported in the research, Habibi Rad et al. (Habibi Rad et al., 2018) presented a kinetics model for PDA layer growth based on the results obtained on different substrates and temperatures. Based upon the reported data, Gompertz’ equation was chosen, which indicates that the inflection point of Gompertz’ curve occurs at a thickness of 37–40% regardless of the substrate and system temperature. A kinetics model proposed by the authors proposes that polymerization occurs in two stages, with the peak growth rate occurring at 37–40% of the ultimate thickness of the film. Maximum growth rates increase with increasing temperature. Higher temperatures, however, result in a slower growth rate for the system. Alternatively, under normal reaction (air, pH 8.5), PDA thickness reaches 50 nm, which takes nearly a few hours up to a few days (Lee et al., 2007). Moreover, the loss of water permeability that is common in other membrane coating procedures can be alleviated by managing the deposition thickness of the PDA layer. This can be achieved through changing PDA deposition timing or the DA concentration in the coating solution, which should be more than 2 mg/ml to produce a PDA film on the surface (Liu et al., 2014). The PDA coating deposition rate can be expedited using ultraviolet irradiation, electrochemical actuation, and oxidant promotion (Jia et al., 2019). A new approach has been proposed by Zhang et al. (Lee et al., 2017), which uses CuSO4/H2O2 to accelerate the PDA deposition rate.
Evolution of PDA in musculoskeletal regeneration
In recent decades, PDA has attracted significant attention for its unique properties, such as anti-fungal and anti-bacterial properties, adhesion properties, and converting light into heat with high efficiency. This substance has been used in various research fields, such as energy, food industry, and biological applications like cancer treatment, biosensors, drug delivery systems, and TE scaffolds (Xing et al., 2017; Tejido-Rastrilla et al., 2019; Hauser et al., 2020; Shokrollahi et al., 2020). The remainder of this section will discuss the emergence and evolution of PDA in TE and review previous key studies on the applications of PDA in of musculoskeletal TE.
PDA films with DA polymerization showed robust adhesion capabilities when Lee et al. (Lee et al., 2007) synthesized PDA coatings in 2007. Afterwards, PDA has been used extensively in bioengineering, and the use of this material in the coating of scaffolds for TE has a considerable upward trajectory to date (Figure 2).
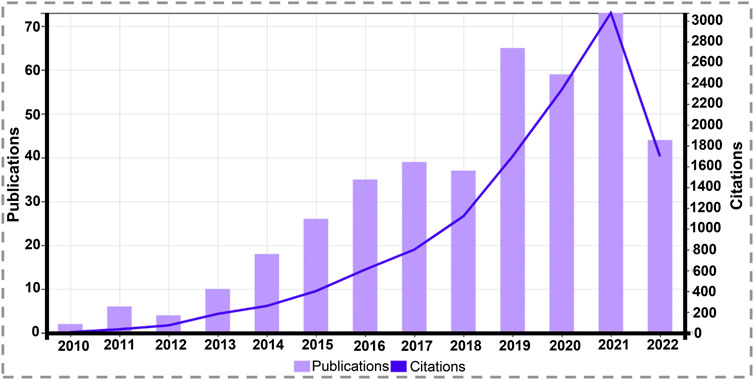
FIGURE 2. Graph of total scientific papers published and citations per year on “tissue engineering” and “polydopamine coating” since 2010 (source: Web of Science, July 2022).
Due to the natural adhesive properties of PDA, as well as the possibility of further modifications, PDA can be used to coat bioinert surfaces with potentially increased bioactivity (Barros et al., 2021). By coating the scaffold with PDA, the researchers enhanced cell attachment to the scaffold and brought these adhesive properties into many biomedical applications, especially TE (Lynge et al., 2011; Ghorbani et al., 2022). In developing fields such as organs on a chip and cell sheet engineering, it is also essential to control the spatial distribution of cells on a cell-adhesive patterned structure formed on a non-interacting surface; For this purpose, PDA can be coated in PDMS mold channels to drive cells to adhere to this non-adhere surface (Lynge et al., 2011; Khetani et al., 2020).
Even though bone grafts such as autografts, allografts, and xenografts are commonly used in clinical treatment, they are not ideal for treating bone defects due to their limitations. Ideally, Bone defects should be addressed with a scaffold that facilitates cell proliferation and attachment, exhibits osteodifferentiation capacity, and promotes mineralization (Huang et al., 2016). In bone TE, polymers are an essential material category. Despite the fact that most polymeric biomaterials have suitable properties, they do not provide sufficient osteointegration. The surface characteristics of these polymers can be significantly enhanced by PDA-assisted surface modification. Immobilization of growth factors can substantially improve osteoblast cells’ response to polymeric materials and accelerate the process of material mineralization. As part of bone TE, bone filling materials are commonly used; the PDA coating layer can improve cytoaffinity of the bone cement, resulting in increased fibroblast attachment to the substrate, thereby enhancing cell proliferation (Ko et al., 2013; Cho et al., 2014; Jun et al., 2014; Ghorbani et al., 2019b).
A study conducted in-vivo has demonstrated that the PDA coating layer contributes significantly to the biocompatibility of bone substitutes. A poly l-lactic acid (PLLA) film coated with PDA reduces quantum dots’ blood toxicity and enhances their immunogenicity and biocompatibility (Hong et al., 2011). Rim and others modified electrospun PLLA fibers using PDA coating. Several assays were used to determine the effects of PDA modification on hMSC differentiation, including alkaline phosphatase (ALP) activity, collagen matrix formation, osteogenic gene expression, and calcium mineralization. According to SEM images, the PDA-PLLA fibers were coated uniformly with PDA. A comparison of PDA-PLLA fibers with PLLA fibers revealed significant improvements in adhesion, spreading area, ALP activity, and osteogenic differentiation genes. On the basis of these results, the PDA modification in the PLLA was able to control stem cell activity and, thus, serve as a viable carrier for stem cell delivery (Rim et al., 2012).
PDA coating layers can immobilize tender biomolecules that are readily deactivated due to differences in environmental circumstances while exerting no or a small impact on their activity. Through the PDA coating, proteins and growth factors, like bone morphogenic protein-2 (BMP-2), Arg-Gly-Asp peptide, and ALP, can adhere to the surface of scaffold surface. Studies have shown that the PDA layer itself does not influence the activity of these biomolecules other than the molecular weight, incubation time, isoelectric point, and concentration of the biomolecules themselves (Nijhuis et al., 2013). Two studies by Chien and others in 2013 demonstrated that PDA coatings can be used to control cellular behavior. Researchers have found that PDA promotes osteoblast proliferation and calcium deposition and that this effect can be amplified when combined with growth factors (Chien et al., 2013; Chien and Tsai, 2013). In a valuable study, Kao and others (Kao et al., 2015) coated three-dimensional (3D) printed PLA scaffolds with PDA for bone TE. In this study, they directly immersed scaffolds in DA solution to create a PDA coating on them, and this work resulted in increased hydrophilicity and subsequent biocompatibility of scaffolds. PDA-coated samples also revealed decreased bacterial activity and indicate that this easy surface modification induces angiogenesis and osteogenesis differentiation and is a potentially advantageous method to adjust stem cell activity and can act as a helpful carrier for stem cells in bone TE. In a valuable study in 2016, Lee et al. (2016) created bone-tissue regenerative scaffolds by grafting recombinant human BMP-2 (rhBMP-2) to 3D printed polycaprolactone (PCL) scaffolds through coating with PDA. PCL has efficient mechanical properties as a scaffold for bone tissue engineering, and the PDA coating has promoted the hydrophilicity of the PCL and caused efficient immobilization of rhBMP-2, resulting in suitable cell proliferation and osteogenic activity, which suggest that this strategy would be a practical approach for the bone TE field. In the same year, using PDA coating, Wang et al. (2016) immobilized the collagen biomimetic peptide and osteogenic growth peptide on the surface of the poly (lactic-co-glycolic-acid) (PLGA)-hydroxyapatite composite scaffold, which the PDA coating resulted in a notable enhancement in the hydrophilicity of the scaffold and effective peptide immobilization on the substrate, which shows that this causes promotion of MC3T3-E1 cells adhesion, proliferation, and osteodifferentiation compared with the bare substrates and demonstrate that biofunctionalized surfaces with bioactive peptides can supply an exciting method for modification of bone substitutes in musculoskeletal TE.
To achieve sustained release of biomolecules, Yao et al. (2018) proposed that heparin-DA modified graphene foam could be used to enhance BMP-2 release. By coating heparin on the surface of scaffolds with a mimetic DA ligand, the researchers developed 3D graphene foam with robust binding of rhBMP-2. Using this method, durable osteogenic differentiation was achieved as well as the production of an innovative carrier that can accommodate proteins and stem cells. Recently, a valuable study by Ghalandari and others (Ghalandari et al., 2021) indicated the basic mechanism of protein coating formation by coating PDA nanospheres with bovine serum albumin (BSA) and evaluating their binding system and the impact of surface modification on BMSCs’ performance. Their results indicate that the PDA nanospheres coating with BSA resulted in a thin homogeneous appropriate layer for cell adhesion and viability. Furthermore, the expression of osteogenic markers proves a promising ability of the coated PDA spheres for bone tissue engineering.
Since cartilage is an avascular and aneural tissue with restricted chondrocyte proliferation potential, its ability to self-heal is limited. In spite of the fact that many surgical and non-surgical techniques have been developed as treatment or replacement methods for damaged articular cartilage, none of them has been successful to restoring articular cartilage’s native structure and biomechanical properties; therefore, researchers have focused on reconstructing strategies for cartilage TE in-vitro (Kessler et al., 2008; Wang et al., 2022).
In 2011 Tsai et al. (2011) demonstrated that PDA-coating on various polymers increased the chondrocytes’ adhesion and proliferation and revealed the potential of this simple strategy for improving cartilage TE scaffolds. In a valuable study in 2013, Cai and others (Cai et al., 2013) coated a 3D printed PCL scaffold with PDA and then grafted it with collagen, making the scaffold hydrophilic and suitable for cell adhesion. Upon these surfaces, chondrocytes are able to attach and maintain a healthy phenotype through the production of cartilage-like extracellular matrix (ECM). PDA was used by Ren et al. (2019) as a coupling agent for grafting chondroitin sulfate onto a PLLA fiber membrane. As a result of their biomimetic surface modifications, the PLLA fibers demonstrate a significant increase in chondrocyte proliferation and attachment, as well as chondrogenic gene expression in rat bone-marrow mesenchymal stem cells (rBMSCs). Additionally, in vivo experiments on 6-week-old rabbits demonstrated that the membranes greatly boosted the repair of cartilage defects and the production of hyaline cartilage at the defect zone.
Mechanical properties of the tendon do not reappear following healing, and in most cases, a rupture occurs again. This may happen due to the cells composing the new regenerated tendon are not tenocytes, which are the dominant cells of a healthy tendon (Linderman et al., 2016; Ruiz-Alonso et al., 2021). In this regard, it has been demonstrated that PDA-coated scaffolds can differentiate stem cells into tenocytes. In a study in 2018 (Madhurakkat Perikamana et al., 2018), platelet-derived growth factor (PDGF), a key mediator in tendon repair, was immobilized on a gradient-aligned PDA-coated PLLA nanofiber to regulate the tenogenic differentiation of adipose-derived MSCs (AdMSCs) and found that PDA coating provides a steady display of immobilized PDGF and bioactivity for 2 weeks of incubation which improved the proliferation and tenogenic differentiation of AdMSCs.
The objective of muscle TE is to restore skeletal muscle function that has been impaired by injury, tumor ablations, or congenital defects. Muscle cells’ capability to regenerate under in-vivo situations is limited, so muscle TE methods aim to mimic the natural environment via scaffolds in conjunction with cells. As a result, several approaches have been developed to enhance the interactions of biomaterials and muscle cells (Madhurakkat Perikamana et al., 2015). For instance, van der Westen and others in 2012 (van der Westen et al., 2012) coated liposomes with PDA and found that this led to higher myoblast viability than unmodified ones. After a year Ku et al. (Ku and Park, 2013) developed PDA-coated aligned PCL nanofibers which is a promising scaffold for skeletal TE, and demonstrated the expression of myogenic proteins and fusion of myoblasts were enhanced compared to their unmodified counterparts.
PDA has been used extensively for in coating neural TE scaffolds in recent years as a result of its desirable properties. Research shows that PDA-modified scaffolds can efficiently absorb neural stem cells, indicating their value for repairing damaged nerves (Yan et al., 2020). In 2012 Yang et al. (2012) demonstrated that PDA modification of substrates promotes the differentiation of stem cells into neurons; in this regard, they immersed various substrates like PLGA and PDMS in a DA solution for 18 h to coat substrates with PDA, and after that, they coated these PDA-coated substrates with adhesion peptides and growth factors such as laminin, fibronectin, and neurotrophic growth factors and then cultured these substrates with neural stem cells. Results demonstrated that PDA is a promising material for effective surface immobilization of proteins and peptides to a manifold variety of biomaterials, and the production of biomimetic scaffolds that promote favorable stem cell activity. On PDA-coated scaffolds, nerve growth factor (NGF) stimulated PC12 cells showed enhanced neuronal differentiation (Bhang et al., 2013). This mussel-inspired bioactive material exhibits exellent potential to modify nerve TE scaffolds, which could be involved in future studies. According to Chen et al. (Chen et al., 2018a), coating a 3D electrically conductive scaffold with PDA increased the hydrophilicity and cytocompatibility of the scaffolds in addition to promoting the proliferation and differentiation of nerve cells, which were enhanced under electrical stimulation. In 2021 Nazeri and co-workers (Nazeri et al., 2021) reported more prolonged growth and adhesion of neurons on PDA-coated PLGA/carbon nanotube scaffolds.
Applications of PDA in musculoskeletal TE
PDA is increasingly being used as a surface modification material, and recently, a number of studies have been conducted on it due to its affordability, availability, and adhesive properties (Ambekar and Kandasubramanian, 2019). This section presents a review of PDA use in coating scaffolds for bioengineering musculoskeletal tissues, with a summary of the findings listed in Table 1.
Bone regeneration
The lack of bioactivity of artificial implants prevents them from osseointegrating well with host bone tissue. Due to this restriction, it cannot be used to regenerate bone. Feng et al. (2021) modified PCL particles with PDA, fabricated them on a scaffold using selective laser sintering, and demonstrated that PDA imposed a negative charge on the scaffold surface, which attracted Ca2+ ions, forming an apatite layer. Also, the hydrophilic groups of PDA reduced water contact angles to an extent, leading to rapid cell proliferation and adhesion as well as protein absorption. Therefore, it is beneficial for bone TE applications (Figure 3A). Similarly, Park et al. (2021) coated the 3D scaffold with PDA and then deposited hydroxyapatite nanoparticles via biomimetic mineralization to immobilize BMP-2. Using PDA coatings and hydroxyapatite layers containing BMP-2, in-vitro results were positive for osteoblast proliferation and indicated an excellent potential for bone repair.
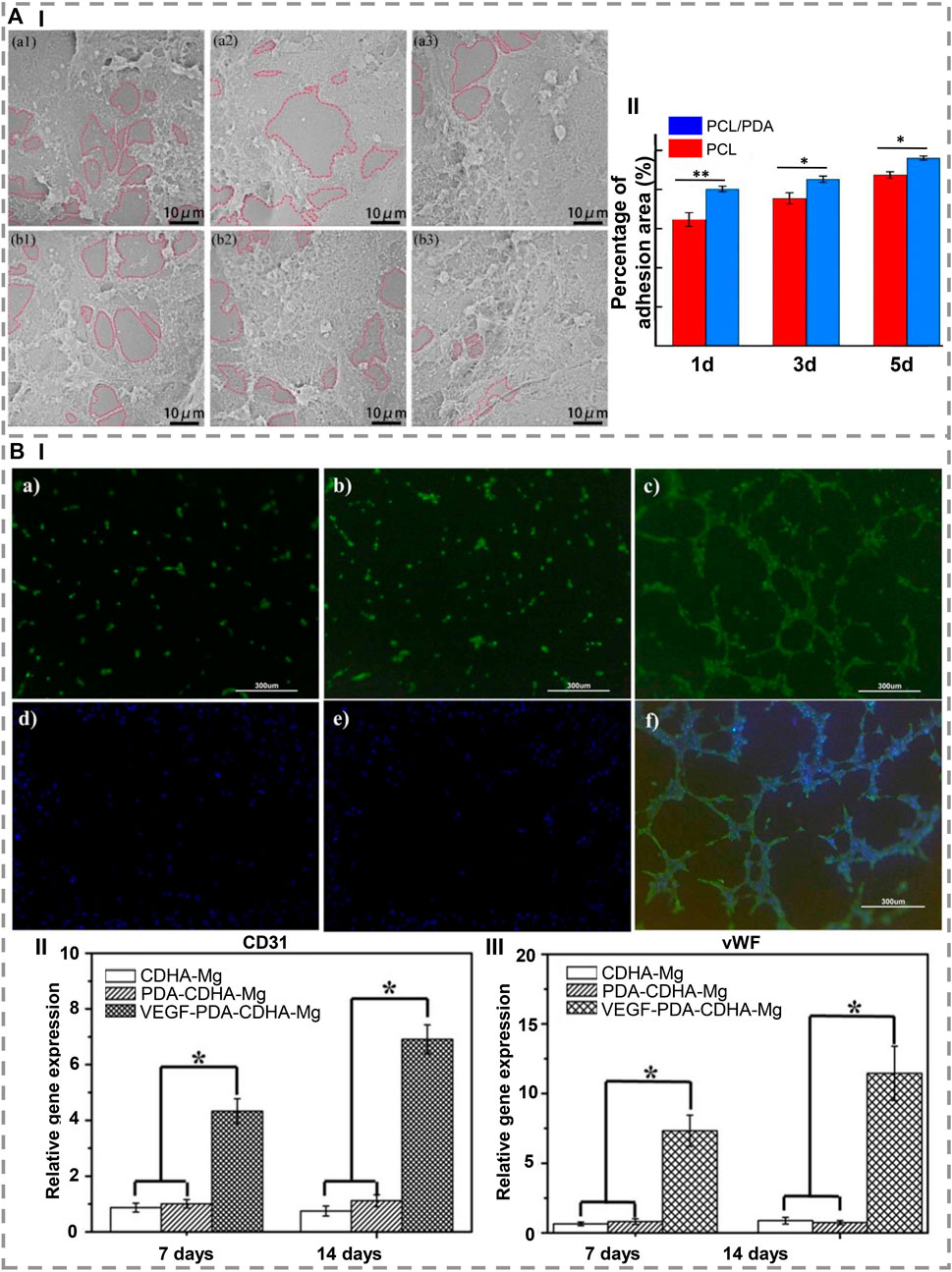
FIGURE 3. (A) (I) SEM images show that, after 1, 3, and 5 days of culture, (a1-a3) PCL and (b1-b3) PCL/PDA scaffolds are capable of adhesion and proliferating cells. (II) After 1, 3, and 5 days of cultivation, the adhesion area of the PCL scaffold and the PCL/PDA scaffold is shown. ∗ indicates a statistical difference (at the same time, *p < 0.05, **p < 0.01) [Reproduced under the terms of the Creative Commons Attribution-NonCommercial-NoDerivatives 4.0 International (CC BY-NC-ND 4.0) license. (Feng et al., 2021) Copyright 2021, Elsevier]. (B) (I) In vitro development of microtubule in rbMSCs cultured on (a) CDHa-Mg, (b) PDA-CDHa-Mg, and (c) VEGF-PDA-CDHa-Mg after 2 weeks. (d–f) Expression of alpha-smag by immunofluorescence in rBMSCs grown on CDHa-Mg, PDA-CDHa-Mg, and VEGF-PDA-CDHa-Mg after 2 weeks. For 7 and 14 days, rBMSCs were cultured on CDHa-Mg, PDA-CDHa-Mg, and VEGF-PDA-CDHa-Mg to determine the expression levels of (II) CD31 and (III) vWF. (n = 3, ∗ indicate statistical significance, p < 0.05) [Reproduced under the terms of the Creative Commons Attribution-NonCommercial-NoDerivatives 4.0 International (CC BY-NC-ND 4.0) license. (Li et al., 2021) Copyright 2021, Elsevier].
Ghorbani et al. (2019c) study investigated electrospun polyurethane (PU)-graphene oxide (GO) scaffolds covered with PDA. Despite the fact that GO incorporated into electrospun scaffolds improved physical and biological properties, PU-GO-PDA scaffolds demonstrated significantly enhanced features, including wettability (contact angle: 54.53° ± 1.34°), water absorption (14% increase in 24 h), MG63 cell attachment, and proliferation. When compared with the control group and the PU-GO group, the PU-GO-PDA scaffold significantly increased proliferation. In addition, the PU-GO-PDA scaffold significantly increased ALP expression, about 70 U/L compared to the control group, about 45 U/L in 7 days, indicating osteogenic differentiation. The PU-GO-PDA scaffold appears to be an effective substrate for bone regeneration in-vivo. The following year, Ghorbani et al. (2020b) studied the physicochemical, mechanical, and in-vitro characteristics of modified electrospun PU-polyaniline (PANI) scaffolds functionalized with PDA as well as the influence of substrate composition chemistry and hydrophilicity on a dense and homogeneous functionalization. In this approach a uniform, dense, and homogeneous coating of PDA applied to the surface of the PU-PANI/PVA scaffold in order to improve its mechanical properties, hydrophilicity, absorption capacity, and mass-loss rate. It was found that the PDA-modified PU-PANI/PVA constructs were able to biomineralize hydroxyapatite-like layers, which is critical for bone regeneration. On high PDA substrates, rBMSCs were shown to adhered and spread well, particularly when electrical stimulation was applied. ALP was expressed, and collagen I was secreted at a suitable level after the membrane was coated with PDA and electrically stimulated.
In order to investigate the effects of polymerizing DA on cell adhesion and electromechanical signaling in poly vinylidene fluoride (PVDF) membranes, Xue et al. (2021) modified PVDF membranes as a bone healing material by polymerizing DA. The results demonstrated that seeded MSCs adhered and spread better on polarized PVDF membranes. The PDA surface modification also improved the hydrophilicity and induced cell focal adhesion formation, thus increasing piezoelectric self-stimulation and intracellular calcium transients. Regarding bone regeneration, Li et al. (2021) immobilized vascular endothelial growth factor (VEGF) on Mg, and as a result, a functional VEGF-PDA-CDHa (Ca-deficient hydroxyapatite) composite coating was successfully applied to Mg. As an intermediate layer between Mg and VEGF, the PDA coating serves as a receptor site for VEGF. This facilitates the development of angiogenesis during the early period of implantation. The VEGF-PDA-CDHa coating on MC3T3-E1 cells on Mg demonstrated great biocompatibility as well as remarkably increased cell adhesion and proliferation (Figure 3B).
According to Ahmad et al. (2020), PDA can be used as a one-step coating to protect adenosine on biomaterial surfaces. In order to create human-adipose-derived stem cell (hADSC) spheroids for bone regeneration, the researchers introduced an adenosine-ligand onto engineered fibers that were inspired by the ECM, using PDA chemistry. Using an adenosine-ligand-engineered fiber, the adenosine 2 b receptor (A2bR) was stimulated, osteogenic differentiation was stimulated, adipogenic differentiation was inhibited, and then engineered spheroids were injected into mice to induce osteogenic differentiation and to regenerate bone in calvarial defects.
In another exciting study, Ghorbani and others (Ghorbani et al., 2020c) fabricated a PCL scaffold through a unidirectional freeze-casting method and modified its surface with PDA, which resulted in increased hydrophilicity and water uptake leading to the modulation of the timing of the PCL scaffold degradation. Also, in-vitro tests revealed that the PDA-modified PCL scaffold appeared to display good biocompatibility and cell proliferation, with more than 85% of L-929 viable cells, and could be a promising platform for further studies in bone tissue engineering.
3D-printed scaffolds of PLGA/β-tricalcium phosphate were coated with PDA by Xu et al. (2019). As a result of the coatings, the mechanical parameters and pores-related features of the multilayer scaffolds are not altered, and the hydrophilicity of the surface is clearly enhanced. In addition to promoting the proliferation and adhesion of cells, the modified surface also promotes osteogenesis through the increase in PDA concentrations. As a result of in-vivo experiments, scaffolds containing a greater amount of PDA coating demonstrate a more remarkable ability to stimulate bone formation.
Deacetylated porous cellulose acetate microspheres (DPCA) with a 3D porous structure are used by Gao et al. (2022) to synthesize hydroxyapatite suspended within a PDA coating. There is a unique porous structure of the DPCA-PDA-hydroxyapatite microspheres (DPPH) scaffold, as well as a uniform coating of PDA and a well-distributed hydroxyapatite crystal distribution throughout the scaffold. MC3T3-E1 preosteoblasts adhered to and grew abundantly on calcium compounds, enhancing osteogenesis in the one-step modification process. With the aid of a facile preparation method, DPPH was fabricated, demonstrating its potential as a scaffold for supporting bone regeneration.
Titanium dioxide (TiO2)-based scaffolds have a great mechanical property besides their biocompatibility for bone tissue engineering. Still, their bioactivity is not enough, and in this regard, Sengottuvelan and coworkers (Sengottuvelan et al., 2017) fabricated TiO2 scaffolds by foam replica molding and then coated them with ALP using PDA to enhance their bioactivity, which resulted in a high porous scaffold with about 97% porosity and compressive strength of ∼2.7 MPa. ALP immobilizing on the surface was resulting in the deposition of HA and demonstrated that this method could enhance the bioactivity of TiO2 scaffolds.
Cartilage regeneration
Cartilage and subchondral bone have a low ability to regenerate and repair; for this reason, researchers such as Wei et al. Wei et al. (2021) coated 3D printed PCL scaffolds with PDA, which increased PCL hydrophilicity, biofunctionality, and biocompatibility as well as improving cell adhesion to the scaffolds. Moreover, it helped scaffold adsorb PLGA nanoparticles loaded with insulin. They discovered that the insulin-PLGA/PDA/PCL scaffolds showed better healing, more stability, and improved cartilage repair in-vivo when used on cartilage and bone. In another study, Luo et al. (2022) constructed a three-layer osteochondral bionic scaffold using hydroxyapatite and silk fibroin. Further, they used PDA on the scaffold, increasing the release and loading of the platelet-driven growth factor for a considerably more extended time than the other group resulting in the differentiation of synovial MSCs into cartilage. They showed that in-vivo experiments, the PDA-modified scaffolds were significantly better at enhancing adhesion and proliferation of cells, leading to the better repair of osteochondral defects and cartilage regeneration. Ding et al. (2022) coated the PU 3D printed scaffold with PDA to enhance biocompatibility and hydrophilicity as well as increase the ability to recruit endogenous MSCs. In addition to reducing the water contact angle of scaffolds, PDA-modification resulted in higher cell viability, uniform adhesion, and improved cell proliferation. Furthermore, implanted scaffolds demonstrated acceptable gene expression for chondrogenic differentiation and cartilage regeneration. In a parallel study, Lai et al. (2021) increased the hydrophilicity of PLLA/graphene electrospun scaffolds with a layer of PDA coating which directly increased cell adhesion, proliferation, and chondrogenic differentiation.
To mimic the ECM of cartilage, a PDA-modified hyaluronic acid (PDA/HA) was integrated into a collagen substrate to achieve a collagen/PDA/HA scaffold (Gan et al., 2022). This scaffold provides cell attachment with 3D framework support by dually cross-linking the collagen substrate to create matrices with durable mechanical characteristics and resembling the ECM’s connective fibers. By functionalizing HA using PDA, adhesive catechol groups are grafted to HA chains, which mimic the ECM’s component glycosaminoglycans, increasing cartilage-specific gene expression and enhancing cell adhesion, which can efficiently direct BMSCs to osteogenic differentiation. The collagen/PDA/HA hydrogel scaffolds displayed remarkable cell affinity and cytocompatibility. To illustrate this, BMSCs were cultured on the scaffold, and, for comparison, it also individually cultured on scaffolds made of hydrogel components. Figures 4Aa,b show that only a small number of cells were present on scaffolds that do not contain PDA. On the other hand, there are considerable numbers of cells on the surface of scaffolds containing PDA. The enlarged images revealed that most cells on the PDA-containing scaffolds showed usual spindle-like morphology (Figures 4Ac,d), confirming that the PDA segment in the hydrogels promoted cell spreading as well as adhesion and demonstrating that PDA functionalization enhanced cell affinity for HA. To assess its capability to support cartilage regeneration in-vivo, collagen/PDA/HA scaffolds were implanted into rabbit cartilage defects. As positive groups, transforming growth factor-β3 (TGF-β3)-loaded hydrogels were implanted too. Macroscopic gross morphology analysis revealed that regenerated tissues mostly filled the defects in all groups 12 weeks after implantation (Figure 4B). Collagen/PDA/HA treated group filled defects by forming cartilage-like tissue maintaining a uniform and homogeneous surface that merged well with host cartilage (Figure 4Bv), which was similar to the TGF-β3-loaded group (Figure 4Bvi). The quality of restored cartilage was assessed by histological analysis after H&E (Figure 4C), safranin-O staining (Figure 4D) and immunohistochemistry (IHC) for collagen type II and aggrecan. In the collagen/PDA/HA group, the injury was filled with a uniform and dense cartilage layer congruent with the neighboring host cartilage (Figure 4Cv). In comparison with normal cartilage, the collagen/PDA/HA hydrogel scaffold showed comparable Safranin-O staining. It was observed in IHC that both collagen II and aggrecan were severely stained in the collagen/PDA/HA groups, as well as in the TGF-β3-loaded groups, indicating that collagen/PDA/HA hydrogel groups produced an adequate amount of ECM and integrated cartilage repair. Furthermore, these results demonstrate that new cartilage formed by collagen/PDA/HA was phenotypically stable. In-vivo investigations indicated that collagen/PDA/HA hydrogel scaffolds were more capable of repairing cartilage tissue than collagen/HA scaffolds, resulting in higher quality cartilage tissue than collagen/HA scaffolds.
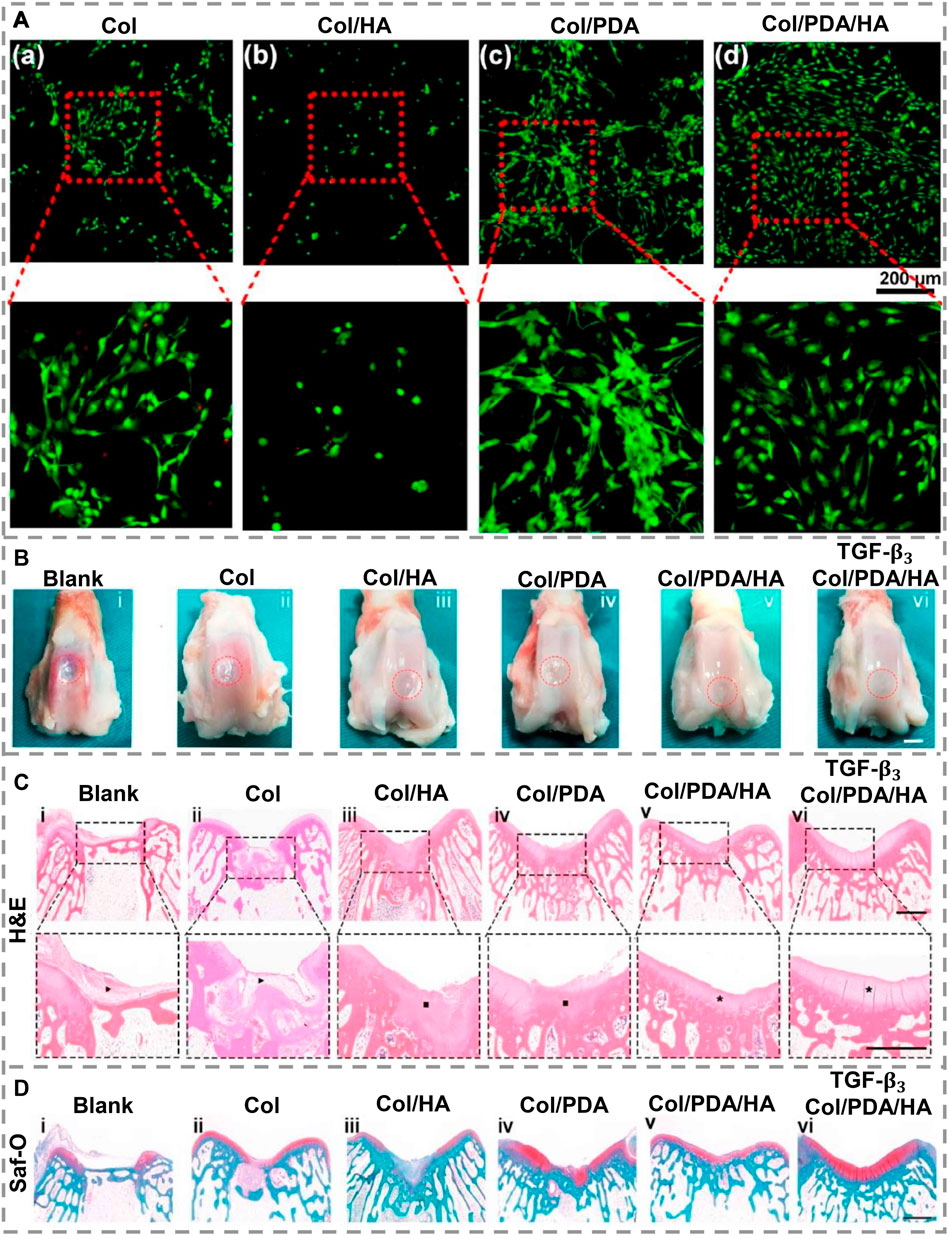
FIGURE 4. (A). In-vitro cytocompatibility assessment of scaffolds after 3 days of cultivation in primary medium (a–d). A green stain was applied to live cells and a red stain to dead cells. (B). Hydrogel scaffolds for cartilage repair in-vivo. After 12 weeks of post-healing, a macroscopic examination of regenerated cartilages was conducted on different groups. (C). H&E and (D). Staining of post-sacrifice defects with Safranin-O. The dashed lines indicate the approximate location of defect sites. Fibrous tissue is indicated by black arrows, while cartilage tissue is indicated by black squares. A black asterisk indicates well-organized cartilage. [Reproduced under the terms of the Creative Commons Attribution-NonCommercial-NoDerivatives 4.0 International (CC BY-NC-ND 4.0) license. (Gan et al., 2022) Copyright 2022, Elsevier].
During the fabrication of their scaffold, Tan et al. (2018) used PDA as a surface modification material and suggested it for cartilage TE. In this investigation, the surface of porous PLLA membrane was coated with PDA, which resulted in a water contact angle of 58.51° ± 3.50°, indicating that PDA led to a more hydrophilic surface. It was observed that porous PLLA membranes without a coating of PDA formed water droplets on their surfaces. The PDA coating instantly absorbed the water droplet into the membrane architecture, demonstrating the benefits of this coating. In an impressive study, Shen and others (Shen et al., 2018) utilized DA and PDA to create a new alginate scaffold via chemical and physical interactions to fabricate a scaffold with optimal mechanical features and biological activity for cartilage TE. To fabricate an alginate-DA/PDA scaffold, alginate has been modified with DA through esterification and then knotted with PDA nanoparticles. In addition to its excellent biocompatibility and cell attachment, this scaffold showed promise for cartilage TE since it exhibits continuous pore structure, reduced pore size, better compressive modulus, and a lower degradation rate than the one of other scaffolds.
Muscle regeneration
Skeletal muscle regeneration still faces the challenge of scattered injury and diseases. Zhou L. et al. (2021) developed polypyrrole (PPy) covered by PDA in a crosslinked nanocomposite hydrogel that displays a thermo-responsive gelation property. Their hydrogel exhibited excellent adhesion, antioxidant abilities, and electrical conductivity for in-vitro and in-vivo stimulation of myoblast differentiation and repair of skeletal muscle (Figure 5A). Xiong et al. (2022) have reported the synthesis of PDA and PLLA nanofibers. Following post-in situ polymerization, PDA can be uniformly coated on PLA nanofiber, and these composites exhibit superior mechanical properties, hydrophilic properties, enhanced oxidation resistance, and near-infrared photothermal properties. PDA/PLLA scavenges reactive oxygen species (ROS) and promotes tissue repair in-vitro as well as good histocompatibility and increase of fibroblasts number after 7 days implantation of PDA/PLLA into mouse back muscles.
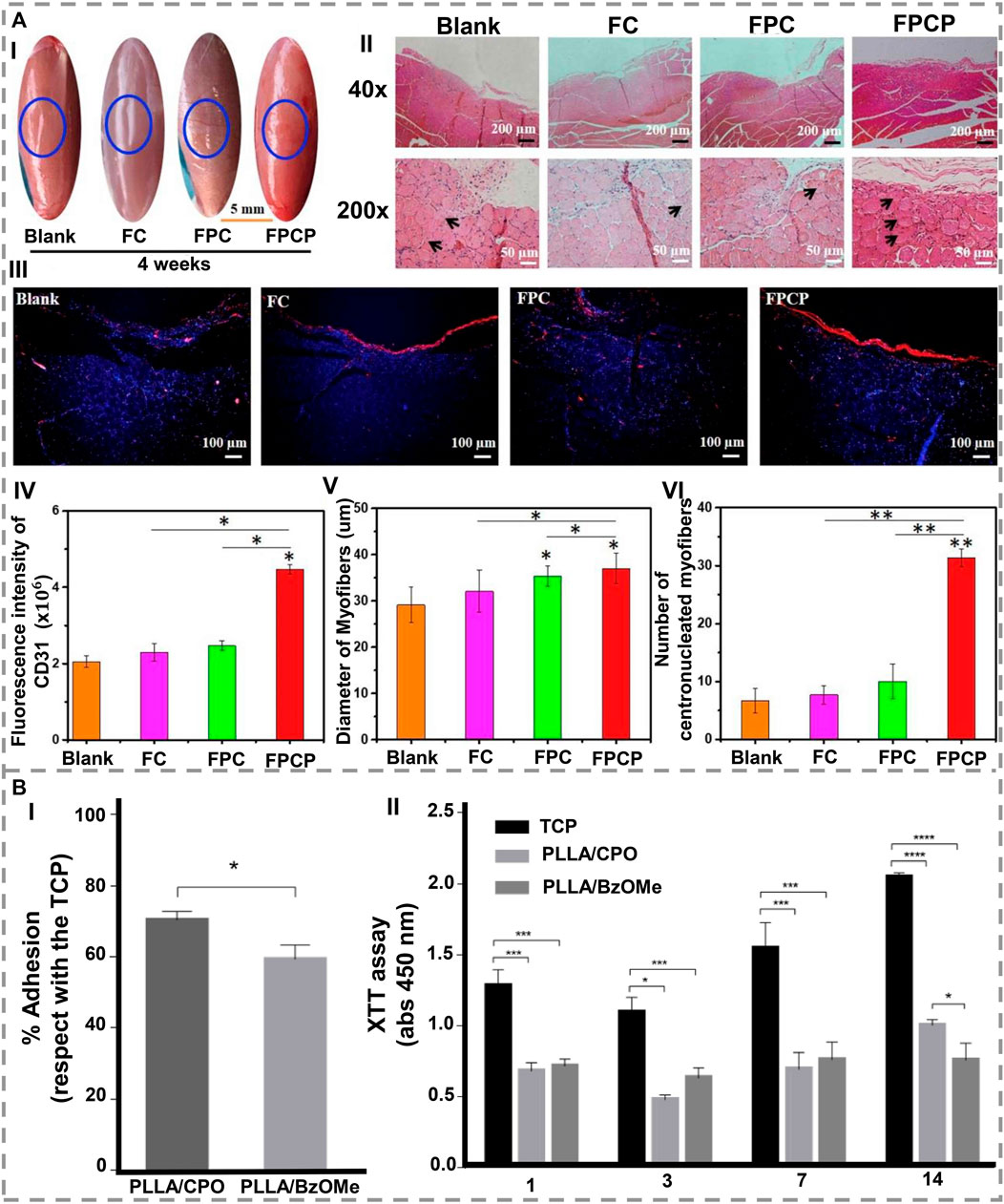
FIGURE 5. (A) A period of 4 weeks of treatment with FPCP hydrogel led to the regeneration of skeletal muscle in-vivo (blue: a sham operation). (I) The following images describe the skeletal muscle defect in detail. (II) Images of the defect area stained with H&E and showing centronucleated myofibers. (III) CD31 stained images (blue represents nuclei, red represents CD31). (IV) Quantitative analysis of CD31. (V) Measurements of myofiber diameter, and (VI) numbers of regenerated myofibers (centronucleated myofibers) near the defect site. By using ImageJ software, three randomly selected fields of each section and three sections per specimen were analyzed (*p < 0.05 and **p < 0.01) [1. Reproduced under the terms of the Creative Commons Attribution-NonCommercial-NoDerivatives 4.0 International (CC BY-NC-ND 4.0) license. (Zhou L. et al., 2021) Copyright 2021, Elsevier]. (B). In-vitro evaluation of PLLA/CPO and PLLA/BzOMe aerogels. (I) Cell adhesion after 24 h. Results illustrate the percentage of adhesion concerning the control (TCP). (II) Cell proliferation after 1, 3, 7, and 14 days (*p < 0.05; ***p < 0.01; ****p < 0.0001) [2. Reproduced under the terms of the Creative Commons Attribution 4.0 International (CC BY 4.0) license. (Orlacchio et al., 2022) Copyright 2022, MDPI].
The surface properties of the matrix influence myogenic differentiation and maturation. Hence, DA self-polymerization can modify nanofiber matrices before coating them with conductive polymers and promote myoblast differentiation by upregulating myogenic markers. Therefore, Tang et al. (2020) fabricated a new stimulus-responsive conductive nanocomposite structure in which they first coated PCL fibers with PDA and then with poly (3,4-ethylene dioxythiophene): poly (styrene sulfonate). The results showed that the DA coating successfully increased hydrophilicity while minimally affecting fiber morphology. Based on the presented results, it has been demonstrated that aligned conductive fibrous matrices can be used to restore skeletal muscle. In a recent study, Orlacchio and others (Orlacchio et al., 2022) observed that PDA enhanced the hydrophilicity of PLLA aerogels and improved their bioactivity, indicating that this could allow electrical signals to be transferred amongst the cells resulting in an effective scaffold for tissues with bioelectrical functionalities such as muscle (Figure 5B).
Nerve regeneration
The main issue concerning nerve repair is the lack of transplantable nerve tissue, and as a widely used material in surface modification, PDA has been utilized to modify nerve conduits to repair and regenerate injured nerves. For this reason, Yang et al. (2020) fabricated a PDA-functionalized carbon microfibrous electrospun scaffold, and they found that the expression of Ki-67 protein has been expanded by PDA, which consequently increased the cell adhesion and proliferation of neural stem cells. Additionally, they realized that neural stem cells (NSCs) formed more rapidly on the PDA-carbon microfibrous surface than on the carbon microfibrous scaffold (Figure 6A). According to other research, Chen et al. (2018b) deposited PDA directly onto 3D printed scaffolds constructed from PCL. In-vitro experiments demonstrated that the existence of PDA improved the hydrophilicity of nerve conduits, and increased Schwann cells (SCs) growth, adhesion, differentiation, proliferation, and cell functions, as well as mechanical properties (Figure 6B). Afrash et al. (2021) fabricated PCL/chitosan nanofibers with PDA and found that coated DA layer conjugated NGF onto the scaffold, improved cell adhesion and proliferation by immobilizing NGF (Figure 6C). Recently, Pi et al. (2022) modified PCL/carbon nanotube fibrous scaffolds with PDA in order to obtain scaffolds that release sustained levels of brain-derived neurotrophic factor (BDNF). These scaffolds were used to culture SCs in-vitro, and they enhanced peripheral nerve regeneration, stimulating cell proliferation and myelination-related gene expression. Furthermore, these scaffolds demonstrated similar regenerative properties of peripheral nerves in-vivo as autologous nerve grafting on a rat sciatic nerve defect model of a 10-mm length. (Li et al. (2022a) developed a sustained, stable release of MSC exosomes using PDA-modified chitin (Chi) conduits. The exosomes isolated from rat MSCs stimulated SCs to proliferate and reprogrammed them toward a repair phenotype in-vitro. A PDA-modified Chi conduit containing MSC-derived exosomes was found to augment neurite growth in the dorsal root ganglia of rats with sciatic nerve defects (Figure 6D). The anti-inflammatory activity of PDA was also illustrated in a study conducted by Yang et al. (2022) that modified a Chi-based nerve guidance conduit and showed its ability to inhibit neuromas during nerve regeneration. In addition, PDA promoted cell viability and protuberance expansion. Based on in-vivo trials, this scaffold facilitated sciatic nerve regeneration with complete biodegradability and displayed an identical amount of muscle fibers and myelin thickness compared to autografts.
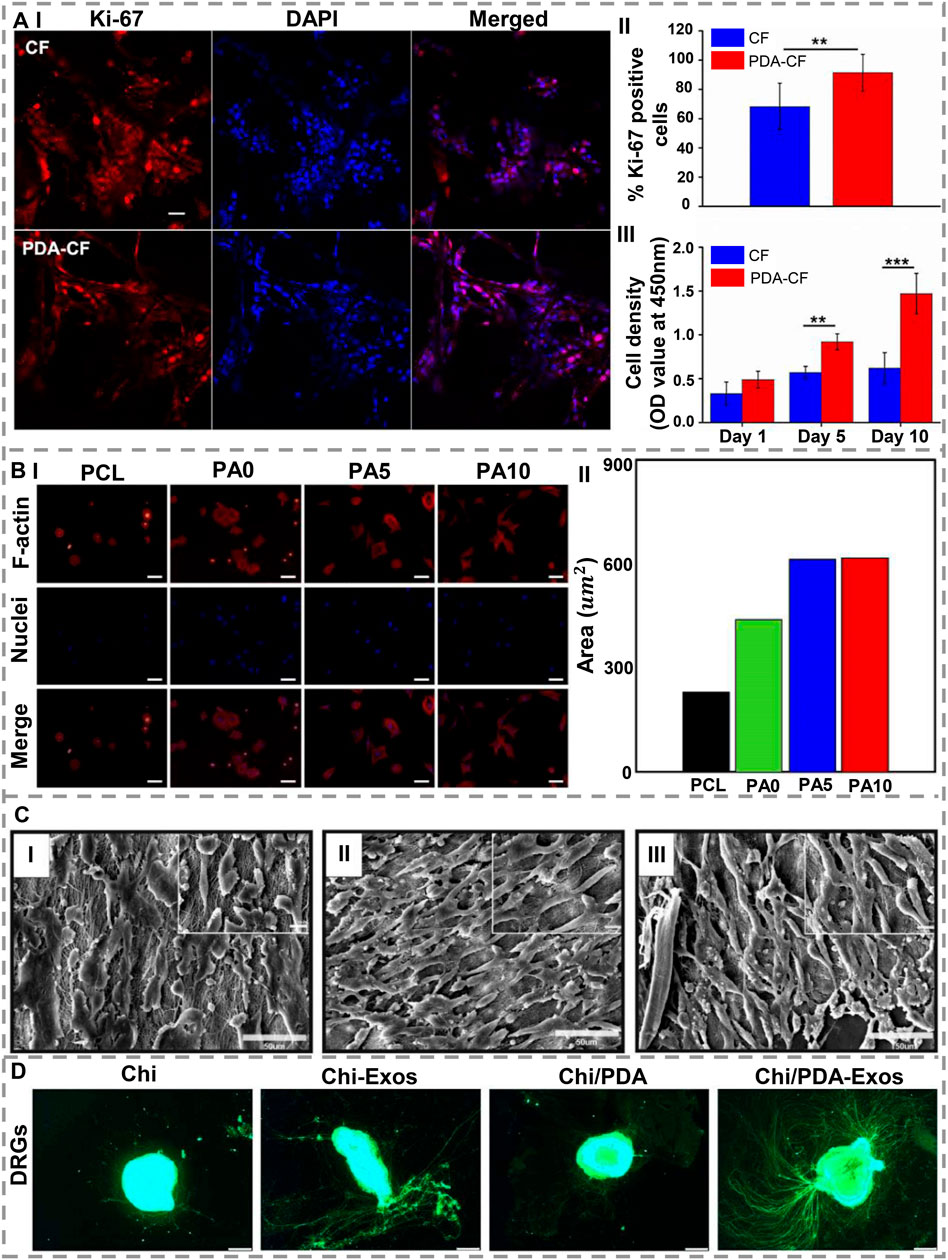
FIGURE 6. (A) (I) Confocal laser scanning microscopy with immunostained NSCs (Ki-67 and DAPI). (II) During the fifth day of culture, Ki-67-positive cells observed. (III) CCK-8 relative viability assay. Scale bar = 50 μm (**p < 0.005; ***p < 0.0005) (reproduced content is open access) [1. Reproduced under the terms of the Creative Commons Attribution 4.0 International (CC BY 4.0) license. (Yang et al., 2020) Copyright 2020, Frontiers]. (B) (I) Staining of nuclei (blue) and F-actin (red), and (II) the site quantification following 3 h seeding of RSCs on dECM/PDA-coated PCL scaffolds. Scale bar= 200 µm [2. Reproduced under the terms of the Creative Attribution 4.0 International (CC BY 4.0) license. (Chen et al., 2018b) Copyright 2018, MDPI]. (C). SEM images of PC12 cells on (I) PCL-Chitosan (Aligned) nanofibers; (II) PCL-Chitosan-PDA nanofibers; (III) PCL-Chitosan-PDA-NGF nanofibers. Nanofibers immobilized with NGF allowed cells to spread and grow longer [3. Reproduced under the terms of the Creative Commons Attribution 4.0 International (CC BY 4.0) license. (Afrash et al., 2021) Copyright 2021, AMG]. (D). After 7 days of treatment with Chi/PDA-Exos, neurite growth is enhanced in DRGs. As a result of the application of BMSC-Exos, DRG axons (green, Alexa Fluor 488) were measured with different conduits and their length was enhanced. In comparison with Chi alone, treatment with Chi/PDA-Exos significantly increased the length of the DRG axons. Scale bars = 50 μm [4. Reproduced under the terms of the Creative Commons Attribution-NonCommercial-ShareAlike (CC BY-NC-SA) license. (Li et al., 2022a) Copyright 2022, Wolters Kluwer Medknow].
VEGF and BDNF affect peripheral nerve regeneration by providing a supportive microenvironment (Lopes et al., 2017; Xia and Lv, 2018). The clinical benefit of these factors is limited due to rapid degradation in-vivo and obstructing axonal growth when used at supraphysiological doses. There has been recent development of bioactive peptides that mimic neurotrophic factors and can stimulate the equivalent receptors but have modified sequences and forms, making them a valuable substitute for the original growth factors (Lu et al., 2018; Rao et al., 2020). Li et al. (2022b) developed PDA-modified Chi conduits loaded with BDNF mimetic peptides and VEGF mimetic peptides and showed that adding PDA to this conduit caused higher biocompatibility and sustained release of peptides. In rat models of sciatic nerve injury, these conduits bridged a 2-mm gap among the nerve stumps. As a result of applying Chi/PDA-Ps conduits, rats were found to have improved motor function and less atrophy in their gastrocnemius muscles. A further restoration of nerve conduction function and remyelination was demonstrated by electrophysiological and microstructural results.
Tendon regeneration
Tendons have a unique composition of their ECM, in addition to being hypocellular and hypovascular, making them less capable of healing. Zhou Y. L. et al. (2021) found that growth factor-loaded PLGA nanoparticles adhered uniformly to PDA-modified suture surfaces and accelerated tendon repair. Even after being sutured into the tendon, the nanoparticles remained on the sutures, allowing proteins to penetrate the tendon tissues. Both chicken and rat tendon injury models have been studied in which basic fibroblast growth factor (bFGF)-releasing and VEGF-releasing sutures resulted in significantly higher tendon strength than those repaired with control sutures. In another interesting research on sutures for tendon regeneration, Zhou et al. (2019) coated nanoparticle/plasmid complex on PDA-modified sutures to transfer the growth factor genes into injured tendon tissues to promote healing. PDA-modified sutures can uniformly and tightly absorb nanoparticle/plasmid complexes and deliver and transfer them into tendon tissue to effectively transfect genes into tendon cells, significantly increasing the expression of growth factors in tendon tissues. As it showed in Figure 7A, the nanoparticle/plasmid complexes can detach from the modified sutures and spread out in tendon tissues after stitching and enhanced EGFP expression, while no EGFP-positive cells were observed in the unmodified sutures group. Figure 7B displays that in vivo experiments and histology of repaired tendons indicate that all of the repaired tendons had no significant inflammatory reactions or necrosis 6 weeks after surgery and indicate that these modified sutures are a promising tool for the treatment of injured tendons. As a result of modifying PDA and kartogenin carboxyl groups, Chen et al. (2021) prepared multi-functionalized nanofibrous scaffolds with amide bonds on the silk fibroin nanofibers’ surface. When used in the early stages of tissue repair, multifunctional nanofibers reduce oxidative stress and inflammation as well as inducing remodeling and regeneration. As a result of the ECM’s biological properties, the nanofiber scaffold also facilitated cell differentiation and tissue formation. Nanofibers modified with PDA and KGN enhanced fibrocartilage regeneration and improved integration of tendons and bones in-vivo, leading to good biomechanical function. Wu et al. (2021) used PDA nano-layers, silver, and nano-hydroxyapatites to eliminate antibiotics after surgery by binding to polyethylene terephthalate (PET) ligament’s biological inertness. As DA undergoes only a mild polymerization reaction, PET remains chemically and mechanically stable. NIH3T3 cell culture for fibroblast growth and staphylococcus aureus was used to test PET artificial ligament’s biocompatibility and antimicrobial effects.
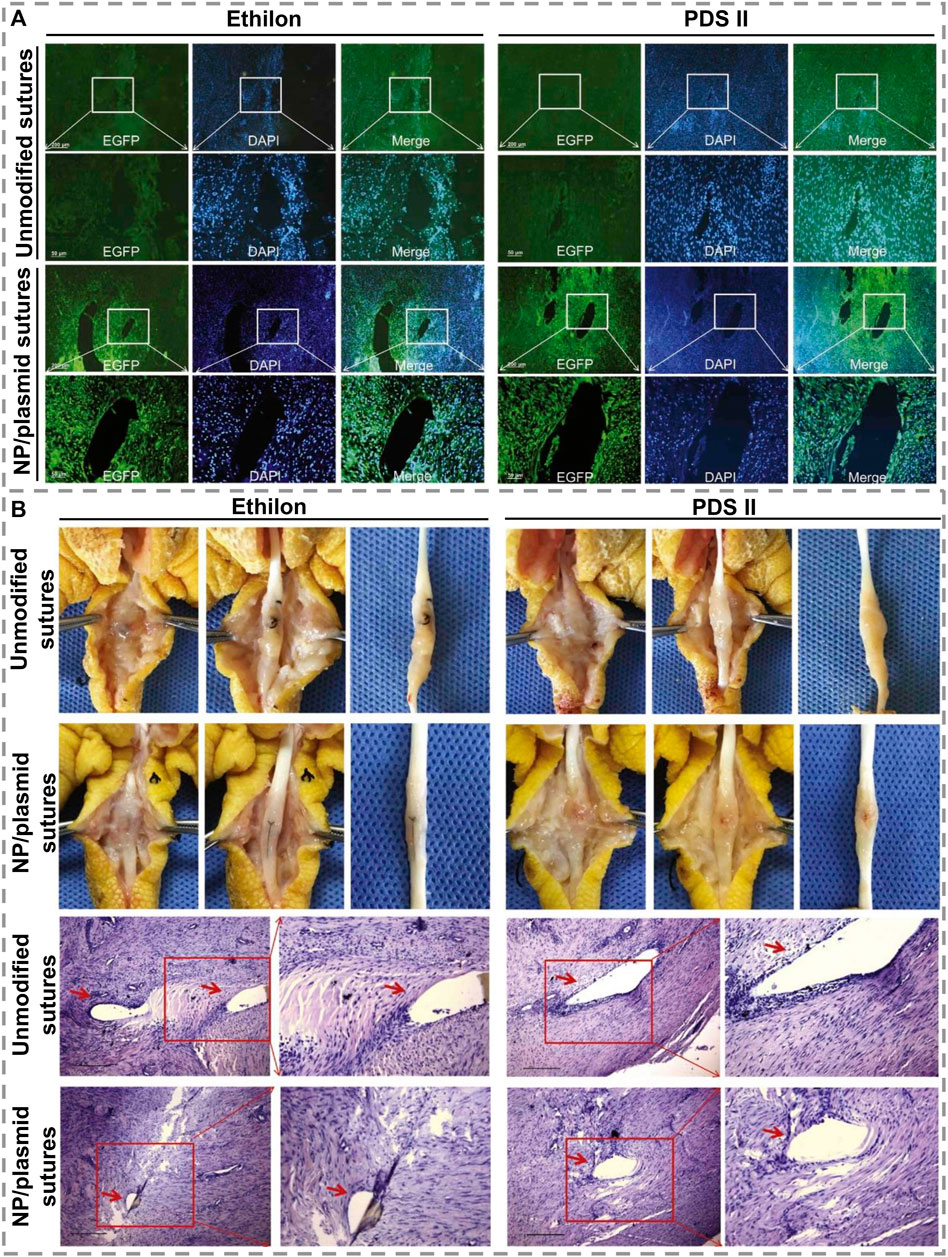
FIGURE 7. (A). Displaying EGFP expression images of modified and unmodified two kinds of sutures, Ethilon and PDS II, in the tendon tissues 1 week after surgery. Scale bars = 50 μm. (B). Images of adhesion morphology in the unmodified or modified sutures group 6 weeks after treatment and histology of tendons treated with modified sutures. Scale bars = 100 μm [Reproduced under the terms of the Creative Commons Attribution-NonCommercial-NoDerivatives 4.0 International (CC BY-NC-ND 4.0) license. (Zhou et al., 2019) Copyright 2019, Elsevier].
Perspective summary
PDA has excellent biocompatibility, hydrophilicity, and adhesion properties. Therefore, this technology has been widely used in a variety of fields and is expected to be further applied in TE and regenerative medicine. PDA can easily be applied to different biomaterials and enhance their biological and mechanical properties using the polymerization process. Over the past few years, PDA has been successfully applied in musculoskeletal TE. It has been demonstrated that surface modification of scaffolds by PDA is an effective method for controlling the behavior of cells, which is based on the super-hydrophilic properties imparted to the scaffold surface which serves as a cell carrier for musculoskeletal TE.
Despite extensive research on PDA, only a few investigations have studied the impacts of PDA on musculoskeletal tissue engineering and its structure and polymerization process remain little understood and should be further investigated. It would also be beneficial to study the mechanisms of PDA coating and the immobilization of growth factors to PDA for improving tissue regeneration. Most of the current research on PDA-modified scaffolds and their impact on tissue regeneration is conducted through in-vitro and in-vivo experiments, many experimental outcomes have confirm that PDA plays a significant role in this domain. There is a need for further research to better understand the underlying mechanisms of PDA-cell interactions and their functions in-vivo, as well as to identify the requirements for future experiments to clarify the role of PDA in clinical practice.
Author contributions
HT and NB: Conceptualization, Methodology, Investigation, Visualization, Writing—original draft, Writing—review and editing. SS: Conceptualization, Methodology, Investigation, Writing—original draft. MT: Conceptualization, Resources, Review and editing, Supervision. FG: Conceptualization, Resources, Review and editing, Supervision. AB: Conceptualization, Resources, Review and editing, Supervision. CL: Conceptualization, Resources, Review and editing, Supervision, Funding acquisition.
Funding
This work was financially supported by the MRC - UCL Therapeutic Acceleration Support (TAS) Fund (project no: 564022 - linked to Lead 559846); NIHR UCLH BRC- UCL Therapeutic Acceleration Support (TAS) Fund (project no 564021 - linked to Lead 557595); Engineering and Physical Sciences Research Council via DTP CASE Programme (Grant no: EP/T517793/1); Wellcome Trust - Translational Partnership Award - UCL Regenerative Medicine TIN Pilot Dara Fund (Project no: 569576 (linked to Lead 553191)); Bavarian Funding Programme for the Initiation of International Projects-Bayerische Forschungsallianz; and Alexander von Humboldt Foundation.
Conflict of interest
The authors declare that the research was conducted in the absence of any commercial or financial relationships that could be construed as a potential conflict of interest.
Publisher’s note
All claims expressed in this article are solely those of the authors and do not necessarily represent those of their affiliated organizations, or those of the publisher, the editors and the reviewers. Any product that may be evaluated in this article, or claim that may be made by its manufacturer, is not guaranteed or endorsed by the publisher.
References
Afrash, H., Nazeri, N., Davoudi, P., and Majidi, R. F. (2021). Hossein ghanbari, development of a bioactive scaffold based on NGF containing PCL/chitosan nanofibers for nerve regeneration. Biointerface Res. Appl. Chem. 11, 12606–12617. doi:10.33263/BRIAC115.1260612617
Ahmad, T., Byun, H., Lee, J., Madhurakat Perikamana, S. K., Shin, Y. M., Kim, E. M., et al. (2020). Stem cell spheroids incorporating fibers coated with adenosine and polydopamine as a modular building blocks for bone tissue engineering. Biomaterials 230, 119652. doi:10.1016/j.biomaterials.2019.119652
Alfieri, M. L., Weil, T., Ng, D. Y. W., and Ball, V. (2022). Polydopamine at biological interfaces. Adv. Colloid Interface Sci. 305, 102689. doi:10.1016/j.cis.2022.102689
Ambekar, R. S., and Kandasubramanian, B. (2019). A polydopamine-based platform for anti-cancer drug delivery. Biomater. Sci. 7, 1776–1793. doi:10.1039/C8BM01642A
Arab-Ahmadi, S., Irani, S., Bakhshi, H., Atyabi, F., and Ghalandari, B. (2021). Immobilization of carboxymethyl chitosan/laponite on polycaprolactone nanofibers as osteoinductive bone scaffolds. Polym. Adv. Technol. 32, 755–765. doi:10.1002/pat.5128
Bakhtiary, N., Liu, C., and Ghorbani, F. (2021). Bioactive inks development for osteochondral tissue engineering: A mini-review. Gels 7, 274. doi:10.3390/gels7040274
Barros, N. R., Chen, Y., Hosseini, V., Wang, W., Nasiri, R., Mahmoodi, M., et al. (2021). Recent developments in mussel-inspired materials for biomedical applications. Biomater. Sci. 9, 6653–6672. doi:10.1039/D1BM01126J
Bhang, S. H., Kwon, S.-H., Lee, S., Kim, G. C., Han, A. M., Kwon, Y. H. K., et al. (2013). Enhanced neuronal differentiation of pheochromocytoma 12 cells on polydopamine-modified surface. Biochem. Biophys. Res. Commun. 430, 1294–1300. doi:10.1016/j.bbrc.2012.11.123
Cai, Y., Li, J., Poh, C. K., Tan, H. C., San Thian, E., Hsi Fuh, J. Y., et al. (2013). Collagen grafted 3D polycaprolactone scaffolds for enhanced cartilage regeneration. J. Mat. Chem. B 1, 5971. doi:10.1039/c3tb20680g
Chen, C.-C., Yu, J., Ng, H.-Y., Lee, A., Chen, C.-C., Chen, Y.-S., et al. (2018). The physicochemical properties of decellularized extracellular matrix-coated 3D printed poly(ε-caprolactone) nerve conduits for promoting Schwann cells proliferation and differentiation. Mater. (Basel) 11, 1665. doi:10.3390/ma11091665
Chen, P., Wang, S., Huang, Z., Gao, Y., Zhang, Y., Wang, C., et al. (2021). Multi-functionalized nanofibers with reactive oxygen species scavenging capability and fibrocartilage inductivity for tendon-bone integration. J. Mat. Sci. Technol. 70, 91–104. doi:10.1016/j.jmst.2020.09.006
Chen, X., Wu, Y., Ranjan, V. D., and Zhang, Y. (2018). Three-dimensional electrical conductive scaffold from biomaterial-based carbon microfiber sponge with bioinspired coating for cell proliferation and differentiation. Carbon N. Y. 134, 174–182. doi:10.1016/j.carbon.2018.03.064
Cheng, W., Zeng, X., Chen, H., Li, Z., Zeng, W., Mei, L., et al. (2019). Versatile polydopamine platforms: Synthesis and promising applications for surface modification and advanced nanomedicine. ACS Nano 13, 8537–8565. doi:10.1021/acsnano.9b04436
Chien, C.-Y., Liu, T.-Y., Kuo, W.-H., Wang, M.-J., and Tsai, W.-B. (2013). Dopamine-assisted immobilization of hydroxyapatite nanoparticles and RGD peptides to improve the osteoconductivity of titanium. J. Biomed. Mat. Res. A 101A, 740–747. doi:10.1002/jbm.a.34376
Chien, C.-Y., and Tsai, W.-B. (2013). Poly(dopamine)-Assisted immobilization of arg-gly-asp peptides, hydroxyapatite, and bone morphogenic protein-2 on titanium to improve the osteogenesis of bone marrow stem cells. ACS Appl. Mat. Interfaces 5, 6975–6983. doi:10.1021/am401071f
Cho, H., Madhurakkat Perikamana, S. K., Lee, J., Lee, J., Lee, K.-M., Shin, C. S., et al. (2014). Effective immobilization of BMP-2 mediated by polydopamine coating on biodegradable nanofibers for enhanced in vivo bone formation. ACS Appl. Mat. Interfaces 6, 11225–11235. doi:10.1021/am501391z
Cui, M., Ren, S., Zhao, H., Xue, Q., and Wang, L. (2018). Polydopamine coated graphene oxide for anticorrosive reinforcement of water-borne epoxy coating. Chem. Eng. J. 335, 255–266. doi:10.1016/j.cej.2017.10.172
Davari, N., Bakhtiary, N., Khajehmohammadi, M., Sarkari, S., Tolabi, H., Ghorbani, F., et al. (2022). Protein-based hydrogels: Promising materials for tissue engineering. Polym. (Basel) 14, 986. doi:10.3390/polym14050986
Ding, G., Li, X., Sun, M., He, Y., Zhao, F., Wu, T., et al. (2022). Meniscal transplantation and regeneration using functionalized polyurethane bionic scaffold and digital light processing 3D printing. Chem. Eng. J. 431, 133861. doi:10.1016/j.cej.2021.133861
Feng, P., Liu, M., Peng, S., Bin, S., Zhao, Z., and Shuai, C. (2021). Polydopamine modified polycaprolactone powder for fabrication bone scaffold owing intrinsic bioactivity. J. Mat. Res. Technol. 15, 3375–3385. doi:10.1016/j.jmrt.2021.09.137
Gan, D., Jiang, Y., Hu, Y., Wang, X., Wang, Q., Wang, K., et al. (2022). Mussel-inspired extracellular matrix-mimicking hydrogel scaffold with high cell affinity and immunomodulation ability for growth factor-free cartilage regeneration. J. Orthop. Transl. 33, 120–131. doi:10.1016/j.jot.2022.02.006
Gao, B., Chen, L., Zhao, Y., Yan, X., Wang, X., Zhou, C., et al. (2019). Methods to prepare dopamine/polydopamine modified alginate hydrogels and their special improved properties for drug delivery. Eur. Polym. J. 110, 192–201. doi:10.1016/j.eurpolymj.2018.11.025
Gao, C., Peng, S., Feng, P., and Shuai, C. (2017). Bone biomaterials and interactions with stem cells. Bone Res. 5, 17059. doi:10.1038/boneres.2017.59
Gao, C., Wang, Y., Han, F., Yuan, Z., Li, Q., Shi, C., et al. (2017). Antibacterial activity and osseointegration of silver-coated poly(ether ether ketone) prepared using the polydopamine-assisted deposition technique. J. Mat. Chem. B 5, 9326–9336. doi:10.1039/C7TB02436C
Gao, F., Zeng, D., Liu, H., Qin, R., Zhang, J., Chen, Y., et al. (2022). Porous cellulose microspheres coated in one step with a polydopamine suspension of hydroxyapatite for bone tissue engineering. Cellulose 29, 1955–1967. doi:10.1007/s10570-021-04395-4
Ghalandari, B., Yu, Y., Ghorbani, F., Warden, A. R., Ahmad, K. Z., Sang, X., et al. (2021). Polydopamine nanospheres coated with bovine serum albumin permit enhanced cell differentiation: Fundamental mechanism and practical application for protein coating formation. Nanoscale 13, 20098–20110. doi:10.1039/D1NR07469E
Ghorbani, F., Ghalandari, B., Khan, A. L., Li, D., Zamanian, A., and Yu, B. (2020). Decoration of electrical conductive polyurethane-polyaniline/polyvinyl alcohol matrixes with mussel-inspired polydopamine for bone tissue engineering. Biotechnol. Prog. 36, e3043. doi:10.1002/btpr.3043
Ghorbani, F., Ghalandari, B., and Liu, C. (2021). A facile method to synthesize 3D pomegranate-like polydopamine microspheres. Front. Bioeng. Biotechnol. 9, 737074. doi:10.3389/fbioe.2021.737074
Ghorbani, F., Kim, M., Monavari, M., Ghalandari, B., and Boccaccini, A. R. (2022). Mussel-inspired polydopamine decorated alginate dialdehyde-gelatin 3D printed scaffolds for bone tissue engineering application. Front. Bioeng. Biotechnol. 10, 940070. doi:10.3389/fbioe.2022.940070
Ghorbani, F., Zamanian, A., and Aidun, A. (2019). Bioinspired polydopamine coating-assisted electrospun polyurethane-graphene oxide nanofibers for bone tissue engineering application. J. Appl. Polym. Sci. 136, 47656. doi:10.1002/app.47656
Ghorbani, F., Zamanian, A., Behnamghader, A., and Daliri-Joupari, M. (2019). Bone-like hydroxyapatite mineralization on the bio-inspired PDA nanoparticles using microwave irradiation. Surfaces Interfaces 15, 38–42. doi:10.1016/j.surfin.2019.01.007
Ghorbani, F., Zamanian, A., Behnamghader, A., and Joupari, M. D. (2019). A facile method to synthesize mussel-inspired polydopamine nanospheres as an active template for in situ formation of biomimetic hydroxyapatite. Mater. Sci. Eng. C 94, 729–739. doi:10.1016/j.msec.2018.10.010
Ghorbani, F., Zamanian, A., and Sahranavard, M. (2020). Mussel-inspired polydopamine-mediated surface modification of freeze-cast poly (ε-caprolactone) scaffolds for bone tissue engineering applications. Biomed. Eng./Biomed. Tech. 65, 273–287. doi:10.1515/bmt-2019-0061
Ghorbani, F., Zamanian, A., and Torabinejad, B. (2020). The effect of oxygen plasma pretreatment on the properties of mussel-inspired polydopamine-decorated polyurethane nanofibers. J. Polym. Eng. 40, 109–119. doi:10.1515/polyeng-2019-0219
Habibi Rad, M., Zamanian, A., Hadavi, S. M. M., and Khanlarkhani, A. (2018). A two-stage kinetics model for polydopamine layer growth. Macromol. Chem. Phys. 219, 1700505. doi:10.1002/macp.201700505
Han, L., Lu, X., Liu, K., Wang, K., Fang, L., Weng, L.-T., et al. (2017). Mussel-inspired adhesive and tough hydrogel based on nanoclay confined dopamine polymerization. ACS Nano 11, 2561–2574. doi:10.1021/acsnano.6b05318
Hauser, D., Septiadi, D., Turner, J., Petri-Fink, A., and Rothen-Rutishauser, B. (2020). From bioinspired glue to medicine: Polydopamine as a biomedical material. Materials 13, 1730. doi:10.3390/ma13071730
Heid, S., Becker, K., Byun, J., Biermann, I., Neščáková, Z., Zhu, H., et al. (2022). Bioprinting with bioactive alginate dialdehyde-gelatin (ADA-GEL) composite bioinks: Time-dependent in-situ crosslinking via addition of calcium-silicate particles tunes in vitro stability of 3D bioprinted constructs. Bioprinting 26, e00200. doi:10.1016/j.bprint.2022.e00200
Hong, S., Kim, K. Y., Wook, H. J., Park, S. Y., Lee, K. D., Lee, D. Y., et al. (2011). Attenuation of the in vivo toxicity of biomaterials by polydopamine surface modification. Nanomedicine 6, 793–801. doi:10.2217/nnm.11.76
Huang, S., Liang, N., Hu, Y., Zhou, X., and Abidi, N. (2016). Polydopamine-assisted surface modification for bone biosubstitutes. Biomed. Res. Int. 2016, 1–9. doi:10.1155/2016/2389895
Jia, L., Han, F., Wang, H., Zhu, C., Guo, Q., Li, J., et al. (2019). Polydopamine-assisted surface modification for orthopaedic implants. J. Orthop. Transl. 17, 82–95. doi:10.1016/j.jot.2019.04.001
Jun, D.-R., Moon, S.-K., and Choi, S.-W. (2014). Uniform polydimethylsiloxane beads coated with polydopamine and their potential biomedical applications. Colloids Surfaces B Biointerfaces 121, 395–399. doi:10.1016/j.colsurfb.2014.06.027
Kang, S. M., Rho, J., Choi, I. S., Messersmith, P. B., and Lee, H. (2009). Norepinephrine: Material-Independent, multifunctional surface modification reagent. J. Am. Chem. Soc. 131, 13224–13225. doi:10.1021/ja905183k
Kao, C.-T., Lin, C.-C., Chen, Y.-W., Yeh, C.-H., Fang, H.-Y., and Shie, M.-Y. (2015). Poly(dopamine) coating of 3D printed poly(lactic acid) scaffolds for bone tissue engineering. Mater. Sci. Eng. C 56, 165–173. doi:10.1016/j.msec.2015.06.028
Kessler, M. W., Ackerman, G., Dines, J. S., and Grande, D. (2008). Emerging technologies and fourth generation issues in cartilage repair. Sports Med. Arthrosc. Rev. 16, 246–254. doi:10.1097/JSA.0b013e31818d56b3
Khetani, S., Yong, K. W., Ozhukil Kollath, V., Eastick, E., Azarmanesh, M., Karan, K., et al. (2020). Engineering shelf-stable coating for microfluidic organ-on-a-chip using bioinspired catecholamine polymers. ACS Appl. Mat. Interfaces 12, 6910–6923. doi:10.1021/acsami.9b20826
Ko, E., Yang, K., Shin, J., and Cho, S.-W. (2013). Polydopamine-assisted osteoinductive peptide immobilization of polymer scaffolds for enhanced bone regeneration by human adipose-derived stem cells. Biomacromolecules 14, 3202–3213. doi:10.1021/bm4008343
Ku, S. H., and Park, C. B. (2013). Combined effect of mussel-inspired surface modification and topographical cues on the behavior of skeletal myoblasts. Adv. Healthc. Mat. 2, 1445–1450. doi:10.1002/adhm.201300067
Lai, Y.-H., Chen, Y.-H., Pal, A., Chou, S.-H., Chang, S.-J., Huang, E.-W., et al. (2021). Regulation of cell differentiation via synergistic self-powered stimulation and degradation behavior of a biodegradable composite piezoelectric scaffold for cartilage tissue. Nano Energy 90, 106545. doi:10.1016/j.nanoen.2021.106545
Lee, H., Dellatore, S. M., Miller, W. M., and Messersmith, P. B. (2007). Mussel-inspired surface chemistry for multifunctional coatings. Science 318, 426–430. doi:10.1126/science.1147241
Lee, M., Lee, S.-H., Oh, I.-K., and Lee, H. (2017). Microwave-accelerated rapid, chemical oxidant-free, material-independent surface chemistry of poly(dopamine). Small 13, 1600443. doi:10.1002/smll.201600443
Lee, S. J., Lee, D., Yoon, T. R., Kim, H. K., Jo, H. H., Park, J. S., et al. (2016). Surface modification of 3D-printed porous scaffolds via mussel-inspired polydopamine and effective immobilization of rhBMP-2 to promote osteogenic differentiation for bone tissue engineering. Acta Biomater. 40, 182–191. doi:10.1016/j.actbio.2016.02.006
Li, C., Liu, S.-Y., Zhang, M., Pi, W., Wang, B., Li, Q.-C., et al. (2022). Sustained release of exosomes loaded into polydopamine-modified chitin conduits promotes peripheral nerve regeneration in rats. Neural Regen. Res. 17, 2050. doi:10.4103/1673-5374.335167
Li, C., Liu, S.-Y., Zhou, L.-P., Min, T.-T., Zhang, M., Pi, W., et al. (2022). Polydopamine-modified chitin conduits with sustained release of bioactive peptides enhance peripheral nerve regeneration in rats. Neural Regen. Res. 17, 2544. doi:10.4103/1673-5374.339006
Li, J., Cao, F., Wu, B., Yang, J., Xu, W., Wang, W., et al. (2021). Immobilization of bioactive vascular endothelial growth factor onto Ca-deficient hydroxyapatite-coated Mg by covalent bonding using polydopamine. J. Orthop. Transl. 30, 82–92. doi:10.1016/j.jot.2021.06.002
Liebscher, J. (2019). Chemistry of polydopamine – scope, variation, and limitation. Eur. J. Org. Chem. 2019, 4976–4994. doi:10.1002/ejoc.201900445
Linderman, S. W., Gelberman, R. H., Thomopoulos, S., and Shen, H. (2016). Cell and biologic-based treatment of flexor tendon injuries. Oper. Tech. Orthop. 26, 206–215. doi:10.1053/j.oto.2016.06.011
Liu, Y., Ai, K., and Lu, L. (2014). Polydopamine and its derivative materials: Synthesis and promising applications in energy, environmental, and biomedical fields. Chem. Rev. 114, 5057–5115. doi:10.1021/cr400407a
Lopes, C. D. F., Gonçalves, N. P., Gomes, C. P., Saraiva, M. J., and Pêgo, A. P. (2017). BDNF gene delivery mediated by neuron-targeted nanoparticles is neuroprotective in peripheral nerve injury. Biomaterials 121, 83–96. doi:10.1016/j.biomaterials.2016.12.025
Lu, C., Wang, Y., Yang, S., Wang, C., Sun, X., Lu, J., et al. (2018). Bioactive self-assembling peptide hydrogels functionalized with brain-derived neurotrophic factor and nerve growth factor mimicking peptides synergistically promote peripheral nerve regeneration. ACS Biomater. Sci. Eng. 4, 2994–3005. doi:10.1021/acsbiomaterials.8b00536
Luo, Y., Cao, X., Chen, J., Gu, J., Yu, H., Sun, J., et al. (2022). Platelet-derived growth factor-functionalized scaffolds for the recruitment of synovial mesenchymal stem cells for osteochondral repair. Stem Cells Int. 2022, 1–15. doi:10.1155/2022/2190447
Lynge, M. E., van der Westen, R., Postma, A., and Städler, B. (2011). Polydopamine—A nature-inspired polymer coating for biomedical science. Nanoscale 3, 4916. doi:10.1039/c1nr10969c
Madhurakkat Perikamana, S. K., Lee, J., Ahmad, T., Kim, E. M., Byun, H., Lee, S., et al. (2018). Harnessing biochemical and structural cues for tenogenic differentiation of adipose derived stem cells (ADSCs) and development of an in vitro tissue interface mimicking tendon-bone insertion graft. Biomaterials 165, 79–93. doi:10.1016/j.biomaterials.2018.02.046
Madhurakkat Perikamana, S. K., Lee, J., Bin Lee, Y., Shin, Y. M., Lee, E. J., Mikos, A. G., et al. (2015). Materials from mussel-inspired chemistry for cell and tissue engineering applications. Biomacromolecules 16, 2541–2555. doi:10.1021/acs.biomac.5b00852
Miller, D. J., Dreyer, D. R., Bielawski, C. W., Paul, D. R., and Freeman, B. D. (2017). Surface modification of water purification membranes. Angew. Chem. Int. Ed. 56, 4662–4711. doi:10.1002/anie.201601509
Naghieh, S., Lindberg, G., Tamaddon, M., and Liu, C. (2021). Biofabrication strategies for musculoskeletal disorders: Evolution towards clinical applications. Bioengineering 8, 123. doi:10.3390/bioengineering8090123
Nazeri, N., Karimi, R., and Ghanbari, H. (2021). The effect of surface modification of poly-lactide- co -glycolide/carbon nanotube nanofibrous scaffolds by laminin protein on nerve tissue engineering. J. Biomed. Mat. Res. A 109, 159–169. doi:10.1002/jbm.a.37013
Nijhuis, A. W. G., van den Beucken, J. J. J. P., Boerman, O. C., Jansen, J. A., and Leeuwenburgh, S. C. G. (2013). 1-Step versus 2-step immobilization of alkaline phosphatase and bone morphogenetic protein-2 onto implant surfaces using polydopamine. Tissue Eng. Part C. Methods 19, 610–619. doi:10.1089/ten.tec.2012.0313
Orlacchio, R., Zuppolini, S., Cruz-Maya, I., Pragliola, S., Borriello, A., Guarino, V., et al. (2022). Polydopamine-coated poly-lactic acid aerogels as scaffolds for tissue engineering applications. Molecules 27, 2137. doi:10.3390/molecules27072137
Park, J., Lee, S. J., Jung, T. G., Lee, J. H., Kim, W. D., Lee, J. Y., et al. (2021). Surface modification of a three-dimensional polycaprolactone scaffold by polydopamine, biomineralization, and BMP-2 immobilization for potential bone tissue applications. Colloids Surfaces B Biointerfaces 199, 111528. doi:10.1016/j.colsurfb.2020.111528
Pi, W., Zhang, Y., Li, L., Li, C., Zhang, M., Zhang, W., et al. (2022). Polydopamine-coated polycaprolactone/carbon nanotube fibrous scaffolds loaded with brain-derived neurotrophic factor for peripheral nerve regeneration. Biofabrication 14, 035006. doi:10.1088/1758-5090/ac57a6
Rao, F., Wang, Y., Zhang, D., Lu, C., Cao, Z., Sui, J., et al. (2020). Aligned chitosan nanofiber hydrogel grafted with peptides mimicking bioactive brain-derived neurotrophic factor and vascular endothelial growth factor repair long-distance sciatic nerve defects in rats. Theranostics 10, 1590–1603. doi:10.7150/thno.36272
Ren, X., Li, J., Li, J., Jiang, Y., Li, L., Yao, Q., et al. (2019). Aligned porous fibrous membrane with a biomimetic surface to accelerate cartilage regeneration. Chem. Eng. J. 370, 1027–1038. doi:10.1016/j.cej.2019.03.271
Rezaei, H., Shahrezaee, M., Jalali Monfared, M., Ghorbani, F., Zamanian, A., and Sahebalzamani, M. (2021). Mussel-inspired polydopamine induced the osteoinductivity to ice-templating PLGA–gelatin matrix for bone tissue engineering application. Biotechnol. Appl. Biochem. 68, 185–196. doi:10.1002/bab.1911
Rim, N. G., Kim, S. J., Shin, Y. M., Jun, I., Lim, D. W., Park, J. H., et al. (2012). Mussel-inspired surface modification of poly(l-lactide) electrospun fibers for modulation of osteogenic differentiation of human mesenchymal stem cells. Colloids Surfaces B Biointerfaces 91, 189–197. doi:10.1016/j.colsurfb.2011.10.057
Ruiz-Alonso, S., Lafuente-Merchan, M., Ciriza, J., Saenz-del-Burgo, L., and Pedraz, J. L. (2021). Tendon tissue engineering: Cells, growth factors, scaffolds and production techniques. J. Control. Release 333, 448–486. doi:10.1016/j.jconrel.2021.03.040
Ryu, J. H., Messersmith, P. B., and Lee, H. (2018). Polydopamine surface chemistry: A decade of discovery. ACS Appl. Mat. Interfaces 10, 7523–7540. doi:10.1021/acsami.7b19865
Sarkari, S., Khajehmohammadi, M., Davari, N., Li, D., and Yu, B. (2022). The effects of process parameters on polydopamine coatings employed in tissue engineering applications. Front. Bioeng. Biotechnol. 10, 1005413. doi:10.3389/fbioe.2022.1005413
Sengottuvelan, A., Balasubramanian, P., Will, J., and Boccaccini, A. R. (2017). Bioactivation of titanium dioxide scaffolds by ALP-functionalization. Bioact. Mat. 2, 108–115. doi:10.1016/j.bioactmat.2017.02.004
Shen, J., Shi, D., Dong, L., Zhang, Z., Li, X., and Chen, M. (2018). Fabrication of polydopamine nanoparticles knotted alginate scaffolds and their properties. J. Biomed. Mat. Res. A 106, 3255–3266. doi:10.1002/jbm.a.36524
Shokrollahi, M., Bahrami, S. H., Nazarpak, M. H., and Solouk, A. (2020). Biomimetic double-sided polypropylene mesh modified by DOPA and ofloxacin loaded carboxyethyl chitosan/polyvinyl alcohol-polycaprolactone nanofibers for potential hernia repair applications. Int. J. Biol. Macromol. 165, 902–917. doi:10.1016/j.ijbiomac.2020.09.229
Singh, I., Dhawan, G., Gupta, S., and Kumar, P. (2021). Recent advances in a polydopamine-mediated antimicrobial adhesion system. Front. Microbiol. 11, 607099. doi:10.3389/fmicb.2020.607099
Tan, S., Gu, J., Han, S. C., Lee, D.-W., and Kang, K. (2018). Design and fabrication of a non-clogging scaffold composed of semi-permeable membrane. Mat. Des. 142, 229–239. doi:10.1016/j.matdes.2018.01.033
Tang, X., Saveh-Shemshaki, N., Kan, H.-M., Khan, Y., and Laurencin, C. T. (2020). Biomimetic electroconductive nanofibrous matrices for skeletal muscle regenerative engineering. Regen. Eng. Transl. Med. 6, 228–237. doi:10.1007/s40883-019-00136-z
Tejido-Rastrilla, R., Ferraris, S., Goldmann, W. H., Grünewald, A., Detsch, R., Baldi, G., et al. (2019). Studies on cell compatibility, antibacterial behavior, and zeta potential of Ag-containing polydopamine-coated bioactive glass-ceramic. Mater. (Basel) 12, 500. doi:10.3390/ma12030500
Tsai, W.-B., Chen, W.-T., Chien, H.-W., Kuo, W.-H., and Wang, M.-J. (2011). Poly(dopamine) coating of scaffolds for articular cartilage tissue engineering. Acta Biomater. 7, 4187–4194. doi:10.1016/j.actbio.2011.07.024
van der Leeden, M. C. (2005). Are conformational changes, induced by osmotic pressure variations, the underlying mechanism of controlling the adhesive activity of mussel adhesive proteins? Langmuir 21, 11373–11379. doi:10.1021/la0515468
van der Westen, R., Hosta-Rigau, L., Sutherland, D. S., Goldie, K. N., Albericio, F., Postma, A., et al. (2012). Myoblast cell interaction with polydopamine coated liposomes. Biointerphases 7, 8. doi:10.1007/s13758-011-0008-4
Wang, Z.-G., Wang, Y., Huang, Y., Lu, Q., Zheng, L., Hu, D., et al. (2015). bFGF regulates autophagy and ubiquitinated protein accumulation induced by myocardial ischemia/reperfusion via the activation of the PI3K/Akt/mTOR pathway. Sci. Rep. 5, 9287. doi:10.1038/srep09287
Wang, Z., Chen, L., Wang, Y., Chen, X., and Zhang, P. (2016). Improved cell adhesion and osteogenesis of op-HA/PLGA composite by poly(dopamine)-assisted immobilization of collagen mimetic peptide and osteogenic growth peptide. ACS Appl. Mat. Interfaces 8, 26559–26569. doi:10.1021/acsami.6b08733
Wang, Z., Le, H., Wang, Y., Liu, H., Li, Z., Yang, X., et al. (2022). Instructive cartilage regeneration modalities with advanced therapeutic implantations under abnormal conditions. Bioact. Mat. 11, 317–338. doi:10.1016/j.bioactmat.2021.10.002
Wei, P., Xu, Y., Zhang, H., and Wang, L. (2021). Continued sustained insulin-releasing PLGA nanoparticles modified 3D-Printed PCL composite scaffolds for osteochondral repair. Chem. Eng. J. 422, 130051. doi:10.1016/j.cej.2021.130051
WHO (2021). Musculoskeletal conditions. Available at: https://www.who.int/news-room/fact-sheets/detail/musculoskeletal-conditions#:∼:text=Musculoskeletal%20conditions%20are%20typically%20characterized,form%20of%20non%2Dcancer%20pain.
Wu, Y., Zhang, Y., Zhang, R., and Chen, S. (2021). Preparation and properties of antibacterial polydopamine and nano-hydroxyapatite modified polyethylene terephthalate artificial ligament. Front. Bioeng. Biotechnol. 9, 630745. doi:10.3389/fbioe.2021.630745
Xia, B., and Lv, Y. (2018). Dual-delivery of VEGF and NGF by emulsion electrospun nanofibrous scaffold for peripheral nerve regeneration. Mater. Sci. Eng. C 82, 253–264. doi:10.1016/j.msec.2017.08.030
Xing, Y., Zhang, J., Chen, F., Liu, J., and Cai, K. (2017). Mesoporous polydopamine nanoparticles with co-delivery function for overcoming multidrug resistance via synergistic chemo-photothermal therapy. Nanoscale 9, 8781–8790. doi:10.1039/C7NR01857F
Xiong, F., Wei, S., Sheng, H., Han, X., Jiang, W., Zhang, Z., et al. (2022). In situ polydopamine functionalized poly-L-lactic acid nanofibers with near-infrared-triggered antibacterial and reactive oxygen species scavenging capability. Int. J. Biol. Macromol. 201, 338–350. doi:10.1016/j.ijbiomac.2022.01.024
Xu, Z., Wang, N., Liu, P., Sun, Y., Wang, Y., Fei, F., et al. (2019). Poly(Dopamine) coating on 3D-printed poly-lactic-Co-glycolic acid/β-tricalcium phosphate scaffolds for bone tissue engineering. Molecules 24, 4397. doi:10.3390/molecules24234397
Xue, G., Zhang, Y., Xie, T., Zhang, Z., Liu, Q., Li, X., et al. (2021). Cell adhesion-mediated piezoelectric self-stimulation on polydopamine-modified poly(vinylidene fluoride) membranes. ACS Appl. Mat. Interfaces 13, 17361–17371. doi:10.1021/acsami.1c02457
Yan, J., Wu, R., Liao, S., Jiang, M., and Qian, Y. (2020). Applications of polydopamine-modified scaffolds in the peripheral nerve tissue engineering. Front. Bioeng. Biotechnol. 8, 590998. doi:10.3389/fbioe.2020.590998
Yang, D., Xiao, J., Wang, B., Li, L., Kong, X., and Liao, J. (2019). The immune reaction and degradation fate of scaffold in cartilage/bone tissue engineering. Mater. Sci. Eng. C 104, 109927. doi:10.1016/j.msec.2019.109927
Yang, K., Lee, J. S., Kim, J., Bin Lee, Y., Shin, H., Um, S. H., et al. (2012). Polydopamine-mediated surface modification of scaffold materials for human neural stem cell engineering. Biomaterials 33, 6952–6964. doi:10.1016/j.biomaterials.2012.06.067
Yang, X., Huang, L., Yi, X., Huang, S., Duan, B., and Yu, A. (2022). Multifunctional chitin-based hollow nerve conduit for peripheral nerve regeneration and neuroma inhibition. Carbohydr. Polym. 289, 119443. doi:10.1016/j.carbpol.2022.119443
Yang, Y., Zhang, Y., Chai, R., and Gu, Z. (2020). A polydopamine-functionalized carbon microfibrous scaffold accelerates the development of neural stem cells. Front. Bioeng. Biotechnol. 8, 616. doi:10.3389/fbioe.2020.00616
Yao, Q., Liu, Y., and Sun, H. (2018). Heparin-dopamine functionalized graphene foam for sustained release of bone morphogenetic protein-2. J. Tissue Eng. Regen. Med. 12, 1519–1529. doi:10.1002/term.2681
Zhao, L., Pei, X., Jiang, L., Hu, C., Sun, J., Xing, F., et al. (2019). Bionic design and 3D printing of porous titanium alloy scaffolds for bone tissue repair. Compos. Part B Eng. 162, 154–161. doi:10.1016/j.compositesb.2018.10.094
Zhou, L., Ge, J., Wang, M., Chen, M., Cheng, W., Ji, W., et al. (2021). Injectable muscle-adhesive antioxidant conductive photothermal bioactive nanomatrix for efficiently promoting full-thickness skeletal muscle regeneration. Bioact. Mat. 6, 1605–1617. doi:10.1016/j.bioactmat.2020.11.005
Zhou, Y. L., Yang, Q. Q., Yan, Y. Y., Zhang, L., Wang, Q. H., Ju, F., et al. (2019). Gene-loaded nanoparticle-coated sutures provide effective gene delivery to enhance tendon healing. Mol. Ther. 27, 1534–1546. doi:10.1016/j.ymthe.2019.05.024
Keywords: polydopamine, surface engineering, tissue engineering, musculoskeletal tissue, scaffolds
Citation: Tolabi H, Bakhtiary N, Sayadi S, Tamaddon M, Ghorbani F, Boccaccini AR and Liu C (2022) A critical review on polydopamine surface-modified scaffolds in musculoskeletal regeneration. Front. Bioeng. Biotechnol. 10:1008360. doi: 10.3389/fbioe.2022.1008360
Received: 31 July 2022; Accepted: 04 November 2022;
Published: 18 November 2022.
Edited by:
Jianxun Ding, Changchun Institute of Applied Chemistry (CAS), ChinaReviewed by:
Guowei Zhou, Qilu University of Technology, ChinaWeizhen Chen, Zhejiang University, China
Copyright © 2022 Tolabi, Bakhtiary, Sayadi, Tamaddon, Ghorbani, Boccaccini and Liu. This is an open-access article distributed under the terms of the Creative Commons Attribution License (CC BY). The use, distribution or reproduction in other forums is permitted, provided the original author(s) and the copyright owner(s) are credited and that the original publication in this journal is cited, in accordance with accepted academic practice. No use, distribution or reproduction is permitted which does not comply with these terms.
*Correspondence: Chaozong Liu, chaozong.liu@ucl.ac.uk
†These authors have contributed equally to this work