- 1Department of Orthopaedics, Chinese PLA General Hospital, Beijing, China
- 2Chinese PLA Medical School, Beijing, China
- 3Beijing National Laboratory for Molecular Sciences, Institute of Chemistry, Chinese Academy of Sciences, Beijing, China
- 4University of Chinese Academy of Sciences, Beijing, China
Rapid and effective hemostasis is of great importance to improve the quality of treatment and save lives in emergency, surgical practice, civilian, and military settings. Traditional hemostatic materials such as tourniquets, gauze, bandages, and sponges have shown limited efficacy in the management of uncontrollable bleeding, resulting in widespread interest in the development of novel hemostatic materials and techniques. Benefiting from biocompatibility, degradability, injectability, tunable mechanical properties, and potential abilities to promote coagulation, wound healing, and anti-infection, hydrogel-based biomaterials, especially those on the basis of natural polysaccharides and proteins, have been increasingly explored in preclinical studies over the past few years. Despite the exciting research progress and initial commercial development of several hemostatic hydrogels, there is still a significant distance from the desired hemostatic effect applicable to clinical treatment. In this review, after elucidating the process of biological hemostasis, the latest progress of hydrogel biomaterials engineered from natural polysaccharides and proteins for hemostasis is discussed on the basis of comprehensive literature review. We have focused on the preparation strategies, physicochemical properties, hemostatic and wound-healing abilities of these novel biomaterials, and highlighted the challenges that needed to be addressed to achieve the transformation of laboratory research into clinical practice, and finally presented future research directions in this area.
Introduction
Traumatic, surgical, disease-related, or drug-induced bleeding can lead to serious clinical outcomes or even death (Wang et al., 2019a). Traumatic blood loss caused by various sudden accidents (e. g. traffic accidents, natural disasters and violent attacks) is closely related to pre-hospital death. It is statistically estimated that about one-third of emergency deaths originate from acute blood loss and its secondary injuries (Kragh et al., 2012; Behrens et al., 2014), and more than half of the battlefield deaths are ascribed to massive blood loss (Hemostatic wound dressing, 2013). On the other hand, interventional diagnostic and surgical treatments during medical procedures are prone to hemorrhage or intracavitary bleeding, especially in areas adjacent to the heart, parenchymal organs, vital vessels, etc. Statistically, severe intraoperative blood loss directly contributes to increased mortality (Marietta et al., 2006). In addition, hematologic disorders or anticoagulant drugs are known to be responsible for abnormal coagulation, which likewise exposes many patients to high bleeding risks (Blajchman, 2003). Therefore, the exploration of rapid and effective methods for bleeding control in different situations has always been an important topic of multidisciplinary research.
Hemostatic techniques and materials with excellent properties are essential for saving lives and reducing adverse side effects. Traditional hemostatic materials (e. g. tourniquets, hemostatic gauze, bandages, and etc.) have been widely used for rapid hemostasis of superficial wounds (Leonard et al., 2016; Montaser et al., 2020; Farahani and Shafiee, 2021). However, as to injuries with intracavitary hemorrhage or involving non-compressive vital tissues/organs (e. g. brain tissue, spinal cord, fragile organs, and etc.), traditional techniques are difficult to meet the demand for rapid and effective hemostasis (Jones et al., 2018; Montaser et al., 2020). Moreover, gauze or bandages need to be completely removed after hemostasis because of their non-degradability, which may cause secondary injury, delayed healing, and additional pain (Hoekstra et al., 2002). The exploration of advanced hemostatic materials including biodegradable gauze, sponges, powders, sprays, and hydrogels have been ongoing. In recent years, the rapid development of hydrogel-based biomaterials for hemostatic and wound healing have led to the emergence of solutions to clinical hemostatic challenges.
Hydrogel-based biomaterials have shown many advantages compared with traditional hemostatic methods. Based on their injectability and flowability, hydrogels can be applied to a variety of irregular wounds and intracavity injuries, which is meaningful for rapid and effective hemostasis. Furthermore, the excellent biocompatibility and biodegradability ensure the safety of hydrogel-based biomaterials for in vivo applications and enhance their ability to promote wound healing. Other properties such as drug delivery, self-healing, self-growth, and stimulation response can be tailored as needed, thus endowing the materials with additional therapeutic functions (Khunmanee et al., 2017; Vakalopoulos et al., 2017; Wang et al., 2019b; Hashemnejad and Kundu, 2019; Matsuda et al., 2019; Xue et al., 2019). Nevertheless, further clinical application of hydrogel-based biomaterials is constrained by certain shortcomings, including weak wet surface adhesion, poor mechanical properties, delayed in situ gel formation, and uncontrollable degradation (Bu et al., 2017; Li et al., 2017; Zhu et al., 2018a; Rao et al., 2018).
This review demonstrates a brief introduction of the physiological mechanisms of hemostasis, and focuses on the natural-derived polysaccharide- and protein-based hemostatic hydrogels in terms of preparation strategies, physicochemical properties, hemostatic and wound-healing abilities, as well as recent advances for clinical applications.
Biological Mechanisms of Hemostasis
The physiological mechanism of hemostasis in the human body is a complex and dynamic process consisting of a series of spatiotemporal reactions. In healthy conditions, vascular endothelial cells secrete a series of anticoagulant factors (heparin-like molecules, thrombomodulators, nitric oxide and prostacyclin) to avoid blood clotting (Hickman et al., 2018). When tissue injury leads to vascular rupture and bleeding, the body will respond rapidly by promoting reactive local vasoconstriction to reduce blood loss, while at the same time promoting the secretion of clotting factors and related proteins and mobilizing the action of platelets (Ruggeri and Mendolicchio, 2007). The damage to vascular endothelium exposes the subendothelial collagen, which attracts platelets for adhesion, and the formation of platelet thrombi is initiated (Versteeg et al., 2013). Normally, platelets adhere to collagen with integrin α2β1 and glycoprotein (GP) VI as the two main receptors, while adhesion under high-shear conditions is enhanced with the help of GPIb-V-IX receptor complexes and von Willebrand factor (vWF) (Wang et al., 2018). The adhesion process promotes platelet activation and aggregation at the bleeding site, enabling rapid platelet thrombus formation, which is known as “primary hemostasis” (Boulton, 2006; Varga-Szabo et al., 2008; Makris et al., 2011). In tandem, the coagulation cascade, known as “secondary hemostasis”, is initiated by both endogenous and exogenous pathways (Roberts et al., 2006). The exogenous pathway is activated by the exposure of tissue factor in the blood, while the endogenous pathway is triggered by the exposure of factor XII to foreign substances with negative charges on the surface, both of which ultimately lead to the activation of coagulation factor X (Hoffman, 2003). Activated factor X converts prothrombinogen into thrombin, which in turn rapidly converts fibrinogen into fibrin monomers, and the monomers could aggregate to form a fibrin network structure (Gale, 2011). Finally, activated factor XIII immobilizes platelet thrombi and other blood components at the bleeding site and forms the final clot by inducing intrafibrillar cross-linking (Morrissey, 2012; Chan et al., 2015). Therefore, hemostatic biomaterials should focus on mimicking and exploiting the complex coagulation mechanisms described above in order to achieve more rapid and effective hemostatic efficacy.
Polysaccharide-based Hemostatic Hydrogels
Polysaccharides are widely present in the natural world as reproducible substances. They have excellent biocompatibility and biodegradability, and in some cases exhibit the ability to promote hemostasis, accelerate wound healing, and antibacterial properties (Yang et al., 2017; Khan and Mujahid, 2019; Chen et al., 2020). However, the deficiency of adhesion strength and mechanical properties constrains the application of polysaccharides being used for the preparation of hydrogel adhesives (Zhong et al., 2021). Therefore, chemical modification and cross-linking with various of performance-enhanced materials has become widely accepted programs (Zhang et al., 2021).
Chitosan
The excellent biocompatibility and degradability, as well as the hemostatic and antibacterial properties reported in previous studies, make chitosan an ideal candidate for hemostatic biomaterials (Jayakumar et al., 2011; Malette et al., 1983; Chou et al., 2003; Cheung et al., 2015). Wang et al. prepared a novel photo-crosslinked chitosan hemostatic hydrogel by introducing two crosslinking mechanisms (photo-induced carbon-carbon double bond crosslinking; catechol-Fe3+ chelation), and two chitosan polymer networks (catechol-modified methacryloyl chitosan; methacryloyl chitosan) (Wang et al., 2020a). The hydrogel not only showed hemostatic properties in a mice liver hemorrhage model, but also exhibited excellent antimicrobial and healing-promoting abilities through Staphylococcus aureus-infected full-thickness skin wound model. Zhao et al. prepared an injectable multifunctional nanocomposite cryogel for hemostasis and healing of incompressible wounds. The cryogel based on carbon nanotubes (CNT) and glycidyl methacrylate functionalized quaternized chitosan (QCSG) showed better coagulation ability, higher blood cell and platelet adhesion and activation than gelatin sponge and gauze, and excellent hemostatic performance in a rabbit liver defect lethal incompressible hemorrhage model. It even showed better hemostasis than Combat Gauze in a standardized round liver hemorrhage model (Zhao et al., 2018) (Figure 1). In another study by Zhao et al., an antimicrobial electroactive injectable hydrogel dressing was discussed. Hydrogels with the main components of quaternized chitosan-g-polyaniline and benzaldehyde group functionalized polyethylene glycol (PEG)-co-poly (glycerol sebacate) was able to promote wound healing, increase granulation tissue thickness and collagen deposition in a full-thickness skin defect model. In addition, the hydrogel dressing could rely on its self-healing ability to extend its service life, which may provide significant convenience for potential clinical applications (Zhao et al., 2017).
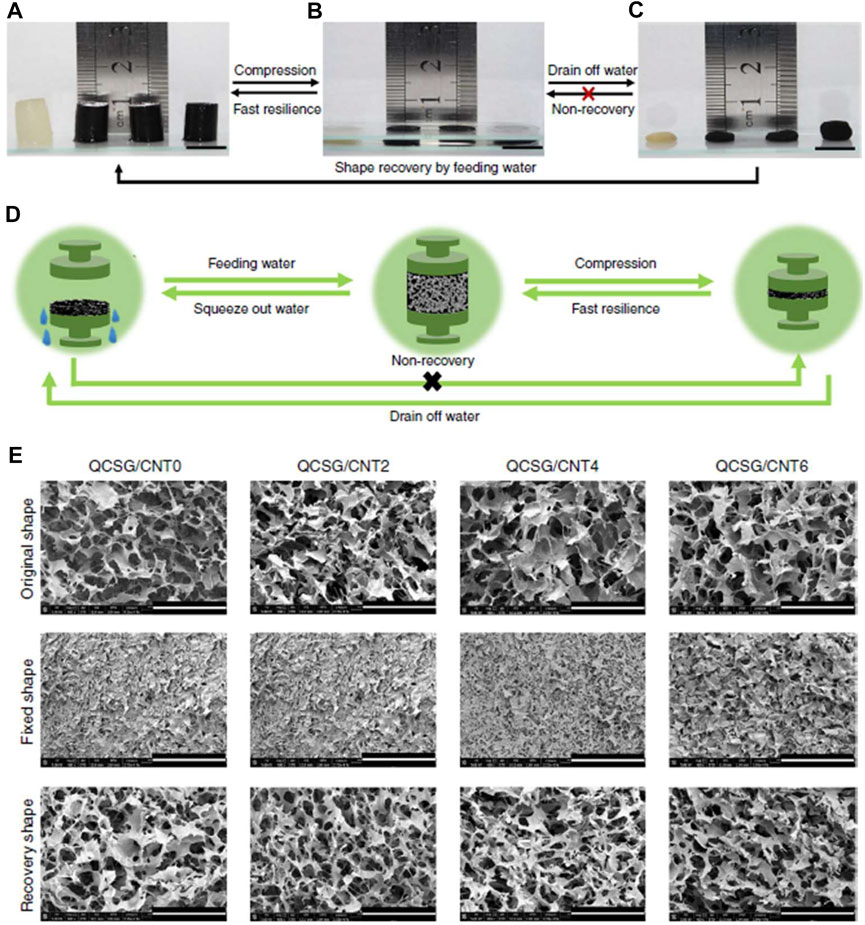
FIGURE 1. Shape memory properties of the cryogels. (A–C) Fast resilience and macroscopical shape memory property of the cryogels. Scale bar: 1 cm. (D) Schematic representation of the shape memory mechanism of the cryogel. (E) Microtopography of the cryogels in original state, shape-fixed state and shape recovery state after fixing. Scale bar: 400 μm. Reproduced from (Zhao et al., 2018) with permission from Copyright 2018 Springer.
Qu et al. prepared a self-healing injectable wound dressing with quaternized chitosan (QCS)-based hydrogel (Qu et al., 2018). The dressing showed tensile and compressive properties comparable to those of human skin and could be used for joint skin injuries. Curcumin loading enabled the dressing to demonstrate favorable antioxidant capacity and pH-responsive release profile, which significantly accelerated wound healing and upregulated vascular endothelial growth factor (VEGF) in a full-thickness skin wound model. To manage bone bleeding and bone defects derived from trauma or bone tumor resection, Huang et al. introduced a multifunctional hydrogel fabricated with catechol-conjugated chitosan (CHI-C) and dialdehyde cellulose nanocrystal (DACNC) (Huang et al., 2021a). After injection into the bone defect area, the hydrogel could be coagulated in situ within 2 min. The ability of this hydrogel to stop bleeding and promote bone regeneration was demonstrated in a rabbit iliac bone defect model (Figure 2). In addition, Eugene et al. prepared a kind of chitosan-PEG-tyramine hydrogel and explored its performance as effective tissue adhesive. Using sutures, fibrin glue and cyanoacrylate as controls, the hydrogels showed rapid glue-forming ability within 5 s and better wound healing in a rat skin incision model (Lih et al., 2012).
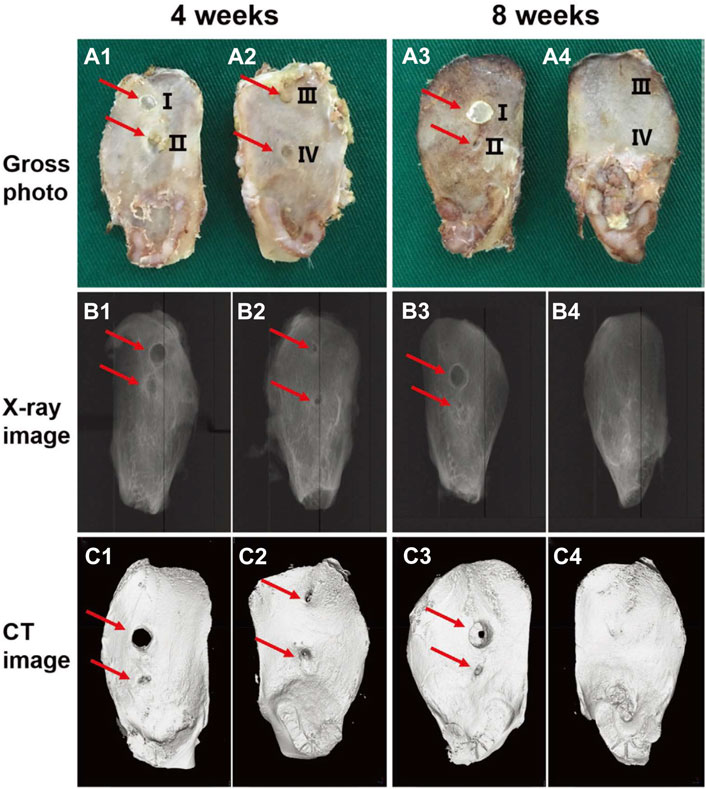
FIGURE 2. Representative gross photograph (A1–A4), radiophograph (B1–B4), and micro-CT scan images (C1–C4) of the ilium bone defects of New Zealand rabbits receiving no treatment (III) or treated with bone wax (I), CHI-C solution (II), or CHI-C/DACNC hydrogel (IV) after 4 and 8 weeks of surgery. The CHI-C/DACNC group shows more abundant bone formation than other groups. Reproduced from (Huang et al., 2021a) with permission from Copyright 2021 Wiley.
Hyaluronic Acid
In response to the weak adhesion of existing hemostatic agents to moist and mobile tissues, an impressive study on hydrogel adhesives was conducted by Hong et al. (2019) The design of this hydrogel system with gelatin and hyaluronic acid (HA) as the main components was inspired by the extracellular matrix. To verify the powerful hemostatic properties of the hydrogel, the authors selected porcine carotid artery and heart hemorrhage models, which placed high demands on the wet surface adhesive ability, mechanical properties, and rapid gelling and fixation ability of the hemostatic material. Encouragingly, the hydrogel successfully sealed the high-pressure hemorrhage from a 4- to 5-mm carotid artery incision and a 6-mm diameter cardiac perforation (Figure 3). Zhu et al. designed a hemostatic and anti-infective hydrogel with sustained drug release capability (Zhu et al., 2018b). The composite material was consisted of aminoethyl methacrylate HA (HA-AEMA), methacrylated methoxy PEG (mPEG-MA) hybrid hydrogels and chlorhexidine diacetate (CHX)-loaded nanogels. In vivo hemostasis and wound healing were evaluated using mice liver hemorrhage and full-thickness skin wound models. The results confirmed the ability of the composite hydrogel to rapidly stop bleeding, accelerate wound healing, and prevent infection.
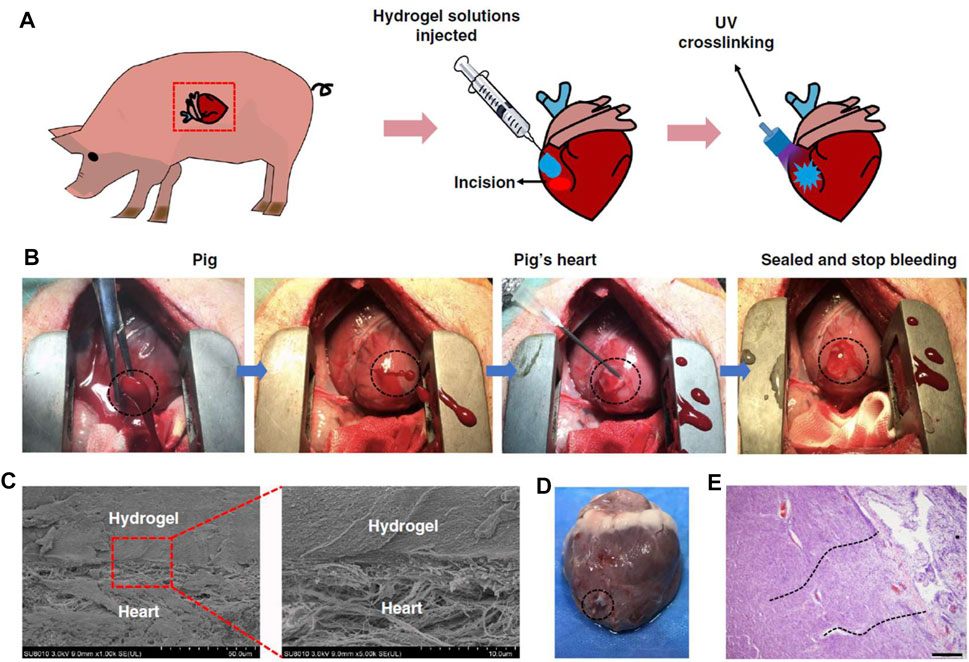
FIGURE 3. Hemostatic properties of the matrix gel in a pig cardiac puncture injury model. (A) Schematic diagram of the surgical procedure. (B) Gross view of the rapid hemostasis and sealing following cardiac puncture injury. (C) Scanning electron micrographs of the interface between the pig heart puncture wound and the hydrogel. Scale bar: 50 μm (left plates); 10 μm (right plates, enlarged). (D) Images of a heart autopsy following killing after two 2 weeks of postoperative recovery, the hydrogel still adhering to the wound. (E) Tissue staining images of the interface between pig heart cardiac tissue and the matrix gel, after 2 weeks of postoperative recovery. Scale bar: 200 μm (n = 4). Reproduced from (Hong et al., 2019) with permission from Copyright 2019 Springer.
Luo et al. introduced a HA/collagen hemostatic hydrogel that was prepared in situ on the tissue surface. In vitro experiments revealed that the hydrogel was stronger than fibrin glue in terms of rupture strength, and a rat liver hemorrhage model showed its hemostatic ability comparable to that of fibrin glue (Luo et al., 2019). As a novel procoagulant, inorganic polyphosphate (PolyP) stored in platelet-dense granules is receiving increasing attention (Ruiz et al., 2004). PolyP has been reported to promote coagulation through four pathways: 1) initiation of the coagulation cascade reaction; 2) activation of coagulation factor V; 3) stabilization of fibrin clots; and 4) facilitation of factor XI feedback activation by thrombin (Müller et al., 2009; Mutch et al., 2010; Choi et al., 2011). Sacoda et al. prepared a hemostatic hydrogel with HA and PolyP. Biocompatibility of the composite hydrogel in vitro and in vivo was demonstrated by viability assays of three cell lines (macrophages, fibroblasts and mesothelial cells) and by intraperitoneal and subcutaneous injections. In addition, the hydrogels showed similar hemostatic effects compared to fibrin glue in a mise liver hemorrhage model (Sakoda et al., 2018).
Alginate
Alginate is an anionic polysaccharide with a strong water absorption capacity (Aderibigbe and Buyana, 2018). It also can activate the coagulation cascade reaction and accelerate platelet aggregation after cross-linking with Ca2+, thus accelerating hemostasis (Lee and Mooney, 2012). Namitha et al. constructed a supramolecular hydrogel scaffold with alginate/poly (N-vinyl caprolactam), followed by ionic cross-linking with Ca2+ and tannic acid (TA) to prepare a pH and temperature dual responsive supramolecular hydrogel (Preman et al., 2020). Hydrogels showed good mechanical properties, and their pore size could be regulated by changing the ratio of polymers. The proper porosity promoted the migration of fibroblasts, the exchange of nutrients and the absorption of exudate. By coating syringe needles with hemostatic hydrogels, Ren et al. prepared a kind of hemostatic needles. The Alginate-based hydrogel (Alg-Ca) would detach from the surface of the needle and stay at the puncture site, preventing further bleeding (Ren et al., 2020). The hemostatic capability of the hydrogel-coated needles in the blood vessel puncture was tested by models of rat external jugular vein and rabbit ear vein, and in viscera puncture was tested by rat kidney and liver. In addition, the hemostatic effect was also investigated in the external jugular vein of hemophilic mice (Figure 4).
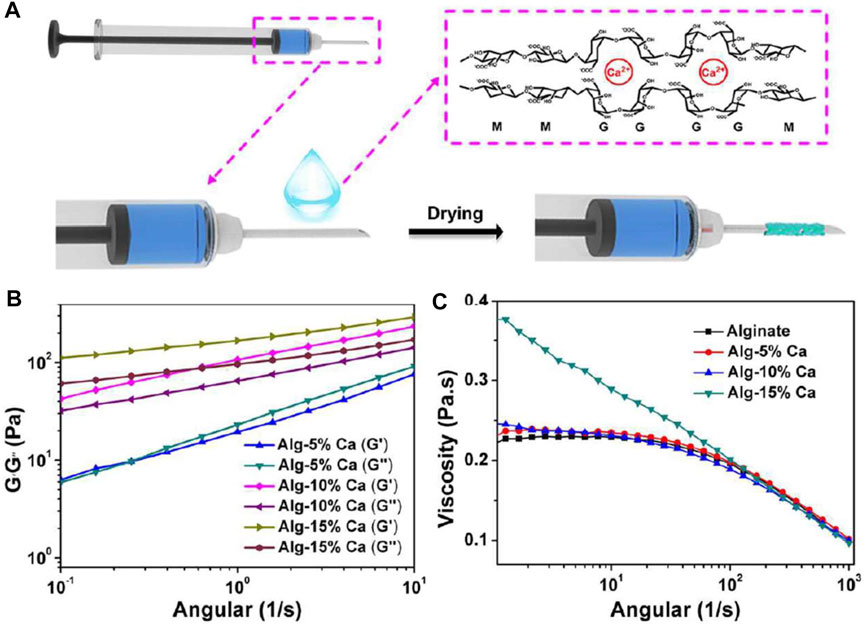
FIGURE 4. Preparation of the hemostatic needles with the Alg-Ca coatings. (A) Schematic illustration of the preparation of hemostatic hydrogel coatings on syringe needles. (B) Rheological analysis of Alg-5% Ca, Alg-10% Ca, and Alg-15% Ca hydrogel films after exposure to serum. (C) The viscosity of alginate, Alg-5% Ca, Alg-10% Ca, and Alg-15% Ca precursor solutions. Reproduced from (Ren et al., 2020) with permission from Copyright 2020 Elsevier.
Oxidized alginate (OA), a dialdehyde derivative of alginate, with a large number of aldehyde groups on its surface which can form Schiff base bonds with the amine groups of histones, thus greatly enhancing the adhesion properties(Reakasame and Boccaccini, 2018). Song et al. prepared dopamine-alginate oxide hydrogels (Dopa-OA) by introducing dopamine into the OA molecular chain in order to further enhance the adhesion properties of OA hydrogels. Meanwhile, polyallylamine (PAA) was selected as the internal structural polymer to improve the mechanical properties (Song et al., 2019). The composite hydrogel could be rapidly gelled in 5–10 s and showed good hemostatic properties in a mice liver hemorrhage model. In the study by Kong et al., a series of hydrogel dressings based on N-carboxyethyl chitosan (CEC) and OA were developed for wound healing (Kong et al., 2021). In a mice full-thickness skin wound model, these hydrogels could significantly promote the healing of infected wounds. Liu et al. developed composite hydrogels with tissue factor-integrated liposomes combined with alginate. Fluorescence measurements showed that the proteoliposomes were uniformly distributed in the alginate matrix and remained intact even after release into simulated body fluids, which exhibited excellent hemostatic properties (Liu et al., 2020).
Cellulose
Cellulose is a major component of plant cell walls and can also be produced by certain microorganisms (Mano et al., 2007). Due to the cost-effective production and the excellent mechanical properties, cellulose has been widely researched in biomedical area (Xiao et al., 2019; Alven and Aderibigbe, 2020). In the study by Huang et al., a hydrogel (CMC/PEG-BA) based on carboxymethyl cellulose (CMC) and a four-armed PEG capped with benzaldehyde (PEG-BA) was developed (Huang et al., 2016). At the same crosslink density, the four-armed PEG network of CMC/PEG-BA was more resistant to fracture than the normal two-armed PEG. After application to rabbit liver incisions, CMC/PEG-BA hydrogel showed good hemostatic ability compared to sterile gauze. Histological evaluation revealed that sterile gauze resulted in a large gap between the wound interfaces, while CMC/PEG-BA was able to trap red blood cells and fill the injury space. Deng et al. made a composite hydrogel by combining fenugreek gum with cellulose through hydrogen bonding in order to impart better mechanical properties to the hemostatic hydrogel (Deng et al., 2020). Based on a porous fiber network structure, the hydrogel can rapidly absorb wound exudate and demonstrated the ability to stop bleeding and promote wound healing through mice liver hemorrhage and skin defect models.
The study by Wang et al. demonstrated a CO2-mediated chemical cross-linking strategy that avoids the use of toxic cross-linking agents to make biocompatible, mechanically strong double-network cellulose/silk fibroin hydrogels (CSH). Through a rat full-thickness skin injury model and a rabbit liver hemorrhage model, the authors verified the hemostatic and wound healing potential of this dual-network hydrogel (Figure 5) (Wang et al., 2020b). Tavakoli et al. developed a novel Kappa carrageenan (κCA)-coated cellulose nanofiber (CNF)/starch nanocomposite hydrogel for hemostasis. The κCA coating imparted higher mechanical strength and lower swelling and degradation rates to the hydrogel, while maintaining the good biocompatibility and hemostatic ability of the starch and cellulose matrices, making κCA-coated starch/CNF hydrogel an ideal candidate for hemostatic applications (Tavakoli et al., 2021). Mendes et al. proposed a porous network hemostatic cryogel based on platelet lysate (PL) and aldehyde-functionalized cellulose nanocrystals (a-CNC) covalently cross-linked. Upon immersion into blood, PL-CNC cryogels showed more powerful absorption capacity compared to commercial hemostatic gelatin sponges. Impressively, the cryogel can release biomolecules to increase stem cell proliferation and migration and down-regulate the expression of fibrinolytic process markers (Mendes et al., 2020).
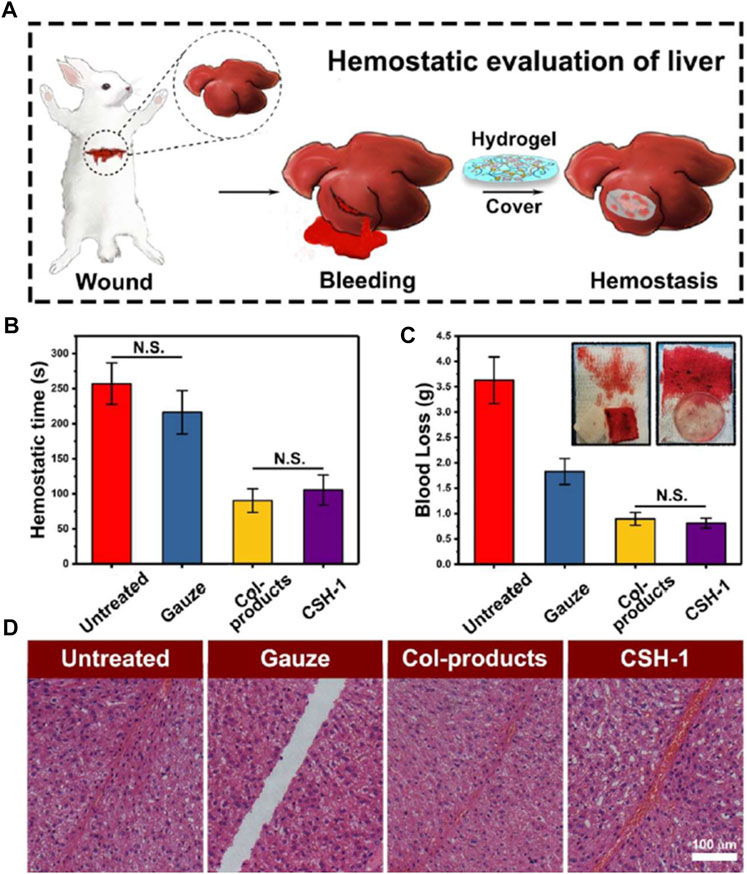
FIGURE 5. Hemostatic evaluation of cellulose/SF hydrogels (CSHs). (A) Surgery scheme, showing that the hemostatic assay was performed with rabbit liver (rabbit experimental number = 4). (B) Hemostatic time from the assay. (C) Blood loss assay. (D) HE staining of the incisions (the scale bar was 100 μm), showing the CSH-1 activated hemocytes’ aggregation in the wounded sites in comparison with the untreated, gauze samples, and Col-products. For hemostatic time and blood loss test, the results were calculated as mean ± SD, n = 4. Reproduced from (Wang et al., 2020b) with permission from Copyright 2020 American Chemical Society.
Protein-Based Hemostatic Hydrogels
Protein-based biomaterials are of wide interest in the biomedical field due to their high mechanical strength, biocompatibility, biodegradability, and flexibility in structure-directed mechanics (Löwik et al., 2010; Woolfson and Mahmoud, 2010; Silva et al., 2014; Nguyen et al., 2018; Zhou et al., 2018). To date, protein-based hydrogels have been extensively developed and are considered as one of the ideal candidates for hemostasis and promotion of tissue healing therapy (Maham et al., 2009; Huang et al., 2018). Collagen, silk, and elastin are common structural proteins for the preparation of protein-based hemostatic biomaterials (Altman et al., 2003; Almine et al., 2010; Chattopadhyay and Raines, 2014).
Gelatin
Gelatin, derived from partial hydrolysis of collagen, has been well reported as a highly promising candidate for biomaterials. However, its further applications are facing some challenges, including low shape stability, rapid degradation profile, and poor mechanical properties (Wang et al., 2014; Sun et al., 2020). Xuan et al. designed a flexible antimicrobial hemostatic dressing with two layers of dopamine/antimicrobial peptide modified gelatin (GDP) and Ca2+ ions (GDP@Ca2+) to provide antimicrobial and hemostatic properties, and another was composed of polycaprolactone (PCL) to provide mechanical strength (Xuan et al., 2020). In vivo evaluation of the bilayer dressing with mice dorsal skin and liver models further demonstrated that the dressing successfully adhered to the tissue surface in a wet bleeding environment, promoting healing while maintaining antimicrobial action for up to 2 weeks (Figure 6).
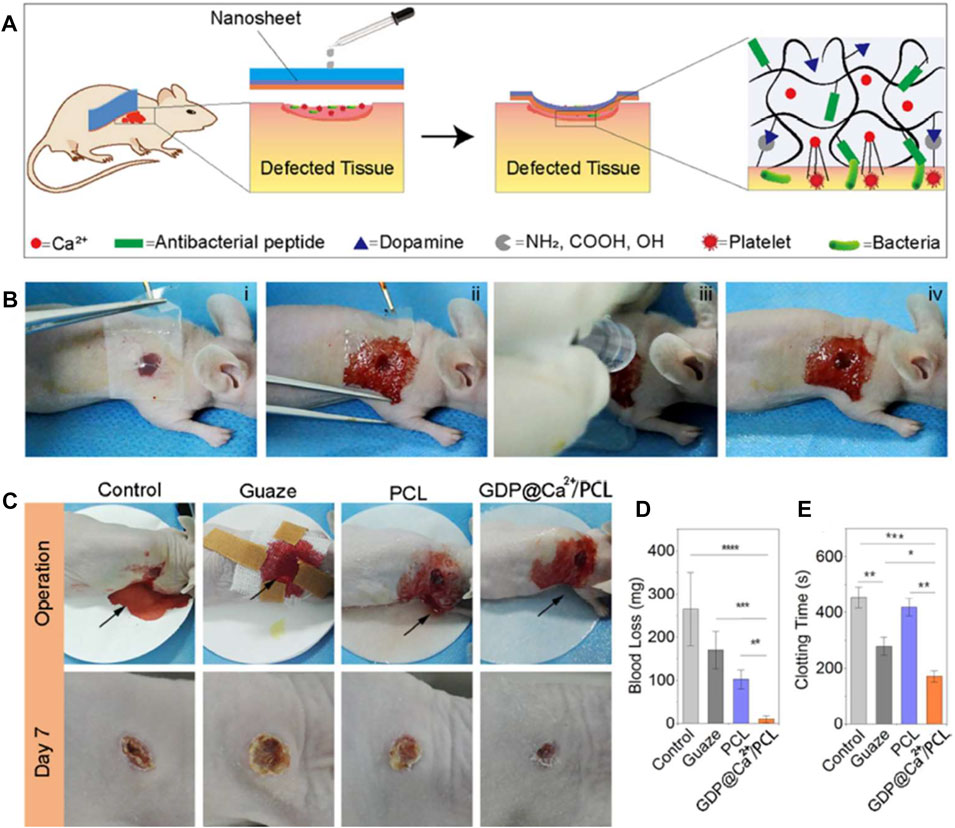
FIGURE 6. The hemostatic and sealing performances of nanosheets on the dorsal skin of a nude mice model. (A) Schematic of using a nanosheet to heal skin wounds with bleeding and infection. (B) The operation process of a GDP@Ca2+/PCL nanosheet on a wound. (C) Images of wound healing after treatment with gauze, PCL and the GDP@Ca2+/PCL nanosheets, with an untreated wound serving as the control group. (D,E) The blood loss (D) and clotting time (E) evaluations of the samples. Reproduced from (Xuan et al., 2020) with permission from Copyright 2020 Elsevier.
Tang et al. constructed a three-dimensional network hydrogel, consisting of methacrylated hyaluronan-polyacrylamide, silver nanoparticles and gelatin (Tang et al., 2020). The adhesive was capable of sustained release of silver ions and thus possessed broad-spectrum antimicrobial activity. In a rat wound infection model, the adhesive showed superior ability to promote wound healing. Yuk et al. reported a dry double-sided tape (DST) for tissue adhesion in wet environment. The tape had a thin hydrogel surface made from a combination of gelatin/chitosan and crosslinked poly (acrylic acid) grafted with N-hydrosuccinimide ester (Yuk et al., 2019). The adhesive mechanism relied on the ability to remove interfacial fluids from tissue surface, thus allowing rapid temporary cross-linking of the material to the tissue surface. The subsequent interfacial covalent cross-linking further improved the adhesion strength and stability. The authors showed through a series of ex vivo organ rupture models (lung, stomach, heart and intestine) that DST could achieve strong adhesion to a variety of wet dynamic tissue surfaces within seconds (Figure 7).
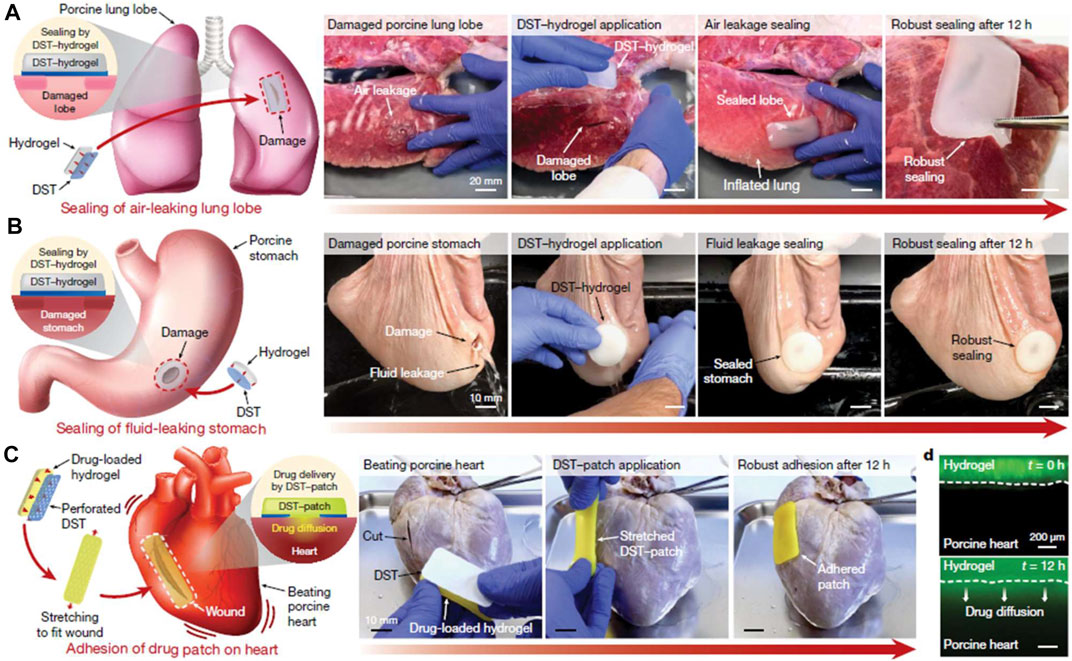
FIGURE 7. Potential applications of the DST. (A) Sealing of an air-leaking lacerated ex vivo porcine lung lobe by a hydrogel patch adhered with the DST. (B) Sealing of a fluid-leaking ex vivo porcine stomach by a hydrogel patch adhered with the DST. (C) DST-mediated adhesion of a drug-loaded patch on a beating ex vivo porcine heart with a cut. (D) Diffusion of a mock drug (fluorescein) from a DST-adhered drug patch into the ex vivo porcine heart tissue over time. Reproduced from (Yuk et al., 2019) with permission from Copyright 2019 Springer.
Gaharwar et al. reported a nanocomposite hemostatic hydrogel consisting of gelatin and synthetic silicate nanoplates (Ak et al., 2014). The nanocomposite reduced blood clotting time by 77% and formed stable clot-gel systems in vitro, and in vivo testing demonstrated its ability to promote hemostasis in fatal liver lacerations. In a study by Liu et al., double network hydrogels were prepared using TA and gelatin methacrylate (GelMA), and the changes in morphology and mechanical properties of hydrogels were explored at different TA concentrations and treatment times (Liu et al., 2018). The results showed that the mechanical properties and adhesion capacity of GelMA-TA hydrogels were significantly improved.
Silk
Silk is a common natural fibrous protein, usually produced by arthropods (e.g., silkworms, bees, spiders, etc.), with unique physicochemical properties (Ma et al., 2018; Tomeh et al., 2019). Low molecular weight Silk fibroin (SF) has been shown to have a definite hemostatic ability (Lei et al., 2016). Huang et al. prepared hemostatic microspheres with different degrees of surface roughness by cross-linking sodium alginate and SF in order to accelerate the blood coagulation. The results showed that when the volume ratio of SA to SF was adjusted to 2:1 (SF/SA2), the surface of the microspheres was the roughest, and thus more red blood cells could be aggregated and the coagulation speed of the hemostatic agent was apparently accelerated (Huang et al., 2021b). Shefa et al. constructed a hydrogel scaffold using oxidized cellulose nanofibers and SF, and explored the effect of thrombin loading on the hemostatic properties of this scaffold. Ultimately, the thrombin-loaded hydrogel scaffold was shown to have good hemostatic ability through the rabbit ear artery as well as the rat severed tail and liver hemorrhage models (Shefa et al., 2019). In the study by Serban et al. SF and PEG were used in the research of hemostatic adhesives. The composite hydrogel can be rapidly formed within seconds by chemical cross-linking and exhibits lower swelling rates and longer degradation times (Serban et al., 2011).
Referring to the chemical composition and hierarchical nanostructure of mussel foot proteins, Bai et al. introduced TA into SF and developed a hemostatic hydrogel sealant with excellent wet adhesion properties (Bai et al., 2019). When being used to seal ruptured bleeding tissue in vivo, the composite hydrogel demonstrated rapid and effective hemostasis, while maintaining good biocompatibility and biodegradability, as well as outstanding antimicrobial activity. The hydrogel was able to adhere firmly to the tissue surface even in a wet dynamic environment, which undoubtedly increased the reliability of its hemostatic effect. Likewise inspired by mussel adhesion proteins, Burke et al. designed a mechanically enhanced catechol-functionalized filamentous protein hydrogel (Burke et al., 2016). The potential advantage of catechol-SF over the available water-soluble catechol adducts based primarily on the PEG was that the catechol-SF were water processable and its hydrophobicity resulted in lower swelling in vivo than that of catechol-PEG. Sen et al. explored the role of hydrogel scaffolds synthesized from SF and polyurethane in diabetic wound healing (Sen et al., 2020). As a wound dressing, the composite material with excellent exudate absorption and broad-spectrum antibacterial ability could significantly promote the healing of chronic hyperglycemic wounds.
Elastin
Elastin is an important protein component of the extracellular matrix (ECM) in tissues such as the vascular system, skin, and lung (Vindin et al., 2019). It is once regarded as an expected source of biomaterials. However, the insolubility and structural stability of elastin hamper its mass production and further research (Kielty et al., 2002). Accordingly, soluble tropoelastin (precise replicas of the natural elastin precursor) as well as elastin-like polypeptides (ELP) have emerged as candidates for elastin (Wise and Weiss, 2009; Kozel and Mecham, 2019; Reichheld et al., 2019). Annabi et al. designed a highly elastic hydrogel sealant with tunable adhesion properties for surgical applications. The methacrylate-substituted tropoelastin (MeTro) hydrogel was obtained by photo-crosslinking the recombinant human protein tropoelastin (Annabi et al., 2017a). After MeTro hydrogel was used to seal a series of in vivo incision models (rat arteries, lungs, and porcine lungs), all animals were able to survive without functional abnormalities during the observation period. Based on the above work, Annabi et al. reported a sprayable, elastic composite hydrogel composed of two ECM-derived biopolymers (GelMA and MeTro) for the treatment of chronic wounds (Annabi et al., 2017b). Interestingly, the physical properties of hydrogel can be fine-tuned by varying the MeTro/GelMA ratio and the final polymer concentration. Moreover, loading of antimicrobial peptides conferred broad-spectrum antimicrobial ability to the composite hydrogel.
Brennan et al. prepared an adhesive with underwater adhesion and “smart” environmental response behavior. The adhesive is constructed with an ELP from Escherichia coli and modified with 3,4-dihydroxyphenylalanine (DOPA), which can coacervate in response to environmental factors such as temperature, pH and salinity (Brennan et al., 2017). Compared to commercially available fibrin glues, this adhesive exhibits significantly higher adhesion strength in dry, wet and even submerged environments. In the study by Desai et al. flexible hydrogel adhesive was prepared, similarly based on the chemical modification of ELP by dopamine (Desai et al., 2020). The hydrogel exhibited a stable swelling rate at 37°C under aqueous conditions. In addition, the adhesive strength of the flexible adhesive was revealed through tensile pull-off and lap-shear testing on porcine skin. In another attempt to overcome adhesion challenges in the underwater environment, Narayanan prepared a kind of tropoelastin-like self-coacervating polyesters that could mimic the self-coacervation and environmental stability of a mussel adhesive protein (Narayanan et al., 2020). This smart material demonstrates the potential for rapid underwater adhesion applications.
Conclusion and Outlook
When facing a hemorrhagic injury, the body’s inherent hemostatic mechanisms become insufficient to rapidly stop blood loss, and certain materials and techniques are often needed to achieve rapid hemostasis (Neubauer and Zieger, 2021). Traditional hemostatic methods (tourniquets, gauze, bandages, etc.) are mainly relied on the physical blockage and limited activation of the coagulation system, which can hardly achieve effective hemostasis and may instead face additional problems such as secondary injury, immune rejection and infectious risk (Hagemann et al., 2013; Hickman et al., 2018). Commercially available hemostatic materials such as porcine-derived fibrin sealant and gelatin matrix have been approved for clinical application, but adhesion and mechanical deficiencies combined with high cost and risk of disease transmission greatly limit their clinical efficacy, especially in acute or severe bleeding situations. Therefore, modern medicine needs a revolution in hemostatic techniques urgently to address the numerous medical risks associated with excessive blood loss. Hydrogels derived from polysaccharides and proteins are biocompatible, biodegradable, non-immunogenic, and can provide powerful hemostatic ability by promoting, enhancing, compensating, or mimicking the natural mechanisms of hemostasis (Ryu et al., 2015; Zhang et al., 2015; Hu et al., 2019; Rizzo and Kehr, 2021). Although research on polysaccharide- and protein-based hydrogels for hemostasis continues to gain momentum, there are still critical issues to overcome before they can be translated for clinical applications. The challenge lies mainly in the difficulty of achieving the harmonization between biosafety, hemostatic effectiveness, and practical feasibility.
It is of great significance to define the key properties that an ideal hemostatic hydrogel-based biomaterial should have and thus guide the future research. When being applied to the human body, hydrogel-based materials must primarily ensure biosafety. In this regard, biocompatibility, biodegradability, non-toxicity, and non-immunogenicity should be the prerequisites for the application of ideal hemostatic hydrogels. On the other hand, the effectiveness of hemostasis needs to be considered, which requires biomaterials to possess not only superior hemostatic ability, but also outstanding physical and chemical properties to meet the needs of specific conditions, for example, a wet, dynamic, irregular wound. Therefore, reliable biocompatibility, biodegradability, mechanical strength, wet surface adhesion, viscoelasticity, and fatigue resistance are necessary for becoming ideal hemostatic hydrogel-based biomaterials. In addition to biosafety and hemostatic effectiveness, practical feasibility is equally significant and easily ignored. Reduced production costs, simplified preparation processes, ease of handling, and convenient preservation are important prerequisites for clinical applications. In addition, hemostatic hydrogels need to be individually designed to meet the requirement of complicated clinical situations. For example, stronger wet surface adhesion is required in the face of abnormal coagulation due to chronic disease. Hemostasis around nerve tissue imposes stringent requirements on the control of swelling rates. hemostasis of internal organs requires hydrogels that can resist specific enzyme without enzymatic breakdown before complete hemostasis is achieved. Other properties such as wound healing promotion, anti-inflammatory and antimicrobial capabilities are also part of the hemostasis requirements in certain circumstances and need to be addressed on a situation-specific basis. There are no uniform standards for the adhesion and mechanical strength of ideal hemostatic materials, which may be due to differences in application requirements (skin, organ, arterial or cardiac surface hemostasis) and hemostatic mechanisms (physical sealing, coagulation mechanisms). In general, the ideal hemostatic hydrogel should have an adhesion strength of at least tens to hundreds KPa.
Natural-derived polysaccharide- and protein-based hydrogels have the advantages of excellent biosafety, however, their hemostatic effectiveness are often compromised by insufficient mechanical and adhesion strength (Zia et al., 2015; Alven and Aderibigbe, 2020; Graça et al., 2020; Zhang et al., 2020). Further research is expected to improve wet surface adhesion, pressure-resistant intensity and rapid gelation ability through multiple methods, including chemical modifications, cross-linking with functionalized components, advanced preparation techniques, and thus facilitating the transformation of hemostatic biomaterials from laboratory research to clinical applications. Further research should be devoted to enhancing hemostatic efficacy through a variety of methods. However, in order to enhance the mechanical properties, many of the current hemostatic hydrogels are prepared by complex synthetic routes and often with the help of non-biocompatible synthetic polymers, a process that is highly susceptible to reduced biosafety. Therefore, to ensure biosafety, the reaction steps should be simplified as much as possible, while avoiding the addition of components with poor biocompatibility. The prepared materials should undergo comprehensive safety verification, including the metabolic pathways of degradation products as well as a toxicological testing. Simplified chemistry reactions also facilitate control production costs, and materials consisting of simple ingredients approved by regulatory agencies are more likely to enter clinical trials. Future research on the enhancement of hemostatic ability can be achieved either by increasing the adhesion and mechanical properties of hydrogels or by enhancing the physiological coagulation process. If the rapid formation of blood clots can be promoted to reinforce the hemorrhage seal, the requirements for the adhesive and mechanical properties of the hydrogels can be relatively reduced. This process can be achieved with the loading of key coagulation factors or proteins.
In conclusion, by summarizing previous preclinical studies, we discussed the natural-derived polysaccharide-based (chitosan, HA, alginate, cellulose) and protein-based (gelatin, silk, elastin) hemostatic hydrogels in terms of preparation strategies, physicochemical properties, hemostatic and wound-healing abilities. These life-saving materials are expected to revolutionize civilian or military bleeding control options, improve the quality of treatment, and ultimately lead to a radical change in hemostasis technology.
Author Contributions
JC and JL contributed equally to this reviewed paper. LZ and XW initiated the project. ML and ZL searched the data base. JC and JL wrote and finalized the manuscript. XW and ZW made suggestions and revised the paper. All authors reviewed and commented on the entire manuscript.
Funding
This work was supported by Major Projects of Military Logistics Research Projects (No. AWS17J004), National Natural Science Foundation of China (21935011 and 51973226), The Youth Innovation Promotion Association CAS (No. 2019031), Scientific Research Project of Capital Health Development (No. 2018-4–5014), The Military Medical Science and Technology Youth Development Program (19QNP052 and 21QNPY112), The China Postdoctoral Science Foundation funded project (2019M664007 and 2021T140793) and Research on Prevention and Treatment of Military Training Injuries (20XLS28).
Conflict of Interest
The authors declare that the research was conducted in the absence of any commercial or financial relationships that could be construed as a potential conflict of interest.
Publisher’s Note
All claims expressed in this article are solely those of the authors and do not necessarily represent those of their affiliated organizations, or those of the publisher, the editors and the reviewers. Any product that may be evaluated in this article, or claim that may be made by its manufacturer, is not guaranteed or endorsed by the publisher.
References
Aderibigbe, B. A., and Buyana, B. (2018). Alginate in Wound Dressings. Pharmaceutics 10 (2), E42. doi:10.3390/pharmaceutics10020042
Almine, J. F., Bax, D. V., Mithieux, S. M., Nivison-Smith, L., Jelena Rnjak, J., Waterhouse, A., et al. (2010). Elastin-based Materials. Chem. Soc. Rev. 39 (9), 3371–3379. doi:10.1039/b919452p
Altman, G. H., Diaz, F., Jakuba, C., Calabro, T., Horan, R. L., Chen, J., et al. (2003). Silk-based Biomaterials. Biomaterials 24 (3), 401–416. doi:10.1016/s0142-9612(02)00353-8
Alven, S., and Aderibigbe, B. A. (2020). Chitosan and Cellulose-Based Hydrogels for Wound Management. IJMS 21 (24), 9656. doi:10.3390/ijms21249656
Annabi, N., Rana, D., Shirzaei Sani, E., Portillo-Lara, R., Gifford, J. L., Fares, M. M., et al. (2017). Engineering a Sprayable and Elastic Hydrogel Adhesive with Antimicrobial Properties for Wound Healing. Biomaterials 139, 229–243. doi:10.1016/j.biomaterials.2017.05.011
Annabi, N., Zhang, Y.-N., Assmann, A., Sani, E. S., Cheng, G., Lassaletta, A. D., et al. (2017). Engineering a Highly Elastic Human Protein-Based Sealant for Surgical Applications. Sci. Transl Med. 9 (410), eaai7466. doi:10.1126/scitranslmed.aai7466
Bai, S., Zhang, X., Cai, P., Huang, X., Huang, Y., Liu, R., et al. (2019). A Silk-Based Sealant with Tough Adhesion for Instant Hemostasis of Bleeding Tissues. Nanoscale Horizons 4 (6), 1333–1341. doi:10.1039/C9NH00317G
Behrens, A. M., Sikorski, M. J., and Kofinas, P. (2014). Hemostatic Strategies for Traumatic and Surgical Bleeding. J. Biomed. Mater. Res. A. 102 (11), 4182–4194. doi:10.1002/jbm.a.35052
Blajchman, M. A. (2003). Substitutes and Alternatives to Platelet Transfusions in Thrombocytopenic Patients. J. Thromb. Haemost. 1 (7), 1637–1641. doi:10.1046/j.1538-7836.2003.00332.x
Boulton, F. (2006). A Hundred Years of Cascading - Started by Paul Morawitz (1879-1936), a pioneer of Haemostasis and of Transfusion. Transfus. Med. 16 (1), 1–10. doi:10.1111/j.1365-3148.2006.00643.x
Brennan, M. J., Kilbride, B. F., Wilker, J. J., and Liu, J. C. (2017). A Bioinspired Elastin-Based Protein for a Cytocompatible Underwater Adhesive. Biomaterials 124, 116–125. doi:10.1016/j.biomaterials.2017.01.034
Bu, Y., Shen, H., Yang, F., Yang, Y., Wang, X., and Wu, D. (2017). Construction of Tough, In Situ Forming Double-Network Hydrogels with Good Biocompatibility. ACS Appl. Mater. Inter. 9 (3), 2205–2212. doi:10.1021/acsami.6b15364
Burke, K. A., Roberts, D. C., and Kaplan, D. L. (2016). Silk Fibroin Aqueous-Based Adhesives Inspired by Mussel Adhesive Proteins. Biomacromolecules 17 (1), 237–245. doi:10.1021/acs.biomac.5b01330
Chan, L. W., Wang, X., Wei, H., Pozzo, L. D., White, N. J., and Pun, S. H.. A Synthetic Fibrin Cross-Linking Polymer for Modulating Clot Properties and Inducing Hemostasis. Sci. Transl Med.. 2015;7(277):277ra29. doi:10.1126/scitranslmed.3010383
Chattopadhyay, S., and Raines, R. T. (2014). Review Collagen-Based Biomaterials for Wound Healing. Biopolymers 101 (8), 821–833. doi:10.1002/bip.22486
Chen, Y., Wu, L., Li, P., Hao, X., Yang, X., Xi, G., et al. (2020). Polysaccharide Based Hemostatic Strategy for Ultrarapid Hemostasis. Macromol Biosci. 20 (4), e1900370. doi:10.1002/mabi.201900370
Cheung, R., Ng, T., Wong, J., and Chan, W. (2015). Chitosan: An Update on Potential Biomedical and Pharmaceutical Applications. Mar. Drugs 13 (8), 5156–5186. doi:10.3390/md13085156
Choi, S. H., Smith, S. A., and Morrissey, J. H. (2011). Polyphosphate Is a Cofactor for the Activation of Factor XI by Thrombin. Blood 118 (26), 6963–6970. doi:10.1182/blood-2011-07-368811
Chou, T-C., Fu, E., Wu, C-J., and Yeh, J-H. (2003). Chitosan Enhances Platelet Adhesion and Aggregation. Biochem. Biophys. Res. Commun. 302 (3), 480–483. doi:10.1016/s0006-291x(03)00173-6
Deng, Y., Yang, X., Zhang, X., Cao, H., Mao, L., Yuan, M., et al. (2020). Novel Fenugreek Gum-Cellulose Composite Hydrogel with Wound Healing Synergism: Facile Preparation, Characterization and Wound Healing Activity Evaluation. Int. J. Biol. Macromolecules 160, 1242–1251. doi:10.1016/j.ijbiomac.2020.05.220
Desai, M. S., Chen, M., Hong, F. H. J., Lee, J. H., Wu, Y., and Lee, S-W. (2020). Catechol-Functionalized Elastin-like Polypeptides as Tissue Adhesives. Biomacromolecules 21 (7), 2938–2948. doi:10.1021/acs.biomac.0c00740
Farahani, M., and Shafiee, A.. Wound Healing: From Passive to Smart Dressings. Adv. Healthc. Mater. Published Online June 26, 2021e2100477. doi:10.1002/adhm.202100477
Gaharwar, A. K., Avery, R. K., Assmann, A., Paul, A, McKinley, G. H., Khademhosseini, A., et al. (2014). Shear-thinning Nanocomposite Hydrogels for the Treatment of Hemorrhage. ACS nano 8 (10). doi:10.1021/nn503719n
Gale, A. J. (2011). Continuing Education Course #2: Current Understanding of Hemostasis. Toxicol. Pathol. 39 (1), 273–280. doi:10.1177/0192623310389474
Graça, M. F. P., Miguel, S. P., Cabral, C. S. D., and Correia, I. J. (2020). Hyaluronic Acid—Based Wound Dressings: A Review. Carbohydr. Polym. 241, 116364. doi:10.1016/j.carbpol.2020.116364
Hagemann, J. H., Haegele, H., Müller, S., and Anders, H-J. (2013). Danger Control Programs Cause Tissue Injury and Remodeling. Int. J. Mol. Sci. 14 (6), 11319–11346. doi:10.3390/ijms140611319
Hashemnejad, S. M., and Kundu, S. (2019). Rheological Properties and Failure of Alginate Hydrogels with Ionic and Covalent Crosslinks. Soft Matter 15 (39), 7852–7862. doi:10.1039/c9sm01039d
Hemostatic wound dressings. Biotextiles as Medical Implants. Published online January 1, 2013:563–589. doi:10.1533/9780857095602.2.563
Hickman, D. A., Pawlowski, C. L., Sekhon, U. D. S., Marks, J., and Gupta, A. S. (2018). Biomaterials and Advanced Technologies for Hemostatic Management of Bleeding. Adv. Mater. 30 (4). doi:10.1002/adma.201700859
Hoekstra, M. J., Hermans, M. H. E., Richters, C. D., and Dutrieux, R. P. (2002). A Histological Comparison of Acute Inflammatory Responses with a Hydrofibre or Tulle Gauze Dressing. J. Wound Care 11 (3), 113–117. doi:10.12968/jowc.2002.11.3.26384
Hoffman, M. (2003). Remodeling the Blood Coagulation cascade. J. Thromb. Thrombolysis 16 (1-2), 17–20. doi:10.1023/B:THRO.0000014588.95061.28
Hong, Y., Zhou, F., Hua, Y., Zhang, X., Ni, C., Pan, D., et al. (2019). A Strongly Adhesive Hemostatic Hydrogel for the Repair of Arterial and Heart Bleeds. Nat. Commun. 10 (1), 2060. doi:10.1038/s41467-019-10004-7
Hu, W., Wang, Z., Xiao, Y., Zhang, S., and Wang, J. (2019). Advances in Crosslinking Strategies of Biomedical Hydrogels. Biomater. Sci. 7 (3), 843–855. doi:10.1039/C8BM01246F
Huang, W., Cheng, S., Wang, X., Zhang, Y., Chen, L., and Zhang, L. (2021). Noncompressible Hemostasis and Bone Regeneration Induced by an Absorbable Bioadhesive Self‐Healing Hydrogel. Adv. Funct. Mater. 31 (22), 2009189. doi:10.1002/adfm.202009189
Huang, W., Ling, S., Li, C., Omenetto, F. G., and Kaplan, D. L. (2018). Silkworm Silk-Based Materials and Devices Generated Using Bio-Nanotechnology. Chem. Soc. Rev. 47 (17), 6486–6504. doi:10.1039/c8cs00187a
Huang, W., Wang, Y., Chen, Y., Zhao, Y., Zhang, Q., Zheng, X., et al. (2016). Strong and Rapidly Self-Healing Hydrogels: Potential Hemostatic Materials. Adv. Healthc. Mater 5 (21), 2813–2822. doi:10.1002/adhm.201600720
Huang, X., Fu, Q., Deng, Y., Wang, F., Xia, B., Chen, Z., et al. (2021). Surface Roughness of Silk Fibroin/alginate Microspheres for Rapid Hemostasis In Vitro and In Vivo. Carbohydr. Polym. 253, 117256. doi:10.1016/j.carbpol.2020.117256
Jayakumar, R., Prabaharan, M., Sudheesh Kumar, P. T., Nair, S. V., and Tamura, H. (2011). Biomaterials Based on Chitin and Chitosan in Wound Dressing Applications. Biotechnol. Adv. 29 (3), 322–337. doi:10.1016/j.biotechadv.2011.01.005
Jones, R. E., Foster, D. S., and Longaker, M. T. (2018). Management of Chronic Wounds-2018. JAMA 320 (14), 1481–1482. doi:10.1001/jama.2018.12426
Khan, M. A., and Mujahid, M. (2019). A Review on Recent Advances in Chitosan Based Composite for Hemostatic Dressings. Int. J. Biol. Macromol 124, 138–147. doi:10.1016/j.ijbiomac.2018.11.045
Khunmanee, S., Jeong, Y., and Park, H. (2017). Crosslinking Method of Hyaluronic-Based Hydrogel for Biomedical Applications. J. Tissue Eng. 8, 2041731417726464. doi:10.1177/2041731417726464
Kielty, C. M., Sherratt, M. J., and Shuttleworth, C. A. (2002). Elastic Fibres. J. Cel Sci 115 (Pt 14), 2817–2828. doi:10.1242/jcs.115.14.2817
Kong, Y., Hou, Z., Zhou, L., Zhang, P., Ouyang, Y., Wang, P., et al. (2021). Injectable Self-Healing Hydrogels Containing CuS Nanoparticles with Abilities of Hemostasis, Antibacterial Activity, and Promoting Wound Healing. ACS Biomater. Sci. Eng. 7 (1), 335–349. doi:10.1021/acsbiomaterials.0c01473
Kozel, B. A., and Mecham, R. P. (2019). Elastic Fiber Ultrastructure and Assembly. Matrix Biol. 84, 31–40. doi:10.1016/j.matbio.2019.10.002
Kragh, J. F., Swan, K. G., Smith, D. C., Mabry, R. L., and Blackbourne, L. H. (2012). Historical Review of Emergency Tourniquet Use to Stop Bleeding. Am. J. Surg. 203 (2), 242–252. doi:10.1016/j.amjsurg.2011.01.028
Lee, K. Y., and Mooney, D. J. (2012). Alginate: Properties and Biomedical Applications. Prog. Polym. Sci. 37 (1), 106–126. doi:10.1016/j.progpolymsci.2011.06.003
Lei, C., Zhu, H., Li, J., Feng, X., and Chen, J. (2016). Preparation and Hemostatic Property of Low Molecular Weight Silk Fibroin. J. Biomater. Sci. Polym. Ed. 27 (5), 403–418. doi:10.1080/09205063.2015.1136918
Leonard, J., Zietlow, J., Morris, D., Berns, K., Eyer, S., Martinson, K., et al. (2016). A Multi-Institutional Study of Hemostatic Gauze and Tourniquets in Rural Civilian Trauma. J. Trauma Acute Care Surg. 81 (3), 441–444. doi:10.1097/TA.0000000000001115
Li, J., Celiz, A. D., Yang, J., Yang, Q., Wamala, I., Whyte, W., et al. (2017). Tough Adhesives for Diverse Wet Surfaces. Science 357 (6349), 378–381. doi:10.1126/science.aah6362
Lih, E., Lee, J. S., Park, K. M., and Park, K. D. (2012). Rapidly Curable Chitosan-PEG Hydrogels as Tissue Adhesives for Hemostasis and Wound Healing. Acta Biomater. 8 (9), 3261–3269. doi:10.1016/j.actbio.2012.05.001
Liu, B., Wang, Y., Miao, Y., Zhang, X., Fan, Z., Singh, G., et al. (2018). Hydrogen Bonds Autonomously Powered Gelatin Methacrylate Hydrogels with Super-elasticity, Self-Heal and Underwater Self-Adhesion for Sutureless Skin and Stomach Surgery and E-Skin. Biomaterials 171, 83–96. doi:10.1016/j.biomaterials.2018.04.023
Liu, C., Shi, Z., Sun, H., Mujuni, C. J., Zhao, L., Wang, X., et al. (2020). Preparation and Characterization of Tissue-Factor-Loaded Alginate: Toward a Bioactive Hemostatic Material. Carbohydr. Polym. 249, 116860. doi:10.1016/j.carbpol.2020.116860
Löwik, D. W. P. M., Leunissen, E. H. P., van den Heuvel, M., Hansen, M. B., and van Hest, J. C. M. (2010). Stimulus Responsive Peptide Based Materials. Chem. Soc. Rev. 39 (9), 3394–3412. doi:10.1039/b914342b
Luo, J-W., Liu, C., Wu, J-H., Lin, L.-X., Fan, H.-M., Zhao, D.-H., et al. (2019). In Situ injectable Hyaluronic Acid/gelatin Hydrogel for Hemorrhage Control. Mater. Sci. Eng. C Mater. Biol. Appl. 98, 628–634. doi:10.1016/j.msec.2019.01.034
Ma, D., Wang, Y., and Dai, W. (2018). Silk Fibroin-Based Biomaterials for Musculoskeletal Tissue Engineering. Mater. Sci. Eng. C Mater. Biol. Appl. 89, 456–469. doi:10.1016/j.msec.2018.04.062
Maham, A., Tang, Z., Wu, H., Wang, J., and Lin, Y. (2009). Protein-based Nanomedicine Platforms for Drug Delivery. Small 5 (15), 1706–1721. doi:10.1002/smll.200801602
Makris, M., Calizzani, G., Fischer, K., Gilman, E. A., Hay, C. R. M., R., Lassila, et al. (2011). EUHASS: The European Haemophilia Safety Surveillance System. Thromb. Res. 127, S22–S25. doi:10.1016/S0049-3848(10)70150-X
Malette, W. G., Quigley, H. J., Gaines, R. D., Johnson, N. D., and Rainer, W. G. (1983). Chitosan: a New Hemostatic. Ann. Thorac. Surg. 36 (1), 55–58. doi:10.1016/s0003-4975(10)60649-2
Mano, J. F., Silva, G. A., Azevedo, H. S., Malafaya, P. B., Sousa, R. A., Silva, S. S., et al. (2007). Natural Origin Biodegradable Systems in Tissue Engineering and Regenerative Medicine: Present Status and Some Moving Trends. J. R. Soc. Interf. 4 (17), 999–1030. doi:10.1098/rsif.2007.0220
Marietta, M., Facchini, L., Pedrazzi, P., Busani, S., and Torelli, G. (2006). Pathophysiology of Bleeding in Surgery. Transpl. Proc 38 (3), 812–814. doi:10.1016/j.transproceed.2006.01.047
Matsuda, T., Kawakami, R., Namba, R., Nakajima, T., and Gong, J. P. (2019). Mechanoresponsive Self-Growing Hydrogels Inspired by Muscle Training. Science 363 (6426), 504–508. doi:10.1126/science.aau9533
Mendes, B. B., Gómez-Florit, M., Araújo, A. C., Prada, J., Babo, P. S., Domingues, R. M. A., et al. (2020). Intrinsically Bioactive Cryogels Based on Platelet Lysate Nanocomposites for Hemostasis Applications. Biomacromolecules 21 (9), 3678–3692. doi:10.1021/acs.biomac.0c00787
Montaser, A. S., Rehan, M., El-Senousy, W. M., and Zaghloul, S. (2020). Designing Strategy for Coating Cotton Gauze Fabrics and its Application in Wound Healing. Carbohydr. Polym. 244, 116479. doi:10.1016/j.carbpol.2020.116479
Morrissey, J. H. (2012). Polyphosphate: a Link between Platelets, Coagulation and Inflammation. Int. J. Hematol. 95 (4), 346–352. doi:10.1007/s12185-012-1054-5
Müller, F., Mutch, N. J., Schenk, W. A., Smith, S. A., Esterl, L., Spronk, H. M., et al. (2009). Platelet Polyphosphates Are Proinflammatory and Procoagulant Mediators In Vivo. Cell 139 (6), 1143–1156. doi:10.1016/j.cell.2009.11.001
Mutch, N. J., Engel, R., Uitte de Willige, S., Philippou, H., and Ariëns, R. A. S. (2010). Polyphosphate Modifies the Fibrin Network and Down-Regulates Fibrinolysis by Attenuating Binding of tPA and Plasminogen to Fibrin. Blood 115 (19), 3980–3988. doi:10.1182/blood-2009-11-254029
Narayanan, A., Menefee, J. R., Liu, Q., Dhinojwala, A., and Joy, A. (2020). Lower Critical Solution Temperature-Driven Self-Coacervation of Nonionic Polyester Underwater Adhesives. ACS Nano 14 (7), 8359–8367. doi:10.1021/acsnano.0c02396
Neubauer, K., and Zieger, B.. Endothelial Cells and Coagulation. Cell Tissue Res. Published online May 20, 2021. doi:10.1007/s00441-021-03471-2
Nguyen, P. Q., Courchesne, N-M. D., Duraj-Thatte, A., Praveschotinunt, P., and Joshi, N. S. (2018). Engineered Living Materials: Prospects and Challenges for Using Biological Systems to Direct the Assembly of Smart Materials. Adv. Mater. 30 (19), e1704847. doi:10.1002/adma.201704847
Preman, N. K., E S, S. P., Prabhu, A., Shaikh, S. B., C, V., Barki, R. R., et al. (2020). Bioresponsive Supramolecular Hydrogels for Hemostasis, Infection Control and Accelerated Dermal Wound Healing. J. Mater. Chem. B 8 (37), 8585–8598. doi:10.1039/d0tb01468k
Qu, J., Zhao, X., Liang, Y., Zhang, T., Ma, P. X., and Guo, B. (2018). Antibacterial Adhesive Injectable Hydrogels with Rapid Self-Healing, Extensibility and Compressibility as Wound Dressing for Joints Skin Wound Healing. Biomaterials 183, 185–199. doi:10.1016/j.biomaterials.2018.08.044
Rao, P., Sun, T. L., Chen, L., Takahashi, R., Shinohara, G., Guo, H., et al. (2018). Tough Hydrogels with Fast, Strong, and Reversible Underwater Adhesion Based on a Multiscale Design. Adv. Mater. 30 (32), e1801884. doi:10.1002/adma.201801884
Reakasame, S., and Boccaccini, A. R. (2018). Oxidized Alginate-Based Hydrogels for Tissue Engineering Applications: A Review. Biomacromolecules 19 (1), 3–21. doi:10.1021/acs.biomac.7b01331
Reichheld, S. E., Muiznieks, L. D., Lu, R., Sharpe, S., and Keeley, F. W. (2019). Sequence Variants of Human Tropoelastin Affecting Assembly, Structural Characteristics and Functional Properties of Polymeric Elastin in Health and Disease. Matrix Biol. 84, 68–80. doi:10.1016/j.matbio.2019.06.010
Ren, J., Yin, X., Chen, Y., Chen, Y., Su, H., Wang, K., et al. (2020). Alginate Hydrogel-Coated Syringe needles for Rapid Haemostasis of Vessel and Viscera Puncture. Biomaterials 249, 120019. doi:10.1016/j.biomaterials.2020.120019
Rizzo, F., and Kehr, N. S. (2021). Recent Advances in Injectable Hydrogels for Controlled and Local Drug Delivery. Adv. Healthc. Mater 10 (1), 2001341. doi:10.1002/adhm.202001341
Roberts, H. R., Hoffman, M., and Monroe, D. M. (2006). A Cell-Based Model of Thrombin Generation. Semin. Thromb. Hemost. 32 (Suppl. 1), 32–38. doi:10.1055/s-2006-939552
Ruggeri, Z. M., and Mendolicchio, G. L. (2007). Adhesion Mechanisms in Platelet Function. Circ. Res. 100 (12), 1673–1685. doi:10.1161/01.RES.0000267878.97021.ab
Ruiz, F. A., Lea, C. R., Oldfield, E., and Docampo, R. (2004). Human Platelet Dense Granules Contain Polyphosphate and Are Similar to Acidocalcisomes of Bacteria and Unicellular Eukaryotes. J. Biol. Chem. 279 (43), 44250–44257. doi:10.1074/jbc.M406261200
Ryu, J. H., Hong, S., and Lee, H. (2015). Bio-inspired Adhesive Catechol-Conjugated Chitosan for Biomedical Applications: A Mini Review. Acta Biomater. 27, 101–115. doi:10.1016/j.actbio.2015.08.043
Sakoda, M., Kaneko, M., Ohta, S., Qi, P., Ichimura, S., Yatomi, Y., et al. (2018). Injectable Hemostat Composed of a Polyphosphate-Conjugated Hyaluronan Hydrogel. Biomacromolecules 19 (8), 3280–3290. doi:10.1021/acs.biomac.8b00588
Sen, S., Basak, P., Prasad Sinha, B., Maurye, P., Jaiswal, K. K., Das, P., et al. (2020). Anti-inflammatory Effect of Epidermal Growth Factor Conjugated Silk Fibroin Immobilized Polyurethane Ameliorates Diabetic Burn Wound Healing. Int. J. Biol. Macromol 143, 1009–1032. doi:10.1016/j.ijbiomac.2019.09.219
Serban, M. A., Panilaitis, B., and Kaplan, D. L. (2011). Silk Fibroin and Polyethylene Glycol-Based Biocompatible Tissue Adhesives. J. Biomed. Mater. Res. A. 98 (4), 567–575. doi:10.1002/jbm.a.33149
Shefa, A. A., Taz, M., Lee, S. Y., and Lee, B-T. (2019). Enhancement of Hemostatic Property of Plant Derived Oxidized Nanocellulose-Silk Fibroin Based Scaffolds by Thrombin Loading. Carbohydr. Polym. 208, 168–179. doi:10.1016/j.carbpol.2018.12.056
Silva, N. H. C. S., Vilela, C., Marrucho, I. M., Freire, C. S. R., Pascoal Neto, C., and Silvestre, A. J. D. (2014). Protein-based Materials: from Sources to Innovative Sustainable Materials for Biomedical Applications. J. Mater. Chem. B 2 (24), 3715–3740. doi:10.1039/c4tb00168k
Song, C. K., Kim, M-K., Lee, J., Davaa, E., Baskaran, R., and Yang, S-G. (2019). Dopa-Empowered Schiff Base Forming Alginate Hydrogel Glue for Rapid Hemostatic Control. Macromol Res. 27 (2), 119–125. doi:10.1007/s13233-019-7026-3
Sun, J., Su, J., Ma, C., Göstl, R., Herrmann, A., Liu, K., et al. (2020). Fabrication and Mechanical Properties of Engineered Protein‐Based Adhesives and Fibers. Adv. Mater. 32 (6), 1906360. doi:10.1002/adma.201906360
Tang, Q., Chen, C., Jiang, Y., Huang, J., Liu, Y., Nthumba, P. M., et al. (2020). Engineering an Adhesive Based on Photosensitive Polymer Hydrogels and Silver Nanoparticles for Wound Healing. J. Mater. Chem. B 8 (26), 5756–5764. doi:10.1039/d0tb00726a
Tavakoli, S., Kharaziha, M., Nemati, S., and Kalateh, A. (2021). Nanocomposite Hydrogel Based on Carrageenan-Coated Starch/cellulose Nanofibers as a Hemorrhage Control Material. Carbohydr. Polym. 251, 117013. doi:10.1016/j.carbpol.2020.117013
Tomeh, M. A., Hadianamrei, R., and Zhao, X. (2019). Silk Fibroin as a Functional Biomaterial for Drug and Gene Delivery. Pharmaceutics 11 (10), E494. doi:10.3390/pharmaceutics11100494
Vakalopoulos, K. A., Wu, Z., Kroese, L. F., van der Horst, P. H., Lam, K. H., Dodou, D., et al. (2017). Clinical, Mechanical, and Immunohistopathological Effects of Tissue Adhesives on the colon: An Iin-Vvivo Study. J. Biomed. Mater. Res. B Appl. Biomater. 105 (4), 846–854. doi:10.1002/jbm.b.33621
Varga-Szabo, D., Pleines, I., and Nieswandt, B. (2008). Cell Adhesion Mechanisms in Platelets. Arterioscler Thromb. Vasc. Biol. 28 (3), 403–412. doi:10.1161/ATVBAHA.107.150474
Versteeg, H. H., Heemskerk, J. W. M., Levi, M., and Reitsma, P. H. (2013). New Fundamentals in Hemostasis. Physiol. Rev. 93 (1), 327–358. doi:10.1152/physrev.00016.2011
Vindin, H., Mithieux, S. M., and Weiss, A. S. (2019). Elastin Architecture. Matrix Biol. 84, 4–16. doi:10.1016/j.matbio.2019.07.005
Wang, C., Niu, H., Ma, X., Hong, H., Yuan, Y., and Liu, C. (2019). Bioinspired, Injectable, Quaternized Hydroxyethyl Cellulose Composite Hydrogel Coordinated by Mesocellular Silica Foam for Rapid, Noncompressible Hemostasis and Wound Healing. ACS Appl. Mater. Inter. 11 (38), 34595–34608. doi:10.1021/acsami.9b08799
Wang, C-S., Ashton, N. N., Weiss, R. B., and Stewart, R. J. (2014). Peroxinectin Catalyzed Dityrosine Crosslinking in the Adhesive Underwater Silk of a Casemaker Caddisfly Larvae, Hysperophylax Occidentalis. Insect Biochem. Mol. Biol. 54, 69–79. doi:10.1016/j.ibmb.2014.08.009
Wang, L., Zhang, X., Yang, K., Fu, Y. V., Xu, T., Li, S., et al. (2020). A Novel Double‐Crosslinking‐Double‐Network Design for Injectable Hydrogels with Enhanced Tissue Adhesion and Antibacterial Capability for Wound Treatment. Adv. Funct. Mater. 30 (1), 1904156. doi:10.1002/adfm.201904156
Wang, M., Hao, H., Leeper, N. J., and Zhu, L. (2018). Early Career Committee. Thrombotic Regulation from the Endothelial Cell Perspectives. Arterioscler Thromb. Vasc. Biol. 38 (6), e90–e95. doi:10.1161/ATVBAHA.118.310367
Wang, X. X., Liu, Q., Sui, J. X., Ramakrishna, S., Yu, M., Zhou, Y., et al. (2019). Recent Advances in Hemostasis at the Nanoscale. Adv. Healthc. Mater. 8 (23), e1900823. doi:10.1002/adhm.201900823
Wang, Z., Hu, W., Du, Y., Xiao, Y., Wang, X., Zhang, S., et al. (2020). Green Gas-Mediated Cross-Linking Generates Biomolecular Hydrogels with Enhanced Strength and Excellent Hemostasis for Wound Healing. ACS Appl. Mater. Inter. 12 (12), 13622–13633. doi:10.1021/acsami.9b21325
Wise, S. G., and Weiss, A. S. (2009). Tropoelastin. Int. J. Biochem. Cel Biol. 41 (3), 494–497. doi:10.1016/j.biocel.2008.03.017
Woolfson, D. N., and Mahmoud, Z. N. (2010). More Than Just Bare Scaffolds: towards Multi-Component and Decorated Fibrous Biomaterials. Chem. Soc. Rev. 39 (9), 3464–3479. doi:10.1039/c0cs00032a
Xiao, G., Wang, Y., Zhang, H., Chen, L., and Fu, S. (2019). Facile Strategy to Construct a Self-Healing and Biocompatible Cellulose Nanocomposite Hydrogel via Reversible Acylhydrazone. Carbohydr. Polym. 218, 68–77. doi:10.1016/j.carbpol.2019.04.080
Xuan, C., Hao, L., Liu, X., Zhu, Y., Yang, H., Ren, Y., et al. (2020). Wet-adhesive, Haemostatic and Antimicrobial Bilayered Composite Nanosheets for Sealing and Healing Soft-Tissue Bleeding Wounds. Biomaterials 252, 120018. doi:10.1016/j.biomaterials.2020.120018
Xue, H., Hu, L., Xiong, Y., Zhu, X., Wei, C., Cao, F., et al. (2019). Quaternized Chitosan-Matrigel-Polyacrylamide Hydrogels as Wound Dressing for Wound Repair and Regeneration. Carbohydr. Polym. 226, 115302. doi:10.1016/j.carbpol.2019.115302
Yang, X., Liu, W., Li, N., Wang, M., Liang, B., Ullah, I., et al. (2017). Design and Development of Polysaccharide Hemostatic Materials and Their Hemostatic Mechanism. Biomater. Sci. 5 (12), 2357–2368. doi:10.1039/c7bm00554g
Yuk, H., Varela, C. E., Nabzdyk, C. S., Mao, X., Padera, R. F., Roche, E. T., et al. (2019). Dry Double-Sided Tape for Adhesion of Wet Tissues and Devices. Nature 575 (7781), 169–174. doi:10.1038/s41586-019-1710-5
Zhang, S., Li, J., Chen, S., Zhang, X., Ma, J., and He, J. (2020). Oxidized Cellulose-Based Hemostatic Materials. Carbohydr. Polym. 230, 115585. doi:10.1016/j.carbpol.2019.115585
Zhang, X., Malhotra, S., Molina, M., and Haag, R. (2015). Micro- and Nanogels with Labile Crosslinks – from Synthesis to Biomedical Applications. Chem. Soc. Rev. 44 (7), 1948–1973. doi:10.1039/C4CS00341A
Zhang, Z., Zhang, L., Li, C., Xie, X., Li, G., Hu, Z., et al. (2021). Research Progress of Chitosan-Based Biomimetic Materials. Mar. Drugs 19 (7), 372. doi:10.3390/md19070372
Zhao, X., Guo, B., Wu, H., Liang, Y., and Ma, P. X. (2018). Injectable Antibacterial Conductive Nanocomposite Cryogels with Rapid Shape Recovery for Noncompressible Hemorrhage and Wound Healing. Nat. Commun. 9 (1), 2784. doi:10.1038/s41467-018-04998-9
Zhao, X., Wu, H., Guo, B., Dong, R., Qiu, Y., and Ma, P. X. (2017). Antibacterial Anti-oxidant Electroactive Injectable Hydrogel as Self-Healing Wound Dressing with Hemostasis and Adhesiveness for Cutaneous Wound Healing. Biomaterials 122, 34–47. doi:10.1016/j.biomaterials.2017.01.011
Zhong, Y., Hu, H., Min, N., Wei, Y., Li, X., and Li, X. (2021). Application and Outlook of Topical Hemostatic Materials: a Narrative Review. Ann. Transl Med. 9 (7), 577. doi:10.21037/atm-20-7160
Zhou, Z., Zhang, S., Cao, Y., Marelli, B., Xia, X., and Tao, T. H. (2018). Engineering the Future of Silk Materials through Advanced Manufacturing. Adv. Mater. Published Online June 28, e1706983. doi:10.1002/adma.201706983
Zhu, J., Li, F., Wang, X., Yu, J., and Wu, D. (2018). Hyaluronic Acid and Polyethylene Glycol Hybrid Hydrogel Encapsulating Nanogel with Hemostasis and Sustainable Antibacterial Property for Wound Healing. ACS Appl. Mater. Inter. 10 (16), 13304–13316. doi:10.1021/acsami.7b18927
Zhu, W., Chuah, Y. J., and Wang, D-A. (2018). Bioadhesives for Internal Medical Applications: A Review. Acta Biomater. 74, 1–16. doi:10.1016/j.actbio.2018.04.034
Keywords: hydrogel, hemostasis, wound healing, nature-derived polysaccharides, nature-derived proteins
Citation: Cheng J, Liu J, Li M, Liu Z, Wang X, Zhang L and Wang Z (2021) Hydrogel-Based Biomaterials Engineered from Natural-Derived Polysaccharides and Proteins for Hemostasis and Wound Healing. Front. Bioeng. Biotechnol. 9:780187. doi: 10.3389/fbioe.2021.780187
Received: 20 September 2021; Accepted: 25 October 2021;
Published: 22 November 2021.
Edited by:
Da Huang, Missouri University of Science and Technology, United StatesReviewed by:
Daoyang Fan, Peking University Third Hospital, ChinaChao Zhao, University of Alabama, United States
Shuai Xu, Peking University People’s Hospital, China
Copyright © 2021 Cheng, Liu, Li, Liu, Wang, Zhang and Wang. This is an open-access article distributed under the terms of the Creative Commons Attribution License (CC BY). The use, distribution or reproduction in other forums is permitted, provided the original author(s) and the copyright owner(s) are credited and that the original publication in this journal is cited, in accordance with accepted academic practice. No use, distribution or reproduction is permitted which does not comply with these terms.
*Correspondence: Xing Wang, d2FuZ3hpbmdAaWNjYXMuYWMuY24=; Licheng Zhang, emhhbmdsY2hlbmcyMThAMTI2LmNvbQ==; Zheng Wang, d3pzcGluZUAxNjMuY29t