- 1Department of Biomedical Engineering, Science and Research Branch, Islamic Azad University, Tehran, Iran
- 2Department of Mechanical Engineering, Faculty of Engineering, Yazd University, Yazd, Iran
- 3Medical Nanotechnology and Tissue Engineering Research Center, Yazd Reproductive Sciences Institute, Shahid Sadoughi University of Medical Sciences, Yazd, Iran
- 4Department of Life Science Engineering, Faculty of New Sciences and Technologies, University of Tehran, Tehran, Iran
- 5Department of Orthopedics, Shanghai Pudong Hospital, Fudan University Pudong Medical Center, Shanghai, China
- 6Department of Orthopedics, Shanghai Pudong New Area People’s Hospital, Shanghai, China
The biomaterials’ success within the tissue engineering field is hinged on the capability to regulate tissue and cell responses, comprising cellular adhesion, as well as repair and immune processes’ induction. In an attempt to enhance and fulfill these biomaterials’ functions, scholars have been inspired by nature; in this regard, surface modification via coating the biomaterials with polydopamine is one of the most successful inspirations endowing the biomaterials with surface adhesive properties. By employing this approach, favorable results have been achieved in various tissue engineering-related experiments, a significant one of which is the more rapid cellular growth observed on the polydopamine-coated substrates compared to the untreated ones; nonetheless, some considerations regarding polydopamine-coated surfaces should be taken into account to control the ultimate outcomes. In this mini-review, the importance of coatings in the tissue engineering field, the different types of surfaces requiring coatings, the significance of polydopamine coatings, critical factors affecting the result of the coating procedure, and recent investigations concerning applications of polydopamine-coated biomaterials in tissue engineering are thoroughly discussed.
1 Introduction
Material selection and other critical qualities of scaffolds, including possessing an adhesive surface, biodegradability, biocompatibility, mechanical stability, etc., must be meticulously considered so that the various tissues can be successfully regenerated (Zhang et al., 2019; Ghorbani et al., 2020b). Concerning this subject, surface engineering of biomaterials using various techniques is gaining increasing prominence in tissue engineering (TE) applications, and surface modification, as a popular surface engineering strategy, is utilized to alter the microenvironment of cells and transform the scaffolds’ surface into an adhesive one (Arab-Ahmadi et al., 2021; Davari et al., 2022).
Compared to other coating substances, including proteins (Gennadios, 2002), particular peptide sequences (Pagel and Beck-Sickinger, 2017), ceramics (Montazerian et al., 2022), and metals (Yin et al., 2021), polydopamine (PDA), a melanin-like mussel-inspired coating polymer (Ghorbani et al., 2022), possesses a variety of desirable properties, such as outstanding adhesion features (Ghalandari et al., 2021; Feinberg and Hanks, 2022), extraordinary hydrophilicity (Li et al., 2018), biodegradability (Zhang and King, 2020), uniform shape (Saeed et al., 2021), biocompatibility (Zeng et al., 2020), and thermal stability (Yim et al., 2022). Furthermore, its biological properties, including enhanced cellular proliferation, improved bioactivity, free-radical scavenging activities, metal ion chelating capacity, and anti-bacterial capability, originate from its catecholamine and hydroxyl functional groups (Yu et al., 2017; Ghorbani et al., 2019b, 2019a; Deng et al., 2021). Notably, solvent selection is of paramount importance for solution-based chemical reactions associated with polymers like PDA. Water and ethanol have been broadly employed among various solvents (Jiang et al., 2014; Nieto et al., 2021). A self-assembled PDA layer can be formed on the surfaces of a variety of materials, like metals, ceramics, polymers, oxides, and semiconductors, when soaked in a PDA’s weakly alkaline aqueous solution (10 mM Tris-HCl, pH∼8.5) without the addition of sophisticated connecting agents (Kao et al., 2018; Yang et al., 2019; Lee et al., 2020; Li et al., 2021b; Park et al., 2021). Nonetheless, the volume ratio of water to alcohol strongly influences the PDA spheres’ synthesis. It was illustrated that neither did PDA microspheres form in the pure ethanol nor had a perfect shape in the pure water; in essence, when ethanol and water were mixed with a ratio of 30:70, formation happened in the best way possible (Yan et al., 2013). Insufficient conductivity has ever been the major weakness of PDA (Mei et al., 2020). Since the pros of PDA outweigh its cons, various groups of scientists have modified the biomaterials’ surfaces by employing PDA with the aim of augmenting their surface performance. Therefore, within the last couple of years, PDA has been in the spotlight, with diverse applications in the field of biomedical engineering (Lynge et al., 2011), including drug delivery (Huang et al., 2018), implants (Jia et al., 2019), surface engineering (Yang et al., 2015), cancer therapy (Abdollahi et al., 2022; Honmane et al., 2022), TE (bone (Huang et al., 2016; Kaushik et al., 2020), cartilage (Huang et al., 2021), muscle (Zhou et al., 2021), skin (Yazdi et al., 2022), tendon (Lin et al., 2019), and neuron (Qian et al., 2018; Yan et al., 2020)), and microfluidic systems (Niculescu et al., 2021).
The process parameters of PDA considerably impact the coating’s quality and characteristics; thus, the experimental factors, such as coating time, temperature, pH, and DA’s initial concentration, are properly adjusted so that a coating layer with outstanding features can be formed (Ghorbani et al., 2020c).
Within this paper, firstly, the coatings’ significance in the TE field and various kinds of surfaces in need of coatings are comprehensively reviewed. Afterward, the PDA coatings’ importance is exhaustively discussed. Ultimately, the parameters that are regulated to improve the properties of coatings and the employment of these interesting coatings in TE by introducing relevant case studies are explained.
2 Polydopamine-coated surfaces
Synthetic polymers, such as polylactic-co-glycolic acid (PLGA) (Ghorbani et al., 2020c), polyurethane (PU) (Merceron et al., 2015), polycaprolactone (PCL) (Perez-Puyana et al., 2021), polydimethylsiloxane (PDMS) (Cunniffe et al., 2019), poly-l-lactic acid (PLA) (Jenkins and Little, 2019), polyvinyl alcohol (PVA) (Rodríguez-Rodríguez et al., 2020), polyhydroxyalkanoates (PHAs) (Aguilar-Rabiela et al., 2021; Gregory et al., 2022), and polyamide (Razaviye et al., 2022) are frequently utilized in the fabrication of different scaffolds due to their superior characteristics, comprising customizable degradation rate, excellent processability, great mechanical properties, and wide in-vitro and in-vivo availability. Nevertheless, synthetic polymers typically have poor cell adhesion properties; consequently, combinations with natural polymers or various modifications are common approaches to tackle this obstacle (Terzopoulou et al., 2022). Besides, these modifications are also applied to natural polymers, such as chitosan (Bock et al., 2020), cellulose (Nishida and Koga, 2018), peptides (Ji and Parquette, 2020), etc., to enhance their features.
Boosting biomaterials’ performance, modifying their physicochemical properties, and broadening the applications of them are possible by conducting the surface modification procedure (Salatin et al., 2015). Considerable effort has been dedicated to the substances’ surface modification via employing various techniques, such as self-assembly monolayer (Kehr et al., 2015), plasma surface modification (Ghorbani et al., 2020d), and layer-by-layer assembly (Liu et al., 2016). Despite these approaches’ multiple benefits, they suffer from some drawbacks: limited chemical characterization, restricted shape and dimension of the resultant substances, complicated equipment, and complex synthesis processes (Liu et al., 2016). As an alternative, a coating strategy based upon PDA, formed through covalent polymerization and non-covalent self-assembly (Hong et al., 2012), is widely employed.
The convoluted structure of PDA incorporates a mixture of diverse oligomers formed as a result of dopamine (DA) oxidation, including indole units with varying degrees of oxidation, carboxylic acid and amino groups, imine units, indolic/catecholic π-systems, quinone residues, phenol groups, and open-chain DA molecules (Liu et al., 2014; Ghorbani et al., 2019c). Of note, the functional groups in the PDA structure (catechol, imine, and amine) are employed to reinforce the interaction between the biomaterial and the tissue. What’s more, within its irregular, cross-linked network with mixed bonding configurations, radicals are located at quinone residues (Du et al., 2017). Owing to the presence of a series of constant π-electron free radicals, PDA is paramagnetic and operates analogously to a radical trap (Kim et al., 2021b).
As a transitional surface, PDA enables the adhesion of other materials to scaffolds without demanding pre-functionalization. This phenomenon is mainly because of the quinone functional groups that are: 1) electron-deficient and 2) capable of participating in Schiff base reactions occurring on amines and stimulating the Michael addition reactions with nucleophilic groups, including amines and thiols (Rizzo and Kehr, 2021). Notably, the PDA surfaces’ sensitivity towards the nucleophiles relies on the catechol/quinone equilibrium; therefore, the generated alkaline pH speeds up conjugation reactions (Batul et al., 2017). Other functional groups within the structure or generated by PDA serve pivotal roles; for instance, amine groups are involved in displacing hydrated minerals on the surface as well as bringing catechols closer to the surface to create strong molecular interactions (Lee et al., 2019). Another example is the hydroxyl and amine groups produced by PDA on the scaffolds that can promptly release signals to promote integrin binding, resulting in the increment of osteogenic activities (Ghorbani et al., 2020c). The more binding functional groups on the surface, the higher probability of the molecules and inorganic ions loading, leading to the improvement of cellular attachment (Richbourg et al., 2019).
3 Polydopamine coating’s key process parameters in tissue engineering applications
Brimming with outstanding features, PDA coatings have been extensively employed in TE-related investigations. In this regard, several experiments have achieved favorable outcomes when utilizing this coating compared with the others (Sharma et al., 2019; Kopeć et al., 2022). Within a novel study, scaffolds were coated with PDA and gelatin to evaluate the anti-corrosion property. The PDA-coated and gelatin-coated samples were biocompatible and displayed corrosion current density (Icorr) of 2.95 × 10–3 and 3.72 × 10–3
For PDA polymerization on the surface of the biomaterials, multiple factors should be considered to achieve the desired outcomes in research cases. Among these parameters, coating time, temperature, pH of Tris-HCl, and initial DA concentration in the DA/Tris-HCl buffer solution are discussed in the following. Table 1 presents these determining factors and their impacts upon the formed PDA layer. Furthermore, Figure 1 illustrates the outline briefly describing the case studies in terms of the process parameters and their effects.
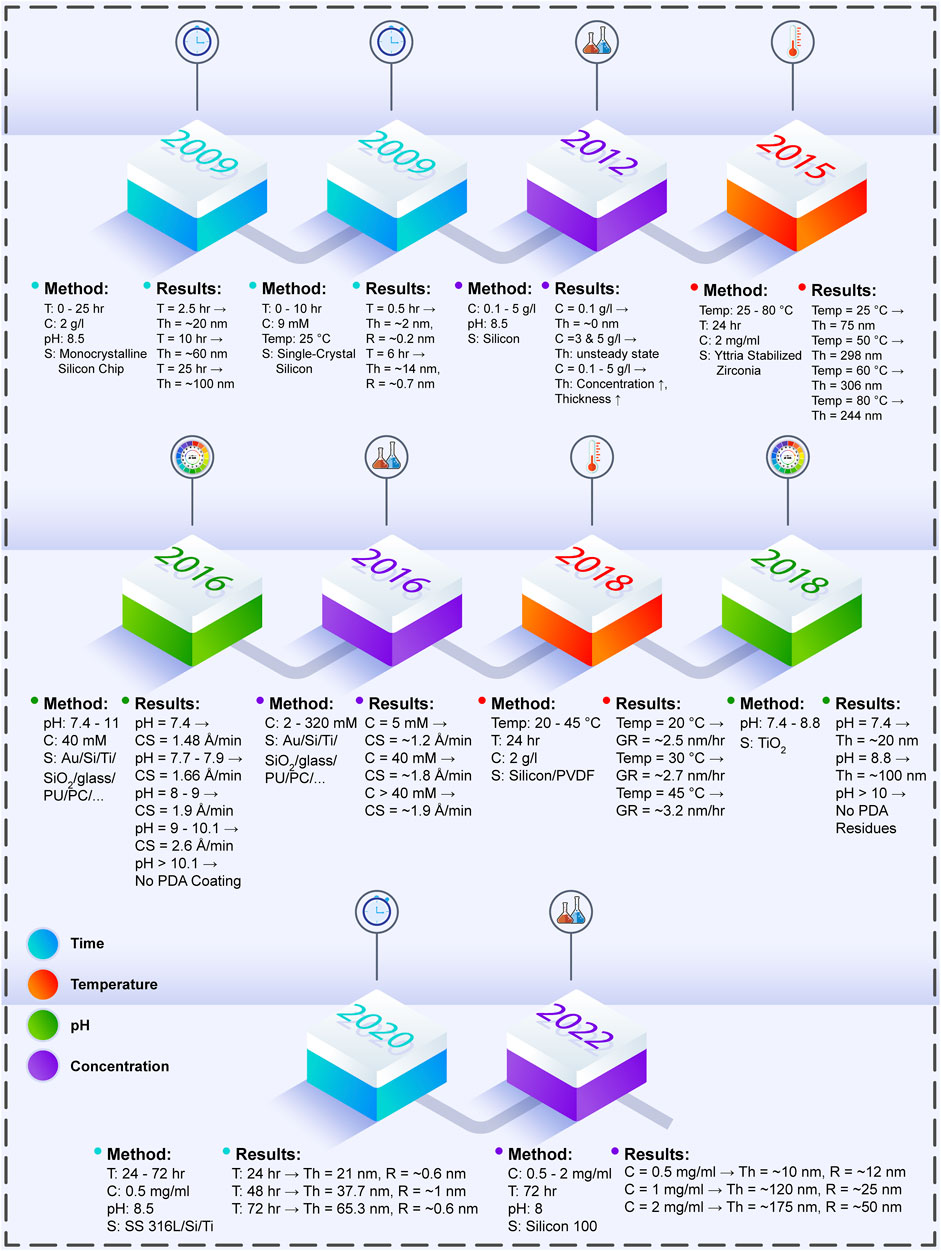
FIGURE 1. The effects of PDA process parameters on the produced PDA layer, which are investigated in various experiments throughout the years (T: time, C: concentration, S: surface, Th: thickness, Temp: temperature, R: roughness, Au: aurum, Ti: titanium, Si: silicon, PU: polyurethane, PC: polycarbonate, CS: coating speed, PVDF: polyvinylidene fluoride, GR: coating layer growth rate, SS 316L: stainless steel 316L) (Li et al., 2009; Ou et al., 2009; Ball et al., 2012; Zain et al., 2015; Hong et al., 2016; Habibi Rad et al., 2018; Hong et al., 2018; Zhou et al., 2020; Szewczyk et al., 2022).
3.1 Coating time
The PDA coating process commonly occurs at room temperature in pH 8.5 for 24 h with the initial concentration of 2
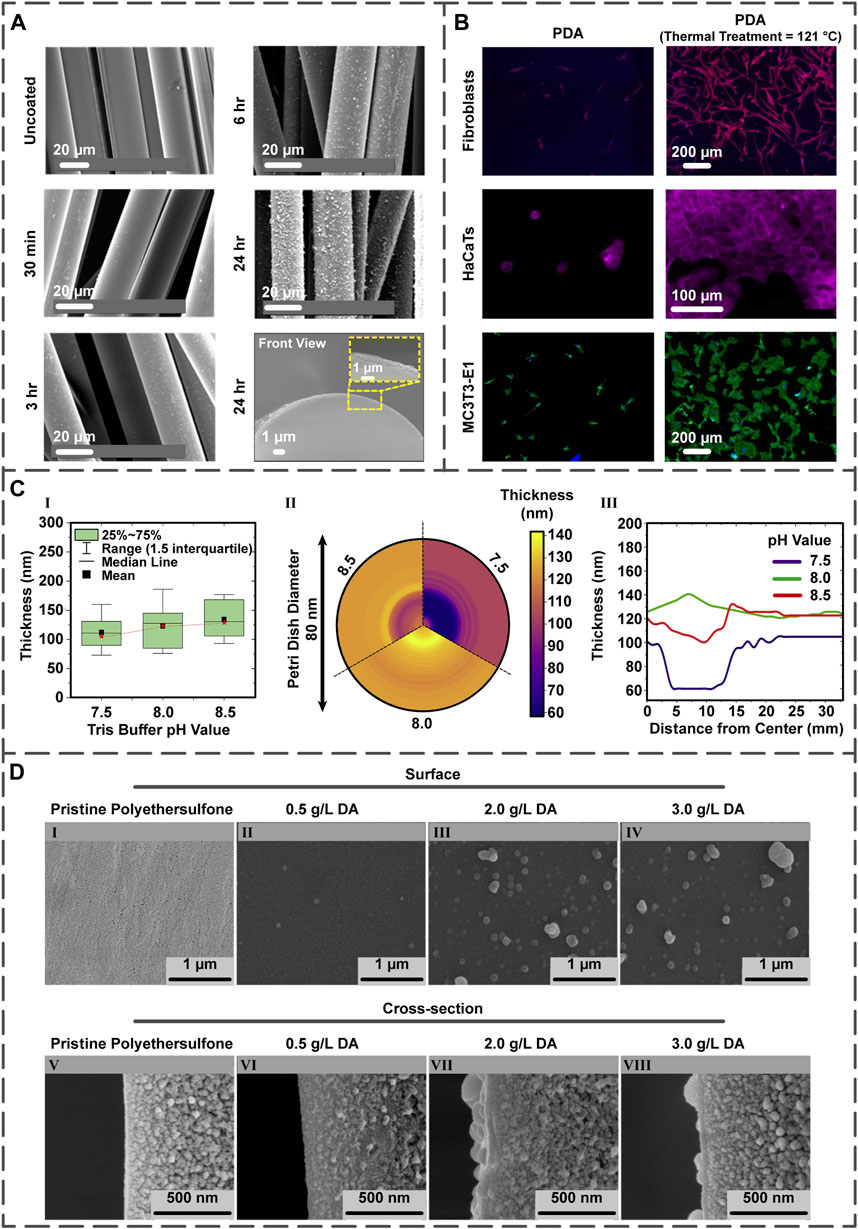
FIGURE 2. (A) The influence of PDA coating time upon roughness and thickness of the PDA layer on the PGF (reproduced content is open access) (Felfel et al., 2021). (B) Post-treatment of the PDA layer present on the PDA-coated Ti samples and the quantification of fibroblast, HaCaT, and MC3T3-E1 cells on the surfaces (reproduced content is open access) (Davidsen et al., 2021). (C) The different pH values of Tris buffer and their effects on the film thickness illustrated in the comparative chart (I), mapping chart (II), and line chart (III) (reproduced content is open access) (Szewczyk et al., 2022). (D) The DA concentration impact on the morphology of coating displayed by field emission scanning electron microscopy images. Surface and cross-section images of (I,V) non-coated polyethersulfone membranes as well as membranes coated with PDA possessing DA concentrations of (II,VI) 0.5
Multiple studies have suggested the direct influence of coating time on the PDA layer roughness (Ou et al., 2009; Dang et al., 2015; Liu et al., 2020). Concerning this matter, Kim and co-workers engineered high robust PDA-coated polybenzimidazole membranes under different coating times (5, 10, and 30 min). The atomic force microscope image of the PDA layer illustrated that with increasing time, the roughness enhanced from nearly 1 nm in 5 min to 4 nm in 30 min (Kim et al., 2021a).
As an intermediate layer, PDA coating has been employed in various experiments. Recently, Zia et al. (Zia et al., 2021) used a PDA layer between chitosan and porous PLA via applying different polymerization times (1, 6, 12, 18, and 24 h). It was observed that the PDA layer thickness increased with time; to delineate, although some uncovered areas lacking the PDA layer were found after 1 h, a layer with an acceptable thickness appeared when polymerization continued for 6 h. Moreover, the scaffold’s diameter and hydrophilicity were both enhanced by time; to elucidate on, the diameter increased from 1.2 μm after 6 h to 1.8 μm after 24 h, and the water contact angle decreased from 85° at 6 h to 70.6° at 24 h.
3.2 Temperature
The process temperature has been shown to improve thickness, roughness, as well as polymerization rate and shorten the polymerization time of the PDA layer. In an investigation conducted by a group of researchers (Zhou et al., 2014), it was illustrated that among various temperatures (25, 37, and 60°C), 60°C and 300
Another effect of temperature is the increment of the PDA layer’s thickness (Zain et al., 2015; Oymaci et al., 2020) and roughness (Schaubroeck et al., 2015; Oymaci et al., 2020), which was carefully assessed in a project. It was reported that the coating thickness on several materials, such as Si/polyvinylidene fluoride (PVDF), was augmented by raising the temperature; to be more precise, thickness increased from nearly 20 nm at 20°C to 65 nm at 45°C. It is also noteworthy to mention that the modified surfaces’ water contact angle decreased with augmenting the reaction temperature (from 63.9° at 20°C to 53.2° at 45°C). Regarding the roughness, further tests indicated that the layer roughness increased from 6.4 ± 0.9 nm (20°C) to 10.4 ± 1.6 nm (45°C) (Jiang et al., 2011).
Concerning the influence of temperature on the polymerization rate, several studies have reported the rate enhancement with temperature raising (Deng et al., 2018; Habibi Rad et al., 2018). In a novel experiment in which PDA-coated Si surfaces were fabricated, the mentioned effect was investigated within a temperature range of 25–35°C. They found that higher temperature led to the accelerated rate; indeed, polymerization speed increased from 1.8
Improving the coating stability can be achieved by the coating’s thermal treatment. In essence, the thermal treatment affects the number of quinone and amine groups at the PDA surface (Proks et al., 2013; Malollari et al., 2019). Within an intriguing study, titanium (Ti)/Si wafer samples were coated via PDA; specifically, some were thermally treated by storing at 121°C for 24 h, while others were post-treated by 2-week storing at room temperature. In both treatments, the numbers of the quinone and primary amine groups increased and decreased, respectively, improving layer stability and favoring cellular proliferation. The proliferation of fibroblast, human keratinocytes (HaCaT), and MC3T3-E1 cells on post-treated PDA-coated samples was more enhanced in comparison with the PDA-coated ones (Figure 2B) (Davidsen et al., 2021).
3.3 pH of Tris-HCl
In the synthesis process of PDA coating, various experiments have reported the creation of a weak alkaline environment (pH > 8.5) with oxygen as the oxidant in the Tris-HCl buffer presence. When oxygen is constantly present as an oxidant in the reaction, an even coating of PDA is formed. As another synthesis method, creating an oxygen gradient can lead to the formation of a PDA gradient coating (Lee et al., 2019). In some synthesis approaches with other oxidants, the pH may vary between weakly acidic and neutral (Wei et al., 2010; Bernsmann et al., 2011; Ghorbani et al., 2021). Furthermore, PDA can be formed via self-polymerization within an acidic environment employing hydrothermal methods (160°C in 6 h) (Zheng et al., 2015).
The pH value directly affects the PDA layer thickness (Hong et al., 2018) since the initial DA oxidation depends on the amount of pH (Yang et al., 2014; Salomäki et al., 2018). Regarding this subject, the influence of pH on the PDA two-dimensional films’ thickness was examined by choosing pH values of 7.5, 8.0, and 8.5. Interestingly, the lowest thickness (24 nm) was achieved at pH 7.5, whereas the highest one (35 nm) was obtained at pH 8.5. However, the most favorable result was found in pH 8.0 because of the fewer variations between the central and external regions of the film (Figure 2C) (Szewczyk et al., 2022).
Concerning the impact of pH on the polymerization rate, multiple investigations have revealed that the rate increases with the pH increment (Dong et al., 2018; Mei et al., 2021). Within an innovative project, sprayable PDA with ultra-fast polymerization was employed to coat solid surfaces. In this regard, the pH was selected in the range of 7–11, and when it went beyond 10.1, no PDA layer could be formed. Nevertheless, the polymerization speed enhanced from 1.48
Given that better outcomes concerning the PDA polymerization have been produced in the alkaline environment, most studies have concentrated on this pH range. For example, as a modification factor, PDA was grafted onto the hyaluronic acid (HA) chains under a pH value of 8.0, followed by the production of hydrogel scaffolds through incorporating PDA/HA complex in a dual cross-linked collagen type I (Col I) matrix for use in cartilage regeneration. The final results showed that the employed PDA coating enhanced BMSCs’ proliferation, improved cellular affinity, and induced chondrogenic differentiation of cells compared to those of the cell-seeded scaffolds without the PDA layer. Additionally, it augmented anti-inflammation capacity, immune modulation ability, and in-vivo cartilage repair in the rabbit model (Gan et al., 2022).
3.4 Concentration
Like other discussed parameters, concentration is another critical factor impacting the final PDA layer. Of note, the concentration of the DA monomer must be at least 2
For investigating the effect of concentration on the PDA layer, the porous Col I-HA scaffold was modified via immersing into various DA concentrations (0.5, 1.0, and 2.0
With respect to the effect of concentration on the polymerization rate, the rate boosts with the concentration increment (Hong et al., 2016). For instance, in the DA concentration range of 0.1–5
The thickness and roughness of the PDA layer can be controlled by tuning this factor; regarding this matter, several studies have stated that lower concentrations result in lower PDA’s thickness (Dong et al., 2018; Wu et al., 2020) and roughness (Ball et al., 2012; Ding et al., 2014). To evaluate these phenomena, a team of scientists (Oymaci et al., 2020) coated the polyethersulfone membranes with PDA possessing diverse concentrations (0.5–3.0
4 Conclusion
According to the ability of PDA as a surface modifier, this nature-inspired material has been widely employed in TE applications. Notably, certain conditions and parameters should be considered for the PDA polymerization process, including coating time, temperature, pH of Tris-HCl, and initial DA concentration, to acquire beneficial results. Even though the mentioned parameters have been given fixed values (24 h, room temperature, pH 8.5, and 2
There is still a long way to go regarding translating these innovative coated biomaterials into the clinic; consequently, successfully managing parameters together for furnishing the modified scaffolds with the desired properties has become a prominent goal in the preclinical experiments.
Author contributions
Conceptualization, SS; Methodology, SS and MKH; Validation, SS, MKH, DL, and ND; Investigation, SS, MKH, DL, and ND; Resources, SS and MKH; Data Curation, SS, MKH, DL, and ND; Writing “original draft preparation, SS, MKH, DL, and ND; Writing ” review and editing, BY; Visualization, MKH; Supervision, BY; Project Administration, BY; Funding Acquisition, BY. All authors have read and agreed to the published version of the manuscript.
Funding
This work was supported by the Outstanding Clinical Discipline Project of Shanghai Pudong (Grant No. PWYgy 2021-08), the Joint project of Shanghai Pudong New Area Health Commission (PW2021D-12), and the National Natural Science Foundation of China (Grant No. 81971753, 82170897).
Conflict of interest
The authors declare that the research was conducted in the absence of any commercial or financial relationships that could be construed as a potential conflict of interest.
Publisher’s note
All claims expressed in this article are solely those of the authors and do not necessarily represent those of their affiliated organizations, or those of the publisher, the editors and the reviewers. Any product that may be evaluated in this article, or claim that may be made by its manufacturer, is not guaranteed or endorsed by the publisher.
References
Abdollahi, J., Davari, N., Panahi, Y., and Gardaneh, M. (2022). Detection of metastatic breast cancer from whole-slide pathology images using an ensemble deep-learning method. Archives Breast Cancer 9 (3), 364–376. doi:10.32768/abc.202293364-376
Aguilar-Rabiela, A. E., Leal-Egaña, A., Nawaz, Q., and Boccaccini, A. R. (2021). Integration of mesoporous bioactive glass nanoparticles and curcumin into PHBV microspheres as biocompatible composite for drug delivery applications. Molecules 26 (11), 3177. doi:10.3390/molecules26113177
Arab-Ahmadi, S., Irani, S., Bakhshi, H., Atyabi, F., and Ghalandari, B. (2021). Immobilization of carboxymethyl chitosan/laponite on polycaprolactone nanofibers as osteoinductive bone scaffolds. Polym. Adv. Technol. 32 (2), 755–765. doi:10.1002/pat.5128
Ball, V., Frari, D., Toniazzo, V., and Ruch, D. (2012). Kinetics of polydopamine film deposition as a function of pH and dopamine concentration: Insights in the polydopamine deposition mechanism. J. Colloid Interface Sci. 386 (1), 366–372. doi:10.1016/j.jcis.2012.07.030
Batul, R., Tamanna, T., Khaliq, A., and Yu, A. (2017). Recent progress in the biomedical applications of polydopamine nanostructures. Biomater. Sci. 5 (7), 1204–1229. doi:10.1039/C7BM00187H
Bernsmann, F., Ball, V., Addiego, F., Ponche, A., Michel, M., Gracio, J. J. d. A., et al. (2011). Dopamine−Melanin film deposition depends on the used oxidant and buffer solution. Langmuir 27 (6), 2819–2825. doi:10.1021/la104981s
Bock, N., Pham, T. L.-B., Nguyen, T. B., Tran, H. A., and Tran, P. A. (2020). Polydopamine coating of uncrosslinked chitosan as an acellular scaffold for full thickness skin grafts. Carbohydr. Polym. 245, 116524. doi:10.1016/j.carbpol.2020.116524
Cunniffe, G. M., Díaz-Payno, P. J., Sheehy, E. J., Critchley, S. E., Almeida, H. V., Pitacco, P., et al. (2019). Tissue-specific extracellular matrix scaffolds for the regeneration of spatially complex musculoskeletal tissues. Biomaterials 188, 63–73. doi:10.1016/j.biomaterials.2018.09.044
Dang, Y., Xing, C.-M., Quan, M., Wang, Y. B., Zhang, S. P., Shi, S. Q., et al. (2015). Substrate independent coating formation and anti-biofouling performance improvement of mussel inspired polydopamine. J. Mat. Chem. B 3 (20), 4181–4190. doi:10.1039/C5TB00341E
Davari, N., Bakhtiary, N., Khajehmohammadi, M., Sarkari, S., Tolabi, H., Ghorbani, F., et al. (2022). Protein-based hydrogels: Promising materials for tissue engineering. Polymers 14 (5), 986. doi:10.3390/polym14050986
Davidsen, M. B., Teixeira, J. F. L., Dehli, J., Karlsson, C., Kraft, D., Souza, P. P., et al. (2021). Post-treatments of polydopamine coatings influence cellular response. Colloids Surfaces B Biointerfaces 207, 111972. doi:10.1016/j.colsurfb.2021.111972
Deng, Z., Shang, B., and Peng, B. (2018). Polydopamine based colloidal materials: Synthesis and applications. Chem. Rec. 18 (4), 410–432. doi:10.1002/tcr.201700051
Deng, Z., Wang, W., Xu, X., Nie, Y., Liu, Y., Gould, O. E. C., et al. (2021). Biofunction of polydopamine coating in stem cell culture. ACS Appl. Mat. Interfaces 13 (9), 10748–10759. doi:10.1021/acsami.0c22565
Ding, Y., Weng, L.-T., Yang, M., Yang, Z., Lu, X., Huang, N., et al. (2014). Insights into the aggregation/deposition and structure of a polydopamine film. Langmuir 30 (41), 12258–12269. doi:10.1021/la5026608
Dong, L., Liu, X., Xiong, Z., Sheng, D., Lin, C., Zhou, Y., et al. (2018). Fabrication of highly efficient ultraviolet absorbing PVDF membranes via surface polydopamine deposition. J. Appl. Polym. Sci. 135 (4), 45746. doi:10.1002/app.45746
Du, Q., An, J., Li, J., Zhou, L., Li, N., and Wang, X. (2017). Polydopamine as a new modification material to accelerate startup and promote anode performance in microbial fuel cells. J. Power Sources 343, 477–482. doi:10.1016/j.jpowsour.2017.01.093
Feinberg, H., and Hanks, T. W. (2022). Polydopamine: A bioinspired adhesive and surface modification platform. Polym. Int. 71 (5), 578–582. doi:10.1002/pi.6358
Felfel, R. M., Parsons, A. J., Chen, M., Stuart, B. W., Wadge, M. D., and Grant, D. M. (2021). Water resistant fibre/matrix interface in a degradable composite: Synergistic effects of heat treatment and polydopamine coating. Compos. Part A Appl. Sci. Manuf. 146, 106415. doi:10.1016/j.compositesa.2021.106415
Gan, D., Jiang, Y., Hu, Y., Wang, X., Wang, Q., Wang, K., et al. (2022). Mussel-inspired extracellular matrix-mimicking hydrogel scaffold with high cell affinity and immunomodulation ability for growth factor-free cartilage regeneration. J. Orthop. Transl. 33, 120–131. doi:10.1016/j.jot.2022.02.006
Gennadios, A. (2002). Protein-based films and coatings. 1st Ed. Boston: CRC Press. doi:10.1201/9781420031980
Ghalandari, B., Yu, Y., Ghorbani, F., Warden, A. R., Ahmad, K. Z., and Sang, X. (2021). Polydopamine nanospheres coated with bovine serum albumin permit enhanced cell differentiation: Fundamental mechanism and practical application for protein coating formation. Nanoscale 47. doi:10.1039/D1NR07469E
Ghorbani, F., Ghalandari, B., Khan, A. L., Li, D., Zamanian, A., and Yu, B. (2020a). Decoration of electrical conductive polyurethane-polyaniline/polyvinyl alcohol matrixes with mussel-inspired polydopamine for bone tissue engineering. Biotechnol. Prog. 36 (6), e3043. doi:10.1002/btpr.3043
Ghorbani, F., Ghalandari, B., and Liu, C. (2021). A facile method to synthesize 3D pomegranate-like polydopamine microspheres. Front. Bioeng. Biotechnol. 9, 737074. doi:10.3389/fbioe.2021.737074
Ghorbani, F., Kim, M., Monavari, M., Ghalandari, B., and Boccaccini, A. R. (2022). Mussel-inspired polydopamine decorated alginate dialdehyde-gelatin 3D printed scaffolds for bone tissue engineering application. Front. Bioeng. Biotechnol. 10, 940070. doi:10.3389/fbioe.2022.940070
Ghorbani, F., Zamanian, A., and Aidun, A. (2019a). Bioinspired polydopamine coating-assisted electrospun polyurethane-graphene oxide nanofibers for bone tissue engineering application. J. Appl. Polym. Sci. 136 (24), 47656. doi:10.1002/app.47656
Ghorbani, F., Zamanian, A., Behnamghader, A., and Daliri-Joupari, M. (2019c). Bone-like hydroxyapatite mineralization on the bio-inspired PDA nanoparticles using microwave irradiation. Surfaces Interfaces 15, 38–42. doi:10.1016/j.surfin.2019.01.007
Ghorbani, F., Zamanian, A., Behnamghader, A., and Joupari, M. D. (2019b). A facile method to synthesize mussel-inspired polydopamine nanospheres as an active template for in situ formation of biomimetic hydroxyapatite. Mater. Sci. Eng. C 94, 729–739. doi:10.1016/j.msec.2018.10.010
Ghorbani, F., Zamanian, A., Kermanian, F., and Shamoosi, A. (2020b). A bioinspired 3D shape olibanum-collagen-gelatin scaffolds with tunable porous microstructure for efficient neural tissue regeneration. Biotechnol. Prog. 36 (1), e2918–12. doi:10.1002/btpr.2918
Ghorbani, F., Zamanian, A., and Sahranavard, M. (2020c). Mussel-inspired polydopamine-mediated surface modification of freeze-cast poly (ε-caprolactone) scaffolds for bone tissue engineering applications. Biomed. Eng./Biomed. Tech. 65 (3), 273–287. doi:10.1515/bmt-2019-0061
Ghorbani, F., Zamanian, A., and Torabinejad, B. (2020d). The effect of oxygen plasma pretreatment on the properties of mussel-inspired polydopamine-decorated polyurethane nanofibers. J. Polym. Eng. 40 (2), 109–119. doi:10.1515/polyeng-2019-0219
Gregory, D. A., Taylor, C. S., Fricker, A. T. R., Asare, E., Tetali, S. S., Haycock, J. W., et al. (2022). Polyhydroxyalkanoates and their advances for biomedical applications. Trends Mol. Med. 28 (4), 331–342. doi:10.1016/j.molmed.2022.01.007
Habibi Rad, M., Zamanian, A., Hadavi, S. M. M., and Khanlarkhani, A. (2018). A two-stage kinetics model for polydopamine layer growth. Macromol. Chem. Phys. 219 (9), 1700505. doi:10.1002/macp.201700505
Hong, S. H., Hong, S., Ryou, M.-H., Choi, J. W., Kang, S. M., and Lee, H. (2016). Sprayable ultrafast polydopamine surface modifications. Adv. Mat. Interfaces 3 (11), 1500857. doi:10.1002/admi.201500857
Hong, S., Na, Y. S., Choi, S., Song, I. T., Kim, W. Y., and Lee, H. (2012). Non-covalent self-assembly and covalent polymerization Co-contribute to polydopamine formation. Adv. Funct. Mat. 22 (22), 4711–4717. doi:10.1002/adfm.201201156
Hong, S., Wang, Y., Park, S. Y., and Lee, H. (2018). Progressive fuzzy cation-π assembly of biological catecholamines. Sci. Adv. 4 (9), eaat7457. doi:10.1126/sciadv.aat7457
Honmane, S. M., Charde, M. S., Salunkhe, S. S., Choudhari, P. B., and Nangare, S. N. (2022). Polydopamine surface-modified nanocarriers for improved anticancer activity: Current progress and future prospects. OpenNano 7, 100059. doi:10.1016/j.onano.2022.100059
Huang, J., Xiong, J., Wang, D., Zhang, J., Yang, L., Sun, S., et al. (2021). 3D bioprinting of hydrogels for cartilage tissue engineering. Gels 7 (3), 144. doi:10.3390/gels7030144
Huang, L., Liu, M., Huang, H., Wen, Y., Zhang, X., and Wei, Y. (2018). Recent advances and progress on melanin-like materials and their biomedical applications. Biomacromolecules 19 (6), 1858–1868. doi:10.1021/acs.biomac.8b00437
Huang, S., Liang, N., Hu, Y., Zhou, X., and Abidi, N. (2016). Polydopamine-Assisted surface modification for bone biosubstitutes. Biomed. Res. Int. 2016, 2389895. doi:10.1155/2016/2389895
Jenkins, T. L., and Little, D. (2019). Synthetic scaffolds for musculoskeletal tissue engineering: Cellular responses to fiber parameters. npj Regen. Med. 4 (1), 15. doi:10.1038/s41536-019-0076-5
Ji, M., and Parquette, J. R. (2020). Enhanced stability of peptide nanofibers coated with a conformal layer of polydopamine. Chem. Eur. J. 26 (39), 8572–8578. doi:10.1002/chem.202000403
Jia, L., Han, F., Wang, H., Zhu, C., Guo, Q., Li, J., et al. (2019). Polydopamine-assisted surface modification for orthopaedic implants. J. Orthop. Transl. 17, 82–95. doi:10.1016/j.jot.2019.04.001
Jiang, J., Zhu, L., Zhu, L., Zhu, B., and Xu, Y. (2011). Surface characteristics of a self-polymerized dopamine coating deposited on hydrophobic polymer films. Langmuir 27 (23), 14180–14187. doi:10.1021/la202877k
Jiang, X., Wang, Y., and Li, M. (2014). Selecting water-alcohol mixed solvent for synthesis of polydopamine nano-spheres using solubility parameter. Sci. Rep. 4 (1), 6070. doi:10.1038/srep06070
Kao, C.-T., Chen, Y.-J., Ng, H.-Y., Lee, A., Huang, T. H., Lin, T. F., et al. (2018). Surface modification of calcium silicate via mussel-inspired polydopamine and effective adsorption of extracellular matrix to promote osteogenesis differentiation for bone tissue engineering. Materials 11 (9), 1664. doi:10.3390/ma11091664
Kaushik, N., Nhat Nguyen, L., Kim, J. H., Choi, E. H., and Kumar Kaushik, N. (2020). Strategies for using polydopamine to induce biomineralization of hydroxyapatite on implant materials for bone tissue engineering. Int. J. Mol. Sci. 21 (18), 6544. doi:10.3390/ijms21186544
Kehr, N. S., Atay, S., and Ergün, B. (2015). Self-assembled monolayers and nanocomposite hydrogels of functional nanomaterials for tissue engineering applications. Macromol. Biosci. 15 (4), 445–463. doi:10.1002/mabi.201400363
Kim, S. D., Won, G. Y., Shah, A. A., Park, A., Park, Y. I., Nam, S. E., et al. (2021a). Reinforcing the polybenzimidazole membrane surface by an ultrathin co-crosslinked polydopamine layer for organic solvent nanofiltration applications. J. Membr. Sci. 636, 119587. doi:10.1016/j.memsci.2021.119587
Kim, S. Y., Park, C. S., Hosseini, S., Lampert, J., Kim, Y. J., and Nazar, L. F. (2021b). Inhibiting oxygen release from Li-rich, Mn-rich layered oxides at the surface with a solution processable oxygen scavenger polymer. Adv. Energy Mat. 11 (30), 2100552. doi:10.1002/aenm.202100552
Kopeć, K., Wojasiński, M., Eichler, M., Genc, H., Friedrich, R. P., Stein, R., et al. (2022). Polydopamine and gelatin coating for rapid endothelialization of vascular scaffolds. Biomater. Adv. 134, 112544. doi:10.1016/j.msec.2021.112544
Lee, H. A., Ma, Y., Zhou, F., and Hong, S. (2019). Material-Independent surface chemistry beyond polydopamine coating. Acc. Chem. Res. 52 (3), 704–713. doi:10.1021/acs.accounts.8b00583
Lee, H. A., Park, E., and Lee, H. (2020). Polydopamine and its derivative surface chemistry in material science: A focused review for studies at kaist. Adv. Mat. 32 (35), 1907505. doi:10.1002/adma.201907505
Li, B., Liu, W., Jiang, Z., Dong, X., Wang, B., and Zhong, Y. (2009). Ultrathin and stable Active layer of dense composite membrane enabled by poly(dopamine). Langmuir 25 (13), 7368–7374. doi:10.1021/la900262p
Li, J.-H., Ni, X.-X., Zhang, D.-B., Zheng, H., Wang, J. B., and Zhang, Q. Q. (2018). Engineering a self-driven PVDF/PDA hybrid membranes based on membrane micro-reactor effect to achieve super-hydrophilicity, excellent antifouling properties and hemocompatibility. Appl. Surf. Sci. 444, 672–690. doi:10.1016/j.apsusc.2018.03.034
Li, J., Cao, F., Wu, B., Yang, J., Xu, W., Wang, W., et al. (2021a). Immobilization of bioactive vascular endothelial growth factor onto Ca-de fi cient hydroxyapatite-coated Mg by covalent bonding using polydopamine. J. Orthop. Transl. 30, 82–92. doi:10.1016/j.jot.2021.06.002
Li, S., Hou, X., Lu, S., Xu, W., Tao, J., Zhao, Z., et al. (2021b). Fabrication and simulation of a layered ultrahigh thermal conductive material made of self-assembled graphene and polydopamine on a copper substrate. RSC Adv. 11 (55), 34676–34687. doi:10.1039/D1RA05252G
Lin, Y., Zhang, L., Liu, N. Q., Yao, Q., Van Handel, B., Xu, Y., et al. (2019). In vitro behavior of tendon stem/progenitor cells on bioactive electrospun nanofiber membranes for tendon-bone tissue engineering applications. Int. J. Nanomedicine 14, 5831–5848. doi:10.2147/IJN.S210509
Liu, M., Zeng, G., Wang, K., Wan, Q., Tao, L., Zhang, X., et al. (2016). Recent developments in polydopamine: An emerging soft matter for surface modification and biomedical applications. Nanoscale 8 (38), 16819–16840. doi:10.1039/C5NR09078D
Liu, W., Sun, J., Sun, Y., Xiang, Y., Yan, Y., Han, Z., et al. (2020). Multifunctional injectable protein-based hydrogel for bone regeneration. Chem. Eng. J. 394, 124875. doi:10.1016/j.cej.2020.124875
Liu, Y., Ai, K., and Lu, L. (2014). Polydopamine and its derivative materials: Synthesis and promising applications in energy, environmental, and biomedical fields. Chem. Rev. 114 (9), 5057–5115. doi:10.1021/cr400407a
Lynge, M. E., van der Westen, R., Postma, A., and Stadler, B. (2011). Polydopamine—A nature-inspired polymer coating for biomedical science. Nanoscale 3 (12), 4916. doi:10.1039/c1nr10969c
Malollari, K. G., Delparastan, P., Sobek, C., Vachhani, S. J., Fink, T. D., Zha, R. H., et al. (2019). Mechanical enhancement of bioinspired polydopamine nanocoatings. ACS Appl. Mat. Interfaces 11 (46), 43599–43607. doi:10.1021/acsami.9b15740
Mei, H., Gao, Z., Wang, Q., Sun, H., Zhao, K., Zhang, P., et al. (2021). Ultrasound expands the versatility of polydopamine coatings. Ultrason. Sonochemistry 74, 105571. doi:10.1016/j.ultsonch.2021.105571
Mei, S., Xu, X., Priestley, R. D., and Lu, Y. (2020). Polydopamine-based nanoreactors: Synthesis and applications in bioscience and energy materials. Chem. Sci. 11 (45), 12269–12281. doi:10.1039/D0SC04486E
Merceron, T. K., Burt, M., Seol, Y.-J., Kang, H. W., Lee, S. J., Yoo, J. J., et al. (2015). A 3D bioprinted complex structure for engineering the muscle–tendon unit. Biofabrication 7 (3), 035003. doi:10.1088/1758-5090/7/3/035003
Montazerian, M., Hosseinzadeh, F., Migneco, C., Fook, M. V., and Baino, F. (2022). Bioceramic coatings on metallic implants: An overview. Ceram. Int. 48 (7), 8987–9005. doi:10.1016/j.ceramint.2022.02.055
Niculescu, A.-G., Chircov, C., Bîrcă, A. C., and Grumezescu, A. M. (2021). Fabrication and applications of microfluidic devices: A review. Int. J. Mol. Sci. 22 (4), 2011. doi:10.3390/ijms22042011
Nieto, C., Marcelo, G., Vega, M., and Martin del Valle, E. M. (2021). Antineoplastic behavior of polydopamine nanoparticles prepared in different water/alcohol media. Colloids Surfaces B Biointerfaces 199, 111506. doi:10.1016/j.colsurfb.2020.111506
Nishida, H., and Koga, F. (2018). Potential of oxidative polymerization coating of cellulose nano-fiber by dopamine. IOP Conf. Ser. Mat. Sci. Eng. 368, 012043. doi:10.1088/1757-899X/368/1/012043
Ou, J., Wang, J., Liu, S., Zhou, J., Ren, S., and Yang, S. (2009). Microtribological and electrochemical corrosion behaviors of polydopamine coating on APTS-SAM modified Si substrate. Appl. Surf. Sci. 256 (3), 894–899. doi:10.1016/j.apsusc.2009.08.081
Oymaci, P., Nijmeijer, K., and Borneman, Z. (2020). Development of polydopamine forward osmosis membranes with low reverse salt flux. Membranes 10 (5), 94. doi:10.3390/membranes10050094
Pagel, M., and Beck-Sickinger, A. G. (2017). Multifunctional biomaterial coatings: Synthetic challenges and biological activity. Biol. Chem. 398 (1), 3–22. doi:10.1515/hsz-2016-0204
Park, J., Lee, S. J., Jung, T. G., Lee, J. H., Kim, W. D., Lee, J. Y., et al. (2021). Surface modification of a three-dimensional polycaprolactone scaffold by polydopamine, biomineralization, and BMP-2 immobilization for potential bone tissue applications. Colloids Surfaces B Biointerfaces 199, 111528. doi:10.1016/j.colsurfb.2020.111528
Perez-Puyana, V., Wieringa, P., Yuste, Y., Portilla, F., Guererro, A., Romero, A., et al. (2021). Fabrication of hybrid scaffolds obtained from combinations of PCL with gelatin or collagen via electrospinning for skeletal muscle tissue engineering. J. Biomed. Mat. Res. A 109 (9), 1600–1612. doi:10.1002/jbm.a.37156
Proks, V., Brus, J., Pop-Georgievski, O., Vecernikova, E., Wisniewski, W., Kotek, J., et al. (2013). Thermal-induced transformation of polydopamine structures: An efficient route for the stabilization of the polydopamine surfaces. Macromol. Chem. Phys. 214 (4), 499–507. doi:10.1002/macp.201200505
Qian, Y., Zhao, X., Han, Q., Chen, W., Li, H., and Yuan, W. (2018). An integrated multi-layer 3D-fabrication of PDA/RGD coated graphene loaded PCL nanoscaffold for peripheral nerve restoration. Nat. Commun. 9 (1), 323. doi:10.1038/s41467-017-02598-7
Razaviye, M. K., Tafti, R. A., and Khajehmohammadi, M. (2022). An investigation on mechanical properties of PA12 parts produced by a SLS 3D printer: An experimental approach. CIRP J. Manuf. Sci. Technol. 38, 760–768. doi:10.1016/j.cirpj.2022.06.016
Richbourg, N. R., Peppas, N. A., and Sikavitsas, V. I. (2019). Tuning the biomimetic behavior of scaffolds for regenerative medicine through surface modifications. J. Tissue Eng. Regen. Med. 13 (8), 1275–1293. doi:10.1002/term.2859
Rizzo, F., and Kehr, N. S. (2021). Recent advances in injectable hydrogels for controlled and local drug delivery. Adv. Healthc. Mat. 10 (1), 2001341. doi:10.1002/adhm.202001341
Rodríguez-Rodríguez, R., Espinosa-Andrews, H., Velasquillo-Martínez, C., and Garcia-Carvajal, Z. Y. (2020). Composite hydrogels based on gelatin, chitosan and polyvinyl alcohol to biomedical applications: A review. Int. J. Polym. Mater. Polym. Biomaterials 69 (1), 1–20. doi:10.1080/00914037.2019.1581780
Saeed, M., Beigi-Boroujeni, S., Rajabi, S., Rafati Ashteiani, G., Dolatfarahi, M., and Ozcan, M. (2021). A simple, green chemistry technology for fabrication of tissue-engineered scaffolds based on mussel-inspired 3D centrifugal spun. Mater. Sci. Eng. C 121, 111849. doi:10.1016/j.msec.2020.111849
Salatin, S., Maleki Dizaj, S., and Yari Khosroushahi, A. (2015). Effect of the surface modification, size, and shape on cellular uptake of nanoparticles. Cell Biol. Int. 39 (8), 881–890. doi:10.1002/cbin.10459
Salomäki, M., Marttila, L., Kivelä, H., Ouvinen, T., and Lukkari, J. (2018). Effects of pH and oxidants on the first steps of polydopamine formation: A thermodynamic approach. J. Phys. Chem. B 122 (24), 6314–6327. doi:10.1021/acs.jpcb.8b02304
Schaubroeck, D., Mader, L., Dubruel, P., and Vanfleteren, J. (2015). Surface modification of an epoxy resin with polyamines and polydopamine: Adhesion toward electroless deposited copper. Appl. Surf. Sci. 353, 238–244. doi:10.1016/j.apsusc.2015.06.114
Sharma, D., Jia, W., Long, F., Pati, S., Chen, Q., Qyang, Y., et al. (2019). Polydopamine and collagen coated micro-grated polydimethylsiloxane for human mesenchymal stem cell culture. Bioact. Mater. 4, 142–150. doi:10.1016/j.bioactmat.2019.02.002
Szewczyk, J., Pochylski, M., Szutkowski, K., Kempinski, M., Mrowczynski, R., Iatsunskyi, I., et al. (2022). In-situ thickness control of centimetre-scale 2D-Like polydopamine films with large scalability. Mater. Today Chem. 24, 100935. doi:10.1016/j.mtchem.2022.100935
Terzopoulou, Z., Zamboulis, A., Koumentakou, I., Michailidou, G., Noordam, M. J., and Bikiaris, D. N. (2022). Biocompatible synthetic polymers for tissue engineering purposes. Biomacromolecules 23 (5), 1841–1863. doi:10.1021/acs.biomac.2c00047
Wang, Y., Chen, L., Ren, D.-Y., Feng, Z. X., Zhang, L. Y., Zhong, Y. F., et al. (2022). Mussel-inspired collagen-hyaluronic acid composite scaffold with excellent antioxidant properties and sustained release of a growth factor for enhancing diabetic wound healing. Mater. Today Bio 15, 100320. doi:10.1016/j.mtbio.2022.100320
Wei, Q., Zhang, F. L., Li, J., Li, B., and Zhao, C. (2010). Oxidant-induced dopamine polymerization for multifunctional coatings. Polym. Chem. 1 (9), 1430–1433. doi:10.1039/c0py00215a
Wu, M., Wang, T., Müller, L., and Muller, F. A. (2020). Adjustable synthesis of polydopamine nanospheres and their nucleation and growth. Colloids Surfaces A Physicochem. Eng. Aspects 603, 125196. doi:10.1016/j.colsurfa.2020.125196
Yan, J., Wu, R., Liao, S., Jiang, M., and Qian, Y. (2020). Applications of polydopamine-modified scaffolds in the peripheral nerve tissue engineering. Front. Bioeng. Biotechnol. 8, 590998–591007. doi:10.3389/fbioe.2020.590998
Yan, J., Yang, L., Lin, M., Ma, J., Lu, X., and Lee, P. S. (2013). Polydopamine spheres as active templates for convenient synthesis of various nanostructures. Small 9 (4), 596–603. doi:10.1002/smll.201201064
Yang, C., Huang, X., Huang, Y., Chen, Y., Wang, L., Zheng, X., et al. (2019). Characterization and in vitro experiments of composite membrane materials that polydopamine-loaded on the surface of collagen modified by a novel nanomaterial graphene oxide. Int. J. Polym. Analysis Charact. 24 (8), 741–751. doi:10.1080/1023666X.2019.1670396
Yang, H.-C., Luo, J., Lv, Y., Shen, P., and Xu, Z. K. (2015). Surface engineering of polymer membranes via mussel-inspired chemistry. J. Membr. Sci. 483, 42–59. doi:10.1016/j.memsci.2015.02.027
Yang, J., Cohen Stuart, M. A., and Kamperman, M. (2014). Jack of all trades: Versatile catechol crosslinking mechanisms. Chem. Soc. Rev. 43 (24), 8271–8298. doi:10.1039/C4CS00185K
Yazdi, M. K., Zare, M., Khodadadi, A., Seidi, F., Sajadi, S. M., Zarrintaj, P., et al. (2022). Polydopamine biomaterials for skin regeneration. ACS Biomater. Sci. Eng. 8 (6), 2196–2219. doi:10.1021/acsbiomaterials.1c01436
Yim, W., Borum, R. M., Zhou, J., Mantri, Y., Wu, Z., Zhou, J. V., et al. (2022). Ultrasmall gold nanorod-polydopamine hybrids for enhanced photoacoustic imaging and photothermal therapy in second near-infrared window. Nanotheranostics 6 (1), 79–90. doi:10.7150/ntno.63634
Yin, P., Liu, Y., Xiao, L., and Zhang, C. (2021). Advanced metallic and polymeric coatings for neural interfacing: Structures, properties and tissue responses. Polymers 13 (16), 2834. doi:10.3390/polym13162834
Yu, B., Pei, P., Yu, B., Li, D., Zhang, X., Huang, J., et al. (2017). Enhance the bioactivity and osseointegration of the polyethylene-terephthalate-based artificial ligament via poly(dopamine) coating with mesoporous bioactive glass. Adv. Eng. Mat. 19 (5), 1600708. doi:10.1002/adem.201600708
Zain, N. M., Hussain, R., and Abdul Kadir, M. R. (2015). Quinone-rich polydopamine functionalization of yttria stabilized zirconia for apatite biomineralization: The effects of coating temperature. Appl. Surf. Sci. 346, 317–328. doi:10.1016/j.apsusc.2015.04.007
Zeng, J., Wang, Y., Sun, Z., Chang, H., Cao, M., Zhao, J., et al. (2020). A novel biocompatible PDA/IR820/DAP coating for antibiotic/photodynamic/photothermal triple therapy to inhibit and eliminate Staphylococcus aureus biofilm. Chem. Eng. J. 394, 125017. doi:10.1016/j.cej.2020.125017
Zhang, F., and King, M. W. (2020). Biodegradable polymers as the pivotal player in the design of tissue engineering scaffolds. Adv. Healthc. Mat. 9 (13), 1901358. doi:10.1002/adhm.201901358
Zhang, L., Yang, G., Johnson, B. N., and Jia, X. (2019). Three-dimensional (3D) printed scaffold and material selection for bone repair. Acta Biomater. 84, 16–33. doi:10.1016/j.actbio.2018.11.039
Zheng, W., Fan, H., Wang, L., and Jin, Z. (2015). Oxidative self-polymerization of dopamine in an acidic environment. Langmuir 31 (42), 11671–11677. doi:10.1021/acs.langmuir.5b02757
Zhou, L., Ge, J., Wang, M., Chen, M., Cheng, W., Ji, W., et al. (2021). Injectable muscle-adhesive antioxidant conductive photothermal bioactive nanomatrix for efficiently promoting full-thickness skeletal muscle regeneration. Bioact. Mater. 6 (6), 1605–1617. doi:10.1016/j.bioactmat.2020.11.005
Zhou, L., Li, X., Wang, K., Shen, F., Zhang, L., Li, P., et al. (2020). Cu∥-loaded polydopamine coatings with in situ nitric oxide generation function for improved hemocompatibility. Regen. Biomater. 7 (2), 153–160. doi:10.1093/rb/rbz043
Zhou, P., Deng, Y., Lyu, B., Zhang, R., Zhang, H., Ma, H., et al. (2014). Rapidly-Deposited polydopamine coating via high temperature and vigorous stirring: Formation, characterization and biofunctional evaluation. PLoS ONE 9 (11), e113087. doi:10.1371/journal.pone.0113087
Zhou, X., OuYang, J., Li, L., Liu, Q., Liu, C., Tang, M., et al. (2019). In vitro and in vivo anti-corrosion properties and bio-compatibility of 5β-TCP/Mg-3Zn scaffold coated with dopamine-gelatin composite. Surf. Coatings Technol. 374, 152–163. doi:10.1016/j.surfcoat.2019.05.046
Keywords: polydopamine, surface modification, process parameters, tissue engineering, biomaterial, coating
Citation: Sarkari S, Khajehmohammadi M, Davari N, Li D and Yu B (2022) The effects of process parameters on polydopamine coatings employed in tissue engineering applications. Front. Bioeng. Biotechnol. 10:1005413. doi: 10.3389/fbioe.2022.1005413
Received: 28 July 2022; Accepted: 17 August 2022;
Published: 12 September 2022.
Edited by:
Behafarid Ghalandari, Shanghai Jiao Tong University, ChinaReviewed by:
Arturo E. Aguilar-Rabiela, Friedrich-Alexander Universität, GermanyXiaojun Zhou, Donghua University, China
Copyright © 2022 Sarkari, Khajehmohammadi, Davari, Li and Yu. This is an open-access article distributed under the terms of the Creative Commons Attribution License (CC BY). The use, distribution or reproduction in other forums is permitted, provided the original author(s) and the copyright owner(s) are credited and that the original publication in this journal is cited, in accordance with accepted academic practice. No use, distribution or reproduction is permitted which does not comply with these terms.
*Correspondence: Dejian Li, bGlkZWppYW44ODA4MjBAMTYzLmNvbQ==; Baoqing Yu, ZG9jdG9yeWJxQDE2My5jb20=
†These authors have contributed equally to this work and share second authorship