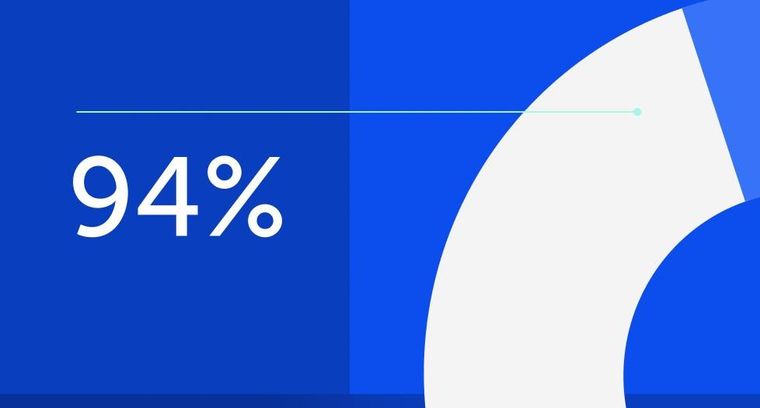
94% of researchers rate our articles as excellent or good
Learn more about the work of our research integrity team to safeguard the quality of each article we publish.
Find out more
REVIEW article
Front. Bioeng. Biotechnol., 10 November 2022
Sec. Biosafety and Biosecurity
Volume 10 - 2022 | https://doi.org/10.3389/fbioe.2022.1001572
This article is part of the Research TopicInsights in Biosafety and Biosecurity 2021: Novel Developments, Current Challenges, and Future PerspectivesView all 9 articles
With the rapid progress of nanotechnology, various nanoparticles (NPs) have been applicated in our daily life. In the field of nanotechnology, metal-based NPs are an important component of engineered NPs, including metal and metal oxide NPs, with a variety of biomedical applications. However, the unique physicochemical properties of metal-based NPs confer not only promising biological effects but also pose unexpected toxic threats to human body at the same time. For safer application of metal-based NPs in humans, we should have a comprehensive understanding of NP toxicity. In this review, we summarize our current knowledge about metal-based NPs, including the physicochemical properties affecting their toxicity, mechanisms of their toxicity, their toxicological assessment, the potential strategies to mitigate their toxicity and current status of regulatory movement on their toxicity. Hopefully, in the near future, through the convergence of related disciplines, the development of nanotoxicity research will be significantly promoted, thereby making the application of metal-based NPs in humans much safer.
Nanoparticles (NPs) are nanoscopic particles ranging from 1 to 100 nm, comprising materials such as carbon, metals, metal oxides, polymers and so on (Lee and Jun 2019; Ferreira Soares et al., 2020; Diez-Pascual, 2021; Qi et al., 2021). Compared to the corresponding bulk, nanoscale materials significantly change their physicochemical, mechanical, and biological properties (Chen et al., 2016; Saifi et al., 2018). The NPs impart many advantages like improved bioavailability and prolonged residence time depending on the small size and surface functionalities (Gwinn and Vallyathan, 2006; Mudshinge et al., 2011). These unique material characteristics make NPs a hotspot of materials science research and commercial/industrial interest (Ragelle et al., 2017; Kumari et al., 2020).
Nanotechnology is a revolutionary science involving fabricating and dealing with nanometer particles of various materials. Nanotechnology has endowed us with a new robust platform with a wide range of potential and practical applications including medicine, diagnostic devices, agriculture, catalysts, cosmetics, biological sensors, and so on (Missaoui et al., 2018; Bayda et al., 2019; He et al., 2019; Puglia and Santonocito, 2019; Zhou et al., 2021). As an important component of engineered NPs, metal-based NPs are generally made of metal precursors, including metal NPs and metal oxide NPs (Makhdoumi et al., 2020). Nowadays, metal-based NPs have received increasing attention due to their different properties such as large surface area, optically active, mechanically strong and chemically reactive (Khan et al., 2019). Owing to these properties, metal-based NPs have been widely used in biomedical application. It holds the promise to treat difficult and poor prognostic diseases such as cancers, complex infections, autoimmune diseases, and other clinical problems (Shen et al., 2018; Singh et al., 2018; Kalashnikova et al., 2020; Rashki et al., 2021). Compared to conventional therapeutics, nanoparticle-based therapy has more advantages in terms of cell specificity and selectivity, targeted delivery, high transport efficiency, and improved biopharmaceutical properties (Ahmad et al., 2018). NPs can significantly improve drug efficacy by altering the rates of drug metabolism and clearance (Seleci et al., 2017; Ravindran et al., 2018). Moreover, conjugated with targeting moieties such as specific antibodies and ligands, metal-based NPs can be actively targeted to the disease sites by recognizing and binding to the corresponding membrane proteins (Meka et al., 2019; Zhang et al., 2020). Thus, specific metal-based nanoparticle-based therapeutics have already been approved by the United States Food and Drug Administration (FDA) (Bobo et al., 2016).
While the increasing need for novel drugs or drug-release systems has led to vast advancements in nanomedicine, some safety concerns exist (Liu et al., 2020a; Najahi-Missaoui et al., 2020). While more and more metal-based NPs have been researched in various applications, information on the impact of NPs on human body is lagging behind (Najahi-Missaoui et al., 2020). It is suggested that NPs have a greater risk of toxicity than the corresponding bulk (Hoet et al., 2004). After metal-based NPs exposure, several side effects such as immunogenic reactions, nephrotoxicity, and neurotoxicity have been observed (Makhdoumi et al., 2020). It is also well known that high reactivity, longer circulation time, off-target nanomaterial accumulation, and unintentional exposure are the main risks of NP toxicity (Johnston et al., 2010; Yokel et al., 2012; Saifi et al., 2018). Although numerous toxicological studies have been conducted on metal-based NPs, the potential acute and chronic hazards to humans are not yet fully elucidated. It is necessary to thoroughly understand the basis of metal-based NP toxicity before extensive metal-based NPs can be safely applied to human beings.
This review briefly introduces the physicochemical properties of metal-based NPs that affect toxicity. Then the discussion of the mechanisms of metal-based NP toxicity will provide an overview of how metal-based NPs interact with the body. This review also covers well-established methodologies for nanoparticle toxicological assessment and evaluation in vitro and in vivo. Furthermore, we summarize some potential strategies to mitigate metal-based NP toxicity for safer biomedical applications in humans. The last section of the review presents current status of regulatory movement on nanotoxicity. We hope this review could improve our understanding of metal-based NP toxicity and guide the development of strategies to mitigate potential hazards to humans.
The physical and chemical properties of NPs act as key factors affecting NP uptake, translocation and accumulation in live tissues, which determines the fate of NPs and their mechanisms of toxicity (Zoroddu et al., 2014). In addition, some studies have confirmed that the physicochemical properties of NPs can dramatically affect nano-bio interactions and the potential toxicity (Kreyling et al., 2009; Attarilar et al., 2020; Manuja et al., 2021). Understanding these physicochemical properties has important implications for designing and fabricating safer metal-based NPs. The toxicity of metal-based NPs depends on their physicochemical properties, such as chemical composition, size, shape, and surface chemistry (Figure 1) (Wu and Tang, 2018).
FIGURE 1. Physicochemical Properties Affecting metal-based NP Toxicity. Different Sizes, shapes, chemical compositions, surface chemistry, solubility and agglomeration are closely related to the toxicity induced by metal-based NPs.
Particle size is a crucial physicochemical property contributing to cytotoxicity (Saifi et al., 2018; Donahue et al., 2019). NP size plays an important role in determining the approach of internalization in the cells, which finally influences the NP distributions in live tissues and mechanisms of nano-bio interactions (Sayes et al., 2007; Sukhanova et al., 2018). Similar to the nanosize of protein globules, DNA helix and cell membrane thickness, NPs can easily penetrate through the cell membrane and enter cells and cell organelles (Sukhanova et al., 2018). Furthermore, it is demonstrated that NPs with smaller size can pass through cell membranes by translocation, whereas NPs with larger size enter cells by other transportation mechanisms like phagocytosis, micropinocytosis, and non-specific translocation (Zhang et al., 2015). The smaller size of NPs enables higher permeability of cell membranes to interact with organelles such as mitochondria, lysosomes, and nucleus, finally causing cell damage (Donahue et al., 2019). In MCF-7 breast cancer cells, gold (Au) NPs smaller than 10 nm (2 and 6 nm) had the deeper internalization into the cell nucleus, while larger NPs (10 and 16 nm) were only accumulated in the cytoplasm (Huo et al., 2014). Some studies also revealed that the size of Titanium dioxide (TiO2) NPs correlated with their cytotoxicity (Kim et al., 2014; Wang et al., 2019). It was reported that smaller TiO2 NPs (6 nm) exposure under illumination caused more oxidative stress and more DNA damage than larger NPs (12 and 15 nm) in developing zebrafish embryos (Kim et al., 2014). More importantly, a kinetic study showed that organ distribution of NPs is highly size-dependent (De Jong et al., 2008). The 10 nm Au NPs had the most extensive organ distribution including blood, liver, spleen, kidney, testis, thymus, heart, lung, and brain, while the larger NPs were only found in blood, liver, and spleen. Silver (Ag) NPs with a size of 10 nm had higher tissue distribution and caused more severe hepatobiliary toxicity than larger NPs (40 and 100 nm) (Recordati et al., 2016). Moreover, the NP size could also have an impact on the mechanism of NP toxicity. After Au NPs exposure in four cell lines, the cellular response depends on the NP size, for the reason that 1.4 nm NPs cause cell death by necrosis whereas 1.2 nm NPs cause programmed cell death by apoptosis (Pan et al., 2007). Additionally, the smaller size of NPs leads to a larger surface area to volume ratio, which could increase the reactivity of NPs as it has more surface area to interact with cellular components (Johnston et al., 2010).
Along with NP size, NP shape can significantly affect NP toxicity (Sukhanova et al., 2018; Demir, 2021). NPs have various shapes including spheres, ellipsoids, cylinders, sheets, cubes, and rods (Sukhanova et al., 2018). For NPs of similar size and composition, their shape of NPs can alter their biological activities, including biodistribution, cellular uptake, deposition, and clearance (Zare et al., 2021). It is evident that the NP shape modulates the endocytosis kinetics of NPs in the efficient coarse-grained molecular dynamics (CGMD) model (Huang et al., 2013). By local energy analyses, the NP shape could affect the symmetry of curvature energy and hence determine the endocytic pathways and the angle of entry during the endocytosis process. Furthermore, there is evidence that non-spherical NPs are internalized by cells at faster rates and in larger amounts than spherical NPs (Hadji and Bouchemal, 2022). Numerous studies have shown that non-spherical NPs had longer circulation time in the blood and higher accumulation in specific organs than spherical ones (Geng et al., 2007; ArnidaJanat-Amsbury et al., 2011; Chu et al., 2013). For instance, rod-shaped PEGylated Au NPs had longer circulation time and higher accumulation in the tumors, compared to spherical NPs (ArnidaJanat-Amsbury et al., 2011). Similarly, the accumulation of discoidal porous silicon nanovectors into the tumor mass of breast cancer bearing mice was 5 times higher than spherical ones with similar size (Godin et al., 2012). Moreover, changes in shape may also significantly alter the surface area, which plays a role in determining their toxicity. Compared with sphere-shaped Iron (III) oxide (Fe2O3) NPs, rod-shaped Fe2O3 NPs were found to cause more severe toxic effects in mouse macrophage cells (RAW 264.7) with higher levels of lactate dehydrogenase (LDH) and tumor necrosis factor-α (TNF-α), reactive oxygen species (ROS) generation and necrosis (Lee et al., 2014). In addition, rod-shaped Cerium oxides (CeO2) NPs produced higher toxic responses with higher extracellular LDH release and pro-inflammatory cytokine TNF-α production in the RAW264.7 cell line, compared to cubic/octahedral-shaped NPs with similar chemical composition and crystallinity (Forest et al., 2017). On the contrary, the results of toxicity comparison of three kinds of CeO2 NPs with different shapes (cube-, octahedron-, and rod-like) in human hepatocellular carcinoma cells reported that cube-like NPs caused highest cytotoxicity and rod-like NPs caused lowest cytotoxicity (Wang et al., 2015). Furthermore, spherical TiO2 NPs exhibited 5-fold increased mortality in the Escherichia coli than elongated TiO2 (Simon-Deckers et al., 2009). It may be that different shapes of metal-based NPs lead to different bioaccumulation or/and reactivity, finally resulting in the differences in toxicity (Dai et al., 2015).
Besides size and shape, surface chemistry is another critical factor that remarkably affects toxicity (Chandran et al., 2017). The surface charge is closely related to NP toxicity, which can affect NP pharmacokinetics and their interactions with organelles and biomolecules. Zeta potential (ζ-potential) has already been adopted to characterize the surface charge of NPs and to predict NP toxicity. Numerous studies show that NPs with positive zeta potential are more prone to impart toxicity than those with negative zeta potential (Li et al., 2013; Shahbazi et al., 2013; Roshanzadeh et al., 2020; Sinclair et al., 2021), partially because that NPs have higher electrostatic interactions to negatively charged cellular membranes, thus increasing cellular uptake of NPs, leading to more cell damages (Albanese et al., 2012; Frohlich, 2012; Chusuei et al., 2013; Jeon et al., 2018; Roshanzadeh et al., 2020). For instance, comparison of the cytotoxicity of positively and negatively charged Au NPs revealed that the positively charged NPs were more toxic, due to their enhanced uptake (Huhn et al., 2013). Furthermore, magnetic NPs of Ferroferric Oxide (Fe3O4), oleic acid-coated Fe3O4, and carbon-coated Fe with different surface charges exhibited different cytotoxic effects on human hepatoma BEL-7402 cells and increased NP surface charges caused higher cytotoxicity by cell cycle arrest and inducing apoptosis (Kai et al., 2011). The phenomenon can be explained as the higher positive charge of NPs results in more significant electrostatic interactions with cells. The longer and greater the electrostatic interactions between NPs and cells, the more endocytic uptake of NPs into cells (Chusuei et al., 2013). In the systemic circulation, metal-based NPs could absorb a variety of proteins like albumin, immunoglobin, and other functional biomolecules to the surface of metal-based NPs to form a “protein corona”, which has been reported to alter the surface properties of metal-based NPs (Duran et al., 2015; Choi et al., 2017; Barbalinardo et al., 2018). On the one hand, NP-protein corona formation can change critical physicochemical characteristics of NP surface, thereby influencing pharmacological and toxicological characteristics of NPs (Ahsan et al., 2018; Ovais et al., 2020; Mishra et al., 2021). On the other hand, the configuration of those proteins absorbed on the NP surface would be altered, which leads to a change in their functional activity and biological processes (Tomak et al., 2021). Recent studies demonstrated that NP-protein corona formation could interact with many immune system components to either stimulate or suppress the immune response and cytotoxic effects (Hu et al., 2011a; Ruge et al., 2011; Lee et al., 2015; Lu et al., 2018). In addition, various coating materials like polyethylene glycol (PEG), poly (lactic-co-glycolic acid) (PLGA), polylactic acid (PLA), lipids, and others have been used to modify the metal-based NP surface, which considerably changes the physicochemical properties of NPs (Tefft et al., 2015; Oertel et al., 2016; Guerrero et al., 2019; Jiao et al., 2020; Murphy et al., 2021). Surface modifications of NPs not only affect their pharmacokinetics and change their biodistribution, clearance, and elimination, which are closely related to their toxicity in the body (Arami et al., 2015; Das et al., 2017; Yu et al., 2018a). For NPs with similar chemical compositions, different surface modifications lead to toxic effects in varying degrees (Zhang et al., 2021). Among three types of Aluminium Oxide (Al2O3) NPs including pristine Al2O3 NPs (p- Al2O3), hydrophilic (w- Al2O3), and lipophilic (o- Al2O3), it was found that o-Al2O3 NPs were more toxic than p-Al2O3 and w-Al2O3 NPs both in vitro and in vivo, as a result of cell membrane damage and over-production of ROS. Moreover, some studies illustrated that some specific modifications of NP surface could mitigate the toxicity of NPs and make them tissue-specific (Das et al., 2017; Lai et al., 2021). For example, after coated with a silica layer, the zinc dioxide (ZnO) NPs exhibited less cytotoxicity in human dermal fibroblast cells with less enzyme leakage, ROS production, and oxidative stress than their bare NPs, as a result of the surface modification restricting formation of free radicals and the release rate of zinc ions, as well as reducing the surface interactions of ZnO NPs with cells (Ramasamy et al., 2014).
The chemical composition and crystal structure of NPs are also critical to determine their toxicity. NPs fabricated by different materials differ in the mechanisms of toxicity. In one comparative study of the toxicity of carbon black, single-wall carbon nanotube, silicon dioxide (SiO2), and ZnO NPs, ZnO induced the most remarkable cytotoxicity by promoting intracellular oxidative stress, whereas SiO2 NPs mainly induced DNA damage (Yang et al., 2009). This result revealed that chemical composition is an important factor in the toxic effects of different NPs. Besides, toxicity screening performed on several types of metal oxide NPs observed that CuO and ZnO NPs induced the highest toxicity in acute models both in vitro and in vivo, mainly due to significant promotion of pro-inflammatory cytokines release and inhibition of macrophage viability (Areecheewakul et al., 2020). It is indicated that the chemical composition of metal-based NPs may influence the severity and mechanism of NP toxicity. Additionally, crystal structure can also affect metal-based NP toxicity (Vandebriel et al., 2018). Despite of same chemical composition, NPs with different crystal structures have different toxic responses. Compared to the anatase structure of TiO2 NPs, TiO2 NPs with rutile structure were more cytotoxic in cultured BEAS-2B cells by inducing hydrogen peroxide and oxidative DNA damage (Gurr et al., 2005). However, the NP crystal structure may be changed in different environments (Zhang et al., 2003). During the coalescence process, the crystal structure of Ag NPs was observed to transform from the common fcc structure to the unusual hcp structure (Grouchko et al., 2009).
Apart from those factors mentioned above, the solubility and agglomeration also affect the NP toxicity. Various intrinsic and extrinsic factors play a role in the solubility of the NPs. The intrinsic physicochemical properties like size, shape, surface chemistry, and crystal structure can affect NP dissolution process (Borm et al., 2006; Zhang et al., 2011; Misra et al., 2012a). Also, the extrinsic factors of surrounding media such as PH, ionic strength and water hardness can influence the NP behavior in solution (Peretyazhko et al., 2014; Fernando and Zhou, 2019). Increasing number of studies illustrate that the solubility of the NPs in surrounding media significantly influences their bioavailability, internalization process and toxicity mechanisms (Misra et al., 2012b). Furthermore, it has been suggested the metal ions release of metal-based NPs in media mainly contribute to the NP toxicity (Wang et al., 2016). For instance, a toxicological evaluation of ZnO NPs in various media showed that the NP toxicity in five types of media deceased as follows: ultrapure water >0.85% NaCl > minimal Davis > Luria-Bertani > phosphate-buffered saline (Li et al., 2011). The reason for the toxicity order is attributed to the decrease of the concentration of Zn2+ ions by the generation of precipitates and zinc complexes in different media. There is evidence that the degree of NP agglomeration also has an effect on the NP exposure, uptake, and distribution in the living tissues, thus influencing the toxicity of NPs (Creutzenberg et al., 2012; Bruinink et al., 2015). Agglomeration status refers as a collection of a group of NPs via weak forces, like van der Waals forces or electrostatic forces. Besides, various factors influencing agglomeration process in solution mainly include size, shape, surface structure, chemical composition, and so on (Ahamed et al., 2008; Bae et al., 2010; Andersson et al., 2011). Moreover, agglomeration status highly depends on the environmental parameters, like temperature, PH, and solution chemistry (Guzman et al., 2006; Karakoti et al., 2008; Bruinink et al., 2015). For example, in an in vitro testing, large agglomerates of 17 nm TiO2 caused stronger toxicity responses including glutathione depletion, IL-8 and IL-1β increase, and DNA damage in monocytic cell lines, compared to small agglomerates. However, it was observed that large agglomerates of 117 nm TiO2 caused higher pulmonary cytotoxicity in the mice inhalation study and more blood DNA damage in gavaged mice than small agglomerates (Murugadoss et al., 2020). Interestingly, large agglomerates do not exhibit less toxicity than small agglomerates.
The physicochemical properties determine the metal-based NP toxicity. Various in vitro and in vivo models have already been adopted to assess the toxicity of NPs, including cell lines, 3D cell culture, zebrafish, rodents, and others (Bahamonde et al., 2018; De Simone et al., 2018; Haque and Ward, 2018; Saifi et al., 2018; Xu et al., 2019; Cao et al., 2021). The exact mechanism of NP toxicity remains unclear. Multiple mechanisms contribute to the metal-based NP toxicity, including oxidative stress via ROS generation, mitochondrial dysfunction, DNA damage, and others (Figure 2) (Khanna et al., 2015; Nemmar et al., 2016; Sukhanova et al., 2018).
FIGURE 2. Mechanisms of cell damage by metal-based NPs. Following cellular uptake of NPs, metal-based NPs can release intracellular metal ions and induce oxidative stress directly or through mitochondrial dysfunction. ROS generation and consequent oxidative stress are shown to be the fundamental cause of metal-based NP toxicity. The excessive ROS generation and metal ions impact multiple cell signaling pathways, mainly including JAK-STAT, NF-κB, PI3K/Akt, MAPK, Nrf2. ROS can also cause DNA damage. Thus, it hinders cell physiological processes like proliferation and differentiation, eventually leading to cell apoptosis or necrosis.
The recent studies correlate the NP toxicity with the consequences of ROS generation (Wang et al., 2017; Angele-Martinez et al., 2022). Most work has shown that ROS generation and consequent oxidative stress are the fundamental cause of NP toxicity (Abdal Dayem et al., 2017; Makhdoumi et al., 2020; Mishra and Panda, 2021). Various metal-based NPs have already been discovered to induce toxicity by generating ROS in the living body (Yu et al., 2018b; Horie and Tabei, 2021). In cells of the normal state, there is a balance between the generation and removal of ROS. ROS mainly includes hydrogen peroxide, hydroxyl radical, singlet oxygen, hypochlorous acids, and superoxide anion, which are crucial triggers of oxidative stress (Poyton et al., 2009; Jakubczyk et al., 2020; Mishra and Panda, 2021). Under physiological conditions, ROS plays a vital role in regulating various aspects of cell behaviors, including cell proliferation, differentiation, and death (Forrester et al., 2018; Jakubczyk et al., 2020). However, the disruption of redox balance may result from increased ROS generation and/or decreased levels of antioxidants thus elevated ROS level results in oxidation of macromolecules, such as proteins, lipids, and nucleic acids, which subsequently leads to DNA damage, disturbed signal transduction, cytotoxicity, and cell death (Ray et al., 2012; Mishra and Panda, 2021).
NP-induced oxidative stress can mainly be divided into two types according to their underlying mechanisms (Horie and Tabei, 2021). One type is direct oxidative stress, also called primary oxidative stress. It is illustrated that the higher surface area of NPs is associated with more reactive sites on the surface, resulting in higher chemical reactivity to increase ROS generation (Wilson et al., 2002; Fu et al., 2014). The reactive surface with oxidants and free radicals can also significantly accelerate the formation of ROS. For instance, surface-bound free radicals on crystalline silica particles can enhance oxidative stress via a surface reaction to generate ROS (Fubini and Hubbard, 2003). Besides, studies have shown that some particular metal-based NPs, such as TiO2 NPs can generate ROS through a photocatalytic process under light irradiation (Toloman et al., 2019; Balaji et al., 2020; Romolini et al., 2021). Additionally, transition metals in NPs such as copper (Cu), and chromium (Cr) can participate in ROS generation via Fenton and Haber-Weiss reactions, ultimately enhancing oxidative stress (Huang et al., 2010; Angele-Martinez et al., 2017). Another type is indirect oxidative stress, also called secondary oxidative stress. In this type, NPs are not the direct cause of oxidative stress. Mitochondria are crucial organelles involved in NP-induced oxidative stress. Intracellular metal ions released from internalized metal-based NPs can depolarize the mitochondrial membrane and disturbance of electron-transport chain, eventually leading to mitochondrial dysfunction (Fu et al., 2014; Hosseini et al., 2014). In mitochondria, molecular oxygen is consumed to synthesize adenosine triphosphate via a series of coupled proton and electron transfer reactions in the physiological process. However, as a result of metal-based NP exposure, the mitochondrial electron-transport chain (METC) would be disrupted, thus resulting in the elevation of intracellular ROS level (Ashrafi Hafez et al., 2019). For cancer-specific cytotoxicity, superparamagnetic iron oxide NPs could specifically target the METC complexes, resulting in mitochondrial ROS production, which induced cancer cell death (He et al., 2016). NP-induced ROS generation could also be derived from the interaction of metal oxide NPs with ROS-associated enzymes and receptors. For instance, in the presence of TiO2 NPs, NADPH oxidase was hyper-activated, and the subsequent increase in ROS generation induced a higher level of oxidative stress (Masoud et al., 2015). In addition, ROS generation by phagocytes, like macrophages and neutrophils, is also the cause of NP-induced oxidative stress (Horie and Tabei, 2021). In the zebrafish model, exposure to Ag NPs would damage the mitochondria of innate immune cells including macrophages and neutrophils, leading to excessive ROS production, which finally induced innate immune toxicity (Chen et al., 2021). Notably, a recent study demonstrated that co-exposure to gold (Au) NPs and lipopolysaccharide would result in upregulating extracellular ROS generation by hepatic macrophage, theraby inducing hepatic apoptosis and aggravating liver injury in mice (Yang et al., 2022). After internalized by macrophages, Fe2O3 NPs can be enclosed and hence biodegraded in the lysosomes, and released atomic iron participates in ROS production via Fenton reaction. More importantly, ROS appears to involve in the Fe2O3 NP-induced macrophage activation through several signal pathways, consequently regulating the innate immune response (Mulens-Arias et al., 2021). Furthermore, the higher level of intracellular ROS may damage the mitochondrial membrane and the electron-transport chain, leading to the more ROS and amplifying the oxidative imbalance, also referred to as ROS-induced ROS release (Zorov et al., 2014; Mishra and Panda, 2021). However, not all NP-induced toxicity is mediated via ROS. A comparison study focused on cytotoxicity, DNA damage, and oxidative stress of different metal oxide NPs (CuO, TiO2, ZnO, CuZnFe2O4, Fe3O4, Fe2O3) in human lung epithelial cell line A549. Among these metal-based NPs, only CuO NPs caused oxidative stress by significantly increasing intracellular ROS (Karlsson et al., 2008).
It has been reported that the excessive ROS generation and metal ions released from internalized metal-based NPs have an impact on multiple cell signaling pathways, mainly including Janus kinase/signal transducers and activators of transcription (JAK-STAT), nuclear factor-kappa-lightchain enhancer of activated B cells (NF-κB), Phosphatidylinositol 3-kinase (PI3K)/protein serine threonidase (Akt), mitogen-activated protein kinase (MAPK), and nuclear factor erythroid 2-related factor 2 (Nrf2) (Ko et al., 2016; Chang et al., 2017; Kim et al., 2017; Mahmoud et al., 2019; Chang et al., 2021). Activation or inhibition of these signaling pathways can impact many significant physiological processes like cell proliferation, differentiation, survival, and immune regulation (Li and Tang, 2020). In HeLa cells, the Ag-NP-hydrogel exposure activated JAK-STAT signaling pathway by upregulating JAK-STAT cascade-related gene, thereby inducing toxicity (Xu et al., 2012). Silica NPs could induce oxidative stress, inflammation, and NO/NOS system disorder, ultimately causing endothelial cytotoxicity by activating the MAPK/Nrf2 and NF-κB signaling pathways (Guo et al., 2015). After intratracheal instillation in male Wistar rats, nickel oxide (Nio) NPs activated the NF-κB pathway by upregulation of mRNA and protein expression of NF-κB, an inhibitor of κB kinase-α and nuclear factor-inducing kinase, partially causing pulmonary damage (Chang et al., 2017). It was demonstrated that SiO2 NPs induced the lung alveolar epithelial cell apoptosis by ROS-regulated PI3K/AKT-mediated mitochondria- and ER stress-dependent signaling pathways, leading to lung injury (Lee et al., 2020). However, no single mechanism or signaling pathway has been yet found to elucidate the NP toxicity fully. Furthermore, the crosstalk between different signaling pathways complicates the exact mechanism of NP toxicity.
With the rapid development and wide application of nanotechnology and nanomaterials, the impact of metal-based NPs on human health has gradually attracted more and more attention, thus, calling for a systemic approach to evaluate the safety of metal-based NPs. Numerous data from in vitro and in vivo studies, epidemiological studies, and occupational health studies have shown the toxicity of NPs. Here, we summarized some standard tools used for nanotoxicity assessment (shown in Table 1). Owing to its low cost and simplicity, in vitro cellular experiments are routinely used to assess metel-based NP toxicity, allowing deeper investigation into molecular mechanisms. However, studies in vitro have apparent flaws, such as the inability to describe the distribution patterns of NPs in vivo. Thus its prediction power is limited. In contrast, in vivo animal studies have the advantage of estimating the toxic effects of metal-based NPs in complex physiological environments.
Since conventional toxicology studies are primarily based on one or two endpoints, this is insufficient to fully understand the mechanisms of metal-based NP toxicity. Recently, various high-throughput-based omics studies have been used to screen for NP toxicity, including genomics, transcriptomics, proteomics, metabolomics, etc., (Matysiak et al., 2016; Frohlich, 2017; Li et al., 2021). Omics technologies provide more comprehensive molecular profiling, and can be employed to screen NP toxicity at low exposure levels, and also have the advantage of identifying new biomarkers and targets of nanotoxicity both in vivo and in vitro. Omics approach also allows detection in miniscule variations within a cell compared to classical methods. However, the information obtained by a single omics approach is often far from complete, providing limited insight into complex molecular pathways and biological events in cells and organisms (Van Assche et al., 2015). Thus, the integration of omics has attracted more and more attention. And integrated omics was proven to help determine nanotoxicity. The combination of gene expression and metabolic profiling will provide more detailed and sensitive toxicological assessment of NPs (Shim et al., 2012). Metabolomics and transcriptomics combination facilitates more sensitive and detailed nano-toxicological assessments (Shin et al., 2018). Ruan et al. (2021) treated normal rat kidney cells with silica NPs (SiNPs) conducted a transcriptomic, proteomic and phosphoproteomic profiling, and established a new computational prediction algorithm of master autophagy-regulating kinases (cMAK) for integrating and analyzing multi-omics data. In this cMAK algorithm, the transcriptional, proteomic, and phosphoproteomic data of predicted kinases were wholly considered to reduce potentially false-positive hits, and predicted kinases with differentially regulated mRNAs at ≥2 time points, changed proteins at ≥1 time point, or altered phosphorylation sites at ≥1 time point were retained. Finally, 21 protein kinases candidates were predicted to be involved in autophage activation regulation induced by SiNPs. Since cMAK powerfully narrowed down the candidates, and then with the help of some additional experiments, two kinases, cyclin dependent kinase 4/7, were detected to be essential in SiNPs triggered autophage activation. Overall, omics analysis provides a promising future for systematically studying metabolic and physiological responses to NP exposure, with relatively low cost and high-dimensional data, and the integration of multi-omics can substantially improve the accuracy of the analysis.
Furthermore, there comes up a new way of predicting metal-based NP toxicity by computer simulation. This computational prediction relies on analyzing reliable experimental data of NPs structure, physicochemical properties and biological activity, as well as the measured toxicity (Richarz et al., 2017). Since computational chemistry can save time, funds and animal sacrifice compared to the traditional experiments, the quantitative structure–activity relationship (QSAR) approach may also be used for NP toxicity prediction. The idea of QSAR modeling of nanomaterials (Nano-QSAR) was introduced in 2009 by Puzyn et al. (2009). As Nano-QSAR model quantitatively describes the relationship in the form of mathematical equations, it is very dependent on the accuracy of the descriptors. The structure of metal-based NPs is highly complex and diversified, making it impossible to calculate theoretical descriptors (Li et al., 2022). Therefore, there comes up an urgent need to develop specific nano-descriptors to match the properties of metal-based NPs. And majority of the nano-QSAR models are based on the intrinsic physicochemical properties but ignoring the environmental influence (Puzyn et al., 2011; Kar et al., 2014), thus, it might be difficult to predict the toxicity directly from the structure. So, Anna et al. proposed to replace the traditional nano-QSAR model with an approach called Structure–Activity Prediction Networks (SAPNets), claiming it can effectively link NP structure with their toxicity through a series of layers built from nodes that correspond to predictive “meta-models” developed with machine learning techniques as well as Artificial Intelligence (Rybinska-Fryca et al., 2020). In contrast, the traditional nano-QSAR models mainly contains only one layer of descriptors, and it is not certain which descriptors are fundamental, and their application in predicting toxicity requires knowledge and experience in computational chemistry. However, the SAPNets are based on understandable descriptors (e.g., size, shape, aspect-ratio, and type of coating) and do not require additional computational calculations, allows consideration in environmental influence (such as solvent and pH), and the users can easily see how the change of the descriptor values will influence the predicted toxicity. Since computer simulations are highly dependent on previous experimental data, and the pathways and mechanisms of NPs toxic effects have not been fully elucidated, computer models may not be able to predict the toxic effects of NPs accurately. However, Gajewicz et al. (2017) proposed a quantitative read-across algorithm based on: one-point-slope, two-point formula, or the equation of a plane passing through three points, providing reliable predictions when only limited data is available. Labouta et al. (2019) developed a data mining method to assemble published evidence on NP cytotoxicity, with decision trees and feature selection algorithms, offering a powerful and relatively accurate tool for predicting cytotoxicity of NPs. Recently, machine learning techniques have also been applied in nanotoxicology. Furxhi et al. (2019) proposed a Copeland Index for machine learning to achieve more accurate predictions of NP toxicity for a given dataset. As more and more systematic data on NPs are available, data mining and machine learning are becoming powerful auxiliary tools for studying the toxic effects of NPs.
Due to their unique properties, metal-based NPs have shown great potential in biomedical applications. Notably, NP toxicity remains a major obstacle for future applications. By understanding the mechanisms of metal-based NP toxicity, researchers have developed several strategies to mitigate their potential adverse impacts and toxicity, especially to achieve the goal of clinical translation of metal-based NPs. As the surface characteristics are primarily responsible for the toxicity of the NPs, modification of surface chemistry and properties has been reported to be one of the most common devised strategies to mitigate NP toxicity (Figure 3) (Amin et al., 2015).
FIGURE 3. Strategies to mitigate metal-based NP toxicity. Surface coating, surface chemistry modifications by altering charge density and hydrophobicity, and introducing naturally derived cell membranes from several cell types can be used to reduce the potential toxicity of metal-based NPs.
Surface coating is one of the most popular surface modification strategies to reduce the potential toxicity of NPs, which is reversible by non-covalent modification (Hwang et al., 2018). Surface coating can alter the dispersion state of NPs, which significantly determines their bioavailability and potential toxicological effects (Wang et al., 2011). There are various types of coating materials such as polyethylene glycol (PEG), polyvinyl pyrrolidone (PVP), zwitterionic polymers, and poly (N -isopropyl acrylamide) (PNIPAM), and Polyvinyl alcohol (PVA) (Zhernenkov et al., 2015; Suk et al., 2016; Debayle et al., 2019; Lou et al., 2019; Ronavari et al., 2021). PEG is currently the most popular and practical material to passivate NP surfaces by shielding surface charges, imparting longer circulation time, and more cellular uptake of PEGylated NPs (Joralemon et al., 2010). Moreover, PEGylation of NPs can enhance their biocompatibility and reduce enzymatic degradation and non-immunogenicity (Karakoti et al., 2011). In vitro and in vivo experiments demonstrated no obvious toxicity of magnetic PEGylated Pt3Co NPs over a period of 60 days (Yin et al., 2013). Compared to conventional γ-Fe2O3 particles, no cytotoxicity and immunotoxicity were observed for PA-PEG@Fe3O4 and HA-PEG@Fe3O4 NPs in peripheral blood mononuclear cells (Patsula et al., 2019). A recent comparative study indicated that PEG-coated Au NPs induced less hepatotoxic outcomes than uncoated Au NPs in Sprague Dawley rats, which showed that PEG-coated Au NPs might be safer for biomedical applications (Patlolla et al., 2019).
Surface chemistry modifications by altering charge density and hydrophobicity have been reported to ameliorate NP toxicity and enhance the efficacy of NPs in biomedical applications (Mout et al., 2012; Nicol et al., 2015; Abd Ellah and Abouelmagd, 2017). The surface chemistry properties of NPs can be modified by covalently coupling with functional groups like anionic, nonionic, zwitterionic, and cationic groups onto the surface (Blanco et al., 2015; Zakharova et al., 2019; Han et al., 2020). These functional groups can influence the charge density and hydrophobicity of NPs (Nel et al., 2009; Aimonen et al., 2022). Au NPs can be an appropriate example where surface chemistry modification could influence NP toxicity. Au NPs functionalized with cationic groups were more toxic than NPs functionalized with anionic groups (Goodman et al., 2004). Furthermore, Fe2O3 NPs can generate ROS from the Fenton reaction on their surface, leading to cytotoxic effects (Voinov et al., 2011; Patil et al., 2015). The surface of Fe2O3 NPs can be functionalized with organic or inorganic materials to help stabilize iron oxidation, thereby reducing toxicity and improving biocompatibility (Wu et al., 2008; Zhu et al., 2018; Liu et al., 2020b). According to a recent study, ZnO NPs with hydrophoboic coatings had less toxic effects than those with hydrophilic coatings, possibly owing to the fact that hydrophobic surface coating could decrease bioavailability and thus reduce NP toxicity (Shukla et al., 2015; Lai et al., 2021).
There is a novel approach to introducing naturally derived cell membranes from several cell types such as red blood cells (RBCs), platelets, white blood cells (WBCs), and cancer cells onto the surface of NPs, enabling new properties like immune evasion, long-term circulation, specific recognition and targeting (Fang et al., 2018; Liu et al., 2021). Cell membranes are composed of lipids, proteins, and carbohydrates, which all play crucial roles in cellular signaling (Sarkar and Chattopadhyay, 2021). Further, cell membrane coatings can be employed to make NPs mimic the properties exhibited by the source cells, thereby directly replicating a variety of complex functions (Hu et al., 2015; Xia et al., 2019). The cell membrane coating technology was firstly reported using erythrocyte membranes for NP coating (Hu et al., 2011b). It was demonstrated that RBC membrane-coated NPs could effectively inhibit protein corona formation, which is known to promote biocompatibility, leading to their non-toxic or less toxic effects in vivo (Hu et al., 2011a; Rao et al., 2017). RBC membrane-coated Fe3O4 did not show significant in vivo toxicity in animal models by various assays, including blood biochemistry, whole blood panel examination, and histology analysis (Rao et al., 2016). This cell membrane coating strategy can effectively mitigate the potential toxicity of NPs for further safer biomedical applications.
Nanoscience has developed rapidly in the past 20 years, and many kinds of nanomaterials have been applied into clinical medicine. This raises to great concerns about the safety of nanomaterials, and puts forward higher requirements for the regulation of nanotoxicity. One of the main barriers to advancing nanotoxicology is the lack of harmonized and standardized NP characterization and risk assessment methods (Yang et al., 2021). The definition of NPs is still not universally acceptable. NPs are generally considered to be any particle with a size below 100 nm. But particles between 100–1,000 nm in size are also potentially toxic. Furthermore, the experimental design and data reporting are required to standardize to train predictive computational models. And the dose selected for NP toxicity assessment is also critical, especially in data mining and machine learning. In addition, the toxicity due to long-term low-dose NP exposure is often neglected, owing to the difficulties in simulating by traditional experimental models. The Nanotechnology Task Force (NTF) was launched in 2006 by FDA to help evaluate FDA’s regulatory authorities concerning the current state of nanotechnology. FDA had released a report about nanotechnology in 2020, showing the progress and innovation over a decade. And to date, FDA has issued six guidance documents regarding nanotechnology for industry, including one about drug products containing nanomaterials released in April 2022. FDA does not make definitive judgments about the nature of nanotechnology as safe or harmful. The regulatory approach of FDA is adaptive and flexible, considering the specific characteristics and effects of nanomaterials in the particular biological context of their intended use, as well as focusing on the interactions of nanomaterials with biological systems. To regulate and guide the research and evaluation of nanomedicine, the Center for Drug Evaluation (CDE) of the China National Medical Products Administration formulated the Technical Guidelines for Nanomedicines Non-Clinical Safety Evaluation Research (Trial), which was issued in August 2021 (Center for Drug Evaluation of Chinese National Medical Products Administration, 2021). This CDE guidance emphasized an appropriate selection of the test system, whether it is in vitro or in vivo or an alternative toxicity testing method, and also reminded to carefully choose the dose and testing time period. Furthermore, this guidance focused on the toxicity testing methods of nanomedicines on multiple systems including immunogenicity and immunotoxicity, neurotoxicity, genotoxicity, carcinogenicity, and reproductive toxicity. Although great efforts have been made by different regulatory agencies around the world like the United States FDA and China CDE to develop a proper guidance for the safer use of NPs, currently there are still no worldwide uniform and strict guidelines regarding NP toxicity testing (Saifi et al., 2018). Undoubtedly, the advances in the area of nanotoxicity study will ultimately facilitate to establish and implement a standardized and effective regulatory regime that harnesses the potential of nanotechnology and minimizes harm to humans.
Since metal-based NPs are widely applicated in our daily life due to their unique properties, great attention has been attracted to their toxicity. Studies have shown that the toxic effects of NPs are mainly determined by several factors, such as physicochemical properties, dose, exposure pathways, and duration. Based on our current knowledge, it is challenging to elucidate the exact mechanism of metal-based NP toxicity. Recent studies have focused on oxidative stress as the underlying cause of metal-based NP toxicity. Indeed, as current in vitro and in vivo toxicity testing methods are mainly used to evaluate the acute and subacute toxicity of metal-based NPs, nanotoxicity testing methods for chronic long-term NPs exposure, which are crucial for predicting chronic toxicity in humans, are still lacking. Thus, there is an urgent need to develop new powerful tools to evaluate and understand the mechanisms of metal-based NP toxicity. Many promising effective strategies have been developed to design safer metal-based NPs for further biomedical applications to minimize NP toxicity. Ultimately, we should have a comprehensive understanding of metal-based NP toxicity before a general consensus can be reached on the toxicity of NPs. Shortly, we believe that the convergence of related disciplines like material science, medicine, chemistry, and artificial intelligence will significantly advance the development of nanotoxicity research, thereby making the application of metal-based NPs safer in humans.
Conceptualization and writing—original draft preparation, NZ and GX; Visualization, GX; Writing—review and editing, ZL; All authors contributed to the article and approved the final manuscript.
This work was funded by the National Natural Science Foundation of China (81670433 and 81970398), the Zhejiang Medical and Health Science and Technology Project (2020RC014 and 2023KY110), the Natural Science Foundation of Zhejiang Province (Q20H020059 and LQ20H020008), and the Science Fund for Distinguished Young Scholars of Zhejiang Province (LR22H020002).
The authors declare that the research was conducted in the absence of any commercial or financial relationships that could be construed as a potential conflict of interest.
All claims expressed in this article are solely those of the authors and do not necessarily represent those of their affiliated organizations, or those of the publisher, the editors and the reviewers. Any product that may be evaluated in this article, or claim that may be made by its manufacturer, is not guaranteed or endorsed by the publisher.
Abd Ellah, N. H., and Abouelmagd, S. A. (2017). Surface functionalization of polymeric nanoparticles for tumor drug delivery: Approaches and challenges. Expert Opin. Drug Deliv. 14 (2), 201–214. doi:10.1080/17425247.2016.1213238
Abdal Dayem, A., Hossain, M., Lee, S., Kim, K., Saha, S., Yang, G. M., et al. (2017). The role of reactive oxygen species (ROS) in the biological activities of metallic nanoparticles. Int. J. Mol. Sci. 18 (1), 120. doi:10.3390/ijms18010120
Ahamed, M., Karns, M., Goodson, M., Rowe, J., Hussain, S. M., Schlager, J. J., et al. (2008). DNA damage response to different surface chemistry of silver nanoparticles in mammalian cells. Toxicol. Appl. Pharmacol. 233 (3), 404–410. doi:10.1016/j.taap.2008.09.015
Ahmad, K., Rabbani, G., Baig, M. H., Lim, J. H., Khan, M. E., Lee, E. J., et al. (2018). Nanoparticle-based drugs: A potential armamentarium of effective anti-cancer therapies. Curr. Drug Metab. 19 (10), 839–846. doi:10.2174/1389200218666170823115647
Ahsan, S. M., Rao, C. M., and Ahmad, M. F. (2018). Nanoparticle-protein interaction: The significance and role of protein corona. Adv. Exp. Med. Biol. 1048, 175–198. doi:10.1007/978-3-319-72041-8_11
Aimonen, K., Imani, M., Hartikainen, M., Suhonen, S., Vanhala, E., Moreno, C., et al. (2022). Surface functionalization and size modulate the formation of reactive oxygen species and genotoxic effects of cellulose nanofibrils. Part. Fibre Toxicol. 19 (1), 19. doi:10.1186/s12989-022-00460-3
Albanese, A., Tang, P. S., and Chan, W. C. (2012). The effect of nanoparticle size, shape, and surface chemistry on biological systems. Annu. Rev. Biomed. Eng. 14, 1–16. doi:10.1146/annurev-bioeng-071811-150124
Almeida, J. P., Chen, A. L., Foster, A., and Drezek, R. (2011). In vivo biodistribution of nanoparticles. Nanomedicine (Lond) 6 (5), 815–835. doi:10.2217/nnm.11.79
Amin, M. L., Joo, J. Y., Yi, D. K., and An, S. S. A. (2015). Surface modification and local orientations of surface molecules in nanotherapeutics. J. Control. Release 207, 131–142. doi:10.1016/j.jconrel.2015.04.017
Andersson, P. O., Lejon, C., Ekstrand-Hammarstrom, B., Akfur, C., Ahlinder, L., Bucht, A., et al. (2011). Polymorph- and size-dependent uptake and toxicity of TiO(2) nanoparticles in living lung epithelial cells. Small 7 (4), 514–523. doi:10.1002/smll.201001832
Angele-Martinez, C., Ameer, F. S., Raval, Y. S., Huang, G., Tzeng, T. R. J., Anker, J. N., et al. (2022). Polyphenol effects on CuO-nanoparticle-mediated DNA damage, reactive oxygen species generation, and fibroblast cell death. Toxicol. Vitro 78, 105252. doi:10.1016/j.tiv.2021.105252
Angele-Martinez, C., Nguyen, K. V. T., Ameer, F. S., Anker, J. N., and Brumaghim, J. L. (2017). Reactive oxygen species generation by copper(II) oxide nanoparticles determined by DNA damage assays and EPR spectroscopy. Nanotoxicology 11 (2), 278–288. doi:10.1080/17435390.2017.1293750
Anselmo, A. C., and Mitragotri, S. (2019). Nanoparticles in the clinic: An update. Bioeng. Transl. Med. 4 (3), e10143. doi:10.1002/btm2.10143
Arami, H., Khandhar, A., Liggitt, D., and Krishnan, K. M. (2015). In vivo delivery, pharmacokinetics, biodistribution and toxicity of iron oxide nanoparticles. Chem. Soc. Rev. 44 (23), 8576–8607. doi:10.1039/c5cs00541h
Areecheewakul, S., Adamcakova-Dodd, A., Givens, B. E., Steines, B. R., Wang, Y., Wang, Y., Meyerholz, D. K., et al. (2020). Toxicity assessment of metal oxide nanomaterials using in vitro screening and murine acute inhalation studies, 18. Amsterdam, Netherlands: NanoImpact.
Arnida, , Janat-Amsbury, M., Ray, A., Peterson, C., and Ghandehari, H. (2011). Geometry and surface characteristics of gold nanoparticles influence their biodistribution and uptake by macrophages. Eur. J. Pharm. Biopharm. 77 (3), 417–423. doi:10.1016/j.ejpb.2010.11.010
Ashrafi Hafez, A., Naserzadeh, P., Mortazavian, A. M., Mehravi, B., Ashtari, K., Seydi, E., et al. (2019). Comparison of the effects of MnO2-NPs and MnO2-MPs on mitochondrial complexes in different organs. Toxicol. Mech. Methods 29 (2), 86–94. doi:10.1080/15376516.2018.1512693
Attarilar, S., Yang, J., Ebrahimi, M., Wang, Q., Liu, J., Tang, Y., et al. (2020). The toxicity phenomenon and the related occurrence in metal and metal oxide nanoparticles: A brief review from the biomedical perspective. Front. Bioeng. Biotechnol. 8, 822. doi:10.3389/fbioe.2020.00822
Bae, E., Park, H. J., Lee, J., Kim, Y., Yoon, J., Park, K., et al. (2010). Bacterial cytotoxicity of the silver nanoparticle related to physicochemical metrics and agglomeration properties. Environ. Toxicol. Chem. 29 (10), 2154–2160. doi:10.1002/etc.278
Bahamonde, J., Brenseke, B., Chan, M. Y., Kent, R. D., Vikesland, P. J., and Prater, M. R. (2018). Gold nanoparticle toxicity in mice and rats: Species differences. Toxicol. Pathol. 46 (4), 431–443. doi:10.1177/0192623318770608
Balaji, S., Mandal, B. K., Vinod Kumar Reddy, L., and Sen, D. (2020). Biogenic ceria nanoparticles (CeO2 NPs) for effective photocatalytic and cytotoxic activity. Bioeng. (Basel) 7 (1), 26. doi:10.3390/bioengineering7010026
Balke, J., Volz, P., Neumann, F., Brodwolf, R., Wolf, A., Pischon, H., et al. (2018). Oxidative stress imaging: Visualizing oxidative cellular stress induced by nanoparticles in the subcytotoxic range using fluorescence lifetime imaging (small 23/2018). Small 14 (23), 1870106. doi:10.1002/smll.201870106
Barbalinardo, M., Caicci, F., Cavallini, M., and Gentili, D. (2018). Protein corona mediated uptake and cytotoxicity of silver nanoparticles in mouse embryonic fibroblast. Small 14 (34), e1801219. doi:10.1002/smll.201801219
Bayda, S., Adeel, M., Tuccinardi, T., Cordani, M., and Rizzolio, F. (2019). The history of nanoscience and nanotechnology: From chemical-physical applications to nanomedicine. Molecules 25 (1), 112. doi:10.3390/molecules25010112
Blanco, E., Shen, H., and Ferrari, M. (2015). Principles of nanoparticle design for overcoming biological barriers to drug delivery. Nat. Biotechnol. 33 (9), 941–951. doi:10.1038/nbt.3330
Bobo, D., Robinson, K. J., Islam, J., Thurecht, K. J., and Corrie, S. R. (2016). Nanoparticle-based medicines: A review of FDA-approved materials and clinical trials to date. Pharm. Res. 33 (10), 2373–2387. doi:10.1007/s11095-016-1958-5
Borm, P., Klaessig, F. C., Landry, T. D., Moudgil, B., Pauluhn, J., Thomas, K., et al. (2006). Research strategies for safety evaluation of nanomaterials, part V: Role of dissolution in biological fate and effects of nanoscale particles. Toxicol. Sci. 90 (1), 23–32. doi:10.1093/toxsci/kfj084
Bruinink, A., Wang, J., and Wick, P. (2015). Effect of particle agglomeration in nanotoxicology. Arch. Toxicol. 89 (5), 659–675. doi:10.1007/s00204-015-1460-6
Cao, Y., Li, S., and Chen, J. (2021). Modeling better in vitro models for the prediction of nanoparticle toxicity: A review. Toxicol. Mech. Methods 31 (1), 1–17. doi:10.1080/15376516.2020.1828521
Center for Drug Evaluation of Chinese National Medical Products Administration (2021). Center for drug evaluation of Chinese national medical products administration. Available at https://www.cde.org.cn/main/news/viewInfoCommon/95945bb17a7dcde7b68638525ed38f66.
Chandran, P., Riviere, J. E., and Monteiro-Riviere, N. A. (2017). Surface chemistry of gold nanoparticles determines the biocorona composition impacting cellular uptake, toxicity and gene expression profiles in human endothelial cells. Nanotoxicology 11 (4), 507–519. doi:10.1080/17435390.2017.1314036
Chang, X., Wang, X., Li, J., Shang, M., Niu, S., Zhang, W., et al. (2021). Silver nanoparticles induced cytotoxicity in HT22 cells through autophagy and apoptosis via PI3K/AKT/mTOR signaling pathway. Ecotoxicol. Environ. Saf. 208, 111696. doi:10.1016/j.ecoenv.2020.111696
Chang, X., Zhu, A., Liu, F., Zou, L., Su, L., Li, S., et al. (2017). Role of NF-κB activation and Th1/Th2 imbalance in pulmonary toxicity induced by nano NiO. Environ. Toxicol. 32 (4), 1354–1362. doi:10.1002/tox.22329
Chen, G., Roy, I., Yang, C., and Prasad, P. N. (2016). Nanochemistry and nanomedicine for nanoparticle-based diagnostics and therapy. Chem. Rev. 116 (5), 2826–2885. doi:10.1021/acs.chemrev.5b00148
Chen, R. J., Huang, C. C., Pranata, R., Lee, Y. H., Chen, Y. Y., Wu, Y. H., et al. (2021). Modulation of innate immune toxicity by silver nanoparticle exposure and the preventive effects of pterostilbene. Int. J. Mol. Sci. 22 (5), 2536. doi:10.3390/ijms22052536
Chen, Y. S., Hung, Y. C., Liau, I., and Huang, G. S. (2009). Assessment of the in vivo toxicity of gold nanoparticles. Nanoscale Res. Lett. 4 (8), 858–864. doi:10.1007/s11671-009-9334-6
Cho, W. S., Duffin, R., Bradley, M., Megson, I. L., MacNee, W., Lee, J. K., et al. (2013). Predictive value of in vitro assays depends on the mechanism of toxicity of metal oxide nanoparticles. Part. Fibre Toxicol. 10 (1), 55. doi:10.1186/1743-8977-10-55
Choi, K., Riviere, J. E., and Monteiro-Riviere, N. A. (2017). Protein corona modulation of hepatocyte uptake and molecular mechanisms of gold nanoparticle toxicity. Nanotoxicology 11 (1), 64–75. doi:10.1080/17435390.2016.1264638
Chu, K. S., Hasan, W., Rawal, S., Walsh, M. D., Enlow, E. M., Luft, J. C., et al. (2013). Plasma, tumor and tissue pharmacokinetics of Docetaxel delivered via nanoparticles of different sizes and shapes in mice bearing SKOV-3 human ovarian carcinoma xenograft. Nanomedicine Nanotechnol. Biol. Med. 9 (5), 686–693. doi:10.1016/j.nano.2012.11.008
Chusuei, C. C., Wu, C. H., Mallavarapu, S., Hou, F. Y. S., Hsu, C. M., Winiarz, J. G., et al. (2013). Cytotoxicity in the age of nano: The role of fourth period transition metal oxide nanoparticle physicochemical properties. Chem. Biol. Interact. 206 (2), 319–326. doi:10.1016/j.cbi.2013.09.020
Creutzenberg, O., Bellmann, B., Korolewitz, R., Koch, W., Mangelsdorf, I., Tillmann, T., et al. (2012). Change in agglomeration status and toxicokinetic fate of various nanoparticles in vivo following lung exposure in rats. Inhal. Toxicol. 24 (12), 821–830. doi:10.3109/08958378.2012.721097
Dai, L., Banta, G. T., Selck, H., and Forbes, V. E. (2015). Influence of copper oxide nanoparticle form and shape on toxicity and bioaccumulation in the deposit feeder, Capitella teleta. Mar. Environ. Res. 111, 99–106. doi:10.1016/j.marenvres.2015.06.010
Das, B., Tripathy, S., Adhikary, J., Chattopadhyay, S., Mandal, D., Dash, S. K., et al. (2017). Surface modification minimizes the toxicity of silver nanoparticles: An in vitro and in vivo study. J. Biol. Inorg. Chem. 22 (6), 893–918. doi:10.1007/s00775-017-1468-x
De Jong, W. H., Hagens, W. I., Krystek, P., Burger, M. C., Sips, A. J., and Geertsma, R. E. (2008). Particle size-dependent organ distribution of gold nanoparticles after intravenous administration. Biomaterials 29 (12), 1912–1919. doi:10.1016/j.biomaterials.2007.12.037
De Simone, U., Roccio, M., Gribaldo, L., Spinillo, A., Caloni, F., and Coccini, T. (2018). Human 3D cultures as models for evaluating magnetic nanoparticle CNS cytotoxicity after short- and repeated long-term exposure. Int. J. Mol. Sci. 19 (7), 1993. doi:10.3390/ijms19071993
Debayle, M., Balloul, E., Dembele, F., Xu, X., Hanafi, M., Ribot, F., et al. (2019). Zwitterionic polymer ligands: An ideal surface coating to totally suppress protein-nanoparticle corona formation? Biomaterials 219, 119357. doi:10.1016/j.biomaterials.2019.119357
Demir, E. (2021). A review on nanotoxicity and nanogenotoxicity of different shapes of nanomaterials. J. Appl. Toxicol. 41 (1), 118–147. doi:10.1002/jat.4061
Di Bucchianico, S., Cappellini, F., Le Bihanic, F., Zhang, Y., Dreij, K., and Karlsson, H. L. (2017). Genotoxicity of TiO2 nanoparticles assessed by mini-gel comet assay and micronucleus scoring with flow cytometry. Mutagenesis 32 (1), 127–137. doi:10.1093/mutage/gew030
Diez-Pascual, A. M. (2021). Carbon-based nanomaterials. Int. J. Mol. Sci. 22 (14), 7726. doi:10.3390/ijms22147726
Dobrovolskaia, M. A., Clogston, J. D., Neun, B. W., Hall, J. B., Patri, A. K., and McNeil, S. E. (2008). Method for analysis of nanoparticle hemolytic properties in vitro. Nano Lett. 8 (8), 2180–2187. doi:10.1021/nl0805615
Donahue, N. D., Acar, H., and Wilhelm, S. (2019). Concepts of nanoparticle cellular uptake, intracellular trafficking, and kinetics in nanomedicine. Adv. Drug Deliv. Rev. 143, 68–96. doi:10.1016/j.addr.2019.04.008
Duran, N., Silveira, C. P., Duran, M., and Martinez, D. S. T. (2015). Silver nanoparticle protein corona and toxicity: A mini-review. J. Nanobiotechnology 13, 55. doi:10.1186/s12951-015-0114-4
Fang, R. H., Kroll, A. V., Gao, W., and Zhang, L. (2018). Cell membrane coating nanotechnology. Adv. Mat. 30 (23), e1706759. doi:10.1002/adma.201706759
Fernando, I., and Zhou, Y. (2019). Impact of pH on the stability, dissolution and aggregation kinetics of silver nanoparticles. Chemosphere 216, 297–305. doi:10.1016/j.chemosphere.2018.10.122
Ferreira Soares, D. C., Domingues, S. C., Viana, D. B., and Tebaldi, M. L. (2020). Polymer-hybrid nanoparticles: Current advances in biomedical applications. Biomed. Pharmacother. 131, 110695. doi:10.1016/j.biopha.2020.110695
Forest, V., Leclerc, L., Hochepied, J. F., Trouve, A., Sarry, G., and Pourchez, J. (2017). Impact of cerium oxide nanoparticles shape on their in vitro cellular toxicity. Toxicol. Vitro 38, 136–141. doi:10.1016/j.tiv.2016.09.022
Forest, V., Pourchez, J., Pelissier, C., Audignon Durand, S., Vergnon, J. M., and Fontana, L. (2021). Relationship between occupational exposure to airborne nanoparticles, nanoparticle lung burden and lung diseases. Toxics 9 (9), 204. doi:10.3390/toxics9090204
Forrester, S. J., Kikuchi, D. S., Hernandes, M. S., Xu, Q., and Griendling, K. K. (2018). Reactive oxygen species in metabolic and inflammatory signaling. Circ. Res. 122 (6), 877–902. doi:10.1161/circresaha.117.311401
Frohlich, E. (2017). Role of omics techniques in the toxicity testing of nanoparticles. J. Nanobiotechnology 15 (1), 84. doi:10.1186/s12951-017-0320-3
Frohlich, E. (2012). The role of surface charge in cellular uptake and cytotoxicity of medical nanoparticles. Int. J. Nanomedicine 7, 5577–5591. doi:10.2147/ijn.s36111
Fu, P. P., Xia, Q., Hwang, H. M., Ray, P. C., and Yu, H. (2014). Mechanisms of nanotoxicity: Generation of reactive oxygen species. J. Food Drug Anal. 22 (1), 64–75. doi:10.1016/j.jfda.2014.01.005
Fubini, B., and Hubbard, A. (2003). Reactive oxygen species (ROS) and reactive nitrogen species (RNS) generation by silica in inflammation and fibrosis. Free Radic. Biol. Med. 34 (12), 1507–1516. doi:10.1016/s0891-5849(03)00149-7
Furxhi, I., Murphy, F., Mullins, M., and Poland, C. A. (2019). Machine learning prediction of nanoparticle in vitro toxicity: A comparative study of classifiers and ensemble-classifiers using the Copeland Index. Toxicol. Lett. 312, 157–166. doi:10.1016/j.toxlet.2019.05.016
Gajewicz, A., Jagiello, K., Cronin, M. T. D., Leszczynski, J., and Puzyn, T. (2017). Addressing a bottle neck for regulation of nanomaterials: Quantitative read-across (Nano-QRA) algorithm for cases when only limited data is available. Environ. Sci. Nano 4, 346–358. doi:10.1039/c6en00399k
Geng, Y., Dalhaimer, P., Cai, S., Tsai, R., Tewari, M., Minko, T., et al. (2007). Shape effects of filaments versus spherical particles in flow and drug delivery. Nat. Nanotechnol. 2 (4), 249–255. doi:10.1038/nnano.2007.70
Godin, B., Chiappini, C., Srinivasan, S., Alexander, J. F., Yokoi, K., Ferrari, M., et al. (2012). Discoidal porous silicon particles: Fabrication and biodistribution in breast cancer bearing mice. Adv. Funct. Mat. 22 (20), 4225–4235. doi:10.1002/adfm.201200869
Goodman, C. M., McCusker, C. D., Yilmaz, T., and Rotello, V. M. (2004). Toxicity of gold nanoparticles functionalized with cationic and anionic side chains. Bioconjug. Chem. 15 (4), 897–900. doi:10.1021/bc049951i
Grouchko, M., Popov, I., Uvarov, V., Magdassi, S., and Kamyshny, A. (2009). Coalescence of silver nanoparticles at room temperature: Unusual crystal structure transformation and dendrite formation induced by self-assembly. Langmuir 25 (4), 2501–2503. doi:10.1021/la803843k
Guerrero, D. J. P., Bonilla, J. J. A., Lopez, C. C. O., and Saez, R. G. T. (2019). Encapsulation of silver nanoparticles in polylactic acid or poly(lactic-co-glycolic acid) and their antimicrobial and cytotoxic activities. J. Nanosci. Nanotechnol. 19 (11), 6933–6941. doi:10.1166/jnn.2019.16663
Guo, C., Xia, Y., Niu, P., Jiang, L., Duan, J., Yu, Y., et al. (2015). Silica nanoparticles induce oxidative stress, inflammation, and endothelial dysfunction in vitro via activation of the MAPK/Nrf2 pathway and nuclear factor-κB signaling. Int. J. Nanomedicine 10, 1463–1477. doi:10.2147/ijn.s76114
Gurr, J. R., Wang, A. S., Chen, C. H., and Jan, K. Y. (2005). Ultrafine titanium dioxide particles in the absence of photoactivation can induce oxidative damage to human bronchial epithelial cells. Toxicology 213 (1-2), 66–73. doi:10.1016/j.tox.2005.05.007
Guzman, K. A., Finnegan, M. P., and Banfield, J. F. (2006). Influence of surface potential on aggregation and transport of titania nanoparticles. Environ. Sci. Technol. 40 (24), 7688–7693. doi:10.1021/es060847g
Gwinn, M. R., and Vallyathan, V. (2006). Nanoparticles: Health effects-pros and cons. Environ. Health Perspect. 114 (12), 1818–1825. doi:10.1289/ehp.8871
Hadji, H., and Bouchemal, K. (2022). Effect of micro- and nanoparticle shape on biological processes. J. Control. Release 342, 93–110. doi:10.1016/j.jconrel.2021.12.032
Han, Z., Sarkar, S., and Smith, A. M. (2020). Zwitterion and oligo(ethylene glycol) synergy minimizes nonspecific binding of compact quantum dots. ACS Nano 14 (3), 3227–3241. doi:10.1021/acsnano.9b08658
Haque, E., and Ward, A. C. (2018). Zebrafish as a model to evaluate nanoparticle toxicity. Nanomater. (Basel) 8 (7), 561. doi:10.3390/nano8070561
He, C., Jiang, S., Jin, H., Chen, S., Lin, G., Yao, H., et al. (2016). Mitochondrial electron transport chain identified as a novel molecular target of SPIO nanoparticles mediated cancer-specific cytotoxicity. Biomaterials 83, 102–114. doi:10.1016/j.biomaterials.2016.01.010
He, X., Deng, H., and Hwang, H. M. (2019). The current application of nanotechnology in food and agriculture. J. Food Drug Anal. 27 (1), 1–21. doi:10.1016/j.jfda.2018.12.002
He, X., and Yoshioka, Y. (2011). Quantifying the biodistribution of nanoparticles. Nat. Nanotechnol. 6 (12), 755. doi:10.1038/nnano.2011.220
Hoet, P. H., Bruske-Hohlfeld, I., and Salata, O. V. (2004). Nanoparticles - known and unknown health risks. J. Nanobiotechnology 2 (1), 12. doi:10.1186/1477-3155-2-12
Horie, M., and Tabei, Y. (2021). Role of oxidative stress in nanoparticles toxicity. Free Radic. Res. 55 (4), 331–342. doi:10.1080/10715762.2020.1859108
Hosseini, M. J., Shaki, F., Ghazi-Khansari, M., and Pourahmad, J. (2014). Toxicity of copper on isolated liver mitochondria: Impairment at complexes I, II, and IV leads to increased ROS production. Cell biochem. Biophys. 70 (1), 367–381. doi:10.1007/s12013-014-9922-7
Hu, C. M., Fang, R. H., Wang, K. C., Luk, B. T., Thamphiwatana, S., Dehaini, D., et al. (2015). Nanoparticle biointerfacing by platelet membrane cloaking. Nature 526 (7571), 118–121. doi:10.1038/nature15373
Hu, C. M., Zhang, L., Aryal, S., Cheung, C., Fang, R. H., and Zhang, L. (2011). Erythrocyte membrane-camouflaged polymeric nanoparticles as a biomimetic delivery platform. Proc. Natl. Acad. Sci. U. S. A. 108 (27), 10980–10985. doi:10.1073/pnas.1106634108
Hu, W., Peng, C., Lv, M., Li, X., Zhang, Y., Chen, N., et al. (2011). Protein corona-mediated mitigation of cytotoxicity of graphene oxide. ACS Nano 5 (5), 3693–3700. doi:10.1021/nn200021j
Huang, C., Zhang, Y., Yuan, H., Gao, H., and Zhang, S. (2013). Role of nanoparticle geometry in endocytosis: Laying down to stand up. Nano Lett. 13 (9), 4546–4550. doi:10.1021/nl402628n
Huang, Y. W., Wu, C. H., and Aronstam, R. S. (2010). Toxicity of transition metal oxide nanoparticles: Recent insights from in vitro studies. Mater. (Basel) 3 (10), 4842–4859. doi:10.3390/ma3104842
Huhn, D., Kantner, K., Geidel, C., Brandholt, S., De Cock, I., Soenen, S. J. H., et al. (2013). Polymer-coated nanoparticles interacting with proteins and cells: Focusing on the sign of the net charge. ACS Nano 7 (4), 3253–3263. doi:10.1021/nn3059295
Huo, S., Jin, S., Ma, X., Xue, X., Yang, K., Kumar, A., et al. (2014). Ultrasmall gold nanoparticles as carriers for nucleus-based gene therapy due to size-dependent nuclear entry. ACS Nano 8 (6), 5852–5862. doi:10.1021/nn5008572
Hwang, R., Mirshafiee, V., Zhu, Y., and Xia, T. (2018). Current approaches for safer design of engineered nanomaterials. Ecotoxicol. Environ. Saf. 166, 294–300. doi:10.1016/j.ecoenv.2018.09.077
Jakubczyk, K., Dec, K., Kaldunska, J., Kawczuga, D., Kochman, J., and Janda, K. (2020). Reactive oxygen species - sources, functions, oxidative damage. Pol. Merkur. Lek. 48 (284), 124–127.
Jeon, S., Clavadetscher, J., Lee, D. K., Chankeshwara, S., Bradley, M., and Cho, W. S. (2018). Surface charge-dependent cellular uptake of polystyrene nanoparticles. Nanomater. (Basel) 8 (12), 1028. doi:10.3390/nano8121028
Jiao, F., Sang, J., Liu, Z., Liu, W., and Liang, W. (2020). Effect of concentration of PEG coated gold nanoparticle on lung surfactant studied with coarse-grained molecular dynamics simulations. Biophys. Chem. 266, 106457. doi:10.1016/j.bpc.2020.106457
Johnston, H. J., Hutchison, G., Christensen, F. M., Peters, S., Hankin, S., and Stone, V. (2010). A review of the in vivo and in vitro toxicity of silver and gold particulates: Particle attributes and biological mechanisms responsible for the observed toxicity. Crit. Rev. Toxicol. 40 (4), 328–346. doi:10.3109/10408440903453074
Joralemon, M. J., McRae, S., and Emrick, T. (2010). PEGylated polymers for medicine: From conjugation to self-assembled systems. Chem. Commun. 46 (9), 1377–1393. doi:10.1039/b920570p
Kai, W., Xiaojun, X., Ximing, P., Zhenqing, H., and Qiqing, Z. (2011). Cytotoxic effects and the mechanism of three types of magnetic nanoparticles on human hepatoma BEL-7402 cells. Nanoscale Res. Lett. 6, 480. doi:10.1186/1556-276x-6-480
Kalashnikova, I., Chung, S. J., Nafiujjaman, M., Hill, M. L., Siziba, M. E., Contag, C. H., et al. (2020). Ceria-based nanotheranostic agent for rheumatoid arthritis. Theranostics 10 (26), 11863–11880. doi:10.7150/thno.49069
Kar, S., Gajewicz, A., Puzyn, T., Roy, K., and Leszczynski, J. (2014). Periodic table-based descriptors to encode cytotoxicity profile of metal oxide nanoparticles: A mechanistic QSTR approach. Ecotoxicol. Environ. Saf. 107, 162–169. doi:10.1016/j.ecoenv.2014.05.026
Karakoti, A. S., Das, S., Thevuthasan, S., and Seal, S. (2011). PEGylated inorganic nanoparticles. Angew. Chem. Int. Ed. 50 (9), 1980–1994. doi:10.1002/anie.201002969
Karakoti, A. S., Kuchibhatla, S. V. N. T., Baer, D. R., Thevuthasan, S., Sayle, D. C., and Seal, S. (2008). Self-assembly of cerium oxide nanostructures in ice molds. Small 4 (8), 1210–1216. doi:10.1002/smll.200800219
Karlsson, H. L., Cronholm, P., Gustafsson, J., and Moller, L. (2008). Copper oxide nanoparticles are highly toxic: A comparison between metal oxide nanoparticles and carbon nanotubes. Chem. Res. Toxicol. 21 (9), 1726–1732. doi:10.1021/tx800064j
Khan, I., Saeed, K., and Khan, I. (2019). Nanoparticles: Properties, applications and toxicities. Arabian J. Chem. 12 (7), 908–931. doi:10.1016/j.arabjc.2017.05.011
Khanna, P., Ong, C., Bay, B., and Baeg, G. (2015). Nanotoxicity: An interplay of oxidative stress, inflammation and cell death. Nanomater. (Basel) 5 (3), 1163–1180. doi:10.3390/nano5031163
Kim, H., Jeong, J., Chatterjee, N., Roca, C. P., Yoon, D., Kim, S., et al. (2017). JAK/STAT and TGF-ß activation as potential adverse outcome pathway of TiO2NPs phototoxicity in Caenorhabditis elegans. Sci. Rep. 7 (1), 17833. doi:10.1038/s41598-017-17495-8
Kim, M. S., Louis, K. M., Pedersen, J. A., Hamers, R. J., Peterson, R. E., and Heideman, W. (2014). Using citrate-functionalized TiO2 nanoparticles to study the effect of particle size on zebrafish embryo toxicity. Analyst 139 (5), 964–972. doi:10.1039/c3an01966g
Ko, J. W., Park, J. W., Shin, N. R., Kim, J. H., Cho, Y. K., Shin, D. H., et al. (2016). Copper oxide nanoparticle induces inflammatory response and mucus production via MAPK signaling in human bronchial epithelial cells. Environ. Toxicol. Pharmacol. 43, 21–26. doi:10.1016/j.etap.2016.02.008
Kreyling, W. G., Semmler-Behnke, M., Seitz, J., Scymczak, W., Wenk, A., Mayer, P., et al. (2009). Size dependence of the translocation of inhaled iridium and carbon nanoparticle aggregates from the lung of rats to the blood and secondary target organs. Inhal. Toxicol. 21 (1), 55–60. doi:10.1080/08958370902942517
Kroll, A., Pillukat, M. H., Hahn, D., and Schnekenburger, J. (2012). Interference of engineered nanoparticles with in vitro toxicity assays. Arch. Toxicol. 86 (7), 1123–1136. doi:10.1007/s00204-012-0837-z
Kumari, S., Yadav, B. S., and Yadav, R. B. (2020). Synthesis and modification approaches for starch nanoparticles for their emerging food industrial applications: A review. Food Res. Int. 128, 108765. doi:10.1016/j.foodres.2019.108765
Labouta, H. I., Asgarian, N., Rinker, K., and Cramb, D. T. (2019). Meta-analysis of nanoparticle cytotoxicity via data-mining the literature. ACS Nano 13 (2), 1583–1594. doi:10.1021/acsnano.8b07562
Lai, R. W. S., Kang, H. M., Zhou, G. J., Yung, M. M. N., He, Y. L., et al. (2021). Hydrophobic surface coating can reduce toxicity of zinc oxide nanoparticles to the marine copepod Tigriopus japonicus. Environ. Sci. Technol. 55 (10), 6917–6925. doi:10.1021/acs.est.1c01300
Lanone, S., Rogerieux, F., Geys, J., Dupont, A., Maillot-Marechal, E., Boczkowski, J., et al. (2009). Comparative toxicity of 24 manufactured nanoparticles in human alveolar epithelial and macrophage cell lines. Part. Fibre Toxicol. 6, 14. doi:10.1186/1743-8977-6-14
Lee, J. H., Ju, J. E., Kim, B. I., Pak, P. J., Choi, E. K., Lee, H. S., et al. (2014). Rod-shaped iron oxide nanoparticles are more toxic than sphere-shaped nanoparticles to murine macrophage cells. Environ. Toxicol. Chem. 33 (12), 2759–2766. doi:10.1002/etc.2735
Lee, K. I., Su, C. C., Fang, K. M., Wu, C. C., Wu, C. T., and Chen, Y. W. (2020). Ultrafine silicon dioxide nanoparticles cause lung epithelial cells apoptosis via oxidative stress-activated PI3K/Akt-mediated mitochondria- and endoplasmic reticulum stress-dependent signaling pathways. Sci. Rep. 10 (1), 9928. doi:10.1038/s41598-020-66644-z
Lee, S. H., and Jun, B. H. (2019). Silver nanoparticles: Synthesis and application for nanomedicine. Int. J. Mol. Sci. 20 (4), 865. doi:10.3390/ijms20040865
Lee, Y. K., Choi, E. J., Webster, T. J., Kim, S. H., and Khang, D. (2015). Effect of the protein corona on nanoparticles for modulating cytotoxicity and immunotoxicity. Int. J. Nanomedicine 10, 97–113. doi:10.2147/IJN.S72998
Li, B., and Tang, M. (2020). Research progress of nanoparticle toxicity signaling pathway. Life Sci. 263, 118542. doi:10.1016/j.lfs.2020.118542
Li, H., Li, Q. Q., and Hong, Y. (2021). Global gene expression signatures in response to citrate-coated silver nanoparticles exposure. Toxicology 461, 152898. doi:10.1016/j.tox.2021.152898
Li, J., Wang, C., Yue, L., Chen, F., Cao, X., and Wang, Z. (2022). Nano-QSAR modeling for predicting the cytotoxicity of metallic and metal oxide nanoparticles: A review. Ecotoxicol. Environ. Saf. 243, 113955. doi:10.1016/j.ecoenv.2022.113955
Li, M., Zhu, L., and Lin, D. (2011). Toxicity of ZnO nanoparticles to Escherichia coli: Mechanism and the influence of medium components. Environ. Sci. Technol. 45 (5), 1977–1983. doi:10.1021/es102624t
Li, R., Wang, X., Ji, Z., Sun, B., Zhang, H., Chang, C. H., et al. (2013). Surface charge and cellular processing of covalently functionalized multiwall carbon nanotubes determine pulmonary toxicity. ACS Nano 7 (3), 2352–2368. doi:10.1021/nn305567s
Liu, L., Bai, X., Martikainen, M. V., Karlund, A., Roponen, M., Xu, W., et al. (2021). Cell membrane coating integrity affects the internalization mechanism of biomimetic nanoparticles. Nat. Commun. 12 (1), 5726. doi:10.1038/s41467-021-26052-x
Liu, S., Yu, B., Wang, S., Shen, Y., and Cong, H. (2020). Preparation, surface functionalization and application of Fe3O4 magnetic nanoparticles. Adv. Colloid Interface Sci. 281, 102165. doi:10.1016/j.cis.2020.102165
Liu, X., Tang, I., Wainberg, Z. A., and Meng, H. (2020). Safety considerations of cancer nanomedicine-A key step toward translation. Small 16 (36), e2000673. doi:10.1002/smll.202000673
Lou, L., Subbiah, S., Smith, E., Kendall, R. J., and Ramkumar, S. S. (2019). Functional PVA/VB2/TiO2 nanofiber webs for controlled drug delivery. ACS Appl. Bio Mat. 2 (12), 5916–5929. doi:10.1021/acsabm.9b00726
Lu, N., Sui, Y., Ding, Y., Tian, R., and Peng, Y. Y. (2018). Fibrinogen binding-dependent cytotoxicity and degradation of single-walled carbon nanotubes. J. Mat. Sci. Mat. Med. 29 (8), 115. doi:10.1007/s10856-018-6123-8
Mahmoud, A. M., Desouky, E. M., Hozayen, W. G., Bin-Jumah, M., El-Nahass, E. S., Soliman, H. A., et al. (2019). Mesoporous silica nanoparticles trigger liver and kidney injury and fibrosis via altering TLR4/NF-κB, JAK2/STAT3 and Nrf2/HO-1 signaling in rats. Biomolecules 9 (10), 528. doi:10.3390/biom9100528
Makhdoumi, P., Karimi, H., and Khazaei, M. (2020). Review on metal-based nanoparticles: Role of reactive oxygen species in renal toxicity. Chem. Res. Toxicol. 33 (10), 2503–2514. doi:10.1021/acs.chemrestox.9b00438
Manuja, A., Kumar, B., Kumar, R., Chhabra, D., Ghosh, M., Manuja, M., et al. (2021). Metal/metal oxide nanoparticles: Toxicity concerns associated with their physical state and remediation for biomedical applications. Toxicol. Rep. 8, 1970–1978. doi:10.1016/j.toxrep.2021.11.020
Masoud, R., Bizouarn, T., Trepout, S., Wien, F., Baciou, L., Marco, S., et al. (2015). Titanium dioxide nanoparticles increase superoxide anion production by acting on NADPH oxidase. PLoS One 10 (12), e0144829. doi:10.1371/journal.pone.0144829
Matysiak, M., Kapka-Skrzypczak, L., Brzoska, K., Gutleb, A. C., and Kruszewski, M. (2016). Proteomic approach to nanotoxicity. J. Proteomics 137, 35–44. doi:10.1016/j.jprot.2015.10.025
Meka, R. R., Mukherjee, S., Patra, C. R., and Chaudhuri, A. (2019). Shikimoyl-ligand decorated gold nanoparticles for use in ex vivo engineered dendritic cell based DNA vaccination. Nanoscale 11 (16), 7931–7943. doi:10.1039/c8nr10293g
Mishra, M., and Panda, M. (2021). Reactive oxygen species: The root cause of nanoparticle-induced toxicity in Drosophila melanogaster. Free Radic. Res. 55 (6), 919–935. doi:10.1080/10715762.2021.1914335
Mishra, R. K., Ahmad, A., Vyawahare, A., Alam, P., Khan, T. H., and Khan, R. (2021). Biological effects of formation of protein corona onto nanoparticles. Int. J. Biol. Macromol. 175, 1–18. doi:10.1016/j.ijbiomac.2021.01.152
Misra, S. K., Dybowska, A., Berhanu, D., Croteau, M. N., Luoma, S. N., Boccaccini, A. R., et al. (2012). Isotopically modified nanoparticles for enhanced detection in bioaccumulation studies. Environ. Sci. Technol. 46 (2), 1216–1222. doi:10.1021/es2039757
Misra, S. K., Dybowska, A., Berhanu, D., Luoma, S. N., and Valsami-Jones, E. (2012). The complexity of nanoparticle dissolution and its importance in nanotoxicological studies. Sci. Total Environ. 438, 225–232. doi:10.1016/j.scitotenv.2012.08.066
Missaoui, W. N., Arnold, R. D., and Cummings, B. S. (2018). Toxicological status of nanoparticles: What we know and what we don't know. Chem. Biol. Interact. 295, 1–12. doi:10.1016/j.cbi.2018.07.015
Mout, R., Moyano, D. F., Rana, S., and Rotello, V. M. (2012). Surface functionalization of nanoparticles for nanomedicine. Chem. Soc. Rev. 41 (7), 2539–2544. doi:10.1039/c2cs15294k
Mudshinge, S. R., Deore, A. B., Patil, S., and Bhalgat, C. M. (2011). Nanoparticles: Emerging carriers for drug delivery. Saudi Pharm. J. 19 (3), 129–141. doi:10.1016/j.jsps.2011.04.001
Mulens-Arias, V., Rojas, J. M., and Barber, D. F. (2021). The use of iron oxide nanoparticles to reprogram macrophage responses and the immunological tumor microenvironment. Front. Immunol. 12, 693709. doi:10.3389/fimmu.2021.693709
Murphy, D. A., Cheng, H., Yang, T., Yan, X., and Adjei, I. M. (2021). Reversing hypoxia with PLGA-encapsulated manganese dioxide nanoparticles improves natural killer cell response to tumor spheroids. Mol. Pharm. 18 (8), 2935–2946. doi:10.1021/acs.molpharmaceut.1c00085
Murugadoss, S., Brassinne, F., Sebaihi, N., Petry, J., Cokic, S. M., Van Landuyt, K. L., et al. (2020). Agglomeration of titanium dioxide nanoparticles increases toxicological responses in vitro and in vivo. Part. Fibre Toxicol. 17 (1), 10. doi:10.1186/s12989-020-00341-7
Najahi-Missaoui, W., Arnold, R. D., and Cummings, B. S. (2020). Safe nanoparticles: Are we there yet? Int. J. Mol. Sci. 22 (1), 385. doi:10.3390/ijms22010385
Nel, A. E., Madler, L., Velegol, D., Xia, T., Hoek, E. M. V., Somasundaran, P., et al. (2009). Understanding biophysicochemical interactions at the nano-bio interface. Nat. Mat. 8 (7), 543–557. doi:10.1038/nmat2442
Nemmar, A., Yuvaraju, P., Beegam, S., Pathan, J., Kazzam, E. E., and Ali, B. H. (2016). Oxidative stress, inflammation, and DNA damage in multiple organs of mice acutely exposed to amorphous silica nanoparticles. Int. J. Nanomedicine 11, 919–928. doi:10.2147/ijn.s92278
Nicol, J. R., Dixon, D., and Coulter, J. A. (2015). Gold nanoparticle surface functionalization: A necessary requirement in the development of novel nanotherapeutics. Nanomedicine (Lond) 10 (8), 1315–1326. doi:10.2217/nnm.14.219
Oertel, J., Keller, A., Prinz, J., Schreiber, B., Hubner, R., Kerbusch, J., et al. (2016). Anisotropic metal growth on phospholipid nanodiscs via lipid bilayer expansion. Sci. Rep. 6, 26718. doi:10.1038/srep26718
Ovais, M., Nethi, S. K., Ullah, S., Ahmad, I., Mukherjee, S., and Chen, C. (2020). Recent advances in the analysis of nanoparticle-protein coronas. Nanomedicine (Lond) 15 (10), 1037–1061. doi:10.2217/nnm-2019-0381
Pan, Y., Neuss, S., Leifert, A., Fischler, M., Wen, F., Simon, U., et al. (2007). Size-dependent cytotoxicity of gold nanoparticles. Small 3 (11), 1941–1949. doi:10.1002/smll.200700378
Patil, U. S., Adireddy, S., Jaiswal, A., Mandava, S., Lee, B., Chrisey, D., et al. (2015). In vitro/in vivo toxicity evaluation and quantification of iron oxide nanoparticles. Int. J. Mol. Sci. 16 (10), 24417–24450. doi:10.3390/ijms161024417
Patlolla, A. K., Kumari, S. A., and Tchounwou, P. B. (2019). Erratum: A comparison of poly-ethylene-glycol-coated and uncoated gold nanoparticle-mediated hepatotoxicity and oxidative stress in sprague-dawley rats [corrigendum]. Int. J. Nanomedicine 14, 7793. doi:10.2147/IJN.S185574
Patsula, V., Tulinska, J., Trachtova, S., Kuricova, M., Liskova, A., Spanova, A., et al. (2019). Toxicity evaluation of monodisperse PEGylated magnetic nanoparticles for nanomedicine. Nanotoxicology 13 (4), 510–526. doi:10.1080/17435390.2018.1555624
Peretyazhko, T. S., Zhang, Q., and Colvin, V. L. (2014). Size-controlled dissolution of silver nanoparticles at neutral and acidic pH conditions: Kinetics and size changes. Environ. Sci. Technol. 48 (20), 11954–11961. doi:10.1021/es5023202
Poyton, R. O., Ball, K. A., and Castello, P. R. (2009). Mitochondrial generation of free radicals and hypoxic signaling. Trends Endocrinol. Metab. 20 (7), 332–340. doi:10.1016/j.tem.2009.04.001
Puglia, C., and Santonocito, D. (2019). Cosmeceuticals: Nanotechnology-Based strategies for the delivery of phytocompounds. Curr. Pharm. Des. 25 (21), 2314–2322. doi:10.2174/1381612825666190709211101
Puzyn, T., Leszczynska, D., and Leszczynski, J. (2009). Toward the development of âœNano-QSARsâ: Advances and challenges. Small 5 (22), 2494–2509. doi:10.1002/smll.200900179
Puzyn, T., Rasulev, B., Gajewicz, A., Hu, X., Dasari, T. P., Michalkova, A., et al. (2011). Using nano-QSAR to predict the cytotoxicity of metal oxide nanoparticles. Nat. Nanotechnol. 6 (3), 175–178. doi:10.1038/nnano.2011.10
Qi, X., Yao, M., Jin, M., and Guo, H. (2021). Application of magnetic resonance imaging based on Fe(3)O(4) nanoparticles in the treatment of cerebrovascular diseases. J. Nanosci. Nanotechnol. 21 (2), 843–851. doi:10.1166/jnn.2021.18697
Ragelle, H., Danhier, F., Preat, V., Langer, R., and Anderson, D. G. (2017). Nanoparticle-based drug delivery systems: A commercial and regulatory outlook as the field matures. Expert Opin. Drug Deliv. 14 (7), 851–864. doi:10.1080/17425247.2016.1244187
Ramasamy, M., Yi, D. K., and Das, M. (2014). Role of surface modification in zinc oxide nanoparticles and its toxicity assessment toward human dermal fibroblast cells. Int. J. Nanomedicine 9, 3707–3718. doi:10.2147/ijn.s65086
Rao, L., Meng, Q. F., Bu, L. L., Cai, B., Huang, Q., Sun, Z. J., et al. (2017). Erythrocyte membrane-coated upconversion nanoparticles with minimal protein adsorption for enhanced tumor imaging. ACS Appl. Mat. Interfaces 9 (3), 2159–2168. doi:10.1021/acsami.6b14450
Rao, L., Xu, J. H., Cai, B., Liu, H., Li, M., Jia, Y., et al. (2016). Synthetic nanoparticles camouflaged with biomimetic erythrocyte membranes for reduced reticuloendothelial system uptake. Nanotechnology 27 (8), 085106. doi:10.1088/0957-4484/27/8/085106
Rashki, S., Asgarpour, K., Tarrahimofrad, H., Hashemipour, M., Ebrahimi, M. S., Fathizadeh, H., et al. (2021). Chitosan-based nanoparticles against bacterial infections. Carbohydr. Polym. 251, 117108. doi:10.1016/j.carbpol.2020.117108
Ravindran, S., Suthar, J. K., Rokade, R., Deshpande, P., Singh, P., Pratinidhi, A., et al. (2018). Pharmacokinetics, metabolism, distribution and permeability of nanomedicine. Curr. Drug Metab. 19 (4), 327–334. doi:10.2174/1389200219666180305154119
Ray, P. D., Huang, B. W., and Tsuji, Y. (2012). Reactive oxygen species (ROS) homeostasis and redox regulation in cellular signaling. Cell. Signal. 24 (5), 981–990. doi:10.1016/j.cellsig.2012.01.008
Recordati, C., De Maglie, M., Bianchessi, S., Argentiere, S., Cella, C., Mattiello, S., et al. (2016). Tissue distribution and acute toxicity of silver after single intravenous administration in mice: Nano-specific and size-dependent effects. Part. Fibre Toxicol. 13, 12. doi:10.1186/s12989-016-0124-x
Richarz, A. N., Avramopoulos, A., Benfenati, E., Gajewicz, A., Golbamaki Bakhtyari, N., Leonis, G., et al. (2017). Compilation of data and modelling of nanoparticle interactions and toxicity in the NanoPUZZLES Project. Adv. Exp. Med. Biol. 947, 303–324. doi:10.1007/978-3-319-47754-1_10
Romolini, G., Gambucci, M., Ricciarelli, D., Tarpani, L., Zampini, G., and Latterini, L. (2021). Photocatalytic activity of silica and silica-silver nanocolloids based on photo-induced formation of reactive oxygen species. Photochem. Photobiol. Sci. 20 (9), 1161–1172. doi:10.1007/s43630-021-00089-9
Ronavari, A., Belteky, P., Boka, E., Zakupszky, D., Igaz, N., Szerencses, B., et al. (2021). Polyvinyl-pyrrolidone-coated silver nanoparticles-the colloidal, chemical, and biological consequences of steric stabilization under biorelevant conditions. Int. J. Mol. Sci. 22 (16), 8673. doi:10.3390/ijms22168673
Roshanzadeh, A., Park, S., Ganjbakhsh, S. E., Park, J., Lee, D. H., Lee, S., et al. (2020). Surface charge-dependent cytotoxicity of plastic nanoparticles in alveolar cells under cyclic stretches. Nano Lett. 20 (10), 7168–7176. doi:10.1021/acs.nanolett.0c02463
Ruan, C., Wang, C., Gong, X., Zhang, Y., Deng, W., Zhou, J., et al. (2021). An integrative multi-omics approach uncovers the regulatory role of CDK7 and CDK4 in autophagy activation induced by silica nanoparticles. Autophagy 17 (6), 1426–1447. doi:10.1080/15548627.2020.1763019
Ruge, C. A., Kirch, J., Canadas, O., Schneider, M., Perez-Gil, J., Schaefer, U. F., et al. (2011). Uptake of nanoparticles by alveolar macrophages is triggered by surfactant protein A. Nanomedicine Nanotechnol. Biol. Med. 7 (6), 690–693. doi:10.1016/j.nano.2011.07.009
Rybinska-Fryca, A., Mikolajczyk, A., and Puzyn, T. (2020). Structure-activity prediction networks (SAPNets): A step beyond nano-QSAR for effective implementation of the safe-by-design concept. Nanoscale 12 (40), 20669–20676. doi:10.1039/d0nr05220e
Saifi, M. A., Khan, W., and Godugu, C. (2018). Cytotoxicity of nanomaterials: Using nanotoxicology to address the safety concerns of nanoparticles. Pharm. Nanotechnol. 6 (1), 3–16. doi:10.2174/2211738505666171023152928
Sarkar, P., and Chattopadhyay, A. (2021). Insights into cellular signaling from membrane dynamics. Arch. Biochem. Biophys. 701, 108794. doi:10.1016/j.abb.2021.108794
Sayes, C. M., Reed, K. L., and Warheit, D. B. (2007). Assessing toxicity of fine and nanoparticles: Comparing in vitro measurements to in vivo pulmonary toxicity profiles. Toxicol. Sci. 97 (1), 163–180. doi:10.1093/toxsci/kfm018
Schulte, P. A., Schubauer-Berigan, M. K., Mayweather, C., Geraci, C. L., Zumwalde, R., and McKernan, J. L. (2009). Issues in the development of epidemiologic studies of workers exposed to engineered nanoparticles. J. Occup. Environ. Med. 51 (3), 323–335. doi:10.1097/jom.0b013e3181990c2c
Seleci, M., Scheper, T., and Stahl, F. (2017). Theranostic liposome-nanoparticle hybrids for drug delivery and bioimaging. Int. J. Mol. Sci. 18 (7), 1415. doi:10.3390/ijms18071415
Shahbazi, M. A., Hamidi, M., Makila, E. M., Zhang, H., Almeida, P. V., Kaasalainen, M., et al. (2013). The mechanisms of surface chemistry effects of mesoporous silicon nanoparticles on immunotoxicity and biocompatibility. Biomaterials 34 (31), 7776–7789. doi:10.1016/j.biomaterials.2013.06.052
Shen, N., Yan, F., Pang, J., Gao, Z., Al-Kali, A., Haynes, C. L., et al. (2018). HDL-AuNPs-BMS nanoparticle conjugates as molecularly targeted therapy for leukemia. ACS Appl. Mat. Interfaces 10 (17), 14454–14462. doi:10.1021/acsami.8b01696
Shim, W., Paik, M. J., Nguyen, D. T., Lee, J. K., Lee, Y., Kim, J. H., et al. (2012). Analysis of changes in gene expression and metabolic profiles induced by silica-coated magnetic nanoparticles. ACS Nano 6 (9), 7665–7680. doi:10.1021/nn301113f
Shin, T. H., Lee, D. Y., Lee, H. S., Park, H. J., Jin, M. S., Paik, M. J., et al. (2018). Integration of metabolomics and transcriptomics in nanotoxicity studies. BMB Rep. 51 (1), 14–20. doi:10.5483/bmbrep.2018.51.1.237
Shukla, S., Jadaun, A., Arora, V., Sinha, R. K., Biyani, N., and Jain, V. (2015). In vitro toxicity assessment of chitosan oligosaccharide coated iron oxide nanoparticles. Toxicol. Rep. 2, 27–39. doi:10.1016/j.toxrep.2014.11.002
Simon-Deckers, A., Loo, S., Mayne-L’hermite, M., Herlin-Boime, N., Menguy, N., Reynaud, C., et al. (2009). Size-composition- and shape-dependent toxicological impact of metal oxide nanoparticles and carbon nanotubes toward bacteria. Environ. Sci. Technol. 43 (21), 8423–8429. doi:10.1021/es9016975
Sinclair, T. R., van den Hengel, S. K., Raza, B. G., Rutjes, S. A., de Roda Husman, A. M., Peijnenburg, W. J. G. M., et al. (2021). Surface chemistry-dependent antiviral activity of silver nanoparticles. Nanotechnology 32 (36), 365101. doi:10.1088/1361-6528/ac03d6
Singh, P., Pandit, S., Mokkapati, V., Garg, A., Ravikumar, V., and Mijakovic, I. (2018). Gold nanoparticles in diagnostics and therapeutics for human cancer. Int. J. Mol. Sci. 19 (7), 1979. doi:10.3390/ijms19071979
Suk, J. S., Xu, Q., Kim, N., Hanes, J., and Ensign, L. M. (2016). PEGylation as a strategy for improving nanoparticle-based drug and gene delivery. Adv. Drug Deliv. Rev. 99, 28–51. doi:10.1016/j.addr.2015.09.012
Sukhanova, A., Bozrova, S., Sokolov, P., Berestovoy, M., Karaulov, A., and Nabiev, I. (2018). Dependence of nanoparticle toxicity on their physical and chemical properties. Nanoscale Res. Lett. 13 (1), 44. doi:10.1186/s11671-018-2457-x
Tefft, B. J., Uthamaraj, S., Harburn, J. J., Klabusay, M., Dragomir-Daescu, D., and Sandhu, G. S. (2015). Cell labeling and targeting with superparamagnetic iron oxide nanoparticles. J. Vis. Exp. (105), e53099. doi:10.3791/53099
Toloman, D., Pana, O., Stefan, M., Popa, A., Leostean, C., Macavei, S., et al. (2019). Photocatalytic activity of SnO2-TiO2 composite nanoparticles modified with PVP. J. Colloid Interface Sci. 542, 296–307. doi:10.1016/j.jcis.2019.02.026
Tomak, A., Cesmeli, S., Hanoglu, B. D., Winkler, D., and Oksel Karakus, C. (2021). Nanoparticle-protein corona complex: Understanding multiple interactions between environmental factors, corona formation, and biological activity. Nanotoxicology 15 (10), 1331–1357. doi:10.1080/17435390.2022.2025467
Van Assche, R., Broeckx, V., Boonen, K., Maes, E., De Haes, W., Schoofs, L., et al. (2015). Integrating -omics: Systems biology as explored through C. elegans research. J. Mol. Biol. 427 (21), 3441–3451. doi:10.1016/j.jmb.2015.03.015
Vandebriel, R. J., Vermeulen, J. P., van Engelen, L. B., de Jong, B., Verhagen, L. M., de la Fonteyne-Blankestijn, L. J., et al. (2018). The crystal structure of titanium dioxide nanoparticles influences immune activity in vitro and in vivo. Part. Fibre Toxicol. 15 (1), 9. doi:10.1186/s12989-018-0245-5
Voinov, M. A., Pagan, J. O. S., Morrison, E., Smirnova, T. I., and Smirnov, A. I. (2011). Surface-mediated production of hydroxyl radicals as a mechanism of iron oxide nanoparticle biotoxicity. J. Am. Chem. Soc. 133 (1), 35–41. doi:10.1021/ja104683w
Wang, D., Lin, Z., Wang, T., Yao, Z., Qin, M., Zheng, S., et al. (2016). Where does the toxicity of metal oxide nanoparticles come from: The nanoparticles, the ions, or a combination of both? J. Hazard. Mat. 308, 328–334. doi:10.1016/j.jhazmat.2016.01.066
Wang, E., Huang, Y., Du, Q., and Sun, Y. (2017). Silver nanoparticle induced toxicity to human sperm by increasing ROS(reactive oxygen species) production and DNA damage. Environ. Toxicol. Pharmacol. 52, 193–199. doi:10.1016/j.etap.2017.04.010
Wang, J., Deng, X., Zhang, F., Chen, D., and Ding, W. (2014). ZnO nanoparticle-induced oxidative stress triggers apoptosis by activating JNK signaling pathway in cultured primary astrocytes. Nanoscale Res. Lett. 9 (1), 117. doi:10.1186/1556-276x-9-117
Wang, J., Wang, J., Liu, Y., Nie, Y., Si, B., Wang, T., et al. (2019). Aging-independent and size-dependent genotoxic response induced by titanium dioxide nanoparticles in mammalian cells. J. Environ. Sci. 85, 94–106. doi:10.1016/j.jes.2019.04.024
Wang, L., Ai, W., Zhai, Y., Li, H., Zhou, K., and Chen, H. (2015). Effects of nano-CeO(2) with different nanocrystal morphologies on cytotoxicity in HepG2 cells. Int. J. Environ. Res. Public Health 12 (9), 10806–10819. doi:10.3390/ijerph120910806
Wang, X., Xia, T., Addo Ntim, S., Ji, Z., Lin, S., Meng, H., et al. (2011). Dispersal state of multiwalled carbon nanotubes elicits profibrogenic cellular responses that correlate with fibrogenesis biomarkers and fibrosis in the murine lung. ACS Nano 5 (12), 9772–9787. doi:10.1021/nn2033055
Wilson, C. L., Natarajan, V., Hayward, S. L., Khalimonchuk, O., and Kidambi, S. (2015). Mitochondrial dysfunction and loss of glutamate uptake in primary astrocytes exposed to titanium dioxide nanoparticles. Nanoscale 7 (44), 18477–18488. doi:10.1039/c5nr03646a
Wilson, M. R., Lightbody, J. H., Donaldson, K., Sales, J., and Stone, V. (2002). Interactions between ultrafine particles and transition metals in vivo and in vitro. Toxicol. Appl. Pharmacol. 184 (3), 172–179. doi:10.1006/taap.2002.9501
Wu, T., and Tang, M. (2018). Review of the effects of manufactured nanoparticles on mammalian target organs. J. Appl. Toxicol. 38 (1), 25–40. doi:10.1002/jat.3499
Wu, W., He, Q., and Jiang, C. (2008). Magnetic iron oxide nanoparticles: Synthesis and surface functionalization strategies. Nanoscale Res. Lett. 3 (11), 397–415. doi:10.1007/s11671-008-9174-9
Xia, Q., Zhang, Y., Li, Z., Hou, X., and Feng, N. (2019). Red blood cell membrane-camouflaged nanoparticles: A novel drug delivery system for antitumor application. Acta Pharm. Sin. B 9 (4), 675–689. doi:10.1016/j.apsb.2019.01.011
Xu, L., Li, X., Takemura, T., Hanagata, N., Wu, G., and Chou, L. (2012). Genotoxicity and molecular response of silver nanoparticle (NP)-based hydrogel. J. Nanobiotechnology 10, 16. doi:10.1186/1477-3155-10-16
Xu, M., Halimu, G., Zhang, Q., Song, Y., Fu, X., Li, Y., et al. (2019). Internalization and toxicity: A preliminary study of effects of nanoplastic particles on human lung epithelial cell. Sci. Total Environ. 694, 133794. doi:10.1016/j.scitotenv.2019.133794
Yang, H., Liu, C., Yang, D., Zhang, H., and Xi, Z. (2009). Comparative study of cytotoxicity, oxidative stress and genotoxicity induced by four typical nanomaterials: The role of particle size, shape and composition. J. Appl. Toxicol. 29 (1), 69–78. doi:10.1002/jat.1385
Yang, W., Wang, L., Mettenbrink, E. M., DeAngelis, P. L., and Wilhelm, S. (2021). Nanoparticle toxicology. Annu. Rev. Pharmacol. Toxicol. 61, 269–289. doi:10.1146/annurev-pharmtox-032320-110338
Yang, Y., Fan, S., Chen, Q., Lu, Y., Zhu, Y., Chen, X., et al. (2022). Acute exposure to gold nanoparticles aggravates lipopolysaccharide-induced liver injury by amplifying apoptosis via ROS-mediated macrophage-hepatocyte crosstalk. J. Nanobiotechnology 20 (1), 37. doi:10.1186/s12951-021-01203-w
Yin, S., Li, Z., Cheng, L., Wang, C., Liu, Y., Chen, Q., et al. (2013). Magnetic PEGylated Pt3Co nanoparticles as a novel MR contrast agent: In vivo MR imaging and long-term toxicity study. Nanoscale 5 (24), 12464–12473. doi:10.1039/c3nr04212j
Yokel, R. A., Au, T. C., MacPhail, R., Hardas, S. S., Butterfield, D. A., Sultana, R., et al. (2012). Distribution, elimination, and biopersistence to 90 days of a systemically introduced 30 nm ceria-engineered nanomaterial in rats. Toxicol. Sci. 127 (1), 256–268. doi:10.1093/toxsci/kfs067
Yu, Q., Xiong, X. q., Zhao, L., Xu, T. t., Bi, H., Fu, R., et al. (2018). Biodistribution and toxicity assessment of superparamagnetic iron oxide nanoparticles in vitro and in vivo. Curr. Med. Sci. 38 (6), 1096–1102. doi:10.1007/s11596-018-1989-8
Yu, S., Liu, F., Wang, C., Zhang, J., Zhu, A., Zou, L., et al. (2018). Role of oxidative stress in liver toxicity induced by nickel oxide nanoparticles in rats. Mol. Med. Rep. 17 (2), 3133–3139. doi:10.3892/mmr.2017.8226
Yu, Y., Li, Y., Wang, W., Jin, M., Du, Z., Li, Y., et al. (2013). Acute toxicity of amorphous silica nanoparticles in intravenously exposed ICR mice. PLoS One 8 (4), e61346. doi:10.1371/journal.pone.0061346
Zakharova, L. Y., Pashirova, T. N., Doktorovova, S., Fernandes, A. R., Sanchez-Lopez, E., Silva, A. M., et al. (2019). Cationic surfactants: Self-assembly, structure-activity correlation and their biological applications. Int. J. Mol. Sci. 20 (22), 5534. doi:10.3390/ijms20225534
Zare, E. N., Zheng, X., Makvandi, P., Gheybi, H., Sartorius, R., Yiu, C. K. Y., et al. (2021). Nonspherical metal-based nanoarchitectures: Synthesis and impact of size, shape, and composition on their biological activity. Small 17 (17), e2007073. doi:10.1002/smll.202007073
Zhang, H., Gilbert, B., Huang, F., and Banfield, J. F. (2003). Water-driven structure transformation in nanoparticles at room temperature. Nature 424 (6952), 1025–1029. doi:10.1038/nature01845
Zhang, L., Mazouzi, Y., Salmain, M., Liedberg, B., and Boujday, S. (2020). Antibody-gold nanoparticle bioconjugates for biosensors: Synthesis, characterization and selected applications. Biosens. Bioelectron. X. 165, 112370. doi:10.1016/j.bios.2020.112370
Zhang, S., Chu, Q., Zhang, Z., Xu, Y., Mao, X., and Zhang, M. (2021). Responses of Caenorhabditis elegans to various surface modifications of alumina nanoparticles. Environ. Pollut. 271, 116335. doi:10.1016/j.envpol.2020.116335
Zhang, S., Gao, H., and Bao, G. (2015). Physical principles of nanoparticle cellular endocytosis. ACS Nano 9 (9), 8655–8671. doi:10.1021/acsnano.5b03184
Zhang, W., Yao, Y., Sullivan, N., and Chen, Y. (2011). Modeling the primary size effects of citrate-coated silver nanoparticles on their ion release kinetics. Environ. Sci. Technol. 45 (10), 4422–4428. doi:10.1021/es104205a
Zhernenkov, M., Ashkar, R., Feng, H., Akintewe, O. O., Gallant, N. D., Toomey, R., et al. (2015). Thermoresponsive PNIPAM coatings on nanostructured gratings for cell alignment and release. ACS Appl. Mat. Interfaces 7 (22), 11857–11862. doi:10.1021/acsami.5b01453
Zhou, Q., Pan, J., Deng, S., Xia, F., and Kim, T. (2021). Triboelectric nanogenerator-based sensor systems for chemical or biological detection. Adv. Mat. 33 (35), e2008276. doi:10.1002/adma.202008276
Zhu, N., Ji, H., Yu, P., Niu, J., Farooq, M., Akram, M., et al. (2018). Surface modification of magnetic iron oxide nanoparticles. Nanomater. (Basel) 8 (10), 810. doi:10.3390/nano8100810
Zoroddu, M. A., Medici, S., Ledda, A., Nurchi, V., Lachowicz, J., and Peana, M. (2014). Toxicity of nanoparticles. Curr. Med. Chem. 21 (33), 3837–3853. doi:10.2174/0929867321666140601162314
Keywords: metal-based nanoparticle, physicochemical property, nanotoxicity, mechanism, assessment, mitigation, regulatory movement
Citation: Zhang N, Xiong G and Liu Z (2022) Toxicity of metal-based nanoparticles: Challenges in the nano era. Front. Bioeng. Biotechnol. 10:1001572. doi: 10.3389/fbioe.2022.1001572
Received: 23 July 2022; Accepted: 25 October 2022;
Published: 10 November 2022.
Edited by:
Segaran P. Pillai, United States Department of Health and Human Services, United StatesReviewed by:
Xiaojia He, Emory University, United StatesCopyright © 2022 Zhang, Xiong and Liu. This is an open-access article distributed under the terms of the Creative Commons Attribution License (CC BY). The use, distribution or reproduction in other forums is permitted, provided the original author(s) and the copyright owner(s) are credited and that the original publication in this journal is cited, in accordance with accepted academic practice. No use, distribution or reproduction is permitted which does not comply with these terms.
*Correspondence: Zhenjie Liu, bGF3c29uNDAwMUB6anUuZWR1LmNu
†These authors have contributed equally to this work and share first authorship
Disclaimer: All claims expressed in this article are solely those of the authors and do not necessarily represent those of their affiliated organizations, or those of the publisher, the editors and the reviewers. Any product that may be evaluated in this article or claim that may be made by its manufacturer is not guaranteed or endorsed by the publisher.
Research integrity at Frontiers
Learn more about the work of our research integrity team to safeguard the quality of each article we publish.