- 1Department of Orthopedics, China-Japan Union Hospital of Jilin University, Changchun, China
- 2Department of Nursing, The First Bethune Hospital of Jilin University, Changchun, China
- 3Scientific Research Center, China-Japan Union Hospital of Jilin University, Changchun, China
Meniscal injuries caused by trauma, degeneration, osteoarthritis, or other diseases always result in severe joint pain and motor dysfunction. Due to the unique anatomy of the human meniscus, the damaged meniscus lacks the ability to repair itself. Moreover, current clinical treatments for meniscal injuries, including meniscal suturing or resection, have significant limitations and drawbacks. With developments in tissue engineering, biopolymer scaffolds have shown promise in meniscal injury repair. They act as templates for tissue repair and regeneration, interacting with surrounding cells and providing structural support for newly formed meniscal tissue. Biomaterials offer tremendous advantages in terms of biocompatibility, bioactivity, and modifiable mechanical and degradation kinetics. In this study, the preparation and composition of meniscal biopolymer scaffolds, as well as their properties, are summarized. The current status of research and future research prospects for meniscal biopolymer scaffolds are reviewed in terms of collagen, silk, hyaluronic acid, chitosan, and extracellular matrix (ECM) materials. Overall, such a comprehensive summary provides constructive suggestions for the development of meniscal biopolymer scaffolds in tissue engineering.
1 Introduction
Meniscal tear is one of the commonplace injuries of the knee joint, which is often caused by trauma, degeneration, and osteoarthritis (Beaufils et al., 2017; Culvenor et al., 2019; Kopf et al., 2020). Partial or total meniscectomy and meniscus suture repair are commonly used to treat meniscus injuries and knee pain, but in terms of long-term prognosis, therapeutic effects are often limited (van de Graaf et al., 2018; Abram et al., 2020), and there are certain complications of treatment, such as cartilage injuries (Venjakob et al., 2019). Therefore, finding a safe and effective alternative therapy to repair meniscal tears is critical for orthopedists.
The repair capacity of the meniscus is related to the degree of vascular infiltration and structure. Compared to lateral edge of the meniscus, the medial edge lacks vascularity and therefore meniscus is less capable of self-repair. The complex anisotropic structure of meniscus increases the difficulty of self-repair. To adapt to compression, stretch, and torsion stresses of the knee from all directions at rest or during sports, the collagen arrangement of meniscus shows a gradient change. The continuous development of tissue engineering has made it possible to repair the meniscus perfectly. Tissue engineering can mimic the original natural tissue and stimulate the body’s regenerative potential to promote the growth of tissue into the scaffold and complete the gradual replacement to form functional tissue.
Recent researches have found that biopolymer scaffolds have favorable development foreground in the repair of a variety of tissue injuries (Sandri et al., 2019; Stoppel et al., 2015). They act as patterns for tissue repair and regeneration, interacting with surrounding cells and providing structural support for newly formed tissue (Hsu et al., 2016). Polymers of natural origin, such as collagen, silk, hyaluronic acid, chitosan, and extracellular matrix, are used for tissue engineering due to their good biocompatibility and bioactivity as well as their adjustable mechanical and degradation properties (Figure 1) (Stoppel et al., 2015; Wubneh et al., 2018; Zhang et al., 2019). And biopolymer scaffolds are receiving soaring attention from researchers in the treatment of meniscal injuries (Lin et al., 2017). For example, a collagen meniscus implant (CMI) made from type I collagen from the bovine achilles tendon has been used in clinical treatment (Stone et al., 1997). CMI provides good and consistent clinical effects, especially in terms of knee function and pain, with a low incidence of complications and reoperation (Grassi et al., 2014). However, meniscal scaffolds still have many problems to be solved (Veronesi et al., 2021), such as the lack of gradient bionic and insufficient mechanical properties. Although natural material scaffolds have many advantages in repairing meniscus, they can still be optimized in terms of gradient bionic structure, anti-inflammatory, and repair synergy to improve the repair efficiency (Hao et al., 2021). There is a wide variety of biopolymer scaffolds and most of them are still in the experimental stage, and we still lack a systematic understanding of their investigation. In this review, we refer to the research literature of recent years to systematically elucidate biopolymer meniscal scaffolds from various aspects.
2 Basic properties of the meniscus
2.1 Vascular, neural, and basic anatomy of the meniscus
The meniscus is unique in that it manifests as discrete cartilage-like tissue during embryonic life and subsequently depends on its location to be shaped by different external environmental stimuli. Ultimately, the vascular formation and innervation would be affected to develop into different tissue stratum. Signal transduction, vascular formation, innervation, stem cell origin, and location in the body all produce subtle tissue development differences. These distinctions guide the subpopulations of cells that will ultimately make up the tissue (Figure 2).
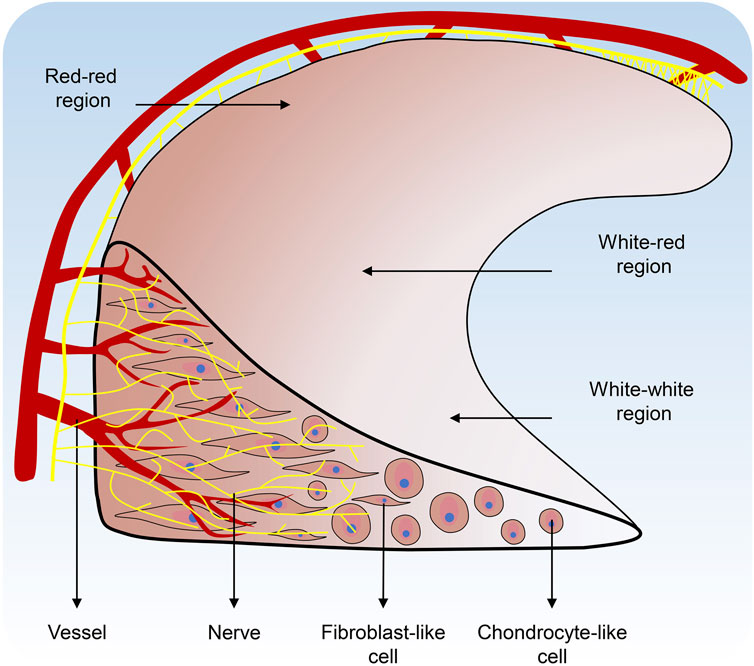
FIGURE 2. Regional variations in vascularization, nerve distribution, and cell populations of the Meniscus.
The capillary plexus surrounding the meniscus, which originates from the joint capsule and synovial tissue, provides 10–25% of the blood supply to the meniscus and is confined to the peripheral third of the meniscus (Day et al., 1985). The meniscus anterior and posterior horn attachments are covered by vascular synovial tissue, which provides an adequate blood supply to the meniscus (Arnoczky & Warren, 1982). The meniscus can be divided into three regions: the outer red-red region (vascular/nerve region), the inner white-white region (non-vascular/non-nerve region), and the transitional red-white region.
The nerves and mechanoreceptors of the meniscus are mainly located in the anterior and posterior horns of the meniscus and the lateral two-thirds of the body. The medial third of the meniscus is thin and lacks nerves (Gray, 1999). This particular nerve arrangement in the meniscus excites the internal mechanical drive system for proper joint alignment and biomechanical function when the meniscus is subjected to forces, resulting in a degree of pressure and tension on the meniscal surface (Zimny et al., 1988).
There is a significant difference in size between the lateral and medial meniscus in humans. The medial meniscus is C-shaped and covers 51%–74% of the area of the medial tibia plateau. The medial meniscus is slightly thinner in volume (Fox et al., 2015). It is more widely open to the medial intercondylar notch and has a relatively flat tibiofemoral articular surface (Renström & Johnson, 1990). The lateral meniscus is rounded and smaller but more flexible than the medial meniscus. It is located in the lateral intercondylar fossa of the tibial plateau and covers 75%–93% of the lateral tibial plateau (Kummer, 1987; Masouros et al., 2008).
2.2 Matrix microenvironment and biochemistry of the meniscus
The meniscus comprises cells and an extracellular matrix of collagen, proteoglycan, matrix glycoproteins, and elastin. The outer third of the Meniscus is dominated by fibroblasts and the inner third is by chondrocytes. The middle third of the meniscus has fibro chondrocytes (Markes et al., 2020). Collagen is the main fibrous component of the meniscus, mainly resists the tensile stress experienced by the meniscus, and its content varies greatly in the different regions of the meniscus. In the red-red region, type I collagen accounts for 80% of the dry weight (Cheung, 1987). The collagen fibers in the main body of the meniscus are arranged in circular patterns, allowing pressure loads to be distributed, while the surface and middle of the meniscus are radially distributed to resist longitudinal tears caused by external forces (Beaupré et al., 1986). Radial fibers are also present in the deeper regions of the meniscus, where they hold the circumferential fibers in place and maintain structural integrity (Shen et al., 2022). In the white-white region, collagen is composed of only Col II (60%) and Col I (40%) (Fox et al., 2012; Bansal et al., 2020).
Therefore, the meniscus is an anisotropic structure, which results in a low capacity for self-repair and the site of injury can influence the choice of treatment options for the orthopedic surgeon. According to the mechanism of injury, meniscal injuries can be classified as laminar, transverse, longitudinal, bucket handle, and oblique tears (Figure 3A). Only injuries that are limited to the vascular red-red and red-white zones are considered to have repair potential with meniscal suturing. Injuries involving the avascular white-white zone have low repair capacity, and orthopedic surgeons often opt for meniscectomy (Figure 3B) to address the patient’s pain. However, when the cartilage loses the protection of the meniscus, degenerative changes may occur and eventually the inevitable development of osteoarthritis.
3 Natural materials used for meniscus tissue engineering
Tissue engineering is the implantation of a scaffold loaded with cytokines or cells into tissue damage, and the gradual transformation of the scaffold into biological tissues, to achieve the purpose of repairing the damage. Scaffolds are the carriers of cytokines and cells. Therefore, as the key to tissue engineering, the selection of scaffold materials is very important. Compared with synthetic materials, natural materials have many significant advantages (Table 1), such as good biocompatibility and biodegradability, a structure similar to tissues, and the most important biological inducing activity. For the repair of the meniscus, good biocompatibility and degradability can make the material or the degradation products of the material not produce an inflammatory response, and the scaffold can be degraded as the tissue grows in. The structure similar to the tissue allows the normal tissue around the injury to better integrate with the repair tissue. The most important bio-inducing activity can maintain the consistency of the regenerated tissue with the original tissue, to restore the function perfectly.
However, homogeneous scaffolds are unable to meet the individualized repair of meniscus, so it is important to design scaffolds that properly guide meniscal repair according to the characteristics of each meniscal department. The fibrous arrangement of the natural meniscus should be simulated so that the meniscal scaffold can resist circumferential and radial stresses in the horizontal direction and compressive stresses in the vertical direction (Figure 4) (Berni et al., 2021). Bahcecioglu et al. 3D printed meniscal scaffolds that mimic the natural collagen arrangement, containing circumferential fibers in the periphery and crossed fibers in the interior. This scaffold exhibited increased compressive and tensile modulus and promoted region-specific protein expression (Bahcecioglu et al., 2019a). In the meantime, well-aligned fibers facilitate the directional migration of cells (Prendergast et al., 2021). In addition, if it involves the repair of the outer two-thirds of meniscus, the scaffold should have a gradient of pores. The gradient reduction of the scaffold pore size from lateral to medial facilitates vascular infiltration, thus allowing the vascular distribution of the repaired tissue to be consistent with that of the natural meniscus (Bahcecioglu et al., 2019a; Guo et al., 2021). We summarize the cases of various natural scaffolds in meniscal injury repair, describe the advantages and disadvantages of structure design of natural material scaffolds, predict the future development direction, and provide ideas for clinical translation of meniscal tissue engineering.
3.1 Collagen
Collagen is the most abundant ECM protein, with biodegradability, low immunogenicity, and adequate sources. Collagen self-assembles into cross-striated fibers that provide support for cell growth and are responsible for the mechanical elasticity of connective tissue (Sorushanova et al., 2019). Col I is the major collagen not only in the red-red region of the meniscus (Kwon et al., 2019), but also in mature tendons and ligaments, giving them weight-bearing mechanical properties (Varma et al., 2016). Based on the wide distribution of Col I in living organisms, its easy access to materials, and superior biological properties, it has great potential for meniscal scaffold development.
Meniscal cells derived from different regions, human bone marrow-derived mesenchymal stem cells (BMSCs), synovial cells, and cells from the infrapatellar fat pad were seeded onto aligned electrospun Col I scaffolds and optionally encapsulated in a hydrogel (Baek et al., 2018). Treatment of the scaffolds with transforming growth factor-β1 (TGF-β1) and TGF-β3 increased Col I deposition in all cell types and increased expression of COL1A1, cartilage oligomeric matrix protein (COMP), Tenascin C (TNC), and Scleraxis (SCX) genes (Baek et al., 2018). Meniscal cells seeded on collagen scaffolds produced higher levels of Col I than cells seeded on polylactic acid (PLA) (Baek et al., 2016), suggesting a potential advantage of biopolymer scaffolds in promoting collagen deposition. However, the initial mechanical properties of the collagen scaffold may not be as good as the natural meniscus based on the processing technic and the collagenic own characteristic (Meyer, 2019)
The ability of the injured meniscus to repair itself is limited to vascularized areas and is more susceptible to permanent post-traumatic and retrogression in internal non-vascular areas. Human meniscus cells were seeded and cultured on a Col I scaffold for 4 weeks. A collagen scaffold was implanted into a longitudinal tear injury model established in the avascular region of the bovine meniscus and incubated in vitro for 3 weeks. Cell-seeded collagen scaffolds showed better integration of natural tissue compared to cell-free collagen scaffolds or the control group (Baek et al., 2016). Histological analysis of in vivo animal experiments showed active integration of meniscus-like cartilage into a tissue-engineered biological scaffold in animal models. Active cellular resorption of collagen scaffold decreased over time. Besides, four cases showed mild nonspecific chronic inflammation, and another showed inflammatory engulfment of the scaffold with giant cells at 3 weeks. More importantly, no clinical or histological infections were found in all animal models at any time (Hansen et al., 2013).
Evidence suggests that treatment of meniscal injuries by implantation of a resorbable collagen scaffold may provide more satisfactory clinical outcomes than partial meniscectomy alone (Warth & Rodkey, 2015). The clinical prognosis after CMI implantation is good and relatively stable, particularly relating to restoring knee functions and relieving pain, and the incidence of postoperative complications and reoperations is low (Grassi et al., 2014). When used in combination with concomitant surgeries, such as anterior cruciate ligament reconstruction and high tibial osteotomy, short-term postoperative clinical outcomes improved in invalids who received CMI, with no significant difference between Actifit polyurethane meniscal scaffold and CMI (Houck et al., 2018). Follow-up results at least 10 years after surgery have shown that the implant size usually decreases, but the long-term outcome of the procedure is safe with a low rate of implant failure. Further progression of degenerative knee disease was not observed in most patients (Monllau et al., 2011). Postoperative magnetic resonance imaging (MRI) follow-up results showed an ongoing remodeling with reduced signal intensity and diminished size in CMI. However, since meniscus extrusion remained at the same level, bone marrow edema decreased from 1 year to longer follow-up and appeared to end remodeling at about 5 years after CMI (Schenk et al., 2020). Depending on the histology, new tissue growth to CMI may also be due to the process of synovial overgrowth, but other regenerative mechanisms may also be possible.
Collagen scaffolds provide strong mechanical strength and effectively promote meniscus regeneration (Pan et al., 2017). However, isolated collagen meniscal scaffolds still have certain limitations of their inherent limitation. For example, the operation and suturing of collagen implants are challenging (Kwon et al., 2019). Because the scaffold has a highly porous surface, which is essential for the differentiation and proliferation of fibrocartilage cells, sutures tend to cut through the implant, making good fixation difficult to achieve. Therefore, most postoperative complications of CMI are due to the suture material rather than the meniscal scaffold (Gwinner et al., 2017). This led us to think about the need to make collagen meniscus scaffolds work better by using a more refined tissue engineering strategy. Since the activation level of epidermal growth factor receptor (EGFR) is in an abnormally high state after meniscal injury, the combination of cell-free collagen scaffold and gefitinib enhances meniscal regeneration (Figures 5A,B A and B) (Pan et al., 2017). Photo-crosslinking using riboflavin and ultraviolet exposure increases the mechanical properties of collagen scaffolds and delays enzyme-triggered degradation of scaffolds (Figures 5C,D) (Heo et al., 2016; Koh et al., 2017). In general, the greatest advantage of collagen is its good biocompatibility, providing a good growth environment for the seed cells, but its lack of processability is also a significant disadvantage, so it needs to be modified or mixed with other materials.
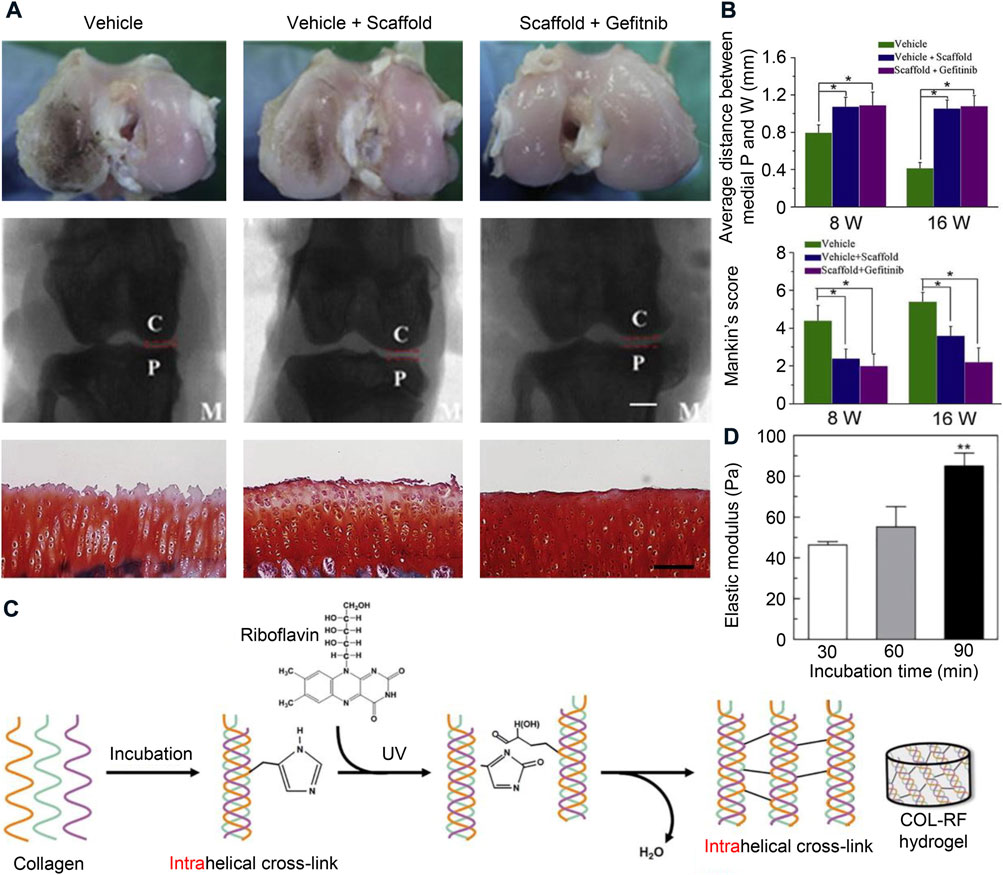
FIGURE 5. Collagen materials used for meniscus tissue engineering. (A) Gross morphology, radiological analysis, and histological staining of the femoral cartilage surface after implantation of collagen scaffold. Bar = 200 μm. C, condyles of femur; P, tibial plateau; M, medial of the knee joint. (B) Average distance between medial knee joint, and histological evaluation according to Mankin’s scoring system. Reprinted with permission (Pan et al., 2017). Copyright (2017), Elsevier. (C) Synthetic routes of riboflavin-induced photocrosslinked collagen (COL-RF) hydrogel. (D) The relationship between elastic modulus and incubation time. Reprinted with permission (Koh et al., 2017). Copyright (2017), Elsevier.
3.2 Silk
Silks are natural fibrin polymers (Huang et al., 2018), which embody superior material characteristics and biological functions achieved through a fine hierarchical structure. Through gentle and efficient processing, and careful and rational material design, they can be used to make high-performance, multifunctional, and well-biocompatible materials (López Barreiro et al., 2019). Although a complete study and analysis of the wide variety of silks remain difficult, regardless of the origin, silks are mainly composed of proteins and a few amounts of polysaccharides and lipids (Bhattacharjee et al., 2017). SF is a linear, water-insoluble protein, and could be combined with other biomaterials to form composites (Huang et al., 2018), while sericin is a globular, water-soluble glycoprotein (Chen et al., 2019). The advantages of silk include good biodegradability, biocompatibility, low immunogenicity (Wang et al., 2019), and the competence to remodel in vivo and serve on a pattern for natural tissue growth, resulting in vascularization and inward tissue growth.
Silk scaffolds have been widely used for meniscal tissue engineering studies. To generate meniscus-like tissues by in vitro experiments, a three-dimensional (3D) silk meniscal scaffold system was designed to mimic the natural meniscal structure by inoculating the scaffold with chondrocytes, human fibroblasts, or adipose-derived stem cells in a spatial separation pattern similar to that of natural tissues (Mandal et al., 2011a; Yang et al., 2017; Ying et al., 2018). The degree of silk scaffold remodeling, tissue inward growth, or other specific cellular behavior can be regulated by increasing growth factors or other signaling factors (Thurber et al., 2015). In a chondrogenic culture in the presence of TGF-β3, cell-seeded constructs increased in cellularity and ECM content. Histology and immunohistochemistry confirmed that having lofty levels of sulfated GAG and type I and II collagen maintained the chondrocyte phenotype (Mandal et al., 2011a). Elevated levels of COL1A1, aggrecan, and SOX9 gene expression further confirmed the differentiated and mature cell phenotype (Mandal et al., 2011b). The compressive elastic modulus of the silk scaffold increased significantly with incubation time (Yang et al., 2017). These in vitro experiments suggest that porous silk structures can serve as an effective micro-patterned template for endogenous cell migration, proliferation, and differentiation during the fresh meniscus-like tissue forming process.
In vivo studies have confirmed that transplantation of different forms of silk products into muscles, skins, bones, or brains showed good biocompatibility (Uebersax et al., 2013; Fernández-García et al., 2016; Ju et al., 2016), causing only low inflammatory responses, such as low infiltration of inflammatory cells, and that these responses were usually transient, this normal response decreases within several weeks after implantation. Depending on the properties of the material and the implantation site, which involves macrophage recruitment and activation, a mild foreign body reaction can result in the formation of multinucleated giant cells (Thurber et al., 2015). All mice were in good condition with no adverse reaction after 4 weeks of subcutaneous transplantation of three types of SF scaffolds, either decellularized, implanted with human meniscus cells, or implanted with human adipose-derived stem cells, into 5-week-old male nude mice (Cengiz et al., 2019). Bandyopadhyay A et al. implanted cell-free scaffolds into the subcutaneous abdominal pocket of SD rats. Enhanced degradation and absorption of structures were observed 14 days after implantation of scaffolds compared to day 7, and they had excellent immunocompatibility (Bandyopadhyay & Mandal, 2019). H&E stained images and Masson’s trichrome stained images show that all scaffolds have a high degree of excellent tissue infiltration, angiogenesis, and collagenous ECM formation. And cross-sections show a homogeneous appearance throughout the sample (Cengiz et al., 2019). A partial meniscectomy was operated on the medial meniscus of sheep and an SF scaffold was implanted into the defect. The joint caused no inflammatory reaction 6 months after surgery, and there was no significant difference in cartilage degeneration between the experimental and sham-operated groups. The compression properties of the scaffold were close to those of natural meniscal tissue (Gruchenberg et al., 2014). Nevertheless, the compressive stiffness of the scaffold is greatly increased after implantation, which may interfere with the permanent binding of sents to the meniscal tissue and reduce the chondroprotection of the underlying cartilage (S. E. C. Stein S. et al., 2019; Warnecke et al., 2018). The current study demonstrates the SF scaffold has the attribute of significantly improving tibiofemoral contact pressures, with up to 81% of tibiofemoral contact pressures being transmitted through the meniscus, within the knee after partial meniscectomy (S. Stein S. E. C. et al., 2019). However, the failure of the SF scaffold to fully recapitulate the contact area and pressure of the intact meniscus, especially at high flexion angles, suggests that the biomechanical properties of the scaffold may need further improvements to fully restore tibiofemoral contact mechanics (S. Stein et al., 2019a). It has been demonstrated in friction tests that SF hydrogels have a friction response similar to that of cartilage, dominated by this interstitial fluid support (Parkes et al., 2015). To elucidate the tribological properties of the fibroin meniscal scaffold, the friction of the implant against cartilage and glass was tested. The coefficient of friction between the silk scaffold and cartilage is 0.056, which is greater than the natural meniscus, but less than the threshold required for meniscus replacement (Warnecke et al., 2017).
Silk fibroin scaffolds still have some shortcomings. The mechanical properties of pure SF scaffolds after freeze-drying are usually unsatisfactory. Fortunately, the biodegradability and mechanical characteristics of SF can be modulated by other suitable polymers (Bandyopadhyay & Mandal, 2019; Y. Li Y et al., 2020; Z. Li Z et al., 2020). For instance, the internally coated collagen enhanced the biocompatibility of the silk sponge, but the outer layer of the collagen would increase the friction properties (Yan et al., 2019). SF and strontium were incorporated with Ɛ-Polycaprolactone by wet-electrospinning method to manufacture the meniscus scaffold, which enhances the secretion of collagen and aggrecan (Figure 6) (Y. Li Y et al., 2020). The combination of silk and cellulose significantly improved the toughness of the hydrogel and promoted the biological activity of fibroblasts (Dorishetty et al., 2020). Compared to collagen, silk proteins are better processed, but silk proteins form β-sheets autonomously, which affect the mechanical properties of the scaffold.
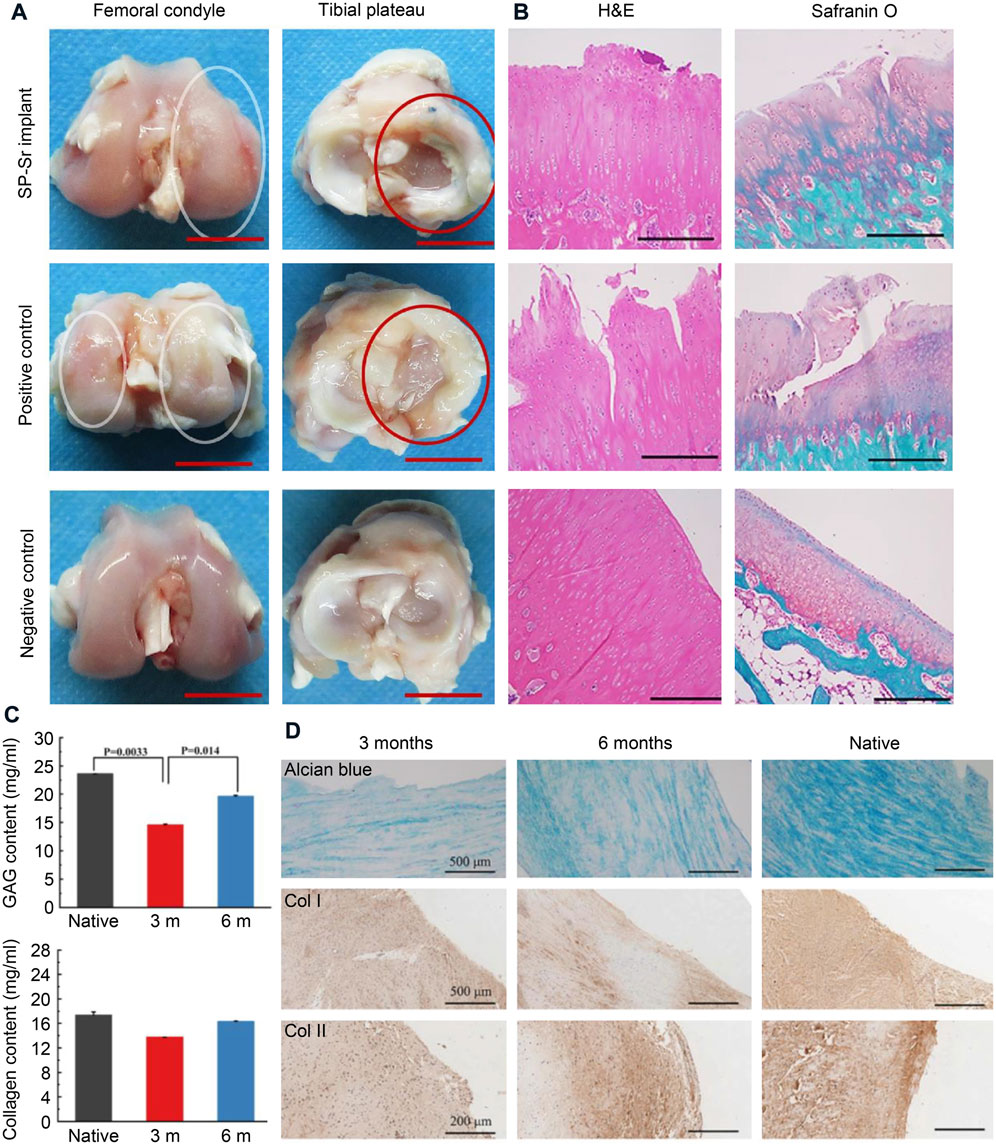
FIGURE 6. Silk materials used for meniscus tissue engineering. (A) Macroscopic observations of regenerated menisci and the corresponding femoral condyles. The red ellipses referred to the meniscus and the white ellipses referred to worn cartilage. Bars = 1 cm. (B) H&E and Safranin O-Fast Green staining of articular cartilage surfaces in the femur cartilage. Bars = 500 μm. (C) The total GAG and collagen content were estimated in native meniscus and neomeniscus. (D) The alcian blue and immunohistochemical staining in native meniscus and neomeniscus. Reprinted with permission (Y. Li Y et al., 2020). Copyright (2017), Elsevier.
3.3 Hyaluronic acid
Hyaluronic acid (HA) is a GAG consisting of the repeating disaccharide portion of d-glucuronic acid and N-acetyl-d-glucosamine and is found in large amounts in the skin and musculoskeletal tissues. Hyaluronic acid has been widely used in tissue regeneration and medicine due to its superior physiological function and biocompatibility. It also has a well-controlled degradation rate and mechanical properties. HA alone has a relatively fast degradation rate and weak mechanical strength, and the most common modification method is the reaction of methacrylic anhydride with HA to form methacrylated hyaluronic acid (MeHA), which has photocrosslinking properties (Kenne et al., 2013). The photocrosslinked HA has a slower degradation rate and higher mechanical stage and is related to the degree of methyl substitution (D'Amora et al., 2018). Zhang et al. obtained a series of photocurable hydrogels with different degrees of crosslinking by adding different concentrations of the water-based tetrathiol crosslinker (PE(NAC)4) to MeHA, which had different gelling time, mechanical strengths, and degradation rates (Zhang et al., 2020). In addition, HA has functional groups that can be chemically modified, carboxyl and hydroxyl. The carboxyl of glucuronic acid is a good target for 1-ethyl-3- [3-(dimethylamino)-propyl]-carbodiimide (EDC) mediated amide and ester formation of HA (Knopf-Marques et al., 2016). Kim et al. covalently bound tyramine (TA) to hyaluronan by EDC and mixed it with gelatin to obtain hydrogels mediated by tyrosinase (TYR) cross-linking. Compared to gelatine alone, mixtures of HA and gelatine in the presence of TYR have faster gel transformation time and higher mechanical properties, and the mechanical peculiarities and degradation kinetics were regulated by TA substitution and TYR concentration (Kim et al., 2018).
HA and its modifications have been widely used to repair with good results. Intra-articular injection of high molecular weight HA has a positive therapeutic effect on osteoarthritis or acute cartilage injury, and it regulates the expression of a variety of mi-RNAs that are associated with cartilage degeneration, apoptosis, and inflammation (Genemaras et al., 2018). However, viscosupplementation therapy prevents macroscopic damage to the meniscus but does not recover its collagen fibrous tissue and viscoelasticity (Levillain et al., 2017). However, cell proliferation and migration were observed when HA was added to the culture medium to stimulate meniscal cells. Not only that, but when the injured meniscal tissue was cultured in cultures containing HA, the accumulation of collagen II around the tear was also observed, but collagen I accumulation around the meniscal injury site was not induced (Tanaka et al., 2017). Thus, the use of HA as raw material and the application of tissue engineering methods to repair damaged meniscus have become a recent research hotspot. Ghodbane et al. injected collagen HA sponge as an extracellular matrix into the meniscal scaffold, which matched the circumferential tensile and axial compressive properties of the natural meniscus and had appropriate porosity (Ghodbane et al., 2019a). At 24 weeks after implantation, inward cell growth was observed, producing dense tissue liked fibrocartilage with significant collagen and GAG deposition. On the downside, some of the scaffolds were displaced postoperatively, resulting in low meniscal repair and loss of meniscal protective effect on articular cartilage (Ghodbane et al., 2019b). Bahcecioglu et al. inoculated porcine fibrocartilage cells with agarose, methacrylated gelatin (GelMA), and MeHA, hybrid hydrogels, and 3D printed PCL scaffolds, and then evaluated the potential for ex vivo meniscal regeneration under dynamic compression. The results showed that hydrogels had a higher potential for meniscal regeneration compared to PCL, with agarose and MeHA favoring the regeneration of the inner meniscal region and gelatin favoring the regeneration of the outer meniscal region. (Bahcecioglu et al., 2019b). Song et al. synthesized soft and stiff MeHA scaffolds by electrostatic spinning, in which stiffness was varied by the degree of MeHA cross bonding. The adhesion and migration of meniscal fibrochondrocytes (MFC) to the fibermesh were also investigated, in which the softer MeHA fibermesh was susceptible to deformation and densification by cell traction, whereas the stiffer MeHA fibrous network supported more MFC invasion when placed near the meniscal tissue (Song et al., 2020). In conclusion, the use of HA scaffolds for tissue repair is a promising approach, and enough investigation has been done on this material, yet its use in meniscal repair is often used as an adjunct to synthesis (Halili et al., 2014; Heo et al., 2016). Most notably because of its good biocompatibility but weak mechanical strength, although the suitability of HA has been enhanced by various modifications, the potential damage of these modifiers to the tissue has yet to be investigated.
3.4 Chitosan
Chitosan (CS) is a biopolymer made by deacetylation of chitin with a structure similar to GAG. CS has a wide range of applications in biomedical fields due to its splendid biodegradability, bioactivity, and biocompatibility. CS can provide active sites for cell adhesion, cell-cell, and cell-matrix interactions, which in turn plays an important role in regulating cell proliferation and function. The cationic property of CS allows for the immobilization of negatively charged enzymes, proteins, GAGs, polysaccharides, or other negatively charged molecules under mildly acidic conditions (Sultankulov et al., 2019). CS can also form interconnected porous structures after lyophilization and allows for large numbers of cells to be inoculated at once, improving cell migration and proliferation. The in vivo degradation of CS also shows synergistic effects on the long-term performance of tissue engineering cells by affecting many cellular processes such as cell growth, tissue regeneration, and host response (Ahmed et al., 2018). The molecular chain of CS contains reactive amino and hydroxyl making it susceptible to chemical modifications. Combining the major ECM molecules, collagen and chondroitin-6-sulfate, onto a hyaluronic acid/chitosan multilayer can be used as a bionic surface for meniscus repair to reverse meniscus cell dedifferentiation (Tan et al., 2010).
Nevertheless, the properties of isolated CS scaffold materials have some limitations in the preparation of meniscal scaffolds, and the modification of CS is currently a hot spot for research in the field of biomaterials. Sarem M et al. prepared chitosan/gelatin composite scaffolds exhibiting highly inter-connected pore architecture with swelling and degradation behavior using genipin as a biocompatible cross-linking agent. Gelatin improves the biocompatibility of CS, and gelatin/chitosan scaffolds have excellent compatibility with meniscus-derived cells (Sarem et al., 2013). In addition, the chitosan/calcium polyphosphate composite scaffold enhances the necessary mechanical properties of the meniscal scaffold. The degradation rate of the scaffold can be controlled by varying the amount of calcium polyphosphate to avoid premature degradation and ensure that the scaffold is filled with new tissue. Moradi et al. produced a polyvinyl alcohol/chitosan scaffold that compared the meniscal repair effects of adipose-derived stem cells and articular chondrocytes. The scaffold inoculated with chondrocytes promoted meniscal regeneration well and improved the mechanical properties of the regenerated meniscal tissue (Moradi et al., 2017).
Overall, CS has good properties and is a significant element of scaffold material research and one of the future research directions in the field of biomaterials. Researchers are continuously exploring the potential of CS as a biomaterial, aiming to eliminate the limitations of existing materials. New CS derivatives and composites could certainly make it an ideal scaffold material.
3.5 Extracellular matrix
ECM is acellular component of tissues that provides scaffolding for cells and regulates normal tissue morphogenesis, differentiation, matrix renewal, and dynamic homeostasis (Swinehart & Badylak, 2016). Bioactive molecules that maintain tissue homeostasis and promote regeneration are present in ECM and can act through a range of cell-cell and cell-matrix interactions (Watt & Huck, 2013; Monibi & Cook, 2017). The tissue-derived stroma has a guiding function in the differentiation of stem cells (Rothrauff et al., 2017; Yuan et al., 2017). A specific comparison of the effects of meniscus-derived ECMs (mECMs) on hBMSCs in different regions showed that internal mECMs promoted fibrocartilage differentiation of hBMSCs, whereas external mECMs enhanced more fibroblast phenotypes (Shimomura et al., 2017). The meniscus of rats, sheep, pigs, and humans can be decellularized using numerous techniques, including physical, (freeze-thawing and sonication), chemicals (detergents, EDTA, and hypotonic buffers), biological (enzymatic digestion), and their combinations (Chen et al., 2017; Monibi & Cook, 2017). In vitro experiments are remarkable for testing the performance of ECM meniscal scaffolds. Porcine meniscus-derived matrixes (MDM) were prepared and tested for their effectiveness in promoting meniscal repair by migrating endogenous meniscus cells from the surrounding meniscus or exogenously seeded hBMSCs. Both endogenous meniscus cells and hBMSCs permeated into the MDM scaffold. In the absence of exogenous cells, 8% of the MDM scaffold promoted the overall repair of meniscal defects in vitro (Ruprecht et al., 2019). In vitro experiments to assess cytotoxicity and cytocompatibility of ECM meniscal scaffold, synovial cells, and meniscal chondrocytes were seeded onto decellularized meniscal scaffolds, after 8 days of incubation under basic conditions, the scaffold was not cytotoxic to either cell. In an in vitro model of meniscal repair, ECM-derived scaffolds were inoculated into excised menisci and cultivated for 42 days, and histological evaluation showed normal tissue architecture and cellularity of meniscus (Monibi et al., 2016). In addition, the scaffolds were implanted subcutaneously into rats for 7, 14, and 28 days. No inflammatory cells were detected to infiltrate the implant. The structure of the scaffold was stable for more than 14 days. Absorption and turnover of the scaffolds were observed on day 28. The tissue around the implant showed no signs of inflammation and was comparable to the sham operation control group (Chen et al., 2015).
The intermediate region of the bovine meniscus is an excellent material for the preparation of ECM scaffolds. The collagenous fibrils of the meniscal fibrocartilage in the middle zone become more highly oriented perpendicular to the direction of compression (Sizeland et al., 2020). The decellularized porcine medial meniscus maintains the tensile biomechanical properties of the native meniscus but has lower elastic moduli in tension and compression compared to the native meniscus. These changes in biomechanical properties may be due to the reduction of GAG content during decellularization (Abdelgaied et al., 2015). GAG plays a significant role in water regulation within the meniscus. Although some compositional changes in the ECM are to be expected during processing, it is clear that numerous essential structural components remained functional while maintaining biomechanical properties (Stabile et al., 2010). Demineralized cancellous bone (DCB) is an extensively used bioactive scaffold for tissue engineering with a natural 3D porous structure and pretty biocompatibility (Wang et al., 2016). Acellular meniscus extracellular matrix (AMECM) and DCB were used to construct different types of triaxial porous meniscal scaffolds, and the AMECM and DCB played a good synergistic repair effect on the meniscus (Figure 7) (Yuan et al., 2016).
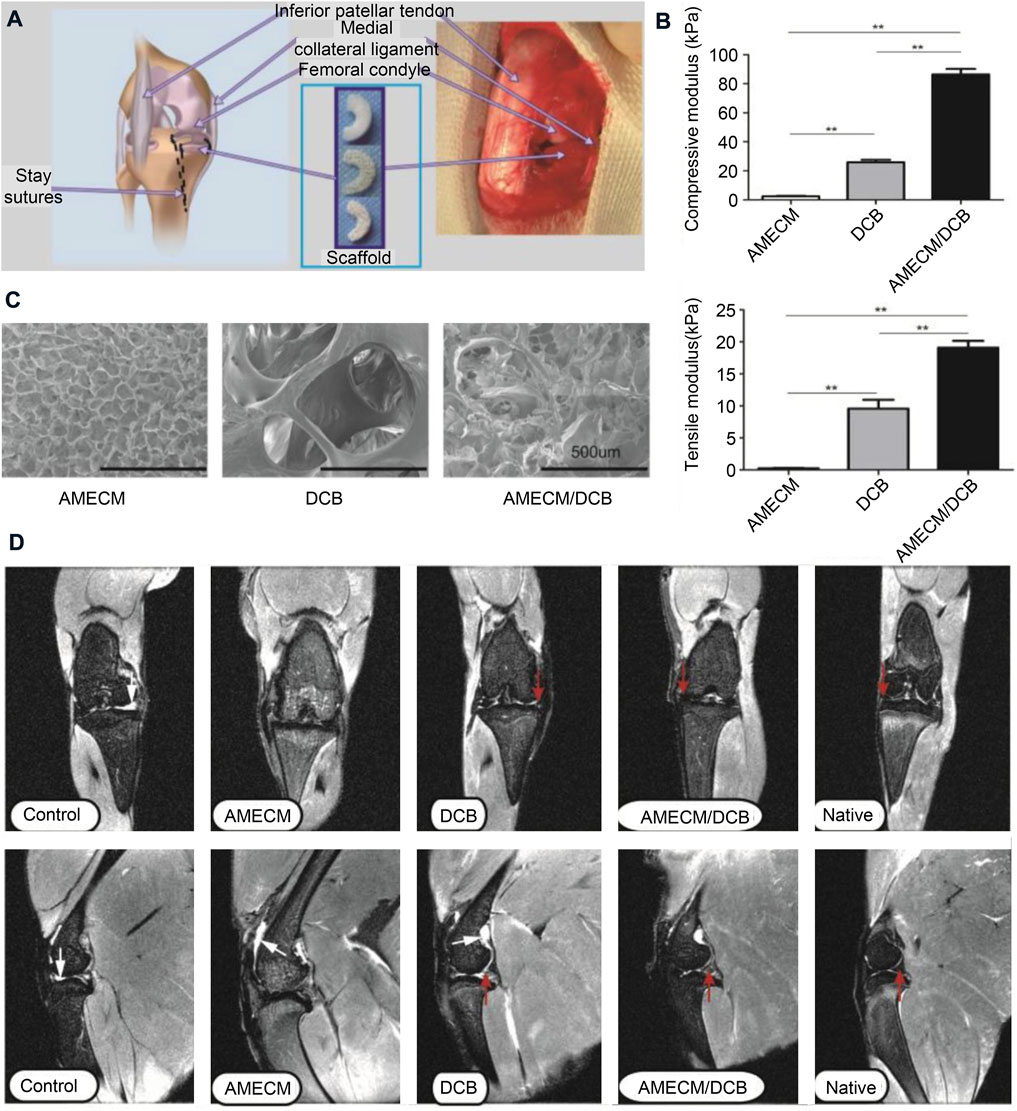
FIGURE 7. Extracellular matrix used for meniscus tissue engineering. (A) Surgical strategies for repairing meniscus with extracellular matrix scaffolds. (B) Comparative mechanical modulus of the different scaffolds. (C) Scanning electron micrographs of the different scaffolds. (D) Magnetic resonance imaging (MRI) of rabbit knees 6 months after surgery. The red arrow indicates the regenerated menisci. Reprinted with permission (Yuan et al., 2016). Copyright (2016), Elsevier.
Injectable hydrogels can be derived from tissue-specific ECM, thus providing a bionic environment for cell delivery and allowing seamless regeneration of tissue defects (Yuan et al., 2017). An injectable ECM hydrogel material was developed in the porcine meniscus. This meniscus-derived ECM hydrogel displays a fibrous morphology with adjustable compressive force and initial modulus. The hydrogel promoted the growth of bovine chondrocytes and mouse 3T3 fibroblasts, showing good biocompatibility. Furthermore, the subcutaneous implantation dedicated that the hydrogels were favorable for cell infiltration. (Wu et al., 2015). Decellularized ECM retains essential proteoglycans and collagen and encapsulates BMSCs in ECM or hydrogels. When applied to an orthotopic model of meniscal injury in SD rats, ECM outperformed collagen I scaffold in reducing bone redundancy formation and preventing narrowing of joint space and osteoarthritis development, as demonstrated in histological and micro-CT analyses(Zhong et al., 2020). Although the mECM had higher concentrations of TGF-β and bFGF (Rothrauff et al., 2017), mECM hydrogels did not significantly support fibrochondrogenesis of hMSCs in the absence of TGF-β3, with a decrease in cell numbers and OHP content of hydrogels, and the lack of sulfated GAG production (Yuan et al., 2017).
However, the traditional AMECM preparation process ignores the regionality of the meniscus and processes the entire meniscus into a homogeneous scaffold. It is important to maintain the regional specificity of meniscus. Yun et al. decellularized the inner cartilage region, transition region, and outer fibrous region of the porcine meniscus, respectively, to prepare a meniscal scaffold with a composition gradient. After this scaffold was implanted in vivo, the expression of region-specific proteins was induced, with a large amount of GAG secreted on the inner side and fibrin mainly on the outer side (Yun et al., 2021). Therefore, ACECM is also an ideal material for meniscal tissue engineering, not only for its biocompatibility and ability to promote stem cell differentiation, but also for its ability to be processed into structurally controlled scaffolds through various techniques.
4 Future directions and conclusion
There is no perfect method for meniscus injury in clinical practice. Meniscectomy removes the damaged part of the meniscus, which to some extent solves the patient’s pain and joint interlocking symptoms, but it may cause direct contact with articular cartilage, leading to more serious cartilage wear, and eventually osteoarthritis. Meniscus suture surgery requires very high postoperative rehabilitation for patients. Patients who have undergone this procedure need to strictly control the angle of joint flexion and extension, and the timing of lower limb weight-bearing. For patients with large meniscus injuries, if they have stable, correctly aligned joints and are in the early or earlier stages of arthritis, it is best to use meniscus replacement. Although it can significantly improve the prognosis of patients, the source of the allogeneic meniscus is very scarce, and artificial meniscus may become the key to meniscus injury repair. A large number of artificial meniscuses have been developed, among which natural material meniscus prosthesis accounts for a large proportion due to its good biocompatibility and biological activity. Various natural materials have their advantages and disadvantages, decellularized ECM is taken from the natural meniscus, and by partitioning decellularization, a scaffold with a highly similar structure to the natural meniscus can be prepared, but the clinical translation must face ethical review; collagen and hyaluronic acid are also components of the organism and are biocompatible, but they need to be modified or mixed with other polymers to improve processability; well-processed silk proteins and chitosan are also ideal materials for meniscal scaffolds, but they are not mammalian in origin and may cause inflammatory reactions in the organism.
Although many kinds of natural materials have been developed to repair the meniscus and achieved some gratifying results. But it is undeniable that there is still a long way to go before the clinical transformation of natural materials meniscus tissue engineering. Natural materials have advantages over synthetic materials in terms of access, biocompatibility, degradation, etc. As the therapeutic power of seed cells is gradually explored, more accurate and efficient loading of seed cells will play a more important role in tissue engineering, and natural materials are ideal carriers for seed cells. With advances in 3D printing technology, such as the development of stereo lithography appearance printing and bio-ink printing, the processing performance of natural materials has also improved considerably. Although it still does not reach the level of controllability of synthetic materials, the mixture of natural and synthetic materials is also an effective way to combine the advantages of both, such as synthetic materials as a skeleton and natural materials as a filler. Therefore, natural materials are ideal for meniscal tissue engineering, but how to improve natural materials for better repair and higher efficiency is still an important trend for natural materials in the future.
Then, the complex regionalized structure of the meniscus is also one of the difficulties of repair. The white-white area of the meniscus has no blood vessels, so its inherent repairability is very poor. This also leads to a defect in this area that cannot be repaired well even in the presence of the meniscus scaffold. This requires future research to focus on the repair of the white-white area. Perhaps finding clues for the specific differentiation of stem cells into the chondrocyte-like cell of the white-white area is the key to the white-white area repair, including mechanical stimulation, differentiation factors, and special arrangements of materials, etc. The red-white area is the junction of the red-red area and the white-white area. The cell phenotype and collagen arrangement gradually change in this area, so it has the function of two areas in meantime. In the face of meniscus damage that spans the red-white zone, reconstructing the gradient of cells and collagen in this area is conducive to the integration of tissues and surroundings, better transmitting the stress on the meniscus, and transforming radial force into circumferential force, to avoid stress concentration. The red-red area is the area rich in blood vessels. The main point of repair in this area is to expand the porosity of the scaffold as much as possible under the premise of ensuring the mechanical strength, to facilitate the penetration and metabolism of nutrients.
As for the entire replacement of the meniscus, it is still a difficult problem. As mentioned above, the three areas of the meniscus have different repair points. Therefore, it is necessary to meet the repair requirements of the three areas on a scaffold. It is best to include some gradient signals that simulate the natural meniscus. Future research directions should design pore size gradients to influence the infiltration of meniscal vessels and precisely replicate the vessels distribution; mechanical strength gradients should also follow the force analysis of the natural meniscus to simulate the mechanical properties of each region, resulting in a collagen arrangement consistent with the natural structure; or load gradient inducing factors to promote regional differentiation of stem cells to form functionally compatible tissues.
In addition, inflammatory and oxidative reactions from meniscal injuries and implantation cannot be ignored, and attention to the immune microenvironment of injured area is an important future direction. Natural materials have good biocompatibility and degradation products can participate in the normal metabolism process, so they won’t cause excessive inflammation or oxidative reactions. In addition, although inflammatory and oxidative responses do not persist throughout the meniscal repair process, modulating the immune microenvironment and terminating the inflammatory response at the right time can improve the efficiency of meniscal repair. A good transition between anti-inflammation and repair can be achieved by releasing anti-inflammatory and inducing agents in a consistent sequence, and the difference in material degradation rates can also be used to construct an integrated anti-inflammatory-repair meniscal scaffold.
In conclusion, the research on the tissue engineering of the meniscus needs to be further in-depth, mainly including the following aspects: 1) Continuing to optimise the materials used in the preparation of meniscal scaffolds; 2) A bionic integrated meniscus prosthesis based on the differences in the various regions of the meniscus; 3) In-depth study of the mechanism of differentiation of stem cells into different cell phenotypes of the meniscus (mechanical stimulation, differentiation factors); 4) Observe the long-term repair effect of the meniscus scaffold implantation.
Author contributions
YP and ML have contributed equally to this work and share the first authorship. YZ, TL and JZ contributed to conception and design. YP, ML, ZZ and CW wrote the manuscript. YP designed the figures and the table. LE performed literature search, and provided valuable comments. All authors contributed to the article and approved the final manuscript.
Funding
This work was supported by the Science and Technology Department Program of Jilin Province (Grant Nos. YDZJ202201ZYTS520, 20190304124YY, and 20190304121YY) and the Department of Finance of Jilin Province (No. 2020SCZ53).
Conflict of interest
The authors declare that the research was conducted in the absence of any commercial or financial relationships that could be construed as a potential conflict of interest.
Publisher’s note
All claims expressed in this article are solely those of the authors and do not necessarily represent those of their affiliated organizations, or those of the publisher, the editors and the reviewers. Any product that may be evaluated in this article, or claim that may be made by its manufacturer, is not guaranteed or endorsed by the publisher.
Abbreviations
3D, three dimension; AC, articular chondrocyte; ADCS, adipose- derived stem cell; BMSC, bone mesenchymal stem cells; Col, collagen; CS, chitosan; DCB, demineralized cancellous bone; ECM, extracellular matrix; GAG, glycosaminoglycan; IPFP, infrapatellar fat pad; MDM, meniscus-derived matrix; PCL, polycaprolactone; PRP, platelet riched plasma; PVA, polyvinyl alcohol; TGF-β, transforming growth factor-β; TMSC, tonsil-derived mesenchymal stem cells.
References
Abdelgaied, A., Stanley, M., Galfe, M., Berry, H., Ingham, E., and Fisher, J. (2015). Comparison of the biomechanical tensile and compressive properties of decellularised and natural porcine meniscus. J. Biomech. 48 (8), 1389–1396. doi:10.1016/j.jbiomech.2015.02.044
Abram, S. G. F., Hopewell, S., Monk, A. P., Bayliss, L. E., Beard, D. J., and Price, A. J. (2020). Arthroscopic partial meniscectomy for meniscal tears of the knee: A systematic review and meta-analysis. Br. J. Sports Med. 54 (11), 652–663. doi:10.1136/bjsports-2018-100223
Ahmed, S., AnnuAli, A., and Sheikh, J. (2018). A review on chitosan centred scaffolds and their applications in tissue engineering. Int. J. Biol. Macromol. 116, 849–862. doi:10.1016/j.ijbiomac.2018.04.176
Arnoczky, S. P., and Warren, R. F. (1982). Microvasculature of the human meniscus. Am. J. Sports Med. 10 (2), 90–95. doi:10.1177/036354658201000205
Baek, J., Sovani, S., Choi, W., Jin, S., Grogan, S. P., and D'Lima, D. D. (2018). Meniscal tissue engineering using aligned collagen fibrous scaffolds: Comparison of different human cell sources. Tissue Eng. Part A 24 (1-2), 81–93. doi:10.1089/ten.TEA.2016.0205
Baek, J., Sovani, S., Glembotski, N. E., Du, J., Jin, S., Grogan, S. P., et al. (2016). Repair of avascular meniscus tears with electrospun collagen scaffolds seeded with human cells. Tissue Eng. Part A 22 (5-6), 436–448. doi:10.1089/ten.TEA.2015.0284
Bahcecioglu, G., Bilgen, B., Hasirci, N., and Hasirci, V. (2019a). Anatomical meniscus construct with zone specific biochemical composition and structural organization. Biomaterials 218, 119361. doi:10.1016/j.biomaterials.2019.119361
Bahcecioglu, G., Hasirci, N., Bilgen, B., and Hasirci, V. (2019b). Hydrogels of agarose, and methacrylated gelatin and hyaluronic acid are more supportive for in vitro meniscus regeneration than three dimensional printed polycaprolactone scaffolds. Int. J. Biol. Macromol. 122, 1152–1162. doi:10.1016/j.ijbiomac.2018.09.065
Bandyopadhyay, A., and Mandal, B. B. (2019). A three-dimensional printed silk-based biomimetic tri-layered meniscus for potential patient-specific implantation. Biofabrication 12 (1), 015003. doi:10.1088/1758-5090/ab40fa
Bansal, S., Peloquin, J. M., Keah, N. M., O'Reilly, O. C., Elliott, D. M., Mauck, R. L., et al. (2020). Structure, function, and defect tolerance with maturation of the radial tie fiber network in the knee meniscus. J. Orthop. Res. 38 (12), 2709–2720. doi:10.1002/jor.24697
Beaufils, P., Becker, R., Kopf, S., Englund, M., Verdonk, R., Ollivier, M., et al. (2017). Surgical management of degenerative meniscus lesions: The 2016 ESSKA meniscus consensus. Knee Surg. Sports Traumatol. Arthrosc. 25 (2), 335–346. doi:10.1007/s00167-016-4407-4
Beaupré, A., Choukroun, R., Guidouin, R., Garneau, R., Gérardin, H., and Cardou, A. (1986). Correlation between microstructure and biomechanics. Clin. Orthop. Relat. Res. 208, 72–75. doi:10.1097/00003086-198607000-00016
Berni, M., Marchiori, G., Cassiolas, G., Grassi, A., Zaffagnini, S., Fini, M., et al. (2021). Anisotropy and inhomogeneity of permeability and fibrous network response in the pars intermedia of the human lateral meniscus. Acta Biomater. 135, 393–402. doi:10.1016/j.actbio.2021.08.020
Bhattacharjee, P., Kundu, B., Naskar, D., Kim, H.-W., Maiti, T. K., Bhattacharya, D., et al. (2017). Silk scaffolds in bone tissue engineering: An overview. Acta Biomater. 63, 1–17. doi:10.1016/j.actbio.2017.09.027
Cengiz, I. F., Pereira, H., Espregueira-Mendes, J., Kwon, I. K., Reis, R. L., and Oliveira, J. M. (2019). Suturable regenerated silk fibroin scaffold reinforced with 3D-printed polycaprolactone mesh: Biomechanical performance and subcutaneous implantation. J. Mat. Sci. Mat. Med. 30 (6), 63. doi:10.1007/s10856-019-6265-3
Chen, R., Zhu, C., Hu, M., Zhou, L., Yang, H., Zheng, H., et al. (2019). Comparative analysis of proteins from Bombyx mori and Antheraea pernyi cocoons for the purpose of silk identification. J. Proteomics 209, 103510. doi:10.1016/j.jprot.2019.103510
Chen, Y. C., Chen, R. N., Jhan, H. J., Liu, D. Z., Ho, H. O., Mao, Y., et al. (2015). Development and characterization of acellular extracellular matrix scaffolds from porcine menisci for use in cartilage tissue engineering. Tissue Eng. Part C. Methods 21 (9), 971–986. doi:10.1089/ten.TEC.2015.0036
Chen, Y., Chen, J., Zhang, Z., Lou, K., Zhang, Q., Wang, S., et al. (2017). Current advances in the development of natural meniscus scaffolds: Innovative approaches to decellularization and recellularization. Cell. Tissue Res. 370 (1), 41–52. doi:10.1007/s00441-017-2605-0
Cheung, H. S. (1987). Distribution of type I, II, III and V in the pepsin solubilized collagens in bovine menisci. Connect. Tissue Res. 16 (4), 343–356. doi:10.3109/03008208709005619
Culvenor, A. G., Oiestad, B. E., Hart, H. F., Stefanik, J. J., Guermazi, A., and Crossley, K. M. (2019). Prevalence of knee osteoarthritis features on magnetic resonance imaging in asymptomatic uninjured adults: A systematic review and meta-analysis. Br. J. Sports Med. 53 (20), 1268–1278. doi:10.1136/bjsports-2018-099257
D'Amora, U., Ronca, A., Raucci, M. G., Lin, H., Soriente, A., Fan, Y., et al. (2018). Bioactive composites based on double network approach with tailored mechanical, physico-chemical, and biological features. J. Biomed. Mat. Res. A 106 (12), 3079–3089. doi:10.1002/jbm.a.36498
Day, B., Mackenzie, W. G., Shim, S. S., and Leung, G. (1985). The vascular and nerve supply of the human meniscus. Arthrosc. J. Arthrosc. Relat. Surg. 1 (1), 58–62. doi:10.1016/s0749-8063(85)80080-3
Dorishetty, P., Balu, R., Athukoralalage, S. S., Greaves, T. L., Mata, J., de Campo, L., et al. (2020). Tunable biomimetic hydrogels from silk fibroin and nanocellulose. ACS Sustain. Chem. Eng. 8 (6), 2375–2389. doi:10.1021/acssuschemeng.9b05317
Fernández-García, L., Marí-Buyé, N., Barios, J. A., Madurga, R., Elices, M., Pérez-Rigueiro, J., et al. (2016). Safety and tolerability of silk fibroin hydrogels implanted into the mouse brain. Acta Biomater. 45, 262–275. doi:10.1016/j.actbio.2016.09.003
Fox, A. J., Bedi, A., and Rodeo, S. A. (2012). The basic science of human knee menisci: Structure, composition, and function. Sports Health. 4 (4), 340–351. doi:10.1177/1941738111429419
Fox, A. J., Wanivenhaus, F., Burge, A. J., Warren, R. F., and Rodeo, S. A. (2015). The human meniscus: A review of anatomy, function, injury, and advances in treatment. Clin. Anat. 28 (2), 269–287. doi:10.1002/ca.22456
Genemaras, A. A., Ennis, H., Bradshaw, B., Kaplan, L., and Huang, C. C. (2018). Effects of anti-inflammatory agents on expression of early responsive inflammatory and catabolic genes in ex vivo porcine model of acute knee cartilage injury. Cartilage 9 (3), 293–303. doi:10.1177/1947603516684589
Ghodbane, S. A., Brzezinski, A., Patel, J. M., Plaff, W. H., Marzano, K. N., Gatt, C. J., et al. (2019a). Partial meniscus replacement with a collagen-hyaluronan infused three-dimensional printed polymeric scaffold. Tissue Eng. Part A 25 (5-6), 379–389. doi:10.1089/ten.TEA.2018.0160
Ghodbane, S. A., Patel, J. M., Brzezinski, A., Lu, T. M., Gatt, C. J., and Dunn, M. G. (2019b). Biomechanical characterization of a novel collagen-hyaluronan infused 3D-printed polymeric device for partial meniscus replacement. J. Biomed. Mat. Res. 107 (8), 2457–2465. doi:10.1002/jbm.b.34336
Grassi, A., Zaffagnini, S., Marcheggiani Muccioli, G. M., Benzi, A., and Marcacci, M. (2014). Clinical outcomes and complications of a collagen meniscus implant: A systematic review. Int. Orthop. 38 (9), 1945–1953. doi:10.1007/s00264-014-2408-9
Gray, J. C. (1999). Neural and vascular anatomy of the menisci of the human knee. J. Orthop. Sports Phys. Ther. 29 (1), 23–30. doi:10.2519/jospt.1999.29.1.23
Gruchenberg, K., Ignatius, A., Friemert, B., von Lübken, F., Skaer, N., Gellynck, K., et al. (2014). In vivo performance of a novel silk fibroin scaffold for partial meniscal replacement in a sheep model. Knee Surg. Sports Traumatol. Arthrosc. 23 (8), 2218–2229. doi:10.1007/s00167-014-3009-2
Guo, W., Chen, M., Wang, Z., Tian, Y., Zheng, J., Gao, S., et al. (2021). 3D-printed cell-free PCL-MECM scaffold with biomimetic micro-structure and micro-environment to enhance in situ meniscus regeneration. Bioact. Mat. 6 (10), 3620–3633. doi:10.1016/j.bioactmat.2021.02.019
Gwinner, C., von Roth, P., Schmidt, S., Ode, J. E., Wulsten, D., and Hoburg, A. (2017). Biomechanical performance of a collagen meniscus implant with regard to suture material and irrigation fluid. Knee 24 (4), 726–732. doi:10.1016/j.knee.2017.04.003
Halili, A. N., Hasirci, N., and Hasirci, V. (2014). A multilayer tissue engineered meniscus substitute. J. Mat. Sci. Mat. Med. 25 (4), 1195–1209. doi:10.1007/s10856-014-5145-0
Hansen, R., Bryk, E., and Vigorita, V. (2013). Collagen scaffold meniscus implant integration in a canine model: A histological analysis. J. Orthop. Res. 31 (12), 1914–1919. doi:10.1002/jor.22456
Hao, L., Tianyuan, Z., Zhen, Y., Fuyang, C., Jiang, W., Zineng, Y., et al. (2021). Biofabrication of cell-free dual drug-releasing biomimetic scaffolds for meniscal regeneration. Biofabrication 14 (1), 015001. doi:10.1088/1758-5090/ac2cd7
Heo, J., Koh, R. H., Shim, W., Kim, H. D., Yim, H. G., and Hwang, N. S. (2016). Riboflavin-induced photo-crosslinking of collagen hydrogel and its application in meniscus tissue engineering. Drug Deliv. Transl. Res. 6 (2), 148–158. doi:10.1007/s13346-015-0224-4
Houck, D. A., Kraeutler, M. J., Belk, J. W., McCarty, E. C., and Bravman, J. T. (2018). Similar clinical outcomes following collagen or polyurethane meniscal scaffold implantation: A systematic review. Knee Surg. Sports Traumatol. Arthrosc. 26 (8), 2259–2269. doi:10.1007/s00167-018-4838-1
Howard, D., Shepherd, J. H., Kew, S. J., Hernandez, P., Ghose, S., Wardale, J. A., et al. (2014). Release of growth factors from a reinforced collagen GAG matrix supplemented with platelet rich plasma: Influence on cultured human meniscal cells. J. Orthop. Res. 32 (2), 273–278. doi:10.1002/jor.22495
Hsu, S. H., Hung, K. C., and Chen, C. W. (2016). Biodegradable polymer scaffolds. J. Mat. Chem. B 4 (47), 7493–7505. doi:10.1039/c6tb02176j
Huang, W., Ling, S., Li, C., Omenetto, F. G., and Kaplan, D. L. (2018). Silkworm silk-based materials and devices generated using bio-nanotechnology. Chem. Soc. Rev. 47 (17), 6486–6504. doi:10.1039/c8cs00187a
Ju, H. W., Lee, O. J., Lee, J. M., Moon, B. M., Park, H. J., Park, Y. R., et al. (2016). Wound healing effect of electrospun silk fibroin nanomatrix in burn-model. Int. J. Biol. Macromol. 85, 29–39. doi:10.1016/j.ijbiomac.2015.12.055
Kenne, L., Gohil, S., Nilsson, E. M., Karlsson, A., Ericsson, D., Helander Kenne, A., et al. (2013). Modification and cross-linking parameters in hyaluronic acid hydrogels--definitions and analytical methods. Carbohydr. Polym. 91 (1), 410–418. doi:10.1016/j.carbpol.2012.08.066
Kim, S. H., An, Y. H., Kim, H. D., Kim, K., Lee, S. H., Yim, H. G., et al. (2018). Enzyme-mediated tissue adhesive hydrogels for meniscus repair. Int. J. Biol. Macromol. 110, 479–487. doi:10.1016/j.ijbiomac.2017.12.053
Knopf-Marques, H., Pravda, M., Wolfova, L., Velebny, V., Schaaf, P., Vrana, N. E., et al. (2016). Hyaluronic acid and its derivatives in coating and delivery systems: Applications in tissue engineering, regenerative medicine and immunomodulation. Adv. Healthc. Mat. 5 (22), 2841–2855. doi:10.1002/adhm.201600316
Koh, R. H., Jin, Y., Kang, B.-J., and Hwang, N. S. (2017). Chondrogenically primed tonsil-derived mesenchymal stem cells encapsulated in riboflavin-induced photocrosslinking collagen-hyaluronic acid hydrogel for meniscus tissue repairs. Acta Biomater. 53, 318–328. doi:10.1016/j.actbio.2017.01.081
Kopf, S., Beaufils, P., Hirschmann, M. T., Rotigliano, N., Ollivier, M., Pereira, H., et al. (2020). Management of traumatic meniscus tears: The 2019 ESSKA meniscus consensus. Knee Surg. Sports Traumatol. Arthrosc. 28 (4), 1177–1194. doi:10.1007/s00167-020-05847-3
Kummer, B. (1987). Anatomy and biomechanics of the meniscus of the knee joint. Langenbecks Arch. Chir. 372, 241–246. Biomechanik des Kniegelenksmeniscus.). doi:10.1007/bf01297822
Kwon, H., Brown, W. E., Lee, C. A., Wang, D., Paschos, N., Hu, J. C., et al. (2019). Surgical and tissue engineering strategies for articular cartilage and meniscus repair. Nat. Rev. Rheumatol. 15 (9), 550–570. doi:10.1038/s41584-019-0255-1
Levillain, A., Magoariec, H., Boulocher, C., Decambron, A., Viateau, V., and Hoc, T. (2017). Effects of a viscosupplementation therapy on rabbit menisci in an anterior cruciate ligament transection model of osteoarthritis. J. Biomech. 58, 147–154. doi:10.1016/j.jbiomech.2017.04.034
Li, Y., Chen, M., Zhou, W., Gao, S., Luo, X., Peng, L., et al. (2020). Cell-free 3D wet-electrospun PCL/silk fibroin/Sr(2+) scaffold promotes successful total meniscus regeneration in a rabbit model. Acta Biomater. 113, 196–209. doi:10.1016/j.actbio.2020.06.017
Li, Z., Wu, N., Cheng, J., Sun, M., Yang, P., Zhao, F., et al. (2020). Biomechanically, structurally and functionally meticulously tailored polycaprolactone/silk fibroin scaffold for meniscus regeneration. Theranostics 10 (11), 5090–5106. doi:10.7150/thno.44270
Lin, D. D., Picardo, N. E., Adesida, A., and Khan, W. S. (2017). Clinical studies using biological and synthetic materials for meniscus replacement. Curr. Stem Cell. Res. Ther. 12 (4), 348–353. doi:10.2174/1574888x11666160429123110
López Barreiro, D., Yeo, J., Tarakanova, A., Martin-Martinez, F. J., and Buehler, M. J. (2019). Multiscale modeling of silk and silk-based biomaterials-A review. Macromol. Biosci. 19 (3), e1800253. doi:10.1002/mabi.201800253
Mandal, B. B., Park, S. H., Gil, E. S., and Kaplan, D. L. (2011a). Multilayered silk scaffolds for meniscus tissue engineering. Biomaterials 32 (2), 639–651. doi:10.1016/j.biomaterials.2010.08.115
Mandal, B. B., Park, S. H., Gil, E. S., and Kaplan, D. L. (2011b). Stem cell-based meniscus tissue engineering. Tissue Eng. Part A 17 (21-22), 2749–2761. doi:10.1089/ten.TEA.2011.0031
Markes, A. R., Hodax, J. D., and Ma, C. B. (2020). Meniscus form and function. Clin. Sports Med. 39 (1), 1–12. doi:10.1016/j.csm.2019.08.007
Masouros, S. D., McDermott, I. D., Amis, A. A., and Bull, A. M. (2008). Biomechanics of the meniscus-meniscal ligament construct of the knee. Knee Surg. Sports Traumatol. Arthrosc. 16 (12), 1121–1132. doi:10.1007/s00167-008-0616-9
Meyer, M. (2019). Processing of collagen based biomaterials and the resulting materials properties. Biomed. Eng. OnLine 18 (1), 24. doi:10.1186/s12938-019-0647-0
Monibi, F. A., Bozynski, C. C., Kuroki, K., Stoker, A. M., Pfeiffer, F. M., Sherman, S. L., et al. (2016). Development of a micronized meniscus extracellular matrix scaffold for potential augmentation of meniscal repair and regeneration. Tissue Eng. Part C. Methods 22 (12), 1059–1070. doi:10.1089/ten.TEC.2016.0276
Monibi, F. A., and Cook, J. L. (2017). Tissue-derived extracellular matrix bioscaffolds: Emerging applications in cartilage and meniscus repair. Tissue Eng. Part B Rev. 23 (4), 386–398. doi:10.1089/ten.TEB.2016.0431
Monllau, J. C., Gelber, P. E., Abat, F., Pelfort, X., Abad, R., Hinarejos, P., et al. (2011). Outcome after partial medial meniscus substitution with the collagen meniscal implant at a minimum of 10 years' follow-up. Arthrosc. J. Arthrosc. Relat. Surg. 27 (7), 933–943. doi:10.1016/j.arthro.2011.02.018
Moradi, L., Vasei, M., Dehghan, M. M., Majidi, M., Farzad Mohajeri, S., and Bonakdar, S. (2017). Regeneration of meniscus tissue using adipose mesenchymal stem cells-chondrocytes co-culture on a hybrid scaffold: In vivo study. Biomaterials 126, 18–30. doi:10.1016/j.biomaterials.2017.02.022
Pan, Z., Wu, Y., Zhang, X., Fu, Q., Li, J., Yang, Y., et al. (2017). Delivery of epidermal growth factor receptor inhibitor via a customized collagen scaffold promotes meniscal defect regeneration in a rabbit model. Acta Biomater. 62, 210–221. doi:10.1016/j.actbio.2017.07.008
Parkes, M., Myant, C., Dini, D., and Cann, P. (2015). Tribology-optimised silk protein hydrogels for articular cartilage repair. Tribol. Int. 89, 9–18. doi:10.1016/j.triboint.2014.11.024
Prendergast, M. E., Davidson, M. D., and Burdick, J. A. (2021). A biofabrication method to align cells within bioprinted photocrosslinkable and cell-degradable hydrogel constructs via embedded fibers. Biofabrication 13 (4), 044108. doi:10.1088/1758-5090/ac25cc
Renström, P., and Johnson, R. J. (1990). Anatomy and biomechanics of the menisci. Clin. Sports Med. 9 (3), 523–538. doi:10.1016/s0278-5919(20)30704-3
Rothrauff, B. B., Shimomura, K., Gottardi, R., Alexander, P. G., and Tuan, R. S. (2017). Anatomical region-dependent enhancement of 3-dimensional chondrogenic differentiation of human mesenchymal stem cells by soluble meniscus extracellular matrix. Acta Biomater. 49, 140–151. doi:10.1016/j.actbio.2016.11.046
Ruprecht, J. C., Waanders, T. D., Rowland, C. R., Nishimuta, J. F., Glass, K. A., Stencel, J., et al. (2019). Meniscus-derived matrix scaffolds promote the integrative repair of meniscal defects. Sci. Rep. 9 (1), 8719. doi:10.1038/s41598-019-44855-3
Sandri, G., Rossi, S., Bonferoni, M. C., Miele, D., Faccendini, A., Del Favero, E., et al. (2019). Chitosan/glycosaminoglycan scaffolds for skin reparation. Carbohydr. Polym. 220, 219–227. doi:10.1016/j.carbpol.2019.05.069
Sarem, M., Moztarzadeh, F., Mozafari, M., and Shastri, V. P. (2013). Optimization strategies on the structural modeling of gelatin/chitosan scaffolds to mimic human meniscus tissue. Mater. Sci. Eng. C 33 (8), 4777–4785. doi:10.1016/j.msec.2013.07.036
Schenk, L., Bethge, L., Hirschmann, A., Berbig, R., Luthi, U., Arnold, M. P., et al. (2020). Ongoing MRI remodeling 3-7 years after collagen meniscus implantation in stable knees. Knee Surg. Sports Traumatol. Arthrosc. 28 (4), 1099–1104. doi:10.1007/s00167-019-05714-w
Shen, J., Zhao, Q., Qi, Y., Cofer, G., Johnson, G. A., and Wang, N. (2022). Tractography of porcine meniscus microstructure using high-resolution diffusion magnetic resonance imaging. Front. Endocrinol. 13, 876784. doi:10.3389/fendo.2022.876784
Shimomura, K., Rothrauff, B. B., and Tuan, R. S. (2017). Region-specific effect of the decellularized meniscus extracellular matrix on mesenchymal stem cell-based meniscus tissue engineering. Am. J. Sports Med. 45 (3), 604–611. doi:10.1177/0363546516674184
Sizeland, K. H., Wells, H. C., Kirby, N. M., Hawley, A., Mudie, S. T., Ryan, T. M., et al. (2020). Bovine meniscus middle zone tissue: Measurement of collagen fibril behavior during compression. Int. J. Nanomedicine 15, 5289–5298. doi:10.2147/IJN.S261298
Song, K. H., Heo, S. J., Peredo, A. P., Davidson, M. D., Mauck, R. L., and Burdick, J. A. (2020). Influence of fiber stiffness on meniscal cell migration into dense fibrous networks. Adv. Healthc. Mat. 9 (8), e1901228. doi:10.1002/adhm.201901228
Sorushanova, A., Delgado, L. M., Wu, Z., Shologu, N., Kshirsagar, A., Raghunath, R., et al. (2019). The collagen suprafamily: From biosynthesis to advanced biomaterial development. Adv. Mat. 31 (1), e1801651. doi:10.1002/adma.201801651
Stabile, K. J., Odom, D., Smith, T. L., Northam, C., Whitlock, P. W., Smith, B. P., et al. (2010). An acellular, allograft-derived meniscus scaffold in an ovine model. Arthrosc. J. Arthrosc. Relat. Surg. 26 (7), 936–948. doi:10.1016/j.arthro.2009.11.024
Stein, S. E. C., von Luebken, F., Warnecke, D., Gentilini, C., Skaer, N., Walker, R., et al. (2019b). The challenge of implant integration in partial meniscal replacement: An experimental study on a silk fibroin scaffold in sheep. Knee Surg. Sports Traumatol. Arthrosc. 27 (2), 369–380. doi:10.1007/s00167-018-5160-7
Stein, S., Höse, S., Warnecke, D., Gentilini, C., Skaer, N., Walker, R., et al. (2019a). Meniscal replacement with a silk fibroin scaffold reduces contact stresses in the human knee. J. Orthop. Res. 37 (12), 2583–2592. doi:10.1002/jor.24437
Stone, K. R., Steadman, J. R., Rodkey, W. G., and Li, S. T. (1997). Regeneration of meniscal cartilage with use of a collagen scaffold. Analysis of preliminary data. J. Bone Jt. Surg. 79 (12), 1770–1777. doi:10.2106/00004623-199712000-00002
Stoppel, W. L., Ghezzi, C. E., McNamara, S. L., Black, L. D., and Kaplan, D. L. (2015). Clinical applications of naturally derived biopolymer-based scaffolds for regenerative medicine. Ann. Biomed. Eng. 43 (3), 657–680. doi:10.1007/s10439-014-1206-2
Sultankulov, B., Berillo, D., Sultankulova, K., Tokay, T., and Saparov, A. (2019). Progress in the development of chitosan-based biomaterials for tissue engineering and regenerative medicine. Biomolecules 9 (9), 470. doi:10.3390/biom9090470
Swinehart, I. T., and Badylak, S. F. (2016). Extracellular matrix bioscaffolds in tissue remodeling and morphogenesis. Dev. Dyn. 245 (3), 351–360. doi:10.1002/dvdy.24379
Tan, G. K., Dinnes, D. L., Butler, L. N., and Cooper-White, J. J. (2010). Interactions between meniscal cells and a self assembled biomimetic surface composed of hyaluronic acid, chitosan and meniscal extracellular matrix molecules. Biomaterials 31 (23), 6104–6118. doi:10.1016/j.biomaterials.2010.04.018
Tanaka, T., Furumatsu, T., Miyazawa, S., Fujii, M., Inoue, H., Kodama, Y., et al. (2017). Hyaluronan stimulates chondrogenic gene expression in human meniscus cells. Connect. Tissue Res. 58 (6), 520–530. doi:10.1080/03008207.2016.1264944
Thurber, A. E., Omenetto, F. G., and Kaplan, D. L. (2015). In vivo bioresponses to silk proteins. Biomaterials 71, 145–157. doi:10.1016/j.biomaterials.2015.08.039
Uebersax, L., Apfel, T., Nuss, K. M., Vogt, R., Kim, H. Y., Meinel, L., et al. (2013). Biocompatibility and osteoconduction of macroporous silk fibroin implants in cortical defects in sheep. Eur. J. Pharm. Biopharm. 85 (1), 107–118. doi:10.1016/j.ejpb.2013.05.008
van de Graaf, V. A., Noorduyn, J. C. A., Willigenburg, N. W., Butter, I. K., de Gast, A., Mol, B. W., et al. (2018). Effect of early surgery vs physical therapy on knee function among patients with nonobstructive meniscal tears: The ESCAPE randomized clinical trial. JAMA 320 (13), 1328–1337. doi:10.1001/jama.2018.13308
Varma, S., Orgel, J. P., and Schieber, J. D. (2016). Nanomechanics of type I collagen. Biophys. J. 111 (1), 50–56. doi:10.1016/j.bpj.2016.05.038
Venjakob, A. J., Föhr, P., Henke, F., Tischer, T., Sandmann, G. H., Blanke, F., et al. (2019). Influence of sutures on cartilage integrity: Do meniscus sutures harm cartilage? An experimental animal study. Arthrosc. J. Arthrosc. Relat. Surg. 35 (5), 1509–1516. doi:10.1016/j.arthro.2018.11.040
Veronesi, F., Di Matteo, B., Vitale, N. D., Filardo, G., Visani, A., Kon, E., et al. (2021). Biosynthetic scaffolds for partial meniscal loss: A systematic review from animal models to clinical practice. Bioact. Mat. 6 (11), 3782–3800. doi:10.1016/j.bioactmat.2021.03.033
Wang, S. J., Jiang, D., Zhang, Z. Z., Huang, A. B., Qi, Y. S., Wang, H. J., et al. (2016). Chondrogenic potential of peripheral blood derived mesenchymal stem cells seeded on demineralized cancellous bone scaffolds. Sci. Rep. 6, 36400. doi:10.1038/srep36400
Wang, Y., Wang, F., Xu, S., Wang, R., Chen, W., Hou, K., et al. (2019). Genetically engineered bi-functional silk material with improved cell proliferation and anti-inflammatory activity for medical application. Acta Biomater. 86, 148–157. doi:10.1016/j.actbio.2018.12.036
Warnecke, D., Schild, N. B., Klose, S., Joos, H., Brenner, R. E., Kessler, O., et al. (2017). Friction properties of a new silk fibroin scaffold for meniscal replacement. Tribol. Int. 109, 586–592. doi:10.1016/j.triboint.2017.01.038
Warnecke, D., Stein, S., Haffner-Luntzer, M., de Roy, L., Skaer, N., Walker, R., et al. (2018). Biomechanical, structural and biological characterisation of a new silk fibroin scaffold for meniscal repair. J. Mech. Behav. Biomed. Mat. 86, 314–324. doi:10.1016/j.jmbbm.2018.06.041
Warth, R. J., and Rodkey, W. G. (2015). Resorbable collagen scaffolds for the treatment of meniscus defects: A systematic review. Arthrosc. J. Arthrosc. Relat. Surg. 31 (5), 927–941. doi:10.1016/j.arthro.2014.11.019
Watt, F. M., and Huck, W. T. (2013). Role of the extracellular matrix in regulating stem cell fate. Nat. Rev. Mol. Cell. Biol. 14 (8), 467–473. doi:10.1038/nrm3620
Wu, J., Ding, Q., Dutta, A., Wang, Y., Huang, Y. H., Weng, H., et al. (2015). An injectable extracellular matrix derived hydrogel for meniscus repair and regeneration. Acta Biomater. 16, 49–59. doi:10.1016/j.actbio.2015.01.027
Wubneh, A., Tsekoura, E. K., Ayranci, C., and Uludağ, H. (2018). Current state of fabrication technologies and materials for bone tissue engineering. Acta Biomater. 80, 1–30. doi:10.1016/j.actbio.2018.09.031
Yan, R., Chen, Y., Gu, Y., Tang, C., Huang, J., Hu, Y., et al. (2019). A collagen-coated sponge silk scaffold for functional meniscus regeneration. J. Tissue Eng. Regen. Med. 13 (2), 156–173. doi:10.1002/term.2777
Yang, Q., Teng, B. H., Wang, L. N., Li, K., Xu, C., Ma, X. L., et al. (2017). Silk fibroin/cartilage extracellular matrix scaffolds with sequential delivery of TGF-β3 for chondrogenic differentiation of adipose-derived stem cells. Int. J. Nanomedicine 12, 6721–6733. doi:10.2147/IJN.S141888
Ying, X. Z., Qian, J. J., Peng, L., Zheng, Q., Zhu, B., and Jin, Y. H. (2018). Model research on repairing meniscus injury in rabbits using bone marrow mesenchymal stem cells and silk fibroin meniscus porous scaffold. Eur. Rev. Med. Pharmacol. Sci. 22 (12), 3689–3693. doi:10.26355/eurrev_201806_15247
Yuan, X., Wei, Y., Villasante, A., Ng, J. J. D., Arkonac, D. E., Chao, P.-h. G., et al. (2017). Stem cell delivery in tissue-specific hydrogel enabled meniscal repair in an orthotopic rat model. Biomaterials 132, 59–71. doi:10.1016/j.biomaterials.2017.04.004
Yuan, Z., Liu, S., Hao, C., Guo, W., Gao, S., Wang, M., et al. (2016). AMECM/DCB scaffold prompts successful total meniscus reconstruction in a rabbit total meniscectomy model. Biomaterials 111, 13–26. doi:10.1016/j.biomaterials.2016.09.017
Yun, H. W., Song, B. R., Shin, D. I., Yin, X. Y., Truong, M. D., Noh, S., et al. (2021). Fabrication of decellularized meniscus extracellular matrix according to inner cartilaginous, middle transitional, and outer fibrous zones result in zone-specific protein expression useful for precise replication of meniscus zones. Mater. Sci. Eng. C 128, 112312. doi:10.1016/j.msec.2021.112312
Zhang, Q., Wei, X., Ji, Y., Yin, L., Dong, Z., Chen, F., et al. (2020). Adjustable and ultrafast light-cured hyaluronic acid hydrogel: Promoting biocompatibility and cell growth. J. Mat. Chem. B 8 (25), 5441–5450. doi:10.1039/c9tb02796c
Zhang, Y., Liu, X., Zeng, L., Zhang, J., Zuo, J., Zou, J., et al. (2019). Polymer fiber scaffolds for bone and cartilage tissue engineering. Adv. Funct. Mat. 29 (36), 1903279. doi:10.1002/adfm.201903279
Zhong, G., Yao, J., Huang, X., Luo, Y., Wang, M., Han, J., et al. (2020). Injectable ECM hydrogel for delivery of BMSCs enabled full-thickness meniscus repair in an orthotopic rat model. Bioact. Mat. 5 (4), 871–879. doi:10.1016/j.bioactmat.2020.06.008
Keywords: sport medicine, tissue engineering, meniscus, natural biopolymer, clinic transition
Citation: Peng Y, Lu M, Zhou Z, Wang C, Liu E, Zhang Y, Liu T and Zuo J (2022) Natural biopolymer scaffold for meniscus tissue engineering. Front. Bioeng. Biotechnol. 10:1003484. doi: 10.3389/fbioe.2022.1003484
Received: 26 July 2022; Accepted: 16 September 2022;
Published: 30 September 2022.
Edited by:
Jianxun Ding, Changchun Institute of Applied Chemistry (CAS), ChinaCopyright © 2022 Peng, Lu, Zhou, Wang, Liu, Zhang, Liu and Zuo. This is an open-access article distributed under the terms of the Creative Commons Attribution License (CC BY). The use, distribution or reproduction in other forums is permitted, provided the original author(s) and the copyright owner(s) are credited and that the original publication in this journal is cited, in accordance with accepted academic practice. No use, distribution or reproduction is permitted which does not comply with these terms.
*Correspondence: Yanbo Zhang, emhhbmd5YjIwMTJAamx1LmVkdS5jbg==; Tong Liu, bGl1dG9uZzE5ODZAamx1LmVkdS5jbg==; Jianlin Zuo, enVvamxAamx1LmVkdS5jbg==
†These authors have contributed equally to this work and share first authorship