- 1The Royal Veterinary College, Pathobiology and Population Sciences, London, England
- 2Blueberry Therapeutics Ltd., Macclesfield, England
Rising global populations due to medicinal advancements increases the patient population susceptible to superficial and severe fungal infections. Fungi often implicated in these diseases includes the dermatophytes (Microsporum spp., Epidermophtyon spp., Trichophyton spp.) as well as species of the Candida spp., Aspergillosis spp. and Cryptococcus spp. genera. In addition, increasing global populations leads to increasing agricultural demands. Thus, fungal infections of preharvested crops and stored food by plant pathogens such as Magnaporthe oryzae and Fusarium oxysporum can have detrimental socioeconomic effects due to food insecurity. Current antifungal strategies are based mainly on small molecule antifungal drugs. However, these drugs are limited by poor solubility and bioavailability. Furthermore, antifungal resistance against these drugs are on the rise. Thus, antimicrobial polymers offer an alternative antifungal strategy. Antifungal polymers are characterised by cationic and hydrophobic regions where the cationic regions have been shown to interact with microbial phospholipids and membranes. These polymers can be synthetic or natural and demonstrate distinct antifungal mechanisms ranging from fungal cell membrane permeabilisation, cell membrane depolarisation or cell entry. Although the relative importance of such mechanisms is difficult to decipher. Due to the chemical properties of these polymers, they can be combined with other antimicrobial compounds including existing antifungal drugs, charcoals, lipids and metal ions to elicit synergistic effects. In some cases, antifungal polymers and nanocomposites show better antifungal effects or reduced toxicity compared to the widely used small molecule antifungal drugs. This review provides an overview of antimicrobial polymers and nanocomposites with antifungal activity and the current understanding of their antifungal mechanisms.
Introduction
Globally, fungal diseases significantly contribute to the increasing morbidity and mortality rates caused by infectious pathogens. Although fungal infections are usually superficial in healthy individuals, superficial infection often leads to more severe invasive infections in immunosuppressed patients (Kaushik, Pujalte and Reese, 2015). Superficial infections are commonly caused by fungal pathogens of the dermatophyte group (Trichophyton spp., Epidermophyton spp., Microsporum spp.) and includes onychomycosis (fungal nail), tinea corporis (fungal skin) and tinea pedis (athlete’s foot) (Kaushik, Pujalte and Reese, 2015).
In contrast, invasive fungal infections in immunosuppressed patients results in more severe consequences and can lead to tissue damage, organ failure and death (von Lilienfeld-Toal et al., 2019). Fungal pathogens commonly associated with invasive fungal infections include Aspergillus spp., Candida spp., Cryptococcus neoformans and Fusarium solani (Enoch et al., 2016). Currently, the rates of invasive infections are increasing, in part due to the increased lifespan of immunosuppressed patients and the use of immunosuppressive treatments (von Lilienfeld-Toal et al., 2019; Suleyman and Alangaden., 2016). It is estimated that the incidence of invasive fungal infection is ∼ 6 per 100,000 people (von Lilienfeld-Toal et al., 2019). However, this estimate is thought to be lower than the true rate due to poor diagnoses of invasive fungal infections (Denning et al., 2017). Indeed, the severity of invasive infections is directly dependent on the severity of immunosuppression (von Lilienfeld-Toal et al., 2019). Furthermore, fungal infections of preharvested crops such as cereals, grains, fruits and vegetables as well as their storage is a potential threat to food security as the global population is expected to be 9.8 billion by 2050 (Fisher, Gow and Gurr, 2016; United Nations, 2017). Thus, there is increasing demand for the production of good quality agricultural crops in high yield. These agricultural crops are susceptible to infection by Candida spp., Fusarium oxysporum and Magnaporthe oryzae; so antifungals have long been adopted within agricultural settings to maintain crop yield (Fisher, Gow and Gurr, 2016; Brauer et al., 2019). The main class of antifungals used in large-scale production and crop storage is the azole class where triazoles are the most commonly used (Brauer et al., 2019). However, the use antifungals within agricultural settings has direct implications in human health and environmental homeostasis due to increased risk of antifungal resistance (Fisher, Gow and Gurr, 2016; Brauer et al., 2019).
Existing antifungal drugs for the treatment of human infections include a limited number of chemical classes (polyene, allylamine, azole and echinocandin). These mainly target ergosterol within the fungal cell membrane or the synthesis of 1,3-β-D-glucan within the fungal cell wall. The treatment of superficial infections are associated with relapses due to improper clearance of infection (Campoy and Adrio, 2017). Thus, superficial infections often progress into chronic infections (Gupta et al., 2018). In addition, clinical applications of these drugs for invasive infections are often limited by their associated toxicities and complex intravenous administrations (Campoy and Adrio, 2017).
The emergence of single and multi-drug resistant fungi further compounds this treatment problem. Azole resistance amongst Candida spp. and Aspergillus spp. is on the rise along with echinocandins C. glabrata and multidrug resistant (azole, polyene, and echinocandin) C. auris (Perlin et al., 2017).
The limited chemical space and targets sites inhibited by current antifungals is compounded by the rising resistance rates. Antifungal resistance mechanisms include the development of efflux pumps, reduced expression of drug targets or structural alterations of drug targets as well as biofilm development (Cowen et al., 2014; Perlin et al., 2017). These mechanisms are innate in fungal species with reduced susceptibility to antifungal drugs such as C. auris but can be acquired within susceptible species. Consequently, the use of antimicrobial polymers provides a potentially superior alternative to improve current antifungal strategies as summarised in Table 1.
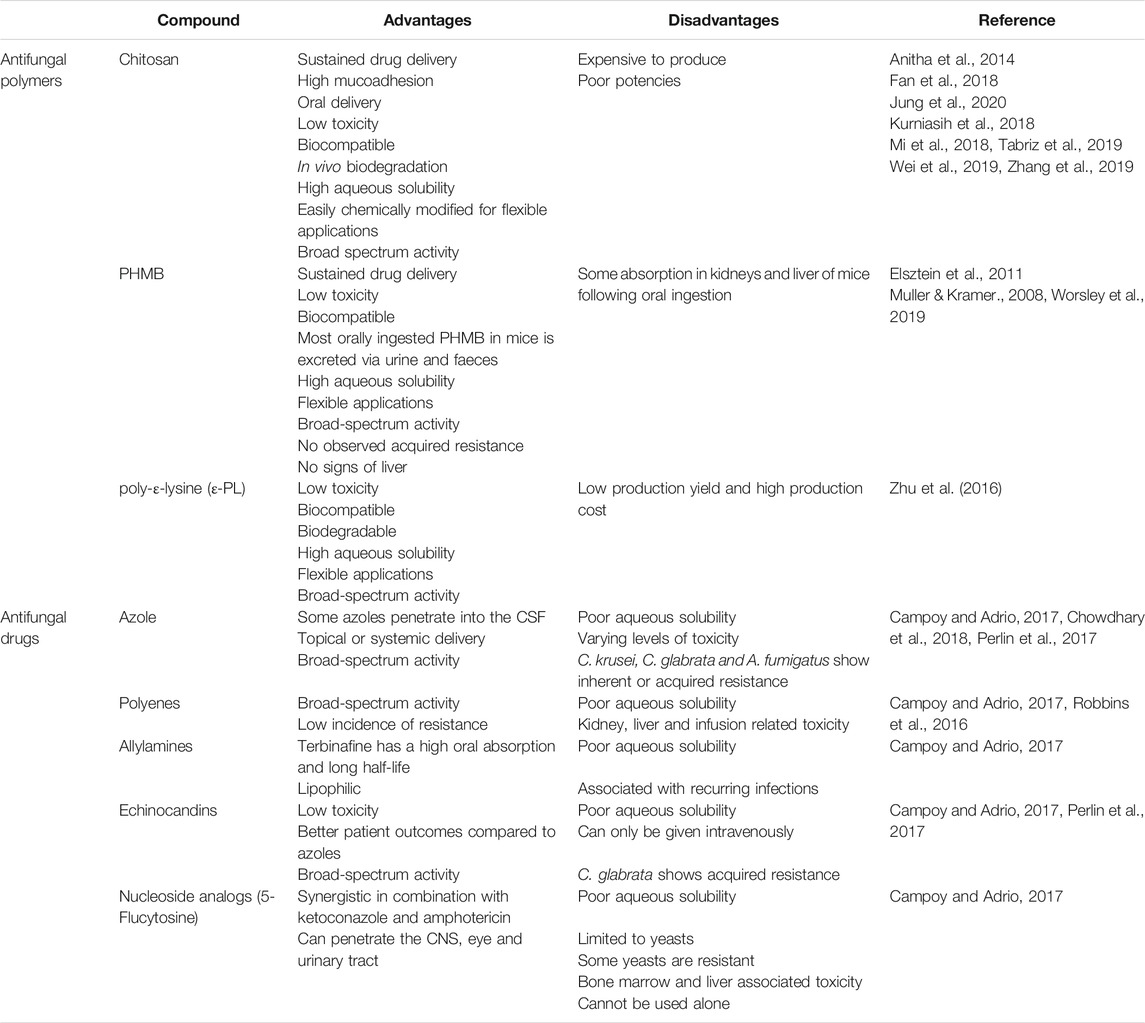
TABLE 1. A generalised overview of the advantages and disadvantages of antifungal polymers in comparison to small molecule antifungals.
Antimicrobial polymers are synthetic or natural organic compounds that exert a broad spectrum cidal effect or growth inhibition of microorganisms. These polymers have broad applications which include eye drops, wound irrigation solutions, wound dressings, cosmetics and water treatment. They also show excellent antibacterial and antifungal properties (Jain et al., 2014), but existing reviews are often focused on the antibacterial mechanisms of these polymers (Kamaruzzaman et al., 2019). In contrast, nanocomposites are hybrid materials and generally refer to a polymer matrix with the addition of nanoparticles. Nanocomposites can refer to colloids, gels, ceramic and metal matrices. The antimicrobial effects of nanocomposites are usually provided via the addition of antimicrobial nanoparticles or metal ions. The addition of these can sometimes improve the electrical and mechanical properties of these materials to widen their potential applications.
Given the burden of fungal diseases and limited treatment options; it is important to highlight the distinct antifungal mechanisms of antimicrobial polymers and nanocomposites to conventional antifungals. Thus, they may help to overcome the challenges of emerging antifungal resistance and to overcome or avoid the challenges of emerging antifungal resistance and biofilm development (Nobile and Johnson, 2015).
In some cases, antifungal polymers and nanocomposites display more potent antifungal effects relative to conventional antifungal drugs. Which begs the question, “Do antifungal polymers and nanocomposites have the potential to replace antifungal drugs?”. This review provides an overview of cationic antimicrobial polymers and nanocomposites with antifungal activity and the current understanding of the antifungal mechanism of action.
Antimicrobial Peptides With Antifungal Activity
Antimicrobial peptides (AMPs) also known as host defence peptides are part of the innate immune response. They are produced by plants, animals and microorganisms and protect the host from invading pathogens (Fernández de Ullivarri et al., 2020). These peptides are amphiphilic with short sequences that are typically less than 100 amino acids (Fernández de Ullivarri et al., 2020). Most AMPs bear a cationic charge where histidine rich AMPs show strong antifungal activity (Kumar, Kizhakkedathu and Straus, 2018). An example of this are Cathelicidins. This class of antifungal AMPs are part of the human innate immune system and are primarily stored in lysosomes of macrophages (Scheenstra et al., 2020). However, there are some AMPs with anionic charges which require metal ions for biological activation (Harris et al., 2009; Phoenix et al., 2013). Anionic AMPs bind metal ions to form cationic salt bridges with anionic microbial membranes for membrane permeabilisation. Although this mechanism is attributed to some anionic AMPs, knowledge of their antimicrobial activity is still limited in comparison to cationic AMPs (Harris et al., 2009).
The main antimicrobial mechanism attributed to cationic AMPs is cell membrane disruption following electrostatic attraction interaction with anionic membranes. In addition, some AMPs can also act translocate across the membrane to act on intracellular targets for DNA and protein synthesis inhibition (Kumar, Kizhakkedathu and Straus, 2018). Despite the antimicrobial effects of AMPs, they are unstable with a short half-life (Kumar, Kizhakkedathu and Straus, 2018). Currently, there are 1,211 peptides of natural, semi synthetic or synthetic origin with antifungal properties in the antimicrobial peptide database (Wang et al., 2016).
Synthetic Antimicrobial Peptides With Antifungal Activity
Synthetic peptide-like polymers are synthesised based on structural moieties of AMPs and therefore usually exert killing or growth inhibition of microbes via their cationic surface charge (Muñoz-Bonilla and Fernández-García, 2012). This class includes polymers with inherent antimicrobial activity such as polymers with quaternary nitrogen groups, halamines or poly-ε-lysine (ε-PL). Despite their excellent antifungal efficacy, many synthetic AMPs are not used clinically due to their potential toxicities in humans. Consequently, there is ongoing research for the design and optimisation of new synthetic AMPs with maintained significant antimicrobial efficacy but reduced toxicity.
Ramamourthy et al. (2020) synthesised peptides with varying number of lysine and tryptophan repeats (KWn-NH2) and their antifungal activity against C. albicans was investigated. The antifungal and biofilm eradication activities of these peptides increases with peptide length; where the shortest peptide KW2 displayed no antifungal activity and the longest peptide KW5, displayed toxicity in a human keratinocyte cell line (Ramamourthy et al., 2020). The KW4 peptide did not disrupt fungal cell membranes. However, laser-scanning confocal microscopy showed KW4 localisation within the cytosol of C. albicans where it was bound to fungal RNA. This suggests the antifungal mechanism of these peptides is not entirely membrane permeabilisation. Instead, these synthetic AMPs enter fungal cells and localises within the cell where they inhibit cellular functions by binding to RNA and DNA resulting in fungal cell death (Ramamourthy et al., 2020).
Dodou Lima et al. (2020) synthesised novel synthetic AMPs with antifungal activity based on the structural determinants of antimicrobial peptides (pilosilin and ponericin) acquired from Dinoponera quadriceps (giant ant) venom. Pilosulin-like (Dq-2562 and Dq-1503) and ponericin-like (Dq-3162) peptides were the most potent antifungal peptides against C. albicans, C. tropicalis, C. parapsilosis and C. krusei. These synthetic peptides in combination with antifungal drugs (amphotericin B, fluconazole, miconazole, cyclopyrox, and nystatin) also showed synergistic effects and with low haemolytic activity (Dodou Lima et al., 2020).
Similarly, (Kodedová et al., 2019), developed synthetic antimicrobial peptides (lasioglossins, halictines and hylanines) based on the antimicrobial peptides of bee venom. These peptides were found to rapidly permeabilise the membranes of Candida species (C. albicans, C. glabrata, C. parapsilosis, C. tropicalis, C. krusei and C. dubliniensis) and Saccharomyces cerevisiae. However, species susceptibility to permeabilisation was dependent on the fungal membrane lipid composition. C. glabrata was observed to have an increased resistance to these synthetic peptides following pre-treatment with terbinafine to reduce fungal membrane ergosterol (Kodedová et al., 2019). The cationic peptides showed strong electrostatic interaction with the anionic membrane lipids (phosphatidylglycerol, phosphatidic acid and cardiolipin) and partly with phosphatidylinositol and the neutral phosphatidylethanolamine (Kodedová et al., 2019) which allows for better fungal selectivity. Thus, synthetic AMPs and peptide-like polymers based on the structural and functional properties of AMPs can overcome the limitations of natural AMPs whilst maintaining or improving their antifungal mechanisms.
Despite synthetic AMPs showing broad-spectrum antifungal activity and low toxicity, research into antimicrobial polymers is often focused on synthetic polymers as they are considerably cheaper to produce in comparison and share functional cationic similarities with AMPs.
Synthetic Antimicrobial Peptide-Like Polymers With Antifungal Activity
Nylon-3 copolymers or poly-β-peptides are a family of synthetic peptide-like polymers based on the structural moiety of AMPs and are made via anionic ring-opening polymerisation of β-lactams (Yang et al., 2011). The resulting polymers (MM-TM, DM-TM, and NM) display improved potency against a diverse range of fungal species including C. albicans, C. krusei, C. auris, C. neoformans, C. amylolentus and A. fumigatus with improved fungal selectivity over mammalian cells (Liu et al., 2013; Liu et al., 2014; Rank et al., 2018). The most effective nylon-3 polymer was found to be poly-βNM, composed of the cationic subunit βNM and the hydrophobic subunit CH in differing proportions. It shows potency against both planktonic, biofilm and mature biofilm C. albicans K1 with an MIC of 3 μg/m (Liu et al., 2015). Polymers with a higher βNM content/cationic charge density are more effective at biofilm inhibition and display more potent fungicidal effect relative to amphotericin B (AmpB). Interestingly, these polymers with increased cationic charge density displayed reduced activity against 48-h biofilm (Liu et al., 2015). This reduction may be due to anionic biofilm components such as DNA, RNA and proteins which may bind to the polymer to hinder penetration to intracellular antifungal sites. Fortunately, certain synthetic peptide-like polymers are unlikely to be toxic and show great safety profiles. For example, the polyamide backbone of the nylon-3 polymers is thought to improve biocompatibility as it is protein-like (Frackenpohl, Arvidsson, Schreiber and Seebach, 2001).A more hydrophobic version of this polymer (70:30, βNM:CH) shows significant haemolysis at 2 mg/ml (Liu et al., 2015). However, this is in keeping with observed toxicities of hydrophobic regions of polymers (Cuervo-Rodríguez, Muñoz-Bonilla, López-Fabal and Fernández-García, 2020).
Synthetic Antimicrobial Polymers With Antifungal Activity
Antimicrobial polymers show cidal effects in both yeasts and filamentous fungi as their cationic charge lends a high binding affinity to the negatively charged microbial membrane surface as summarised in Table 2. These polymers have gained an increase attention in antifungal research as it minimises the challenge of discovering novel antifungal targets and expands the potential applications of new and existing antifungal agents. They are neither costly or labour intensive to manufacture, can easily be chemically modified for broader applications and integrated into nanocomposites for controlled release (Gibney et al., 2012). Subsequently, it has led to interest in developing novel antimicrobial peptides with improved physicochemical properties.
Polyhexamethylene Biguanide
Polyhexamethylene biguanide (PHMB) is a synthetic quarternary ammonium polymer which has been established to be an effective antimicrobial agent with added advantages of low toxicity (Behrens-Baumann et al., 2012; Walls et al., 2013; Sanada et al., 2014; Worsley et al., 2019). It exhibits a high therapeutic index and broad-spectrum antifungal activity due to its biguanide groups and is commonly used as a preservative in cosmetics, water purifications systems and contact lense cleaning solutions. It is also used clinically for wound cleaning where it shows excellent biocompatibility (Gilbert et al., 2001; Muller & Kramer., 2008). Although PHMB shows membrane disruption abilities due to its phospholipid binding (Chindera et al., 2016), the exact antifungal mechanism of action remains unclear. The antifungal mechanism is thought to involve cell wall destabilisation and membrane permeabilisation. Gene expression studies in S. cerevisiae indicated an increase in the expression of cell wall integrity genes and protein kinase C which regulates cell maintenance. This suggests PHMB also damages the b-glucan structure of the S. cerevisiae cell wall (Elsztein et al., 2011).
Polyoctamethylene Guanidine Hydrochloride
Similar to PHMB, Polyoctamethylene guanidine hydrochloride (POGH) is also used as a disinfectant. It has known antibacterial properties but knowledge of its effect on eukaryotic membranes and subsequently fungi is limited. It shows haemolytic activity with an LD50 range of 500–5,000 mg/kg which indicates slight toxicity. Increasing the hydrophobic alkyl carbon chain lengths of this polymer is accompanied by an increase in biocidal and haemolytic activity (Luo et al., 2017). Large unilamellar vesicles loaded with calcein dye showed an increase in polymer/Palmitoyl oleoyl phosphatidyl choline (POPC) membrane interaction and dye leakage rate. Like its analogues, POGH disrupts phospholipid membranes of the eukaryotic cell (Luo et al., 2017).
Polyquartenium-1
Polyquartenium-1 (PQ-1) or polidronium chloride is another quartenary ammonium antimicrobial polymer used in eye contact lense cleaning solutions. Though PQ-1 is known to have predominantly antibacterial properties, it was also found to induce K+ leakage in C. albicans and A. fumigatus, which indicates membrane damage. Furthermore, it caused lysis of spheroplasts of S. marcescens, but not those of C. albicans (Codling, 2003) and prevents the germination of F. solani conidia by a 3 to 4 log10 fold reduction (Kilvington et al., 2013).
In contrast to PHMB and related polymers, PQ-1 is considered too large to enter mammalian cells and lacks hydrophobic regions (Ammar, Noecker and Kahook, 2010). This is made evident by PQ-1’s observed ability to intercalate within the biomembrane structure of small unilamellar vesicles whereas PHMB adsorbs unto the biomembrane surface (Horner et al., 2015).
Polyethylenimines
Polyethylenimines (PEI) are polymers with an amine group and a two carbon (CH2CH2) spacer. They exist in linear and branched polymeric forms with different states at room temperature (Yemuland and Imae, 2008). Linear PEI with secondary amine groups are solid at room temperature whereas branched versions of this polymer with primary, secondary and tertiary amine groups are liquid at room temperature. PEIs are also membrane permeabilisation agents of bacteria and can also enter cells (Yuan and Li, 2017). Thus, they have been used extensively in drug delivery and in vitro transfections. The exact antifungal mechanism is unclear, but PEIs have been shown to depolarise C. albicans membranes (Azevedo et al., 2014). PEI demonstrates antifungal activity for C. albicans with an MIC of 4.83 mg/ml and antibiofilm activity at concentrations above and including the (MIC, 2XMIC, 4XMIC) (Azevedo et al., 2014). Mammalian cells appear tolerant to low molecular weight PEIs. In comparison, high molecular weight PEIs are potentially more toxic to mammalian cells due to their increased cell membrane disruption (Gibney et al., 2012).
Chitosan
Chitosan is a linear β-1,4-polysaccharide of D-glucosamine and N-acetyl-D-glucosamine made from the deacetylation of chitin; a component of the fungal cell wall and exoskeleton of crustaceans (Muxika et al., 2017). It is used as an antimicrobial agent in wound dressings and improves drug delivery across epithelial cell membranes (Anitha et al., 2014). It has applications as a fining agent in winemaking and in agriculture where it is used as a biopesticide to prevent fungal infections of plants as it enhances plant defense systems whilst exerting an antimicrobial effect (Maluin et al., 2020). Chitosan increases the production of the phytoalexin, pisatin and other defence genes including β-glucanase and chitinase (Lopez-Moya et al., 2019). Short chain chitosan, with ≤7 sugar residues are water soluble whereas long polymers of chitosan are only water soluble at pH 7 and below. This has further implications as the short chain chitosan is more effective against planktonic fungi whereas long chain polymers of chitosan show greater effect against fungal biofilms (Jung et al., 2020). In addition, the degree of deacetylation and molecular weight influences the antimicrobial activity of this polymer (Lopez-Moya et al., 2019).
As an antimicrobial, this cationic polymer has a strong binding affinity for nucleic acids and shows membrane permeabilisation ability which results in leakage of cell contents (Young et al., 1982). Chitosan is also able to enter cells where it inhibits growth and RNA synthesis in F. solani (Palma-Guerrero et al., 2008). Acid cleaved chitosan with shorter chains show more potency and have a lower MIC than native chitosan (Kendra and Hadwiger, 1984). However, not all fungi are susceptible to chitosan’s effects.
Some fungi are inherently resistant to chitosan as chitosan makes up a significant proportion of their cell wall which increases their tolerance (Kendra and Hadwiger, 1984). The cell membranes of these fungi also contain saturated phospholipids which decreases membrane fluidity and subsequently reduces membrane permeabilisation of chitosan. An N. crassa mutant with a reduced proportion of unsaturated fatty acids and phospholipids, showed a decrease in chitosan antimicrobial activity compared to wild type N. crassa (Palma-Guerrero et al., 2010). This study also showed that chitosan-resistant and -sensitive fungi belong to different fungal families (Palma-Guerrero et al., 2010).
Chitosan can be modified at the amino group or alcohol groups for phosphorylation, N-modified chitosan, O-modified chitosan, N,O-modified chitosan derivatives, N-alkyl or N-benzyl chitosan. These modifications can improve the solubility, reduce toxicity and increase the antifungal effects of chitosan (Fan et al., 2018; Kurniasih et al., 2018; Mi et al., 2018; Wei et al., 2018; Tabriz et al., 2019; Wei et al., 2019; Zhang et al., 2019).
Derivatives of Synthetic Antifungal Polymers
Polyhexamethylene Guanine Phosphate and Polyhexamethylene Guanidine Hydrochloride
Polyhexamethylene guanine (PHMG) is a cationic polymer closely related to PHMB and is often used in its salt derivatives (polyhexamethylene guanine phosphate (PHMG-P) and polyhexamethylene guanidine hydrochloride (PHMGH)) as a disinfectant. Polyhexamethylene guanidine hydrochloride (PHMGH) can prevent the formation of C. albicans biofilms on solid surfaces (Garcia et al., 2020). It also demonstrates a higher antifungal potency than AmpB and a lower toxicity profile with no haemolytic activity or release of lactate dehydrogenase within the concentration range of 1.25–40.0 μg/ml (Choi, Kim and Lee, 2017). The antifungal mechanisms of PHMG appears to involve the formation of pores within the fungal cell membrane. A membrane study using 1,6-diphenyl-1,3,5-hexatriene labelling showed a significant loss of C. albicans membrane phospholipids and K+ leakage after PHMGH exposure. Dextran leakage from large unilamellar vesicles was also observed following PHMG treatment with pore sizes ranging between 2.3 and 3.3 nm. (Choi, Kim and Lee, 2017). The uses of this polymer also include agriculture, where PHMG inhibits conidia germination at 5 mg/ml and growth at 50 mg/ml of P. digitatum, an important pathogenic fungus of plants. Besides cell wall and membrane disruption, PHMG adsorbs unto the conidial surface, resulting in conidia deformation and collapse (Olmedo et al., 2018). Despite its antifungal advantages, when aerolised for agricultural use and inhaled, it causes lung damage resulting in fibrosis by destroying the membranes of alveolar epithelial cells inducing epithelial-mesenchymal transition (EMT) (Park et al., 2019).
N-(2-Hydroxypropyl)-3-Trimethylammonium Chitosan Chlorides
The chitosan derivative, N-(2-hydroxypropyl)-3-trimethylammonium chitosan chlorides (HTCC) is an antimicrobial polymer used as a preservative within the cosmetics industry. Hoque et al., 2016 showed HTCC to have potent antifungal activity (MIC = 125–250 μg/ml) with killing observed within 2 h. Like chitosan, it also targets the fungal cell membrane to increase membrane permeability and shows very low toxicity (HC50 = >10000 μg/ml) within a mouse model.
Quaternary Ammonium Chloride Derivatives of Chitosan
Amphiphilic quarternary ammonium chloride derivatives of chitosan of differing hydrophobic alkyl chain lengths (C4, C8, and C12) were designed by Jung et al. (2020) with the aim of binding to fungal biofilms. Shorter alkyl chain polymers (C4) showed the most potency against planktonic C. albicans (Jung et al., 2020). However, in the treatment of C. albicans biofilms, longer alkyl chains (C8 and C12) were more effective at eradication (Jung et al., 2020). All the chitosan derivates showed good biocompatibility and low toxicity with rat skin fibroblast cells (L929 cell line) when the MTT assay was performed (Jung et al., 2020).
Functionalised Antifungal Polymers
Not all antimicrobial polymers have an inherent antimicrobial effect. Some inert biocompatible polymers are modified with functional groups which exert an antimicrobial effect. Figure 1 shows the varying antifungal mechanisms displayed by inherent and functionalised antifungal polymers. Ranging from membrane permeabilisation, membrane intercalation and ion sequestering. These functionalised polymers include polystyrenes, polypyridines and polymethylacrylates and do not usually show membrane permeabilisation. In other cases, polymers with inherent antimicrobial properties can also be potentiated through functionalisation.
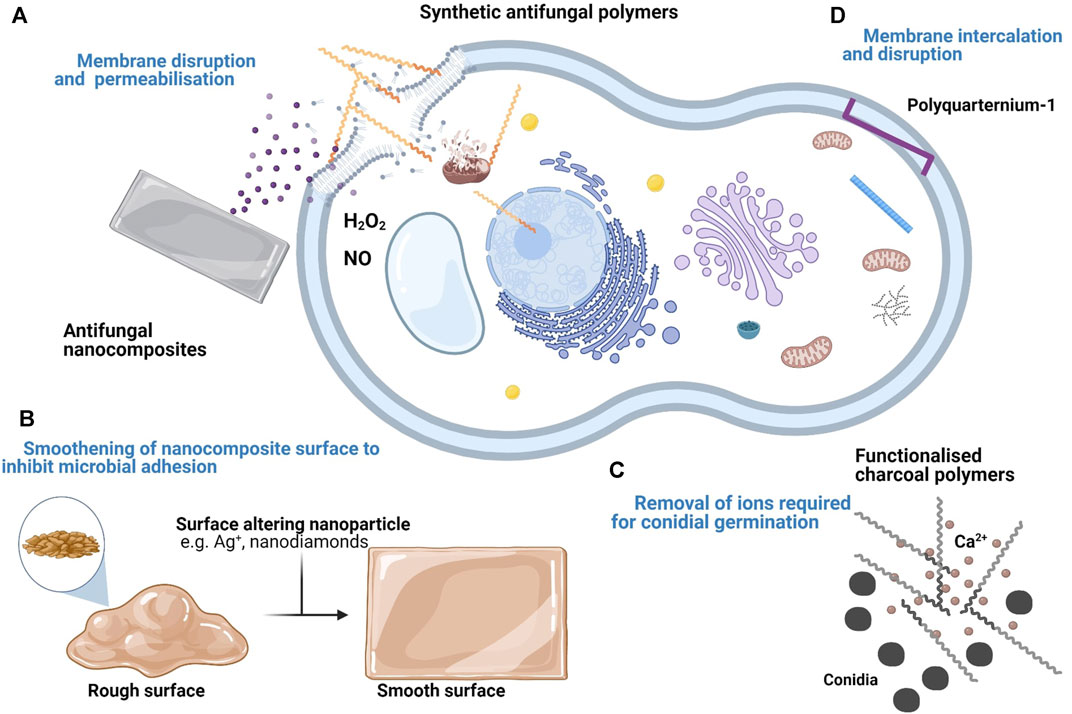
FIGURE 1. Summary of the proposed targets and mechanisms of antifungal polymers and nanocomposites. (A) Cationic polymers and their derivatives disrupt the fungal cell membrane. Upon entry into the fungal cell, they also disrupt organelle membranes and bind to DNA. Some antifungal nanocomposites with membrane disrupting compounds e.g. Chlorhexidine diacetate salt (CDA) also show a similar effect. (B) Some nanocomposites demonstrate an antifungal effect by forming deposits within grooves of the material surface to minimise fungal adhesion. (C) Inert polymers can be functionalised following the addition of antimicrobial compounds. Some functionalised polymers e.g. charcoal polymers inhibit fungal growth by removing essential ions (e.g. Ca2+) necessary for conidia germination. (D) Some antimicrobial polymers e.g. PQ-1 do not permeabilise the fungal cell membrane. Instead, they disrupt the membrane via intercalation.
Functionalised Polymethacrylate Polymers
Polymethacrylates (PMMA) refers to any polymer comprised of a methacrylic acid ester with a wide use of applications. For example, it is used within the cosmetics industry as a wrinkle filler, in ophthalmology as intraocular lenses and in dentistry as dental material (Frazer et al., 2005; Fan et al., 2018). Therefore, it is highly compatible with human tissue and due to its medical applications in medical devices, it is at risk of microbial colonisation from pathogens such as C. albicans. Composites were made of PMMA with undecylenic acid (UA); a monounsaturated lipid with established antifungal activity at differing UA concentrations (3–12%) (Petrović et al., 2018). The addition of UA to PMMA altered the polymer’s surface to increase hydrophilicity. C.albicans exposed to the surface of these PMMA-UA composites showed reduced attachment, growth and increased death of these fungal cells at UA concentrations 6%. Although UA concentrations 9% showed 95% eradication of C. albicans, these composites were highly toxic to human cells with a 50% reduction in viability (Petrović et al., 2018). A balance must therefore be achieved between the ratio of inert polymer to active biocide to produce an effective composite with antifungal activity and lower toxicity.
Composites of PMMA and chlorhexidine diacetate salt (CDA), a cationic disinfectant used to clean the skin prior to surgery showed growth and biofilm inhibition of C. albicans and drug release over time (Maluf et al., 2020). This was observed at different CDA concentrations of 2, 1, and 0.5% with no significant differences in antifungal activity. Chlorhexidine creates pores in the polymer matrix as it diffuses out. This further facilitates diffusion from new to interconnected pores and allows maintained drug release levels from the polymer matrix (Maluf et al., 2020).
Charcoal Polymers
An antifungal food packaging material made from charcoal polymers were developed by Yang et al. (2016). The charcoal polymers were developed by combining charcoal powders with plastic resin under a vacuum (Yang, Cha, Kim and Choi, 2016). These polymers were tested against filamentous fungi (P. variotii, C. globosum and T. virens). P. variotii and T. virens showed growth inhibition of 10 and 30% respectively following exposure. C. globosum conidia, pretreated with these polymers also showed no germination during 5 days of culture following exposure (Yang, Cha, Kim and Choi, 2016). The antifungal mechanism of these polymers involves the adsorption of Ca2+ into the nanosized pores of the charcoal. An estimated 15 mM of Ca2+ were removed from CaCl2 solution with 0.2 g/ml of polymers within 5 h (Yang, Cha, Kim and Choi, 2016). These polymers reduce the amount of readily available Ca2+ required for conidia germination and fungal hyphae growth.
Metal Ion Functionalised Polymers
Metal ions, usually from the d-block of transition metal elements (V, Ti, Cr, Co, Ni, Cu, Zn, Tb, W, Ag, Cd, Au, Hg) are often used as antimicrobial agents as they are toxic to both microorganisms and their biofilms (Turner, 2017). Although studies into the efficacy of these ions are usually focused on bacteria, they also show antifungal effects. Therefore, functional antifungal polymers can be created from the incorporation of these ions into inert polymers. Three new CoII-coordination polymers (Co-CPs), also containing glutarates and bipyridyl ligands, were formulated [Co2(Glu)2(µ-bpa)2]·(H2O)4 (1), [Co4(Glu)4(µ-bpp)2] (2), and [Co2(Glu)2(µ-bpe)2]·(H2O)0.5 (3) (Kim et al., 2019). All three polymers showed more potency against the yeast C. albicans than the filamentous fungi A. niger conidia within the same treatment time with minimal leaching of CoII ions (Kim et al., 2019). C. albicans cells appeared crushed and A. niger conidia appeared wrinkled with peeling outer layers. The antifungal mechanism of these ions includes the generation of reactive species NO and H2O2 which may play a role in fungal killing. For A. niger, it also seems to involve cell wall damage which was not observed in C. albicans and is likely due to difference in cell wall structure of the two fungal species (Kim et al., 2019).
Antifungal Nanocomposites
Antimicrobial nanocomposites are materials that comprise nanosized antimicrobials or biocides to improve the antimicrobial effect. Nanocomposites show a wide range of applications due to a variety of materials and biocides that can be used in combination as shown in Table 3. In some cases, the addition of these nanocompounds improves the structural stability and even thermal stability of the nanocomposite (Norouzi, Zare and Kiany, 2015; Hossain et al., 2019).
Ag+ Based Antifungal Nanocomposites
Antimicrobial metal nanoparticles (NPs) are increasingly used as an alternative to antimicrobial drugs as it minimises the risk of developing antimicrobial resistance (Zare and Shabani., 2016). However, they are associated with toxicity (Grunlan, Choi and Lin, 2005). One approach to improve biocompatibility is to incorporate them into biomaterials such as proteins, peptides and sugars. A green synthesis approach by Jia et al., 2020 was used to generate Ag-Au alloy nanoparticles (Ag-AuNPs) with potential broadspectrum uses which includes as a coating material for medical devices or for drug delivery. The size of these NPs was dependant on Ag content; where increasing Ag, increased NP size. These nanocomposites showed good antifungal activity against C. albicans due to the silver ions. However, the Ag-Au alloy nanoparticles showed improved antifungal activity compared to the silver nanoparticles with MICs as low as 8 mg/ml (Jia et al., 2020). The Ag-Au alloy nanoparticles inhibited growth and biofilm development of fungi in a dose dependent manner with complete suppression and clearance at >16 mg/ml. MTT assays of RAW264.7, Hela and LO2 cells exposed to these nanocomposites showed no significant toxicity at concentrations as high as 128 mg/ml (Jia et al., 2020).
Although the antifungal mechanism(s) associated with AgNPs are unclear, Alghuthaymi et al. (2020) provides some understanding. Chitosan-silver nanocomposites (Ag-Chit-NCs) were developed against P. expansum; a fungal pathogen often associated with animal infections such as dairy cattle and the spoilage of agricultural crops and their storage. The nanocomposite was developed with silver content of 5.9 w/w%. Transmission electron microscopy (TEM) analysis showed 72% of the nanoparticles incorporated into the chitosan matrix were.
∼ 4 nm. Ag-Chit-NCs <10 nm at 0.3 mg/ml showed significant antifungal activity. These particles were observed to internalise within fungi to form cytoplasmic agglomerates (Alghuthaymi et al., 2020). The antifungal mechanism also appears to involve cell organelle and protein binding along with protein and DNA damage (Alghuthaymi et al., 2020). This is in keeping with work by Xia et al., 2016 which shows Ag+ nanoparticles damaged the fungal cell wall, cell membrane, mitochondria, chromatin, and ribosome.
As mentioned earlier under section 5.1, PMMA is a polymeric material frequently used in dental medicine and is therefore at risk of colonisation. Thus, the incorporation of AgNPs within prosthetic matrices could be beneficial to prevent their oral colonisation by microorganisms such as C. albicans. Nanocomposites were made of PMMA and citrate-capping AgNPs with a size of 20 nm and tested for C. albicans colonisation (De Matteis et al., 2019). C. albicans colonisation was analysed by the Miles and Misra technique and scanning electron microscopy (SEM) at 24 and 48 h. The nanocomposites showed a significant reduction in colonisation after 48 h of incubation. At 3.5 wt% AgNPs nanocomposite, the observed colony count was 0.41 × 106 ± 0.43 cfu/ml whereas the control PMMA showed 7 × 106 ± 1.03 cfu/ml (De Matteis et al., 2019). The addition of the AgNPs to PMMA filled the pores of the matrix causing a reduction in surface roughness of PMMA and introduced a negative charge to the polymer surface due to the citrate capping (De Matteis et al., 2019). The alteration of polymer surface topology and introduction of a negative charge reduced the adhesion of C. albicans to the polymer surface whilst the addition of Ag provided an antifungal effect.
Zhang et al. (2017) also tested the antifungal and cytotoxic effects of a developed silver bromide/cationic polymer (poly (4-vinylpyridinium) nano-composite (AgBr/NPVP)-modified PMMA-based dental resin against oral colonisation by C. albicans (Zhang et al., 2017). AgBr/NPVP was added to the PMMA resin at 0.1, 0.2, and 0.3 wt% with PMMA resin only as the control. All nanocomposites showed antifungal activity with both MIC and MFC of 250 μg/ml (Zhang et al., 2017). Following exposure to the nanocomposites, the cell surface of C. albicans cells were observed to have pits and perforations on the cell surface. In addition, confocal laser scanning microscopy (CLSM) showed antibiofilm activity with significantly fewer fungal cells attached to the PMMA nanocomposite surface. Cytotoxicity assays using human dental pulp cells (HDPCs) showed these nanocomposites to be nontoxic with a cell viability >75% (Zhang et al., 2017). The antifungal effect of the (AgBr/NPVP)-modified PMMA nanocomposite is dependent on the amount of AgBr/NPVP composite present and is due to the leaching of Ag+ which damage the fungal cell wall.
Polylactic acid (PLA) is a biocompatible polymer often used in medical implants such as mesh, screws, plates and rods as it produces lactic acid as a breakdown product. PLA electrospun nanofibrous membranes (EFMs) were developed with AgNPs (<0.1%) and cellulose nanofibrils (CNF) as antimicrobial bandages to promote ocular wound healing after trauma to the eyes (Yan et al., 2020). In-vitro cell co-culture experiments with conjunctival epithelial cells (CjECs) and circulating endothelial cells (CECs) showed excellent biocompatibility between the nanocomposite scaffold and the ocular epithelial cells. This compatibility was pronounced in the scaffolds coated with CNF where cell proliferation significantly increased (Yan et al., 2020). Importantly, these nanocomposite scaffolds also inhibited the growth of Fusarium spp.
Antifungal Nanocomposites With Smooth Surfaces for Reduced Microbial Attachment
Improving the surface roughness of the material provides can also have an antimicrobial effect by reducing microbial growth sites. Fouda et al., 2019 introduced antifungal properties to PMMA for use as a dental filler by smoothening the surface of the resin with nanodiamonds (ND). This is similar to the work by De Matteis et al., 2019, where improved surface roughness leads to a reduction in C. albicans attachment. Fouda et al., 2019 added biocompatible NDs at various concentrations (0, 0.5, 1, 1.5% by wt) to PMMA to observe changes in C. albicans adhesion. The surface roughness was measured with a profilometer and the contact angle with a goniometer. The addition of NDs to PMMA smoothed the surface of PMMA and diminished the presence of peaks and valleys. This decreased the attachment of C. albicans cells on the PMMA surface due to loss of settling sites compared to the control group, with the lowest attachment observed at 1% NDs (Fouda et al., 2019).
Antifungal Nanocomposites With Nano Encapsulated Antifungals
Another approach to develop new treatments is to improve existing antifungal drugs to overcome the associated problems of insolubility and drug penetration in human medicine. Therefore, nano encapsulated versions of these drugs are being explored. The antifungal potential of selenium and ketoconazole nanoparticles loaded into hyaluronic acid (HA) hydrogels to treat seborrheic dermatitis (SD) was evaluated by Garg et al., 2020. The antifungal mechanism of ketoconazole is well known where it inhibits the production of ergosterol, an important sterol of fungal cell membranes (Campoy and Adrio, 2017). Meanwhile the antifungal mechanisms of selenium are not as clear. However, it is thought to enhance the production of ROS, with an increase in the level of superoxide radicals resulting in fungal cell deaths (Tran et al., 2009). A hydrogel formulation loaded with ketoconazole and selenium nanoparticles by Garg et al. (2020) showed enhanced solubility, synergy and permeability of the drugs through ex vivo skin. Subsequently, it showed improved antifungal and anti-inflammatory activities, compared with the free drug and nanoparticle suspensions. Another advantage of NP loaded hydrogels is controlled drug release over an extended period. Thus, it allows for a maintained drug level over a specific period at the target site to enhance efficacy whilst reducing adverse side effects. HeLa cells exposed to the hydrogel formulation showed significantly higher percentage of viable cells (∼ > 90%) compared to free drugs. (Garg et al., 2020). The protective effects of the hydrogel may be attributed to HA maintenance of cell hydration as it is a component of the extracellular matrix (Yahya et al., 2014).
Antifungal Nanocomposites Comprising Essential Oils
Essential oils (EOs) are volatile oils extracted from plants. Several EOs have been shown to have excellent antimicrobial activity and there is evidence that they can promote wound healing (Almeida et al., 2016; Saporito et al., 2017; Mahboubi et al., 2018; Labib et al., 2019). The antifungal mechanism of EOs remains unclear but is often attributed to small molecule actives within the oils. The composition of actives in these oils varies depending on the site of extraction e.g. roots or leaves. EOs studied for their antifungal activity are thyme oil (thymol and carvacrol), tea tree oil (terpenes) and peppermint or clove oil (D’agostino et al., 2019). Several studies indicate that the fungal cell wall and membrane are targets of EOs causing loss of membrane integrity and a decrease in the cell membrane ergosterol composition (de Oliveira Lima et al., 2017; D’agostino et al., 2019; Shreaz et al., 2016).
EOs show good biocompatibility when incorporated into materials such as those used in wound dressings and food packaging materials. The use of biopolymers in food packaging has increased recently due to low costs, biodegradability and edibility (Puscaselu et al., 2019). As these polymers have applications in food packaging, natural antifungal biocides such as EOs are being explored to prevent food spoilage. In some cases, the addition of these polymers to EOs also protects EOs against oxidation and evaporation (Hong and Park, 1999).
Starch edible nanocomposite films loaded with Mexican oregano (Lippia berlandieri Schauer) essential oil (MOEO) were evaluated by Aguilar-Sánchez et al. (2019) to characterise their physical and antifungal properties. These nanocomposites were formulated using MOEO (0, 1, or 2% v/v) with bentonite or halloysite (2%) and the antifungal activities against A. niger, Fusarium spp. and Rhizopus spp. were evaluated. A. niger showed the greatest susceptibility to the antifungal effects of the MOEO at 1% with both bentonite and halloysite nanocomposites. Whereas Fusarium spp. showed the most resistance with growth inhibition only observed with 2% MOEO, 2% bentonite nanocomposite (Aguilar-Sánchez et al., 2019). The nanocomposite starch films showed improved physical and antifungal properties where the addition of MOEO decreased pore density and increased pore size of the films (Aguilar-Sánchez et al., 2019).
EOs have also been observed to enhance the antimicrobial activity of antimicrobial polymers (Namivandi-Zangeneh et al., 2019). Clove oil (CO) shows antifungal activity against Candida spp. However, the antifungal activity of CO was greatly improved upon nano emulsification. Nanoemulsions of clove oil and pluronic F-127 with size <40 nm were produced by ultrasonic processing (Betzler de Oliveira de Siqueira et al., 2019). The MICs obtained for the nanoemulsions against C. albicans were 327 ± 154.15 μg/ml compared to 2,180 μg/ml for free CO (Betzler de Oliveira de Siqueira et al., 2019). The improvement in MICs may be due to improved interactions between the EO actives of the nanoemulsions and fungal cells. Eugenol, the major component of CO is thought to exert its antifungal mechanism via membrane and cell wall damage (Jafri, Ansari and Ahmad, 2019) and these effects could be facilitated by inclusion with polymers that are electrostatically attracted to fungal cells and membranes.
Coarse EO (thyme-oregano, thyme-tea tree and thyme-peppermint) emulsions were made using lecithin and tween-80 as emulsifiers. Nanoemulsions with sizes < 100 nm derived from the coarse emulsions were incorporated into chitosan films reinforced with cellulose nanocrystals (CNCs) (Hossain et al., 2019). The nanocomposite films showed significant antifungal activity against A. niger, A. flavus, A. parasiticus, and P. chrysogenum, causing 51–77% growth reduction on inoculated rice (Hossain et al., 2019). The nanocomposite films made with the thyme-oregano EOs showed the most potent antifungal activity. The films also showed a significant increase in antifungal activity and tensile strength following exposure to 750 Gy of ionizing gamma radiation (Hossain et al., 2019).
Like other essential oil actives, thymol, a major component of thyme EO, is thought to provide antifungal effects through cytoplasmic membrane damage in addition to membrane protein interactions and various intracellular targets (D’agostino et al., 2019). It also potentially induces peroxidation of cell membrane lipids due to ROS production (Gao et al., 2016).
An advantage of nanocomposite films is its slow release of volatile components and antifungal actives over time. It has previously been observed that the incorporation of cellulose nanocrystals into the chitosan matrix increases the EO stability and also creates a diffusion matrix for slow release of EOs throughout storage (Boumail et al., 2013; Deng et al., 2017; Hossain et al., 2019). In summary, as shown in Table 4, antifungal nanocomposites show great applications in both medicine and agriculture where they can improve the structure and antifungal properties of materials.
Conclusion
Antimicrobial polymers show excellent antifungal activity, are cheap to synthesise, easily chemically modified and are also stable. Subsequently, they can overcome the limitations of traditional antifungal drugs as well as antimicrobial peptides. However, the exact antifungal modes of action remain unclear. Although existing antimicrobial polymers show significant antifungal properties, further research is required to improve the fungal selectivity. Polymers with innate antifungal activity are characterised by cationic regions that allow for membrane adhesion and in some cases, intracellular nucleic acid binding, hydrophobic regions that allow for membrane embedding and hydrophilic regions that control water solubility and subsequently, bioavailability. Therefore, a balance must be struck between these properties as greater regions of hydrophobicity can also lead to increased toxicity.
Due to these limitations, a supplementary role of these polymers with existing antifungal drugs might be a suitable alternative. Antifungal polymers can be combined with other antimicrobial compounds to enhance antifungal activity. Additional compounds include antifungal drugs, charcoals, lipids or metal ions which can be developed into NPs and incorporated into a range of nanocomposite materials such as creams, packaging materials or surface coatings depending on their downstream applications. This flexibility provides great promise for applications that range from postharvest food preservation to healthcare. Thus, the potential of antifungal polymers to replace antifungal drugs remains.
Author Contributions
WN‐B took the lead in writing the manuscript with support from DC and LG. DC and LG conceived the presented idea and provided direction. LG supervised the findings of this work.
Funding
This work was supported by the London interdisciplinary doctoral training programme (LIDo) which is funded by the Biotechnology and Biological Sciences Research Council (BBSRC) grant number BB/M009513/1. It was also partly funded by Blueberry Therapeutics Ltd. (BBT); a biotechnology company that specialises in nano delivery of antimicrobials.
Conflict of Interest
DC was employed by Blueberry Therapeutics Ltd.
The remaining authors declare that the research was conducted in the absence of any commercial or financial relationships that could be construed as a potential conflict of interest.
Publisher’s Note
All claims expressed in this article are solely those of the authors and do not necessarily represent those of their affiliated organizations, or those of the publisher, the editors and the reviewers. Any product that may be evaluated in this article, or claim that may be made by its manufacturer, is not guaranteed or endorsed by the publisher.
References
Aguilar-Sánchez, R., Munguía-Pérez, R., Reyes-Jurado, F., Navarro-Cruz, A. R., Cid-Pérez, T. S., Hernández-Carranza, P., et al. (2019). Structural, Physical, and Antifungal Characterization of Starch Edible Films Added with Nanocomposites and Mexican Oregano (Lippia Berlandieri Schauer) Essential Oil. Molecules 24 (12), 2340. doi:10.3390/molecules24122340
Alghuthaymi, M. A., Abd-Elsalam, K. A., Shami, A., Said-Galive, E., Shtykova, E. V., and Naumkin, A. V. (2020). Silver/Chitosan Nanocomposites: Preparation and Characterization and Their Fungicidal Activity against Dairy Cattle Toxicosis Penicillium expansum. JoF 6 (2), 51. doi:10.3390/jof6020051
Almeida, L. d. F. D. d., Paula, J. F. d., Almeida, R. V. D. d., Williams, D. W., Hebling, J., and Cavalcanti, Y. W. (2016). Efficacy of Citronella and Cinnamon Essential Oils on Candida Albicans Biofilms. Acta Odontologica Scand. 74 (5), 393–398. doi:10.3109/00016357.2016.1166261
Ammar, D. A., Noecker, R. J., and Kahook, M. Y. (2010). Effects of Benzalkonium Chloride-Preserved, Polyquad-Preserved, and sofZia-Preserved Topical Glaucoma Medications on Human Ocular Epithelial Cells. Adv. Ther. 27 (11), 837–845. doi:10.1007/s12325-010-0070-1
Anitha, A., Sowmya, S., Kumar, P. T. S., Deepthi, S., Chennazhi, K. P., Ehrlich, H., et al. (2014). Chitin and Chitosan in Selected Biomedical Applications. Prog. Polym. Sci. 39 (9), 1644–1667. doi:10.1016/j.progpolymsci.2014.02.008
Azevedo, M. M., Ramalho, P., Silva, A. P., Teixeira-Santos, R., Pina-Vaz, C., and Rodrigues, A. G. (2014). Polyethyleneimine and Polyethyleneimine-Based Nanoparticles: Novel Bacterial and Yeast Biofilm Inhibitors. J. Med. Microbiol. 63 (9), 1167–1173. doi:10.1099/jmm.0.069609-0
Behrens-Baumann, W., Seibold, M., Hofmüller, W., Walter, S., Haeberle, H., Wecke, T., et al. (2012). Benefit of Polyhexamethylene Biguanide in Fusarium Keratitis. Ophthalmic Res. 48 (4), 171–176. doi:10.1159/000337140
Betzler de Oliveira de Siqueira, L., Matos, A. P. D. S., Cardoso, V. D. S., Villanova, J. C. O., Guimarães, B. D. C. L. R., dos Santos, E. P., et al. (2019). Clove Oil Nanoemulsion Showed Potent Inhibitory Effect against Candida Spp. Nanotechnology 30 (42), 425101. doi:10.1088/1361-6528/ab30c1
Boumail, A., Salmieri, S., Klimas, E., Tawema, P. O., Bouchard, J., and Lacroix, M. (2013). Characterization of Trilayer Antimicrobial Diffusion Films (ADFs) Based on Methylcellulose-Polycaprolactone Composites. J. Agric. Food Chem. 61 (4), 811–821. doi:10.1021/jf304439s
Brauer, V. S., Rezende, C. P., Pessoni, A. M., De Paula, R. G., Rangappa, K. S., Nayaka, S. C., et al. (2019). Antifungal Agents in Agriculture: Friends and Foes of Public Health. Biomolecules 9 (10), 521. doi:10.3390/biom9100521
Campoy, S., and Adrio, J. L. (2017). Antifungals. Biochem. Pharmacol. 133, 86–96. doi:10.1016/j.bcp.2016.11.019
Chindera, K., Mahato, M., Kumar Sharma, A., Horsley, H., Kloc-Muniak, K., Kamaruzzaman, N. F., et al. (2016). The Antimicrobial Polymer PHMB Enters Cells and Selectively Condenses Bacterial Chromosomes. Sci. Rep. 6 (1). doi:10.1038/srep23121
Choi, H., Kim, K.-J., and Lee, D. G. (2017). Antifungal Activity of the Cationic Antimicrobial Polymer-Polyhexamethylene Guanidine Hydrochloride and its Mode of Action. Fungal Biol. 121 (1), 53–60. doi:10.1016/j.funbio.2016.09.001
Chowdhary, A., Prakash, A., Sharma, C., Kordalewska, M., Kumar, A., Sarma, S., et al. (2018). A Multicentre Study of Antifungal Susceptibility Patterns Among 350 Candida Auris Isolates (2009-17) in India: Role of the ERG11 and FKS1 Genes in Azole and Echinocandin Resistance. J. Antimicrob. Chemother. 73 (4), 891–899. doi:10.1093/jac/dkx480
Codling, C. E. (2003). Aspects of the Antimicrobial Mechanisms of Action of a Polyquaternium and an Amidoamine. J. Antimicrob. Chemother. 51 (5), 1153–1158. doi:10.1093/jac/dkg228
Cowen, L. E., Sanglard, D., Howard, S. J., Rogers, P. D., and Perlin, D. S. (2014). Mechanisms of Antifungal Drug Resistance. Cold Spring Harb Perspect. Med. 5 (7), a019752. doi:10.1101/cshperspect.a019752
Cuervo-Rodríguez, R., Muñoz-Bonilla, A., López-Fabal, F., and Fernández-García, M. (2020). Hemolytic and Antimicrobial Activities of a Series of Cationic Amphiphilic Copolymers Comprised of Same Centered Comonomers with Thiazole Moieties and Polyethylene Glycol Derivatives. Polymers 12 (4), 972. doi:10.3390/polym12040972
D’agostino, T., Tesse, M., Frippiat, J.P, Machouart, M., and Debourgogne, A. (2019). Essential Oils and Their Natural Active Compounds Presenting Antifungal Properties. Molecules 24 (20), 3713. doi:10.3390/molecules24203713
De Matteis, V., Cascione, M., Toma, C. C., Albanese, G., De Giorgi, M. L., Corsalini, M., et al. (2019). Silver Nanoparticles Addition in Poly(Methyl Methacrylate) Dental Matrix: Topographic and Antimycotic Studies. Ijms 20 (19), 4691. doi:10.3390/ijms20194691
de Oliveira Lima, M. I., Araújo de Medeiros, A. C., Souza Silva, K. V., Cardoso, G. N., de Oliveira Lima, E., and de Oliveira Pereira, F. (2017). Investigation of the Antifungal Potential of Linalool against Clinical Isolates of Fluconazole Resistant Trichophyton Rubrum. J. de Mycologie Médicale 27 (2), 195–202. doi:10.1016/j.mycmed.2017.01.011
Deng, Z., Jung, J., Simonsen, J., Wang, Y., and Zhao, Y. (2017). Cellulose Nanocrystal Reinforced Chitosan Coatings for Improving the Storability of Postharvest Pears under Both Ambient and Cold Storages. J. Food Sci. 82 (2), 453–462. doi:10.1111/1750-3841.13601
Denning, D. W., Perlin, D. S., Muldoon, E. G., Colombo, A. L., Chakrabarti, A., Richardson, M. D., et al. (2017). Delivering on Antimicrobial Resistance Agenda Not Possible without Improving Fungal Diagnostic Capabilities. Emerg. Infect. Dis. 23 (2), 177–183. doi:10.3201/eid2302.152042
Dodou Lima, H. V., de Paula Cavalcante, C. S., and Rádis-Baptista, G. (2020). Antifungal In Vitro Activity of Pilosulin- and Ponericin-like Peptides from the Giant Ant Dinoponera Quadriceps and Synergistic Effects with Antimycotic Drugs. Antibiotics 9 (6), 354. doi:10.3390/antibiotics9060354
Enoch, D. A., Yang, H., Aliyu, S. H., and Micallef, C. (2016). The Changing Epidemiology of Invasive Fungal Infections. Methods Mol. Biol. 1508, 17–65. doi:10.1007/978-1-4939-6515-1_2
Fan, Z., Qin, Y., Liu, S., Xing, R., Yu, H., Chen, X., et al. (2018). Synthesis, Characterization, and Antifungal Evaluation of Diethoxyphosphoryl Polyaminoethyl Chitosan Derivatives. Carbohydr. Polym. 190, 1–11. doi:10.1016/j.carbpol.2018.02.056
Fernández de Ullivarri, M., Arbulu, S., Garcia-Gutierrez, E., and Cotter, P. D. (2020). Antifungal Peptides as Therapeutic Agents. Front. Cell Infect. Microbiol. 10, 105. doi:10.3389/fcimb.2020.00105
Fisher, M. C., Gow, N. A. R., and Gurr, S. J. (2016). Tackling Emerging Fungal Threats to Animal Health, Food Security and Ecosystem Resilience. Phil. Trans. R. Soc. B 371 (1709), 20160332. doi:10.1098/rstb.2016.0332
Fouda, S. M., Gad, M. M., Ellakany, P., Al-Thobity, A. M., Al-Harbi, F. A., Virtanen, J. I., et al. (2019). The Effect of Nanodiamonds on candida Albicans Adhesion and Surface Characteristics of PMMA Denture Base Material - an In Vitro Study. J. Appl. Oral Sci. 27, e20180779. doi:10.1590/1678-7757-2018-0779
Frackenpohl, J., Arvidsson, P. I., Schreiber, J. V., and Seebach, D. (2001). The Outstanding Biological Stability Ofβ- Andγ-Peptides toward Proteolytic Enzymes: An In Vitro Investigation with Fifteen Peptidases. ChemBioChem 2 (6), 445–455. doi:10.1002/1439-7633(20010601)2:6<445:aid-cbic445>3.3.co;2-i
Frazer, R. Q., Byron, R. T., Osborne, P. B., and West, K. P. (2005). PMMA: An Essential Material in Medicine and Dentistry. J. Long Term Eff. Med. Implants 15 (6), 629–639. doi:10.1615/JLongTermEffMedImplants.v15.i6.60
Gao, T., Zhou, H., Zhou, W., Hu, L., Chen, J., and Shi, Z. (2016). The Fungicidal Activity of Thymol against Fusarium Graminearum via Inducing Lipid Peroxidation and Disrupting Ergosterol Biosynthesis. Molecules 21 (6), 770. doi:10.3390/molecules21060770
Garcia, I. M., Rodrigues, S. B., Rodrigues Gama, M. E., Branco Leitune, V. C., Melo, M. A., and Collares, F. M. (2020). Guanidine Derivative Inhibits C. Albicans Biofilm Growth on Denture Liner without Promote Loss of Materials' Resistance. Bioactive Mater. 5 (2), 228–232. doi:10.1016/j.bioactmat.2020.02.007
Garg, A., Singh, C., Pradhan, D., Ghosh, G., and Rath, G. (2020). Topical Application of Nanoparticles Integrated Supramolecular Hydrogels for the Potential Treatment of Seborrhoeic Dermatitis. Pharm. Dev. Techn. 25 (6), 748–756. doi:10.1080/10837450.2020.1740932
Gibney, K. A., Sovadinova, I., Lopez, A. I., Urban, M., Ridgway, Z., Caputo, G. A., et al. (2012). Poly(ethylene Imine)s as Antimicrobial Agents with Selective Activity. Macromol. Biosci. 12 (9), 1279–1289. doi:10.1002/mabi.201200052
Gilbert, P., Das, J. R., Jones, M. V., and Allison, D. G. (2001). Assessment of Resistance towards Biocides Following the Attachment of Micro-organisms to, and Growth on, Surfaces. J. Appl. Microbiol. 91 (2), 248–254. doi:10.1046/j.1365-2672.2001.01385.x
Grunlan, J. C., Choi, J. K., and Lin, A. (2005). Antimicrobial Behavior of Polyelectrolyte Multilayer Films Containing Cetrimide and Silver. Biomacromolecules 6 (2), 1149–1153. doi:10.1021/bm049528c
Gupta, A. K., Mays, R. R., Versteeg, S. G., Piraccini, B. M., Takwale, A., Shemer, A., et al. (2018). Global Perspectives for the Management of Onychomycosis. Int. J. Dermatol. 58 (10), 1118–1129. doi:10.1111/ijd.14346
Harris, F., Dennison, S., and Phoenix, D. (2009). Anionic Antimicrobial Peptides from Eukaryotic Organisms. Cpps 10 (6), 585–606. doi:10.2174/138920309789630589
Hong, K., and Park, S. (1999). Melamine Resin Microcapsules Containing Fragrant Oil: Synthesis and Characterization. Mater. Chem. Phys. 58 (2), 128–131. doi:10.1016/S0254-0584(98)00263-6
Hoque, J., Adhikary, U., Yadav, V., Samaddar, S., Konai, M. M., Prakash, R. G., et al. (2016). Chitosan Derivatives Active against Multidrug-Resistant Bacteria and Pathogenic Fungi: In Vivo Evaluation as Topical Antimicrobials. Mol. Pharmaceutics 13 (10), 3578–3589. doi:10.1021/acs.molpharmaceut.6b00764
Horner, I. J., Kraut, N. D., Hurst, J. J., Rook, A. M., Collado, C. M., Atilla-Gokcumen, G. E., et al. (2015). Effects of Polyhexamethylene Biguanide and Polyquaternium-1 on Phospholipid Bilayer Structure and Dynamics. J. Phys. Chem. B 119 (33), 10531–10542. doi:10.1021/acs.jpcb.5b07162
Hossain, F., Follett, P., Salmieri, S., Vu, K. D., Fraschini, C., and Lacroix, M. (2019). Antifungal Activities of Combined Treatments of Irradiation and Essential Oils (EOs) Encapsulated Chitosan Nanocomposite Films in In Vitro and In Situ Conditions. Int. J. Food Microbiol. 295, 33–40. doi:10.1016/j.ijfoodmicro.2019.02.009
Jafri, H., Ansari, F. A., and Ahmad, I. (2019). New look to phytomedicine : Chapter 9–Prospects of Essential Oils in Controlling Pathogenic Biofilm. London: Academic Press, 203–236. doi:10.1016/B978-0-12-814619-4.00009-4
Jain, A., Duvvuri, L. S., Farah, S., Beyth, N., Domb, A. J., and Khan, W. (2014). Antimicrobial Polymers. Adv. Healthc. Mater. 3 (12), 1969–1985. doi:10.1002/adhm.201400418
Jia, X., Yao, Y., Yu, G., Qu, L., Li, T., Li, Z., et al. (2020). Synthesis of Gold-Silver Nanoalloys under Microwave-Assisted Irradiation by Deposition of Silver on Gold Nanoclusters/triple helix Glucan and Antifungal Activity. Carbohydr. Polym. 238, 116169. doi:10.1016/j.carbpol.2020.116169
Jung, J., Bae, Y., Kwan Cho, Y., Ren, X., and Sun, Y. (2020). Structural Insights into Conformation of Amphiphilic Quaternary Ammonium Chitosans to Control Fungicidal and Anti-biofilm Functions. Carbohydr. Polym. 228, 115391. doi:10.1016/j.carbpol.2019.115391
Kamaruzzaman, N. F., Tan, L. P., Hamdan, R. H., Choong, S. S., Wong, W. K., Gibson, A. J., et al. (2019). Antimicrobial Polymers: The Potential Replacement of Existing Antibiotics. Ijms 20 (11), 2747. doi:10.3390/ijms20112747
Kaushik, N., Pujalte, G. G. A., and Reese, S. T. (2015). Superficial Fungal Infections. Prim. Care Clin. Off. Pract. 42 (4), 501–516. doi:10.1016/j.pop.2015.08.004
Kendra, D. F., and Hadwiger, L. A. (1984). Characterization of the Smallest Chitosan Oligomer that Is Maximally Antifungal toFusarium Solani and Elicits Pisatin Formation inPisum Sativum. Exp. Mycol. 8 (3), 276–281. doi:10.1016/0147-5975(84)90013-6
Kilvington, S., Lam, A., Nikolic, M., and Brady, N. (2013). Resistance and Growth of Fusarium Species in Contact Lens Disinfectant Solutions. Resist. Growth Fusarium Species Contact Lens Disinfectant Solutions. Optom. Vis. Sci. 90 (5), 430–438. doi:10.1097/opx.0b013e31828f4dfe
Kim, H.-C., Mitra, S., Veerana, M., Lim, J.-S., Jeong, H.-R., Park, G., et al. (2019). Cobalt(II)-coordination Polymers Containing Glutarates and Bipyridyl Ligands and Their Antifungal Potential. Sci. Rep. 9 (1). doi:10.1038/s41598-019-50258-1
Kodedová, M., Valachovič, M., Csáky, Z., and Sychrová, H. (2019). Variations in Yeast Plasma‐membrane Lipid Composition Affect Killing Activity of Three Families of Insect Antifungal Peptides. Cell Microbiol. 21 (12). doi:10.1111/cmi.13093
Kumar, P., Kizhakkedathu, J., and Straus, S. (2018). Antimicrobial Peptides: Diversity, Mechanism of Action and Strategies to Improve the Activity and Biocompatibility In Vivo. Biomolecules 8 (1), 4. doi:10.3390/biom8010004
Kurniasih, M., PurwatiCahyati, T., Cahyati, T., and Dewi, R. S. (2018). Carboxymethyl Chitosan as an Antifungal Agent on Gauze. Int. J. Biol. Macromolecules 119, 166–171. doi:10.1016/j.ijbiomac.2018.07.038
Labib, R. M., Ayoub, I. M., Michel, H. E., Mehanny, M., Kamil, V., Hany, M., et al. (2019). Appraisal on the Wound Healing Potential of Melaleuca Alternifolia and Rosmarinus Officinalis L. Essential Oil-Loaded Chitosan Topical Preparations. PLOS ONE 14 (9), e0219561. doi:10.1371/journal.pone.0219561
Liu, R., Chen, X., Falk, S. P., Masters, K. S., Weisblum, B., and Gellman, S. H. (2015). Nylon-3 Polymers Active against Drug-Resistant Candida Albicans Biofilms. J. Am. Chem. Soc. 137 (6), 2183–2186. doi:10.1021/ja512567y
Liu, R., Chen, X., Falk, S. P., Mowery, B. P., Karlsson, A. J., Weisblum, B., et al. (2014). Structure-Activity Relationships Among Antifungal Nylon-3 Polymers: Identification of Materials Active against Drug-Resistant Strains of Candida Albicans. J. Am. Chem. Soc. 136 (11), 4333–4342. doi:10.1021/ja500036r
Liu, R., Chen, X., Hayouka, Z., Chakraborty, S., Falk, S. P., Weisblum, B., et al. (2013). Nylon-3 Polymers with Selective Antifungal Activity. J. Am. Chem. Soc. 135 (14), 5270–5273. doi:10.1021/ja4006404
Lopez-Moya, F., Suarez-Fernandez, M., and Lopez-Llorca, L. (2019). Molecular Mechanisms of Chitosan Interactions with Fungi and Plants. Ijms 20 (2), 332. doi:10.3390/ijms20020332
Luo, X., Jiang, Z., Zhang, N., Yang, Z., and Zhou, Z. (2017). Interactions of Biocidal Polyhexamethylene Guanidine Hydrochloride and its Analogs with POPC Model Membranes. Polymers 9 (12), 517. doi:10.3390/polym9100517
Mahboubi, M., Taghizadeh, M., Khamechian, T., Tamtaji, O. R., Mokhtari, R., and Talaei, S. A. (2018). The Wound Healing Effects of Herbal Cream Containing Oliveria Decumbens and Pelargonium Graveolens Essential Oils in Diabetic Foot Ulcer Model. World J. Plast. Surg. 7 (1), 45–50.
Maluf, C. V., Peroni, L. V., Menezes, L. R., Coutinho, W., Lourenço, E. J. V., and Telles, D. d. M. (2020). Evaluation of the Physical and Antifungal Effects of Chlorhexidine Diacetate Incorporated into Polymethyl Methacrylate. J. Appl. Oral Sci. 28, e20190039. doi:10.1590/1678-7757-2019-0039
Maluin, F. N., Hussein, M. Z., Yusof, N. A., Fakurazi, S., Idris, A. S., Hilmi, N. H. Z., et al. (2020). Phytotoxicity of Chitosan-Based Agronanofungicides in the Vegetative Growth of Oil palm Seedling. PLoS ONE 15 (4), e0231315. doi:10.1371/journal.pone.0231315
Mi, Y., Tan, W., Zhang, J., Wei, L., Chen, Y., Li, Q., et al. (2018). Synthesis, Characterization, and Antifungal Property of Hydroxypropyltrimethyl Ammonium Chitosan Halogenated Acetates. Mar. Drugs 16 (9), 315. doi:10.3390/md16090315
Muller, G., and Kramer, A. (2008). Biocompatibility index of Antiseptic Agents by Parallel Assessment of Antimicrobial Activity and Cellular Cytotoxicity. J. Antimicrob. Chemother. 61 (6), 1281–1287. doi:10.1093/jac/dkn125
Muñoz-Bonilla, A., and Fernández-García, M. (2012). Polymeric Materials with Antimicrobial Activity. Prog. Polym. Sci. 37 (2), 281–339. doi:10.1016/j.progpolymsci.2011.08.005
Muxika, A., Etxabide, A., Uranga, J., Guerrero, P., and de la Caba, K. (2017). Chitosan as a Bioactive Polymer: Processing, Properties and Applications. Int. J. Biol. Macromolecules 105 (2), 1358–1368. doi:10.1016/j.ijbiomac.2017.07.087
Namivandi-Zangeneh, R., Yang, Y., Xu, S., Wong, E. H. H., and Boyer, C. (2019). Antibiofilm Platform Based on the Combination of Antimicrobial Polymers and Essential Oils. Biomacromolecules 21 (1), 262–272. doi:10.1021/acs.biomac.9b01278
Nobile, C. J., and Johnson, A. D. (2015). Candida Albicans Biofilms and Human Disease. Annu. Rev. Microbiol. 69 (1), 71–92. doi:10.1146/annurev-micro-091014-104330
Norouzi, M., Zare, Y., and Kiany, P. (2015). Nanoparticles as Effective Flame Retardants for Natural and Synthetic Textile Polymers: Application, Mechanism, and Optimization. Polym. Rev. 55 (3), 531–560. doi:10.1080/15583724.2014.980427
Olmedo, G. M., Cerioni, L., Sepulveda, M., Ramallo, J., Rapisarda, V. A., and Volentini, S. I. (2018). Polyhexamethylene Guanidine as a Fungicide, Disinfectant and Wound Protector in Lemons Challenged with Penicillium digitatum. Food Microbiol. 76, 128–134. doi:10.1016/j.fm.2018.03.018
Palma-Guerrero, J., Lopez-Jimenez, J. A., Pérez-Berná, A. J., Huang, I.-C., Jansson, H.-B., Salinas, J., et al. (2010). Membrane Fluidity Determines Sensitivity of Filamentous Fungi to Chitosan. Mol. Microbiol. 75 (4), 1021–1032. doi:10.1111/j.1365-2958.2009.07039.x
Park, Y. J., Jeong, M. H., Bang, I. J., Kim, H. R., and Chung, K. H. (2019). Guanidine-based Disinfectants, Polyhexamethylene Guanidine-Phosphate (PHMG-P), Polyhexamethylene Biguanide (PHMB), and Oligo(2-(2-Ethoxy)ethoxyethyl Guanidinium Chloride (PGH) Induced Epithelial-Mesenchymal Transition in A549 Alveolar Epithelial Cells. Inhalation Toxicol. 31 (4), 161–166. doi:10.1080/08958378.2019.1624896
Perlin, D. S., Rautemaa-Richardson, R., and Alastruey-Izquierdo, A. (2017). The Global Problem of Antifungal Resistance: Prevalence, Mechanisms, and Management. Lancet Infect. Dis. 17 (12), e383–e392. doi:10.1016/S1473-3099(17)30316-X
Petrović, M., Bonvin, D., Hofmann, H., and Mionić Ebersold, M. (2018). Fungicidal PMMA-Undecylenic Acid Composites. Ijms 19 (1), 184. doi:10.3390/ijms19010184
Phoenix, D. A., Dennison, S. R., and Harris, F. (2013). Anionic Antimicrobial Peptides: Peptide and Protein Design for Biopharmaceutical Applications. Chichester: Wiley, 83–113. doi:10.1002/9783527652853.ch3
Puscaselu, R., Gutt, G., and Amariei, S. (2019). Rethinking the Future of Food Packaging: Biobased Edible Films for Powdered Food and Drinks. Molecules 24 (17), 3136. doi:10.3390/molecules24173136
Ramamourthy, G., Park, J., Seo, C., J. Vogel, H., and Park, Y. (2020). Antifungal and Antibiofilm Activities and the Mechanism of Action of Repeating Lysine-Tryptophan Peptides against Candida Albicans. Microorganisms 8 (5), 758. doi:10.3390/microorganisms8050758
Rank, L. A., Walsh, N. M., Lim, F. Y., Gellman, S. H., Keller, N. P., and Hull, C. M. (2018). Peptide-Like Nylon-3 Polymers with Activity against Phylogenetically Diverse, Intrinsically Drug-Resistant Pathogenic Fungi. mSphere 3 (3). doi:10.1128/mSphere.00223-18
Robbins, N., Wright, G. D., and Cowen, L. E. (2016). Antifungal Drugs: The Current Armamentarium and Development of New Agents. Microbiol. Spectr. 4 (5). doi:10.1128/microbiolspec.FUNK-0002-2016
Sanada, H., Nakagami, G., Takehara, K., Goto, T., Ishii, N., Yoshida, S., et al. (2014). Antifungal Effect of Non-woven Textiles Containing Polyhexamethylene Biguanide with Sophorolipid: A Potential Method for Tinea Pedis Prevention. Healthcare 2 (2), 183–191. doi:10.3390/healthcare2020183
Saporito, F., Sandri, G., Bonferoni, M. C., Rossi, S., Boselli, C., Icaro Cornaglia, A., et al. (2017). Essential Oil-Loaded Lipid Nanoparticles for Wound Healing. Ijn 13, 175–186. doi:10.2147/IJN.S152529
Scheenstra, M. R., van Harten, R. M., Veldhuizen, E. J. A., Haagsman, H. P., and Coorens, M. (2020). Cathelicidins Modulate TLR-Activation and Inflammation. Front. Immunol. 11, 1137. doi:10.3389/fimmu.2020.01137
Shreaz, S., Shiekh, R. A., Raja, V., Wani, W. A., and Behbehani, J. M. (2016). Impaired Ergosterol Biosynthesis Mediated Fungicidal Activity of Co(II) Complex with Ligand Derived from Cinnamaldehyde. Chemico-Biological Interactions 247, 64–74. doi:10.1016/j.cbi.2016.01.015
Suleyman, G., and Alangaden, G. J. (2016). Nosocomial Fungal Infections. Infect. Dis. Clin. North America 30 (4), 1023–1052. doi:10.1016/j.idc.2016.07.008
Tabriz, A., Ur Rehman Alvi, M. A., Khan Niazi, M. B., Batool, M., Bhatti, M. F., Khan, A. L., et al. (2019). Quaternized Trimethyl Functionalized Chitosan Based Antifungal Membranes for Drinking Water Treatment. Carbohydr. Polym. 207, 17–25. doi:10.1016/j.carbpol.2018.11.066
Tran, P. L., Hammond, A. A., Mosley, T., Cortez, J., Gray, T., Colmer-Hamood, J. A., et al. (2009). Organoselenium Coating on Cellulose Inhibits the Formation of Biofilms by Pseudomonas aeruginosa and Staphylococcus aureus. Appl. Environ. Microbiol. 75 (11), 3586–3592. doi:10.1128/AEM.02683-08
Turner, R. J. (2017). Metal-based Antimicrobial Strategies. Microb. Biotechnol. 10 (5), 1062–1065. doi:10.1111/1751-7915.12785
United Nations (2017). World Population Projected to Reach 9.8 Billion in 2050. and 11.2 billion in 2100, United Nations Department of Economic and Social Affairs. [online] UN DESA |. Available at: https://www.un.org/development/desa/en/news/population/world-population-prospects-2017.html.
von Lilienfeld-Toal, M., Wagener, J., Einsele, H., Cornely, O. A., and Kurzai, O. (2019). Invasive Fungal Infection. Deutsches Arzteblatt Int. 116 (16), 271–278. doi:10.3238/arztebl.2019.0271
Walls, G., Noonan, L., Wilson, E., Holland, D., and Briggs, S. (2013). Successful Use of Locally Applied Polyhexamethylene Biguanide as an Adjunct to the Treatment of Fungal Osteomyelitis. Journal canadien des maladies infectieuses et de la microbiologie medicale 24 (2), 109–112. doi:10.1155/2013/508908
Wang, G., Li, X., and Wang, Z. (2016). APD3: the Antimicrobial Peptide Database as a Tool for Research and Education. Nucleic Acids Res. 44, D1087–D1093. doi:10.1093/nar/gkv1278
Wei, L., Chen, Y., Tan, W., Li, Q., Gu, G., Dong, F., et al. (2018). Synthesis, Characterization, and Antifungal Activity of Pyridine-Based Triple Quaternized Chitosan Derivatives. Molecules 23 (10), 2604. doi:10.3390/molecules23102604
Wei, L., Li, Q., Chen, Y., Zhang, J., Mi, Y., Dong, F., et al. (2019). Enhanced Antioxidant and Antifungal Activity of Chitosan Derivatives Bearing 6-O-Imidazole-Based Quaternary Ammonium Salts. Carbohydr. Polym. 206, 493–503. doi:10.1016/j.carbpol.2018.11.022
Worsley, A., Vassileva, K., Tsui, J., Song, W., and Good, L. (2019). Polyhexamethylene Biguanide:Polyurethane Blend Nanofibrous Membranes for Wound Infection Control. Polymers 11 (5), 915. doi:10.3390/polym11050915
Xia, Z.-K., Ma, Q.-H., Li, S.-Y., Zhang, D.-Q., Cong, L., Tian, Y.-L., et al. (2016). The Antifungal Effect of Silver Nanoparticles on Trichosporon Asahii. J. Microbiol. Immunol. Infect. 49 (2), 182–188. doi:10.1016/j.jmii.2014.04.013
Yahya, R., El-Bindary, A., El-Mezayen, H., Abdelmasseh, H., and Eissa, M. (2014). Biochemical Evaluation of Hyaluronic Acid in Breast Cancer. Clin. Lab. 60 (7), 1115–1121. doi:10.7754/clin.lab.2013.130413
Yan, D., Yao, Q., Yu, F., Chen, L., Zhang, S., Sun, H., et al. (2020). Surface Modified Electrospun Poly(lactic Acid) Fibrous Scaffold with Cellulose Nanofibrils and Ag Nanoparticles for Ocular Cell Proliferation and Antimicrobial Application. Mater. Sci. Eng. C 111, 110767. doi:10.1016/j.msec.2020.110767
Yang, H. J., Cha, Y. J., Kim, H., and Choi, S. S. (2016). Antifungal Activity of Nano and Micro Charcoal Particle Polymers against Paecilomyces variotii, Trichoderma Virens and Chaetomium globosum. New Biotechnol. 33 (1), 55–60. doi:10.1016/j.nbt.2015.08.001
Yang, H., Zhao, J., Yan, M., Pispas, S., and Zhang, G. (2011). Nylon 3 Synthesized by Ring Opening Polymerization with a Metal-free Catalyst. Polym. Chem. 2 (12), 2888. doi:10.1039/c1py00334h
Yemul, O., and Imae, T. (2008). Synthesis and Characterization of Poly(ethyleneimine) Dendrimers. Colloid Polym. Sci. 286 (6–7), 747–752. doi:10.1007/s00396-007-1830-6
Young, D. H., Köhle, H., and Kauss, H. (1982). Effect of Chitosan on Membrane Permeability of Suspension-Cultured Glycine max and Phaseolus vulgaris Cells. Plant Physiol. 70 (5), 1449–1454. doi:10.1104/pp.70.5.1449
Yuan, W., and Li, H. (2017). Nanostructures for Drug Delivery: Polymer-based Nanocarriers for Therapeutic Nucleic Acids Delivery. Oxford: Elsevier Chapter 14, 445–460. doi:10.1016/b978-0-323-46143-6.00014-2
Zare, Y., and Shabani, I. (2016). Polymer/metal Nanocomposites for Biomedical Applications. Mater. Sci. Eng. C 60, 195–203. doi:10.1016/j.msec.2015.11.023
Zhang, J., Tan, W., Wei, L., Chen, Y., Mi, Y., Sun, X., et al. (2019). Synthesis of Urea-Functionalized Chitosan Derivatives for Potential Antifungal and Antioxidant Applications. Carbohydr. Polym. 215, 108–118. doi:10.1016/j.carbpol.2019.03.067
Zhang, Y., Chen, Y.-y., Huang, L., Chai, Z.-g., Shen, L.-j., and Xiao, Y.-h. (2017). The Antifungal Effects and Mechanical Properties of Silver Bromide/cationic Polymer Nano-Composite-Modified Poly-Methyl Methacrylate-Based Dental Resin. Sci. Rep. 7 (1), 1547. doi:10.1038/s41598-017-01686-4
Keywords: antimicrobial polymers, nanocomposites, antifungal, fungal infections, antifungal polymers
Citation: Ntow-Boahene W, Cook D and Good L (2021) Antifungal Polymeric Materials and Nanocomposites. Front. Bioeng. Biotechnol. 9:780328. doi: 10.3389/fbioe.2021.780328
Received: 20 September 2021; Accepted: 02 November 2021;
Published: 24 December 2021.
Edited by:
Marija Vukomanović, Institut Jožef Stefan (IJS), SloveniaReviewed by:
Joanna Mystkowska, Bialystok University of Technology, PolandManuel Ahumada, Universidad Mayor, Chile
Copyright © 2021 Ntow-Boahene, Cook and Good. This is an open-access article distributed under the terms of the Creative Commons Attribution License (CC BY). The use, distribution or reproduction in other forums is permitted, provided the original author(s) and the copyright owner(s) are credited and that the original publication in this journal is cited, in accordance with accepted academic practice. No use, distribution or reproduction is permitted which does not comply with these terms.
*Correspondence: Winnie Ntow-Boahene, d250b3dib2FoZW5lQHJ2Yy5hYy51aw==