- State Key Laboratory of Pathogens and Biosecurity, Institute of Biotechnology, Academy of Military Medical Sciences, Beijng, China
A miniature CRISPR-Cas12f has been demonstrated to serve as an effective genome editing tool in gram negative bacteria as well as human cells. Here, we developed an alternative method to edit the genome of Bacillus anthracis based on the AsCas12f1 nuclease from Acidibacillus sulfuroxidans. When the htrA gene on the chromosome and the lef gene on the plasmid pXO1 were selected as targets, the CRISPR-AsCas12f1 system showed very high efficiency (100%). At the same time, a high efficiency was observed for large-fragment deletion. Our results also indicated that the length of the homologous arms of the donor DNA had a close relationship with the editing efficiency. Furthermore, a two-plasmid CRISPR-AsCas12f1 system was also constructed and combined with the endonuclease I-SceI for potential multi-gene modification. This represents a novel tool for mutant strain construction and gene function analyses in B. anthracis and other Bacillus cereus group bacteria.
Introduction
Clustered regularly interspaced short palindromic repeats (CRISPRs) are part of the bacterial immune system that defends against invading viruses (Westra et al., 2014). They are made up of repeating sequences of genetic code that are interrupted by pieces of genetic code from previous invaders, which allows a cell to detect and destroy returning invaders. Based on this characteristic, CRISPR-associated (Cas) nucleases have been extensively used in genome editing in many species and an increasing number of newly discovered Cas nucleases have been explored as novel genome editing tools (Jiang and Marraffini, 2015; Manghwar et al., 2019). To date, the miniature CRISPR effectors, such as CasΦ, Cas13, and Cas14, have been developed into functional genome editors (Aquino-Jarquin, 2019; Savage, 2019; Pausch et al., 2020; Awan et al., 2021). Of these Cas nucleases, Cas12f has been shown to be superior as an editing system due to its small molecular weight and ease of cellular delivery (Awan et al., 2021).
Cas12f (also known as Cas14) is a family of relatively compact RNA-guided nucleases that were originally found in an uncultivated archaea species. It belongs to the class 2 type V-F CRISPR-associated effector nuclease family. Most Cas12f proteins are approximately 400–700 amino acids in length, include a single RuvC nuclease domain, and are known as miniature Cas proteins (Takeda et al., 2021; Xiao et al., 2021). Cas12f was discovered by mining a database of microbial genomes and metagenomes, and Cas12f was shown to bind and cleave a targeted ssDNA sequence using a specific gRNA sequence. However in this work, they did not determine if Cas12f was able to target dsDNA both in vivo and in vitro (Harrington et al., 2018). Next, Karvelis et al. confirmed that Cas12f nucleases recognize and cleave dsDNA in a TTTR (where R is A or G) PAM-dependent manner and have the potential to be harnessed as programmable nucleases for genome editing (Karvelis et al., 2020). Currently, Cas12f has been demonstrated to serve as an effective genome editing tool in bacteria as well as human cells. Okano et al. showed that the Un1Cas12f1 (529 aa) from an uncultured archaeon (Un1) could modify the Escherichia coli genome with high efficiency (50–100%) (Okano et al., 2021). Kim et al. showed that an optimized CRISPR/Un1Cas12f1 system enabled efficient and specific genome editing in human cells, with efficiency and specificity similar to that of SpCas9 and AsCas12a (Kim et al., 2021). Wu et al. also showed that AsCas12f1 from Acidibacillus sulfuroxidans could serve as an effective genome editing tool in both bacteria and human cells. Moreover, this AsCas12f1 system could be delivered by various delivery methods, including plasmid, ribonucleoprotein and adeno-associated virus (Wu et al., 2021). At the same time, Xu et al. developed a miniature CRISPR system with Cas12f mutants, named CasMINI, which enabled robust gene editing and base editing in mammalian cells (Xu et al., 2021). Bigelyte et al. showed that SpCas12f1 functioned in both plant and human cells to produce targeted modifications (Bigelyte et al., 2021). All of these works provide sufficient evidence that Cas12f can be developed a useful tool for broad genome engineering applications.
In a previous study, we developed a genome-editing protocol for B. cereus group strains based on the CRISPR-Cas9 system (Wang et al., 2019). Both large fragment deletion and precise point mutations could be achieved efficiently. Unfortunately, the large size of SpCas9 (1368 amino acids) is a nonnegligible problem, and this increases the size of any genome editing plasmid using SpCas9. Like many bacteria, the transformation efficiency of B. anthracis decreases with the increasing of plasmid size, and this also is a significant problem in many poorly transformable strains (Ohse et al., 1995; Turgeon et al., 2006). Alternative, miniature Cas nucleases would be a better choice in B. anthracis and other similar strains.
In the present study, the feasibility of CRISPR-Cas12f in B. anthracis was determined. When the htrA gene on the B. anthracis chromosome and the lef gene on the plasmid pXO1 were selected as targets, 100% modification rates were achieved in these experiments. Then the effect of homologous arm length on editing efficiency also was explored by comparative analysis of the results using plasmids with different lengths of homologous arms. At the same time a two-plasmid CRISPR-AsCas12f1 system was also constructed and combined with endonuclease I-SceI for potential multi-gene modification.
Taken together, an efficient genome editing protocol for B. anthracis was developed based on the CRISPR-AsCas12f1 system. This protocol will be a useful tool for mutant strain construction and gene function analyses in B. anthracis.
Materials and Methods
Bacterial Strains, Plasmids, and Growth Conditions
All bacterial strains and plasmids used in this study are listed in Table 1. Escherichia coli Top 10 cells were used as a cloning host, and E. coli SCS110 was used to prepare unmethylated plasmids. E. coli was grown aerobically in LB medium at 37°C, while B. anthracis was grown in BHIG medium (brain heart infusion broth with the addition of 0.5% glycerol). Kanamycin (50 μg/ml for E. coli, 30 μg/ml for B. anthracis) or erythromycin (400 μg/ml for E. coli, 5 μg/ml for B. anthracis) was added to the media at appropriate final concentrations as needed.
Editing Plasmid Construction
All constructed plasmids used in this work are shown in Table 1, and PCR primers and the N20 sequences for each PAM sequence (TTTG) are listed in Table 2. All the sequence information of synthetic DNA fragments is supplied in supplementary information (Supplementary Table S1). B. anthracis-codon-optimized AsCas12f1 from A. sulfuroxidans with the B. subtilis mannose manP promoter (PmanP) and the sgRNA_V1 fragment designed according to a reference were ordered from General Biosystems (China). To introduce the CRISPR-AsCas12f1 system into a plasmid, the Cas9 cassette of plasmid pJOE8999 was replaced by this synthetic AsCas12f1 cassette, and the EcoRI-XbaI fragment of the resulting plasmid was replaced with the synthetic sgRNA sgRNA_V1 fragment. This plasmid was designated pJOE-Cas12f1.
To explore the feasibility of CRISPR-Cas12f system in B. anthracis, the htrA gene on the B. anthracis chromosome and the lef gene on the plasmid pXO1 were selected as targets. The 800-bp upstream and downstream regions of these target genes were amplified using B. anthracis A16R genomic DNA as a template and inserting it into the corresponding SalI and XbaI sites of pJOE-Cas12f1. For the htrA gene, primers UhtrAF/UhtrAR and DhtrAF/DhtrAR were used, respectively. Then the small double stranded target spacer, annealed with the two complementary oligonucleotides (sg-htrAF1/sg-htrAR1or sghtrAF2/sg-htrAR2), was inserted in the location between the two BsaI sites of the plasmid to obtain gene specific genome editing plasmids. In this work, two sgRNAs with different target spacers on each gene were designed and tested respectively in subsequent experiments. In the same manner, plasmids for lef gene deletion were constructed accordingly.
To test the effect of the length of homologous arms on the editing efficiency, a series of htrA specific genome editing plasmids with varying lengths (50, 100, 200, or 400 bp) of upstream and downstream regions were constructed using a similar method and also were used to delete the htrA gene.
To explore the feasibility of large chromosomal fragment deletions using the CRISPR-Cas12f system in B. anthracis, the prophage lambdaBa03 (∼16.8 kb) was selected as the target. The plasmids for lambdaBa03 deletion were constructed accordingly in the similar way. The upstream and downstream regions of lambdaBa03 were amplified using plasmid pJOE-lam03 constructed previously as template (Wang et al., 2019). In this part, two different lengths (400 bp and 800 pb) upstream and downstream regions were designed and tested the editing efficiency.
Genome Editing With a Single Plasmid
The transformation and selection of competent cells was performed as described previously (Wang et al., 2019). pJOE-cas12f1 series plasmids were introduced by electroporation of B. anthracis A16R, and transformants were selected at 30°C on BHIG medium containing kanamycin. Single colonies were transferred to liquid media (with 25 μg/ml kanamycin) and incubated with shaking for 3 h at 37°C. Mannose (final concentration, 0.4%) was added to induce the expression of the Cas12f1 protein. After 3 h of cultivation, serial dilutions of this culture were plated on LB agar with 25 μg/ml kanamycin and 0.4% mannose and then incubated at 37°C overnight. Transformants were identified by colony PCR and DNA sequencing. For PCR tests, the B. anthracis A16R strain was used as a negative control.
Inducible Promoter Screening
All alternative promoter fragments and mScarlet coding sequence were designed according to reference or sequence information from the NCBI database and ordered from GeneralBio Co. (China). To construct the promoter screening plasmid, the plasmid pJOE8999 was digested with BsrGI and EcoRI to release the backbone of the plasmid (from 1 to 2844 bp). Then the synthetic coding region of the mScarlet coding sequence with applicable restriction sites was ligated with this fragment to construct plasmid pJOE-mScarlet (Bindels et al., 2017). Next, alternative promoters, the bacitracin-inducible promoter (Toymentseva et al., 2012), cumate-inducible promoter (Seo and Schmidt-Dannert, 2019), or xylose-inducible promoter from Bacillus megaterium and Bacillus subtilis, were inserted upstream of the mScarlet coding sequence and the resulting plasmids were introduced into the B. anthracis A16R strain and selected at 30°C on BHIG medium containing kanamycin.
For comparison of the mScarlet expression levels of B. anthracis A16R harboring different recombinant plasmid, 5 ml of LB cultures were grown to an OD600 nm = 0.6–1.0, then the suitable inducers were added to induce target protein expression. Cultures without inducers were used as a negative control. After 10 hours of induction, the OD600 nm and fluorescence intensity of cultures (excitation at 569 nm and emission at 594 nm) were measured with a SpectraMax®i3x.
Two-Plasmid System Editing Plasmid Construction
To cure CRISPR plasmids as soon as possible, a two-plasmid system based on CRISPR-Cas12f was also constructed. At first, the I-SceI cassette (containing the xylose induced promoter and the coding region of I-SceI) was inserted at a location between the XbaI and EcoRI sites in plasmid pJOE-Cas12f1 by Gibson assembly. The resulting plasmid was digested with XhoI and PstI to remove the kanamycin resistance cassette, then the erythromycin resistance cassette amplified from pHY304 was cloned into this plasmid to replace the kanamycin resistance cassette. The resulting plasmid was designated pCas12f1-SceI-E.
At the same time, the I-SceI coding region of the modified plasmid pSS4332 (a point mutation was introduced to destroy the BsaI site in repB of this plasmid) was replaced by a synthetic DNA fragment, including the sgRNA_V1 cassette, restriction sites for homologous arm insertion, and two I-SceI sites on both sides, to construct plasmid pSS-FD. Then the homologous arms and the target spacer of the htrA gene were inserted into the plasmid pSS-FD successively using the previously described method. The resulting plasmid was designated pSS-FD-htrA and used for genome editing.
Genome Editing With Two Plasmids
For the two-plasmid system, B. anthracis A16R competent cells harboring pCas12f1-SceI plasmid were prepared using previously described methods, and then the pSS-FD-htrA plasmid was introduced by electroporation. Genome editing was triggered by inducing AsCas12f1 expression. Briefly, a strain harboring pCas12f1-SceI and pSS-FD-thrA was transferred to liquid media (with 25 μg/ml kanamycin and 5 μg/ml erythromycin), and incubated with shaking for 3 h at 37°C. Mannose (final concentration, 0.4%) was added to induce the expression of the Cas12f1 protein. After cultivation for 3 h, serial dilutions of this culture were plated on LB agar with erythromycin and 0.4% mannose and cells were incubated at 37°C overnight. Transformants were identified by colony PCR and the B. anthracis A16R strain was used as a negative control.
Plasmid Curing
To cure the pSS-FD-htrA plasmid, an edited colony harboring both pCas12F and pSS-FD-htrA was transferred to liquid BHIG media (with 5 μg/ml erythromycin), and incubated with shaking for 3 h at 37°C. Xylose (final concentration, 0.4%, w/v) was added to induce the expression of the endonuclease I-SceI and the culture without xylose was used as negative control. After cultivation for 3 h, the culture was diluted and spread onto BHIG plates with 5 μg/ml erythromycin and 0.4% xylose, and these plates were incubated at 37°C for 16–20 h. The curing of plasmid of pSS-FD-htrA was confirmed by determining the colony sensitivity to kanamycin (25 μg/ml).
Results
Establishment and Improvement of the CRISPR-AsCas12f1 Genome Editing System
For demonstration of the feasibility of the CRISPR-Cas12f system in B. anthracis, the plasmid pJOE-AsCas12f1 was constructed and employed for genome editing (Figure 1A). First, as described in Materials and methods section, the plasmid including donor DNA, gene-specific sgRNA, and AsCas12f1 cassettes was electroporated into B. anthracis A16R. Then the genome editing events were performed as described previously (Wang et al., 2019). The htrA gene on the B. anthracis chromosome and the lef gene on the plasmid pXO1 were selected as targets, and alternative mutants were detected by colony PCR. For the htrA gene, compared to the 2.3 kb amplicon from the parental strain A16R, the 1.0 kb PCR products from all randomly selected colonies (9/9 and 9/9 for each target spacer) showed that htrA (1242 bp) had been deleted successfully (Figure 1B). A similar result was also seen for lef deletion (Figure 1C). These results confirmed the predominant feasibility of AsCas12f1 for B. anthracis genome editing.
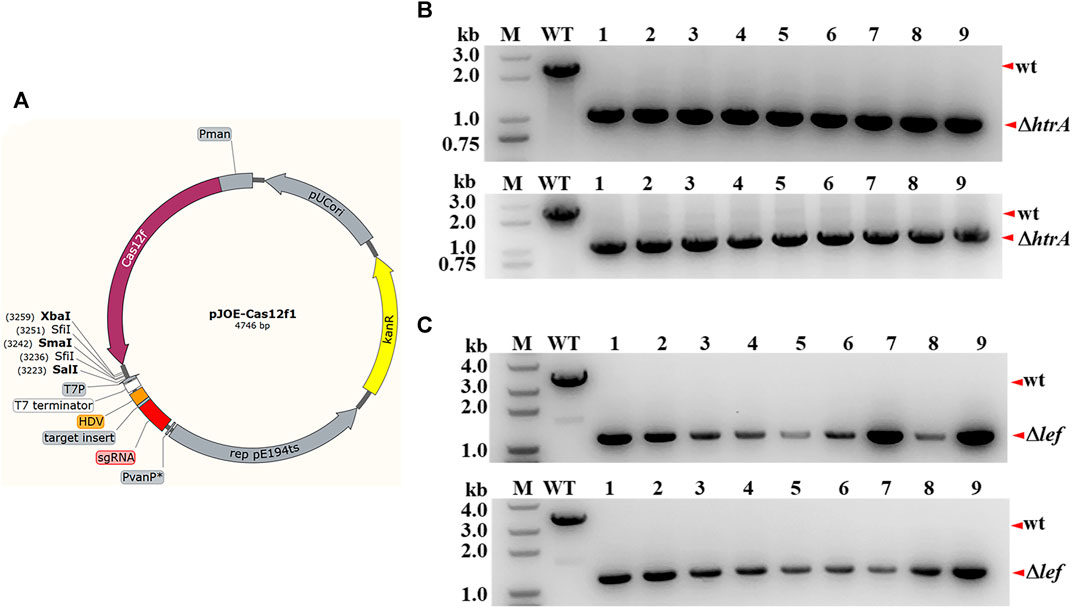
FIGURE 1. Gene deletion via the CRISPR-CRISPR-AsCas12f1 system in B. anthracis. (A) Physical map of plasmid pJOE-cas12f1. Pman, PmanP promoter; PvanP*, semisynthetic promoter PvanP*. The cloning sites and insertion site of the spacer sequence (BsaI restriction sites) are also labeled in the map. (B) PCR verification of htrA deletion in B. anthracis A16R. The htrA gene locus of the randomly selected colonies carrying the editing plasmids was amplified, and the PCR products were verified through agarose gel analysis. M, DNA marker; WT, control with B.anthracis A16R total DNA as the template; “wt” and “ΔhtrA”, wild-type band and the htrA-deleted band, respectively. The correct fragment in the mutant strain was approximately 1.0 kb (lanes 1–9) while in A16R this was 2.3 kb (lane WT). (C) PCR verification of lef deletion in B. anthracis A16R. “wt” and “Δlef”, wild-type band and the lef-deleted band, respectively. The correct fragment in the mutant strain was approximately 1.2 kb (lanes 1–9) while in A16R this was 3.6 kb (lane WT).
To study the relationship between the editing efficiency and the length of homologous arms, four other editing plasmids with different lengths of homologous arms (50, 100, 200, and 400 bp) were constructed. The results of htrA gene deletion indicated that the length of the homologous arms was closely related to the editing efficiency (Figure 2A). PCR tests indicated that when the lengths of the homologous arms were 50 bp or 100 bp, none of the 30 randomly selection colonies showed htrA gene deletion bands and only wild type amplification bands (Figures 2B,C). When the length of the homologous arms was 200 bp, only 2 of the 15 randomly selection colonies exhibited the htrA gene deletion band while most colonies (13/15) were heterozygous (two bands amplification, Figure 2D). When the length of the homologous arms was 400 or 800 bp, 100% of randomly selection colonies (15/15 and 15/15, Figures 2E,F) showed that htrA had been completely deleted (pure deletion type).
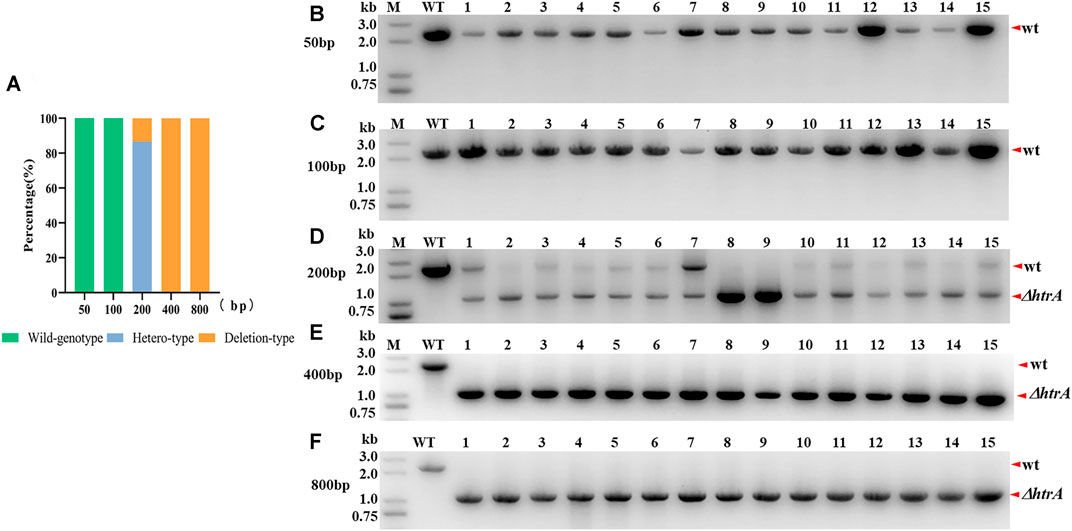
FIGURE 2. Effect of homologous arm length on gene deletion efficiency. (A) Comparison analysis of the genome editing efficiencies of plasmids with different length homologous arms. Hetero-type colonies showed bands for both wild type and htrA gene deletion. (B–F) PCR validation of htrA gene deletion in B. anthracis A16R using editing plasmids with different length homologous arms (50, 100, 200, 400, and 800 bp). M, DNA marker; WT, B. anthracis A16R total DNA as a template; wt, wild-type band; and ΔhtrA, htrA-deleted band. For each plasmid group, 15 colonies were selected to validate by PCR and agarose gel analysis.
To test the availability of the CRISPR-cas12f system for longer DNA fragment deletion, the prophage lambdaBa03 was excised by similar method. 9 randomly selection colonies were screened via PCR. The region amplified by the lamiF/lamiR primers in the control strain A16R was > 19 kb in length, which exceeded the maximum amplification size under our PCR conditions. However, When the length of the homologous arms was 800 bp, all randomly selected colonies (9/9) showed the expected 2.2 kb amplicon, indicating that the prophage lambdaBa03 had been excised successfully (Figure 3A). At the same time, despite the substantially reduced size of the homologous arms, the 100% editing efficiency (9/9) was also seen when the 400 bp homologous arms were employed (Figure 3B).
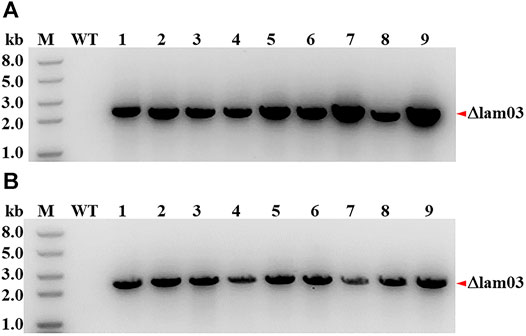
FIGURE 3. Large-fragment deletion via the CRISPR-CRISPR-AsCas12f1 system in B. anthracis. PCR verification of lambdaBa03 excision in B. anthracis A16R using editing plasmids with 800 bp (A) and 400 bp (B) homologous arms. The correct fragment amplified by the lamiF/lamiR primers in the lambdaBa03 excised strain was approximately 2.2 kb (lanes 1–9), while the region in the control strain A16R (>19 kb) exceeded the maximum amplification size under same PCR conditions. M, DNA marker; WT, control with B.anthracis A16R total DNA as the template; “Δlam03”, the lambdaBa03 excised band.
Two-plasmid-based CRISPR-Cas12f System and Plasmid Curing
To strictly induce the expression of the endonuclease I-SceI, four different promoters were selected to drive the expression of the report protein mScarlet. The results demonstrated that the PxylA promoter from B. subtilis could be strictly induced and the expression level of the report gene was relatively high (Figure 4A). Based on this result, a plasmid co-expressing AsCas12f1 and the endonuclease I-SceI under the control of the PxylA promoter was constructed as show in Figure 4B. Combined with another plasmid pSS-FD carrying the sgRNA cassette, this two-plasmid-based CRISPR-Cas12f system for B. anthracis was successfully established.
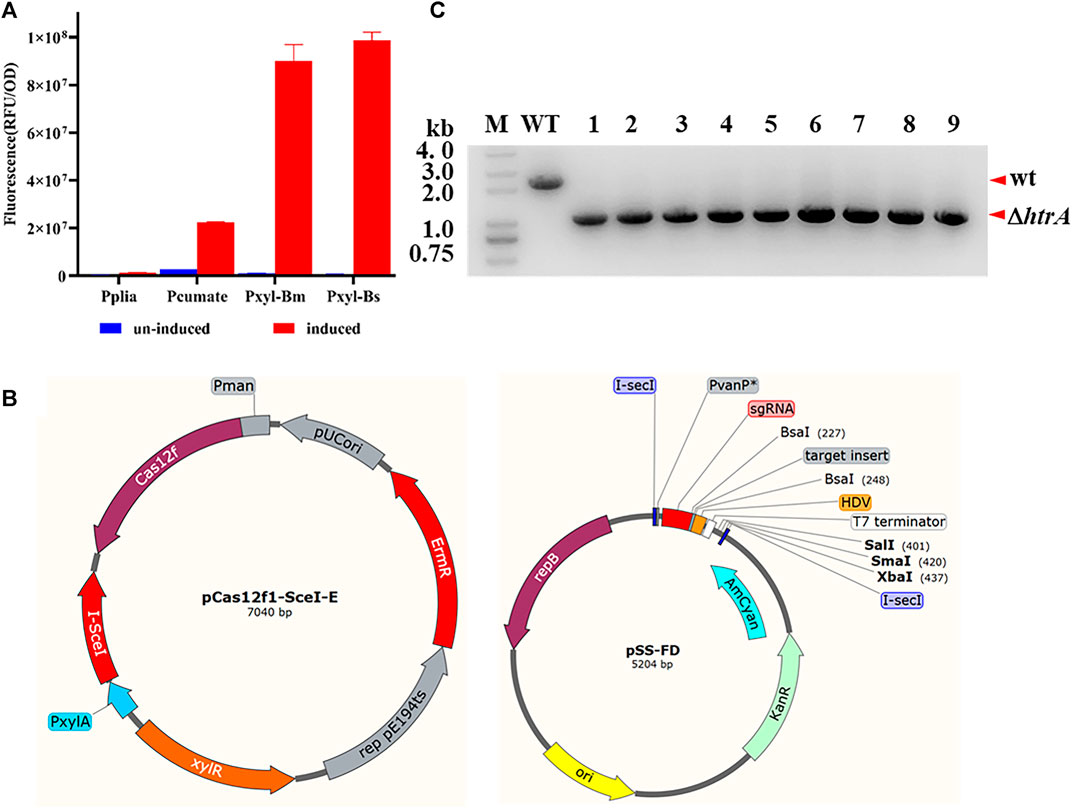
FIGURE 4. Gene deletion via a two-plasmid CRISPR-CRISPR-AsCas12f1 system in B. anthracis. (A) Induced promoter screening using mScarlet as a reporter molecule. (B) Physical map of the two-plasmid system vectors. pCas12f1-SceI-E, the plasmid co-expressed AsCas12f1 and I-SceI endonuclease I-SceI (under the control of the PxylA promoter); pSS-FD, for sgRNA expression and homologous arm supply. (C) PCR verification of htrA deletion via the two-plasmid system. M, DNA marker; WT, B. anthracis A16R total DNA as a template; wt, wild-type band; and ΔhtrA, htrA-deleted band. The correct fragment in the mutant strain was approximately 1.0 kb (lanes 1–9) while in A16R this was 2.3 kb (lane WT).
To demonstrate the feasibility of this two-plasmid system, the htrA gene was selected as a target once again and the editing efficiency was tested. As shown in Figure 4C, all nine randomly selected colonies (9/9) showed that htrA (1242 bp) had been deleted in B. anthracis A16R. At the same time, the result of plasmid curing tests indicated that a portion of the recombinant colonies (12/50, 6/50 and 17/50, three independent experiments) had lost kanamycin resistance and the plasmid pSS-FD-htrA had been partially eliminated after endonuclease I-SceI expression was induced by xylose. While no kanamycin sensitive colony was gained in the non-inducible conditions after passage once.
Discussion
In this work, the CRISPR-Cas12f system was successfully utilized for genome editing in B. anthracis. The efficiency was similar to the CRISPR-SpCas9 system based on our previously reported results (Wang et al., 2019). Either the htrA gene on the B. anthracis chromosome or the lef gene on the plasmid pXO1 was deleted, and deletion mutants achieved rates of 100% after one round of induction and selection in these experiments.
At the same time, a high efficiency was seen for large-fragment deletion when prophage lambdaBa03 was selected as target. These results indicated that the CRISPR-12f system was a high-efficiency genetic operation tool in B. anthracis. Moreover, the protocol developed in this work may be generally applicable to other bacillus group strains.
Compared to the CRISPR-Cas9 system, the CRISPR-Cas12f system had a clear comparative advantage. The AsCas12f1 used in our work consisted of 422 amino acid residues, as this was one of the high-activity miniature CRISPR–Cas effectors (Kim et al., 2021; Wu et al., 2021). This characterization work demonstrated that the size of the genome editing plasmid was smaller and higher transformation efficiency was obtained in B. anthracis relevant experiments using this effector. At the same time, based on published results, the most efficient PAMs recognized by AsCas12f1 were 5′-TTTR (where R represents A or G) (Karvelis et al., 2020). For B. anthrcis, a low-GC-content Gram Positive Bacteria, the distribution of PAMs sequence was thus more general and the spacer screening became easier for many genes. This means that our CRISPR-Cas12f system would be more useful in B. anthracis and other low-GC-content bacteria compared to a more traditional SpCas9-based system.
When a one-plasmid-based CRISPR-Cas system was used for two or more gene edits in same parent strain, the rate of editing plasmid curing was shown to be the major constraint and more attention must be paid to overcome this (Wu et al., 2019). According to published studies and our results, some recombinant plasmids, despite including a temperature sensitive origin of replication, are very difficult to cure at the non-permissive temperature in B. anthracis and some other bacillus species (Hartz et al., 2021). When the plasmids derived from pJOE8999 were used to edit the B. anthracis genome, 8–10 passages in antibiotic free medium at the non-permissive temperature were frequently needed to derive transformants without editing plasmids. The period of these experiments were thus lengthened (unpublished data). To solve this issue, separate Cas9 and sgRNA on different plasmids using a two-plasmid system has been a popular method (Wasels et al., 2017; Ao et al., 2018). Here, we also constructed a two-plasmid CRISPR-Cas12f system combined with endonuclease I-SceI, a tool enzyme can digest plasmids with specific I-SceI sites (TAGGGATAACAGGGTAAT) in vivo (Wang et al., 2018). Two I-SceI sites were introduced into the plasmid with target gene-specific sgRNA and homologous arms. After inducing the expression of the endonuclease I-SceI on the other plasmid, the plasmid with the sgRNA was digested during growth and passage. The final plasmid curing rate was improved substantially using this method. The interval time was thus shortened for the next target gene editing. Although the curing rate of target plasmids in our work was not as high as some other reports (Jiang et al., 2015), in subsequent research, we will investigate the cause of this and test this method rigorously by selecting more genes of interest as disruption targets and confirm that this is a universal method for gene inactivation in B. anthracis. Additionally, other plasmid curing methods will also be studied to optimize and improve this current protocol.
Data Availability Statement
The original contributions presented in the study are included in the article/Supplementary Material, further inquiries can be directed to the corresponding authors.
Author Contributions
YW, and CL designed the research. YW, HT, XZ, SS, and QG performed all experiments. YW and CL analyzed data. YW wrote the paper. All authors reviewed the final manuscript.
Conflict of Interest
The authors declare that the research was conducted in the absence of any commercial or financial relationships that could be construed as a potential conflict of interest.
Publisher’s Note
All claims expressed in this article are solely those of the authors and do not necessarily represent those of their affiliated organizations, or those of the publisher, the editors and the reviewers. Any product that may be evaluated in this article, or claim that may be made by its manufacturer, is not guaranteed or endorsed by the publisher.
Supplementary Material
The Supplementary Material for this article can be found online at: https://www.frontiersin.org/articles/10.3389/fbioe.2021.825493/full#supplementary-material
References
Altenbuchner, J. (2016). Editing of the Bacillus Subtilis Genome by the CRISPR-Cas9 System. Appl. Environ. Microbiol. 82 (17), 5421–5427. doi:10.1128/AEM.01453-16
Ao, X., Yao, Y., Li, T., Yang, T.-T., Dong, X., Zheng, Z.-T., et al. (2018). A Multiplex Genome Editing Method for Escherichia coli Based on CRISPR-Cas12a. Front. Microbiol. 9, 2307. doi:10.3389/fmicb.2018.02307
Aquino-Jarquin, G. (2019). CRISPR-Cas14 Is Now Part of the Artillery for Gene Editing and Molecular Diagnostic. Nanomedicine: Nanotechnology, Biol. Med. 18, 428–431. doi:10.1016/j.nano.2019.03.006
Awan, M. J. A., Amin, I., and Mansoor, S. (2021). Mini CRISPR-Cas12f1: a New Genome Editing Tool. Trends Plant Sci. doi:10.1016/j.tplants.2021.11.002
Bigelyte, G., Young, J. K., Karvelis, T., Budre, K., Zedaveinyte, R., Djukanovic, V., et al. (2021). Miniature Type V-F CRISPR-Cas Nucleases Enable Targeted DNA Modification in Cells. Nat. Commun. 12 (1), 6191. doi:10.1038/s41467-021-26469-4
Bindels, D. S., Haarbosch, L., van Weeren, L., Postma, M., Wiese, K. E., Mastop, M., et al. (2017). mScarlet: a Bright Monomeric Red Fluorescent Protein for Cellular Imaging. Nat. Methods 14 (1), 53–56. doi:10.1038/nmeth.4074
Cybulski, R. J., Sanz, P., Alem, F., Stibitz, S., Bull, R. L., and O'Brien, A. D. (2009). Four Superoxide Dismutases Contribute to Bacillus Anthracis Virulence and Provide Spores with Redundant protection from Oxidative Stress. Infect. Immun. 77 (1), 274–285. doi:10.1128/IAI.00515-08
Harrington, L. B., Burstein, D., Chen, J. S., Paez-Espino, D., Ma, E., Witte, I. P., et al. (2018). Programmed DNA Destruction by Miniature CRISPR-Cas14 Enzymes. Science 362 (6416), 839–842. doi:10.1126/science.aav4294
Hartz, P., Gehl, M., König, L., Bernhardt, R., and Hannemann, F. (2021). Development and Application of a Highly Efficient CRISPR-Cas9 System for Genome Engineering in Bacillus Megaterium. J. Biotechnol. 329, 170–179. doi:10.1016/j.jbiotec.2021.02.006
Jeng, A., Sakota, V., Li, Z., Datta, V., Beall, B., and Nizet, V. (2003). Molecular Genetic Analysis of a Group A Streptococcus Operon Encoding Serum Opacity Factor and a Novel Fibronectin-Binding Protein, SfbX. J. Bacteriol. 185 (4), 1208–1217. doi:10.1128/JB.185.4.1208-1217.2003
Jiang, W., and Marraffini, L. A. (2015). CRISPR-cas: New Tools for Genetic Manipulations from Bacterial Immunity Systems. Annu. Rev. Microbiol. 69, 209–228. doi:10.1146/annurev-micro-091014-104441
Jiang, Y., Chen, B., Duan, C., Sun, B., Yang, J., and Yang, S. (2015). Multigene Editing in the Escherichia coli Genome via the CRISPR-Cas9 System. Appl. Environ. Microbiol. 81 (7), 2506–2514. doi:10.1128/AEM.04023-14
Karvelis, T., Bigelyte, G., Young, J. K., Hou, Z., Zedaveinyte, R., Budre, K., et al. (2020). PAM Recognition by Miniature CRISPR-Cas12f Nucleases Triggers Programmable Double-Stranded DNA Target Cleavage. Nucleic Acids Res. 48 (9), 5016–5023. doi:10.1093/nar/gkaa208
Kim, D. Y., Lee, J. M., Moon, S. B., Chin, H. J., Park, S., Lim, Y., et al. (2021). Efficient CRISPR Editing with a Hypercompact Cas12f1 and Engineered Guide RNAs Delivered by Adeno-Associated Virus. Nat. Biotechnol. doi:10.1038/s41587-021-01009-z
Manghwar, H., Lindsey, K., Zhang, X., and Jin, S. (2019). CRISPR/Cas System: Recent Advances and Future Prospects for Genome Editing. Trends Plant Sci. 24 (12), 1102–1125. doi:10.1016/j.tplants.2019.09.006
Ohse, M., Takahashi, K., Kadowaki, Y., and Kusaoke, H. (1995). Effects of Plasmid DNA Sizes and Several Other Factors on Transformation ofBacillus subtilisISW1214 with Plasmid DNA by Electroporation. Biosci. Biotechnol. Biochem. 59 (8), 1433–1437. doi:10.1271/bbb.59.1433
Okano, K., Sato, Y., Hizume, T., and Honda, K. (2021). Genome Editing by Miniature CRISPR/Cas12f1 Enzyme in Escherichia coli. J. Biosci. Bioeng. 132 (2), 120–124. doi:10.1016/j.jbiosc.2021.04.009
Pausch, P., Al-Shayeb, B., Bisom-Rapp, E., Tsuchida, C. A., Li, Z., Cress, B. F., et al. (2020). CRISPR-CasΦ from Huge Phages Is a Hypercompact Genome Editor. Science 369 (6501), 333–337. doi:10.1126/science.abb1400
Savage, D. F. (2019). Cas14: Big Advances from Small CRISPR Proteins. Biochemistry 58 (8), 1024–1025. doi:10.1021/acs.biochem.9b00035
Seo, S.-O., and Schmidt-Dannert, C. (2019). Development of a Synthetic Cumate-Inducible Gene Expression System for Bacillus. Appl. Microbiol. Biotechnol. 103 (1), 303–313. doi:10.1007/s00253-018-9485-4
Takeda, S. N., Nakagawa, R., Okazaki, S., Hirano, H., Kobayashi, K., Kusakizako, T., et al. (2021). Structure of the Miniature Type V-F CRISPR-Cas Effector Enzyme. Mol. Cel 81 (3), 558–570. e553. doi:10.1016/j.molcel.2020.11.035
Toymentseva, A. A., Schrecke, K., Sharipova, M. R., and Mascher, T. (2012). The LIKE System, a Novel Protein Expression Toolbox for Bacillus Subtilis Based on the liaI Promoter. Microb. Cel Fact 11, 143. doi:10.1186/1475-2859-11-143
Turgeon, N., Laflamme, C., Ho, J., and Duchaine, C. (2006). Elaboration of an Electroporation Protocol for Bacillus Cereus ATCC 14579. J. Microbiol. Methods 67 (3), 543–548. doi:10.1016/j.mimet.2006.05.005
Wang, T., Wang, D., Lyu, Y., Feng, E., Zhu, L., Liu, C., et al. (2018). Construction of a High-Efficiency Cloning System Using the Golden Gate Method and I-SceI Endonuclease for Targeted Gene Replacement in Bacillus Anthracis. J. Biotechnol. 271, 8–16. doi:10.1016/j.jbiotec.2018.02.006
Wang, Y., Wang, D., Wang, X., Tao, H., Feng, E., Zhu, L., et al. (2019). Highly Efficient Genome Engineering in Bacillus Anthracis and Bacillus Cereus Using the CRISPR/Cas9 System. Front. Microbiol. 10, 1932. doi:10.3389/fmicb.2019.01932
Wasels, F., Jean-Marie, J., Collas, F., López-Contreras, A. M., and Lopes Ferreira, N. (2017). A Two-Plasmid Inducible CRISPR/Cas9 Genome Editing Tool for Clostridium Acetobutylicum. J. Microbiol. Methods 140, 5–11. doi:10.1016/j.mimet.2017.06.010
Westra, E. R., Buckling, A., and Fineran, P. C. (2014). CRISPR-cas Systems: beyond Adaptive Immunity. Nat. Rev. Microbiol. 12 (5), 317–326. doi:10.1038/nrmicro3241
Wu, Z., Wang, Y., Zhang, Y., Chen, W., Wang, Y., and Ji, Q. (2019). Strategies for Developing CRISPR‐Based Gene Editing Methods in Bacteria. Small Methods 4, 1900560. doi:10.1002/smtd.201900560
Wu, Z., Zhang, Y., Yu, H., Pan, D., Wang, Y., Wang, Y., et al. (2021). Programmed Genome Editing by a Miniature CRISPR-Cas12f Nuclease. Nat. Chem. Biol. 17 (11), 1132–1138. doi:10.1038/s41589-021-00868-6
Xiao, R., Li, Z., Wang, S., Han, R., and Chang, L. (2021). Structural Basis for Substrate Recognition and Cleavage by the Dimerization-dependent CRISPR-Cas12f Nuclease. Nucleic Acids Res. 49 (7), 4120–4128. doi:10.1093/nar/gkab179
Keywords: CRISPR-Cas12f, Bacillus anthracis, genome editing, endonuclease I-SceI, plasmid curing
Citation: Wang Y, Sang S, Zhang X, Tao H, Guan Q and Liu C (2022) Efficient Genome Editing by a Miniature CRISPR-AsCas12f1 Nuclease in Bacillus anthracis. Front. Bioeng. Biotechnol. 9:825493. doi: 10.3389/fbioe.2021.825493
Received: 01 December 2021; Accepted: 27 December 2021;
Published: 14 January 2022.
Edited by:
Tao Chen, Tianjin University, ChinaReviewed by:
Quanjiang Ji, ShanghaiTech University, ChinaChaoyou Xue, Tianjin Institute of Industrial Biotechnology (CAS), China
Copyright © 2022 Wang, Sang, Zhang, Tao, Guan and Liu. This is an open-access article distributed under the terms of the Creative Commons Attribution License (CC BY). The use, distribution or reproduction in other forums is permitted, provided the original author(s) and the copyright owner(s) are credited and that the original publication in this journal is cited, in accordance with accepted academic practice. No use, distribution or reproduction is permitted which does not comply with these terms.
*Correspondence: Yanchun Wang, springwyc@163.com; Chunjie Liu, liucj@nic.bmi.ac.cn