- 1Polish Stem Cell Bank, FamiCord Group, Warsaw, Poland
- 2Department of Immunology, Faculty of Biology, University of Warsaw, Warsaw, Poland
- 3Clinic of Paediatric Neurology, III Faculty of Paediatrics, Medical University of Lublin, Lublin, Poland
- 4Institute of Human Genetics, Polish Academy of Sciences, Poznan, Poland
- 5Laboratory for Cell Research and Application, Medical University of Warsaw, Warsaw, Poland
Processing of MSCs to obtain a therapeutic product consists of two main steps: 1) the in vitro expansion of the cells until an appropriate number of them is obtained, and 2) freezing and storage of the expanded cells. The last step is critical and must be optimized so that after thawing the cells retain all their physiological properties including the secretory function. In this paper, we evaluated physiological parameters of AT-MSC’s after a full cycle of their processing, particularly freezing and storing at the liquid nitrogen vapor temperature. Based on the recovered proliferative and secretory capacities of the thawed cells, we have designed the optimal technique for processing of MSCs for clinical applications. In our work, we tried to select the best DMSO-based cryoprotectant mixture on the base of post thawing fully retain their properties. We have demonstrated the effectiveness of the use of DMSO in various configurations of the constituent cryoprotective fluids. We have also shown that AT-MSCs that show control levels in most standard tests (viability, shape, culture behaviour, and proliferative properties) after thawing, may show transient variations in some important physiological properties, such as the level of secreted growth factors. Obtained results let us to indicate how to optimize the AT-MSC preparation process for clinical applications. We suggest that before their clinical application the cells should be cultured for at least one passage to recover their physiological stability and thus assure their optimal therapeutic potential.
Introduction
Adipose tissue-derived mesenchymal stromal cells (AT-MSCs) and MSCs from other sources possess such attributes as adherence to surfaces, no surface expression of the CD14, CD19, CD34, CD45, HLA-DR antigens, expression of the CD73, CD90, CD105 antigens and the capacity to undergo adipogenic, osteogenic and chondrogenic differentiation (Dominici et al., 2006). MSCs secrete various growth factors, such as tumor necrosis factor-alpha (TNF-α), transforming growth factor-beta (TGF-β), vascular endothelial growth factor (VEGF) and hepatocyte growth factor (HGF), chemokines MCP-1 and IL-8, and cytokines IL-3, IL-5 and IL-6 (Salgado et al., 2010; Carmeliet and Jain, 2011; Doorn et al., 2012; Reinders et al., 2013; Schepers and Fibbe, 2016). MSCs cells also tend to migrate to the sites of injury or inflammation (Spaeth et al., 2008; Rustad and Gurtner, 2012; Ullah et al., 2019). Due to these unique properties AT-MSCs are widely used in regenerative medicine and other clinical applications (Wang et al., 2012; Kim and Cho, 2013; Semenova et al., 2018; Han et al., 2019; Pittenger et al., 2019). The therapeutic mechanism of these cells has not been fully elucidated, but is likely associated with either direct interaction between MSCs and the recipient cells or stimulation of the recipient tissue by soluble factors secreted by MSCs. In the former case, the main role is played by the MSCs’ capacity to differentiate into many different types of cells. In the latter case, the secretory function of MSCs is likely to play a major role.
Processing of MSCs to obtain a therapeutic product consists of two main steps: 1) the in vitro expansion of the cells until an appropriate number of them is obtained, and 2) freezing and storage of the expanded cells. The last step is critical and must be optimized so that after thawing the cells retain all their physiological properties including the secretory function. Of crucial importance is maintenance of the freezing rate at about 1°C per minute as is the appropriate selection of a cryoprotective solution. The latter should effectively prevent formation of ice crystals inside and outside of the cells, keep them viable during the freezing and storing and save them physiological properties intact after thawing. For all these purposes dimethyl sulfoxide (DMSO) seems to be the agent of choice (Davis et al., 1990; Stroncek et al., 1991; Lane and Gardner, 2005; Benkhalifa et al., 2014; Cantero Peral et al., 2015; Truong et al., 2016; Kang et al., 2017).
In this paper, we evaluated physiological parameters of AT-MSC’s after a full cycle of their processing, particularly freezing and storing at the liquid nitrogen vapor temperature. Based on the recovered proliferative and secretory capacities of the thawed cells, we have designed the optimal technique for processing of MSCs for clinical applications.
Materials and Methods
Cell Isolation and Culture
Adipose tissue samples were collected in accordance with a protocol approved by the Polish Ministry of Health and proceeded within 24 h after the collection. The samples were obtained anonymously from healthy volunteers (n = 5) by liposuction. Every sample of adipose tissue (lipoaspirate) was 100–150 ml of capacity. The lipoaspirate was washed several times until a clear yellow tissue was obtained. The tissue was then digested with 0.1% collagenase NB 6 (SERVA) for about 30–60 min at 37°C with a rotation (depending on the quality of the lipoaspirate and content of adipose tissue in it, the incubation time varies. The criterion for interrupting the digestion process is the achievement of the appropriate consistency and homogeneity by the processed lipoaspirate). The obtained suspension was centrifuged (300 g, 7 min) to remove the collagenase and to isolate the stromal vascular fraction (SVF), the heterogeneous cell population consisting with preadipocytes, macrophages, lymphocytes, endothelial cells, AT-MSC and many others. SVF was then suspended in StemMACS™ human MSC Expansion Media Kit XF (Miltenyi Biotec) supplemented with antibiotics/antimicotics (Thermo Fisher) and adhered AT-MSCs were expanded in the 75 cm2 flasks (NUNC, Thermo Scientific) at 37°C, 5% CO2 and 90% humidity. AT-MSCs were cultured in these standard conditions until passage 3, which was used in further experiments.
Freezing and Thawing Procedures
The freezing procedure was performed using the IceCube device (Sylab). Four DMSO-based cryoprotectants were selected for our purposes: Cryo1—commercially available from Biological Industries; Cryo2—commercially available from ZENOAQ/AMSBIO; Cry3—composed of 10% DMSO, dextran40 in NaCl and 5% human albumin Alburex 5 (CSL Bechring) (Gao and Critser, 2000; Fuller, 2004); Cryo4—composed of 10% DMSO in Alburex 5.
For the experiments AT-MSCs from the third passage (P3) were used. The cells were frozen at the density of 5 × 10⁶ cell/ml using our own freezing program formulated for the 2–4.5 ml tubes (Figure 1).
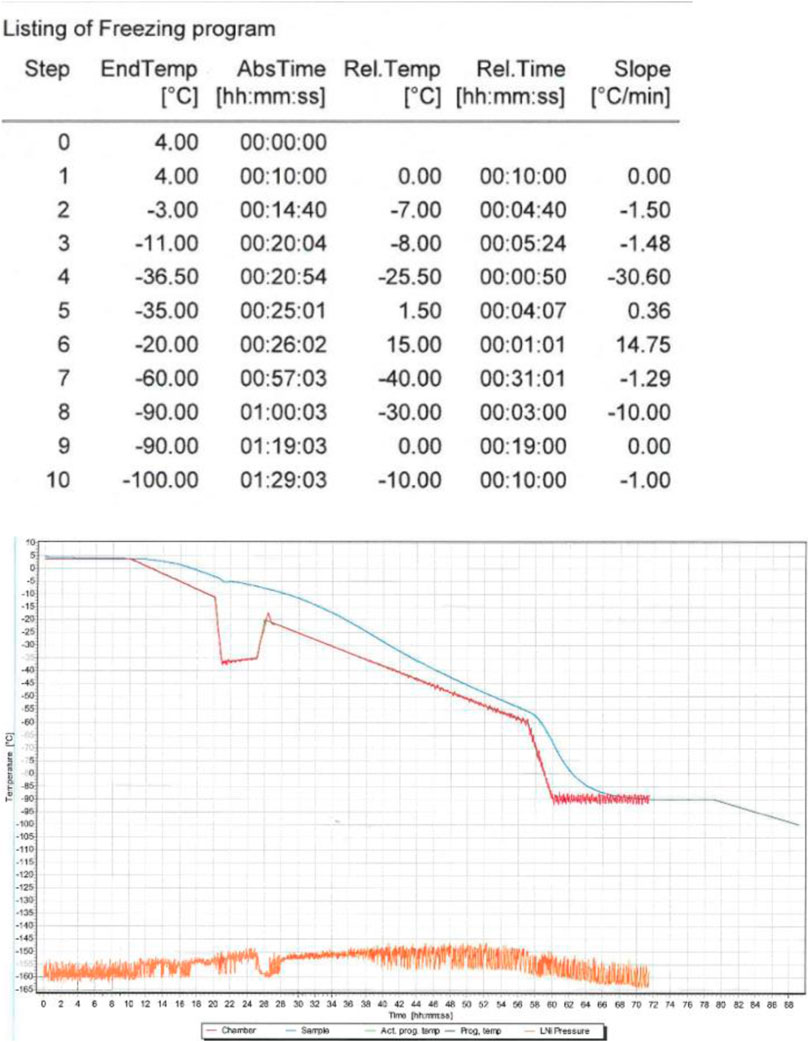
FIGURE 1. The freezing procedure was implemented using the IceCube device. The freezing program started at 4°C and finished at −80°C. After freezing AT-MSCs were transferred to cryogenic containers (−170°C).
After an arbitrarily selected weekly cryopreservation period, all samples were placed in the 37°C water bath for rapid thawing until no ice was detectable. The thawed samples were slowly dissolved in the culture medium (1:20) and after centrifugation the supernatant containing DMSO was removed. The obtained cells were suspended in the medium using the same seeding density and cultured at 37°C, 5% CO2 and 90% humidity in the 75 cm2 flasks (NUNC, Thermo Scientific) until the 5th passage. Non-frozen AT-MSCs from passages 4 and 5 were used as control.
Flow Cytometry Analysis
The phenotypic profile of AT-MSCs was determined using the PE-conjugated antibodies against human antigens CD73 (catalog no. 550257, BD Pharmingen™, BD Bioscence United States), CD90 (catalog no. 561970, BD Pharmingen™, BD Bioscence United States) and CD105 (catalog no. 560839, BD Pharmingen™, BD Bioscence United States) and using the FITC-conjugated antibodies against human antigens (later referred as Lin cocktail) HLA-DR (catalog no. 555811, BD Pharmingen™, BD Bioscence United States), CD34 (catalog no. 345801, BD Pharmingen™, BD Bioscence United States), CD45 (catalog no. 345808, BD Pharmingen™, BD Bioscence United States), CD 19 (catalog no. 345788, BD Pharmingen™, BD Bioscence United States) and CD14 (catalog no. 345784, BD Pharmingen™, BD Bioscence United States) (BD Bioscience) according to protocol. At least 10 × 103 cells per sample were analyzed in a FACSCalibur cytometer (BD Bioscience). The results were analysed with use of the CellQuest Pro v.6.0 software (BD Bioscence).
Proliferative Potential—The PDT and CFU-F Assays
The proliferative potential of the cells was evaluated using the population doubling time (PDT) test. The results were calculated using the following formula: PDT = (CT*Ln2)/ln (Nf/Ni), where CT is the cell-culture time, Nf—the final number of cells, and Ni—the initial number of cells.
The colony-forming unit-fibroblast (CFU-F) assay was used for estimation of the cells’ proliferation capacity. The cells from each sample suspended in MACS medium were placed in the 12-well culture plates (NUNC, Thermo Scientific) at 500 cells/well. On day 5 of the culture the cells were fixed with 4% PFA (CHEMLAND) and stained with crystal violet (Gibco). Colonies containing at least 50 cells were counted.
Post-Thaw Cell Viability
The frozen cells were thawed in the 37°C water bath, centrifuged (300 g, 7 min) to remove the remaining cryoprotectant and suspended in the MACS medium. After 30 min of incubation at 37°C to restore their membranes the cells’ viability was estimated using the Trypan Blue test and the Nikon Eclipse E 200 (Nikon) light microscope at ×20 magnification. Using hemocytometer chamber all living and dead cells are counted and the alive are presented as a percentage of all cells.
Cytoskeleton Analysis
Cell suspensions obtained from both fresh and frozen/thawed P4 cells were seeded in the 6-well culture plates (NUNC, Thermo Scientific) at 1 × 104 cells per well and cultured until about 60% confluency. The coverslips were removed and the attached cells were fixed with 4% PFA. The cells were then permeabilized with Triton X-100 (Merck) and blocked with 1% BSA in the phosphate-buffered saline (PBS) for 30 min. The obtained cell suspensions were incubated with the primary antibody tagged with the TRITC-conjugated Phalloidin (Merck) for 60 min at room temperature, washed with a washing buffer (1xPBS containing 0.05% Tween-20) and incubated with the FITC-conjugated secondary antibody for 60 min. To stain the nuclei, the cells were additionally incubated with DAPI for 5 min at room temperature. The cell preparations were then examined under the Leica DMi8 (Leica) microscope.
Multilineage Differentiation and Staining
The P4 cells were seeded at 5 × 10³ cells/cm2 onto the wells of the 24-well plate (BD Falcon), grown to the 50% confluence and then cultured in the following media (Thermo Fisher): 1) the StemPro Adipogenesis Differentiation Medium for the evaluation of the cells’ adipogenic potential, 2) the StemPro Chondrogenesis Differentiation Medium for the evaluation of the chondrogenic potential, and 3) the StemPro Osteogenesis Differentiation Medium for the evaluation of the osteogenic potential. After 3 weeks of incubation in standard conditions the cultured cells were fixed with 4% Paraformaldehyde, stained with suitable dye and analyzed under the Leica DM IL LED light microscope (Leica) for the presence of adipocytes (after staining with Oil Red O, Sigma), chondrocytes (after staining with Alcain Blue, Sigma) and osteocytes (after staining with Alizarin Red, Sigma).
Gene Expression Analysis
The expression of genes was analyzed with qPCR performed in triplicates from three independent experiments. Total RNA was isolated using the RNeasy mini kit (Qiagen, Valencia, CA) from the control and frozen/thawed AT-MSCs (P4 and P5 cells). cDNA was synthesized using the Maxima H Minus First Strand cDNA Synthesis Kit (Thermo Scientific, United States) according to the manufacturer’s instruction. After the reverse transcription, all the cDNA samples were diluted to a final concentration of 8.333 ng/μl and stored at −80°C until further use. The specified primers are presented in Table 1. PowerUp™ SYBR™ Green Master Mix (Thermo Fischer) was used under the following conditions: activation—95°C for 2 min, denaturation—95°C for 5 s, annealing—60°C for 30 s for a total of 40 cycles. Results were analyzed using the 2−∆Ct method relating gene expression to glyceraldehyde-3-phosphate dehydrogenase (GAPDH) and hypoxanthine phosphoribosyltransferase 1 (HPRT 1).
Multiplex
Supernatants from the P4 and P5 cells were collected, centrifuged (10,000 g, 10 min) and stored at −80°C. According to the producer’s guideline, the MILLIPLEX MAP HCYTOMAG-60K and the TGF-β1,2,3 magnetic bead kits (Merck KGaA) were used to evaluate the following cytokines, chemokines, and growth factors: the pro-inflammatory IL-8, IFN-γ, IL-2, IL-1β, MCP-1, IL-3, IL-12, IL-17, IP-10, MIP-1β, and MIP-1α, the anti-inflammatory IL-4, IL-5, IL-6, IL-10, IL-13, IFN-α, TGF-β1, and TGF-β2, and other growth factors, such as PDGF AA, and G-CSF. All the reagents and samples were prepared according to the manufacturer’s protocol. The Belysa 1.0.19 program was used for the analysis of the results.
Statistical Analysis
Results are presented as means ± standard error of the mean (SEM). The GraphPad Prism V6.0 software (GraphPad Software, La Jolla, CA) was used to perform t-tests and estimate statistical differences between the results. p-values less than 0.05 were considered statistically significant.
Results
Morphology and Phenotype
Cells from all the groups exhibited a typical fibroblast-like, spindle-shaped morphology (Figure 2) and adhered to plastic surfaces. No marked differences between the non-frozen (control) and frozen/thawed cells were noted with respect to these features.
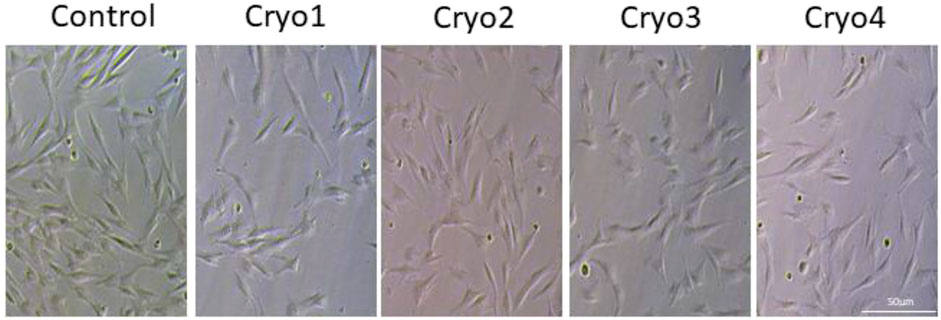
FIGURE 2. Morphology of cultured MSCs without freezing (Control) and after cryopreservation with four cryoprotectants. The cells were grown attached to the bottom of the flasks and exhibited typical morphology of mesenchymal cells.
Cells from the four experimental groups as well as from the control group demonstrated classical phenotype of cultured AT-MSCs, i.e., expressed on the surface the CD73, CD90, CD105 antigens and did not express the CD14, CD19, CD34, CD45, HLA-DR antigens (Lin cocktail) (Table 2). No significant differences in the expression of these antigens were detected between the groups.
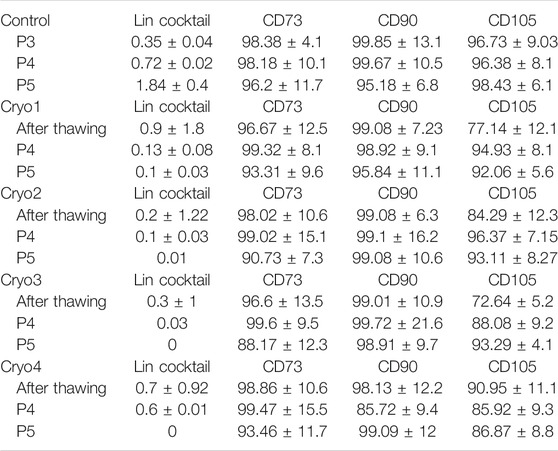
TABLE 2. Flow cytometry of non-frozen (Control) and frozen/thawed (Cryo1 through Cryo4) AT-MSCs. The Lin cocktail contained antibodies against the CD34, CD45, CD19, CD14, HLA-DR antigens. The flow cytometry analysis of the surface antigen profile was performed from 5 samples.
Proliferative Potential: PDT and CFU-F Assays
Proliferative capacities of the control and frozen/thawed AT-MSCs are presented in Figure 3. In the cryopreserved cells obtained from passage 4 the population doubling times were slightly (but not significantly) shorter than that of the control cells (Figure 3, left side). Likewise, PDTs of the cells from passage 5, which were treated with cryoprotectants 1 through 3 were insignificantly less than in the control cells and comparable to those of the P4 cells. In contrast, in the passage 5 cells pre-treated with cryoprotectant 4 PDT was longer than in cells treated with cryoprotectans 1 through 3, but the differences were not statistically significant (Figure 3, right side).
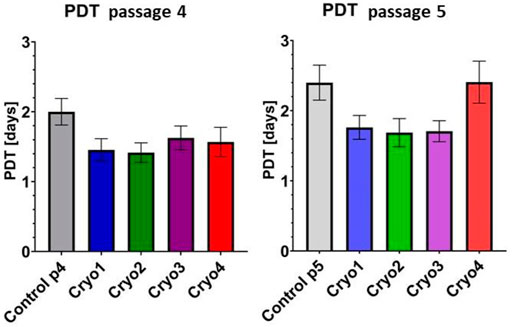
FIGURE 3. Comparison of PDTs of the non-frozen (Control) and frozen with cryoprotectans 1 through 4 (Cryo 1 through Cryo 4) AT- MSCs from passages 4 and 5. Mean values ± SEM are shown. The PDT analysis was performed from 5 samples.
As indicated in Figure 4 clonogenic potential of the non-frozen (Control) cells was slightly higher than those of the frozen/thawed cells. In the passage 4 cells clonogenic potential of cells treated with cryoprotectant 2 was significantly suppressed compared to the control cells; it was also lower than the potentials of the cells from other experimental groups, but the differences were not significant (Figure 4, left side). Clonogenic potential of the passage 5 cells was similar in all the experimental groups (including cryo 2) and did not differ from that of the non-frozen cells (Figure 4, right side).
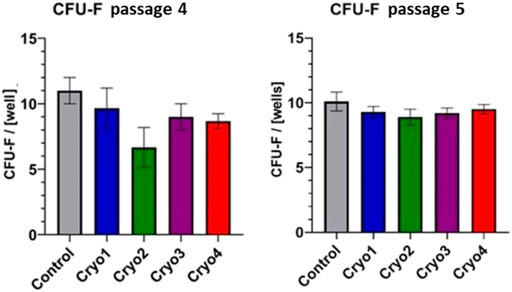
FIGURE 4. Clonogenic potential of the non-frozen (Control) and frozen/thawed (Cryo 1 through Cryo 4) AT-MSCs obtained from passages 4 and 5. Mean values ± SEM are shown. The CFU-F analysis was performed from 5 samples.
Post-Thaw Cell Viability
As shown in Table 3, average survival of the frozen-thawed cells from the four experimental groups ranged from 77 to 92% and the differences in cell viability between the groups were not statistically significant.
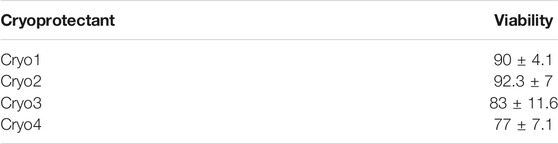
TABLE 3. Viability of the frozen-thawed (Cryo 1 through Cryo 4) cells after their incubation for 30 min at 37°C. Mean values ± SEM are shown. The viability was performed from 5 samples.
Cytoskeleton Structure
Analysis of the cytoskeleton was done because this structure helps cells maintain their shape and internal organization. By analyzing the cytoskeleton, we wanted to check whether the composition of the cryoprotective fluid may have any impact on its structure. As indicated by the actin staining test there is no such relationship. No evident differences in the microfilament structure were detected between the non-frozen (control) and frozen/thawed AT-MSCs (Figure 5).
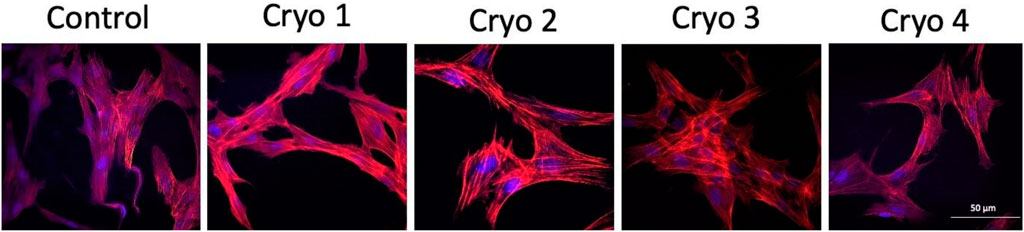
FIGURE 5. Representative images of the non-frozen (Control) and frozen/thawed (Cryo 1 through Cryo 4) AT-MSCs cells stained for the detection of actin filaments using TRITC-conjugated Phalloidin (red color) and the nucleus of the cells revealed with DAPI (blue color).
Differentiation Potential
As shown in Figure 6, none of the four cryoprotectants used affected differentiation of the AT-MSCs along the chondrogenic, adipogenic and osteogenic lineages which was similarly expressed in all the three cases (Figure 6).
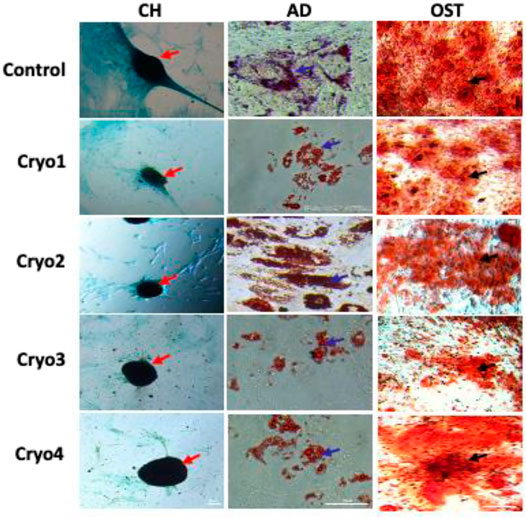
FIGURE 6. Representative images showing the potential to differentiate into chondrocytes (CH), adipocytes (AD), and osteoblasts (OST) of the non-frozen (Control) and cryoprocessed AT-MSCs. Chondrogenic differentiation was confirmed by Alcian blue staining, blue color indicates glycosaminoglycans in cartilage (red arrows); adipogenic differentiation was evaluated by Oil Red, the differentiated culture demonstrates lipid vacuoles, which typical for mature adipocytes (blue arrows); osteogenic differentiation was assessed by Alizarin Red staining of deposition of matrix calcification (black arrows).
Gene Expression
The impact of the freezing/thawing on the expression of the selected genes in the AT-MSCs is presented in Figure 7. The level of expression of C-MYC in the cryoprocessed cells was in most cases similar to that demonstrated by the non-processed control cells except for the cryo2 P4 and cryo4 P5 cells which exhibited the significantly increased expression of this gene (Figure 7).
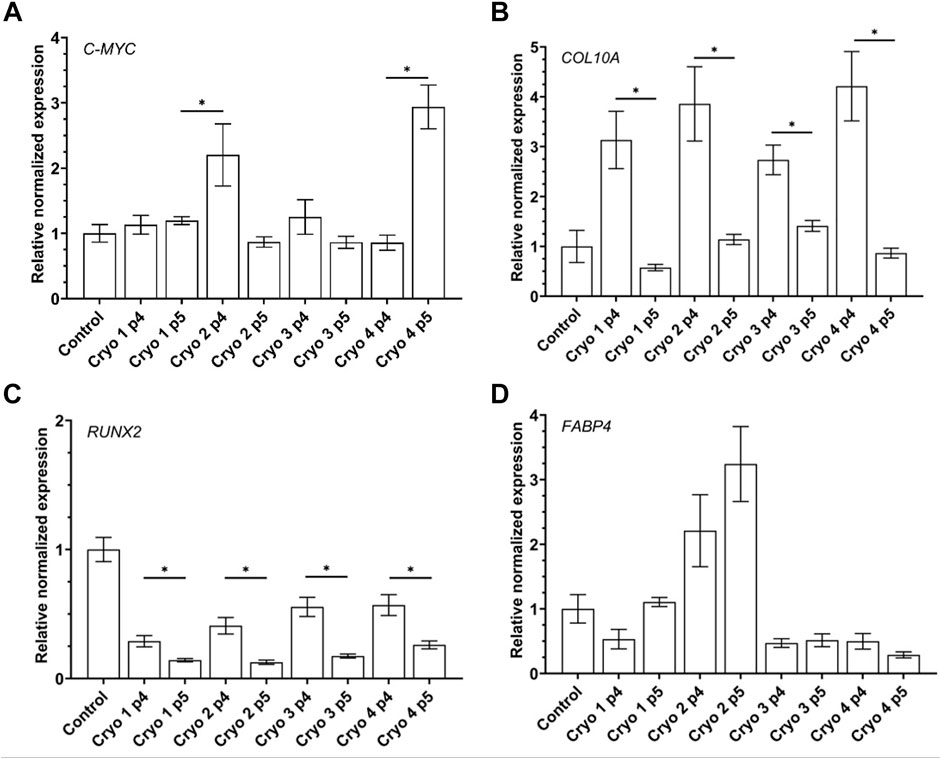
FIGURE 7. Gene expression of pluripotency related gene C-MYC (A) and differentiation-related genes: chondrogenic COL10A1 (B), osteogenic RUNX2 (C), adipogenic FABP4 (D) of the non-frozen (Control) and frozen/thawed AT-MSCs obtained from passages 4 and 5. Mean values ± SEM are shown; * indicates statistical significance (p < 0.05) of the differences between groups marked above with the horizontal solid line.
Compared to the non-frozen control cells expression of COL10A was markedly albeit insignificantly suppressed in the cryopreserved cells from passage 5, especially in those treated with Cryo 1. In the latter cells, expression of COL10A was also significantly lower than in the cryopreserved cells P4 cells in which the gene was markedly but insignificantly better expressed than in the non-frozen control cells (Figure 7).
The pattern of expression of RUNX2 was similar to that of COL10A1 as demonstrated by the significantly suppressed expression of this gene in the passage 5 cells compared to their counterparts from passage 4 from all the four experimental groups. However, compared to the control level, expression of RUNX2 was lower in the passage 4 cells, esp. in those treated with cryo 1 and cryo2, but the differences were not statistically significant (Figure 7).
In cells treated with cryo1, cryo 3 and cryo4 expression of FABP4 was insignificantly lower than in the non-frozen control cells. In contrast, in cells treated with cryo2 and obtained from passages 4 and 5 this expression was slightly but insignificantly higher than in the control cells (Figure 7, panel AD).
With respect to BGLAP no statistically significant differences in the expression of this gene were noted between the experimental groups of the cells as well as between the experimental and the control cells (data non shown).
With respect to the pluripotency-associated gene SOX-2, the adipogenesis-associated gene ADIPOQ, and the chondrogenesis-associated gene COL2A no expression thereof was detected in the investigated cells from both the control and the experimental groups (data not shown).
Multiplex
As shown in Table 1, there was a noticeable variation in the secretion of IL-6, MCP-1, TGF-β1, fractalkine, G-CSF, IP-10, MIP-1α, MIP-1β, IL-10 and IFNα by the cryopreserved AT-MDCs obtained from passage 4, but generally the levels produced by these cells and that secreted by the non-frozen, control cells were not statistically significant. For example, average concentrations of IL-6 in the culture media of the cryopreserved cells ranged from 247 to 1781 pg/ml depending on the cryoprotectant used as compared to 625 pg/ml detected in the medium of the control cells. In contrast, compared to the control values, significantly lower levels of TGF-β2 and FLT-3L were detected in the media of the passage 4 AT-MDCs treated with either of the four cryoprotectants.
In the case of passage 5 cells no statistically significant differences were detected between the amounts of the tested cytokines and growth factors produced by the cryopreserved and the non-frozen, control AT-MDCs. For example, secretion of IL-6 by the cryoprocessed cells averaged from 306 to 356 pg/ml whereas in the medium of the control cells the average concentration of this cytokine equaled to 345 pg/ml. Likewise, no statistical differences could be detected between the levels of other cytokines/factors secreted by the cryoprocessed and the control cells obtained from passage 5, including TGF-β2 and Flt-3L the production of which was significantly suppressed in the frozen cells from passage 4 (Table 4).
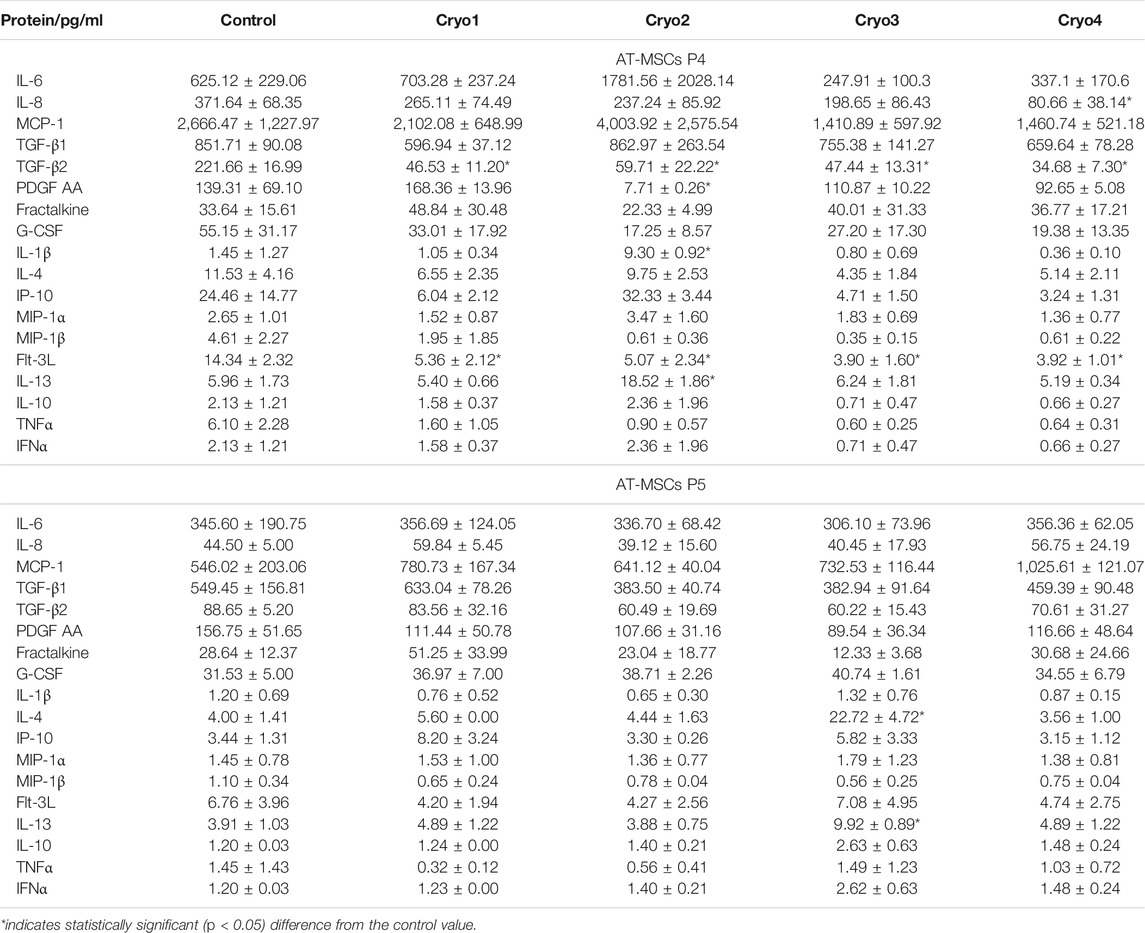
TABLE 4. Cytokines secreted by the non-frozen (Control) and cryopreserved (Cryo1, Cryo2, Cryo3, Cryo4) AT-MSCs obtained from passages 4 (P4) and 5 (P5). Cytokine levels detected in the medium from the non-frozen cells served as the Control values. Mean values ± SEM are shown.
Discussion
Over the recent years, mesenchymal stromal cells, including the adipose-derived MSCs, have secured a wide spectrum of clinical applications (Wang et al., 2012; Sharma et al., 2014). However, before they can be used in therapy, MSCs obtained from a certain tissue must be expanded in vitro to the appropriate number and then stored until their quality studies are completed and the cells are released as a medical product. In order for the cells to be stored safely and for a long time, they must be subjected to deep-freezing. That is why cryopreservation, as one of the crucial steps in the preparation of MSCs, must be optimized and applied as a safe and thoroughly mustered technique. As was previously indicated, neither the recovery rate, viability nor phenotype of MSCs were seriously affected by thawing (Luetzkendorf et al., 2015; Pollock et al., 2015; Oja et al., 2019), the observation confirmed by us in the present study. It is also important, however, to assess physiological stability of the frozen-thawed cells and to estimate the impact of various freezing conditions on the cells’ properties. Hence, in the present investigation four different DMSO-based cryoprotectants were employed to check their effect on the physiological properties of the cells after thawing and to select the optimal freezing formula.
As indicated by the present results, none of the cryoprotectants markedly affected the shape, adhesion to plastic or the cytoskeleton structure of the tested cells. Likewise, no statistically significant differences between the cells from the experimental and control groups were detected in the rate of proliferation and the colony-forming capacity of these cells, as indicated by the results of the PDT and CFU assays, respectively.
As demonstrated by Shaik and co-workers cryopreservation of the adipose tissue-derived stem cells can be successfully performed for as long as a decade. These authors found that the viability of MSCs thawed after the 10 years storage remained at about 78% which was not significantly less compared to the cells kept frozen for a shorter time (Shaik et al., 2018). In accord with this the viability of our AT-MSCs ranged from 78 to 90% although we stored the cells markedly shorter. On the other hand, it was demonstrated that long-term storage of hematopoietic cells at −150°C did not affect the cells’ viability proliferative potential (Broxmeyer et al., 2003; Broxmeyer et al., 2011; Kim et al., 2021). Some authors (Oja et al., 2019) demonstrated a better than our viability of the frozen-thawed cells, but only in cells obtained from the first passages. Overall, our present results confirm those of other authors’ indicating that freezing of MSCs does not markedly disturb the viability of the frozen-thawed cells (Luetzkendorf et al., 2015; Shaik et al., 2018; Oja et al., 2019).
In our present study, the phenotype of the frozen-thawed AT-MSCs did not differ from that of the non-frozen, control cells, irrespectively of the cryoprotectant or cell passage used. Variation in the homogeneity of our cell populations with respect to the expression of hematopoietic markers did not exceed 1% and was similar to or better than that reported by others (Mitchell et al., 2006; Baer et al., 2013). Moreover, our present findings, in accord with those of others’ (Luetzkendorf et al., 2015; Oja et al., 2019), indicate that freezing of MSCs does not affect the phenotype of these cells. In fact, although expression of CD105 which was low just after thawing returned to normal during passages 4 and 5.
To confirm the multilineage potential of the cells under study we checked the expression of genes whose function is associated with osteogenesis, chondrogenesis and adipogenesis as well as those associated with pluripotency. We found that in all the cryopreserved (experimental) groups of cells expression of the osteogenesis-associated gene RUNX2 was substantively muted when the cells were obtained from the passage 5 compared to those from passage 4. In turn, the latter cells expressed less RUNX2 than did the non-frozen control cells, but the difference was not statistically significant. These findings are in agreement with those of James and co-workers (James et al., 2011). With respect to adipogenesis we found that expression of the FABP4 gene was significantly down-regulated in cells from all the experimental groups except for those treated with cryoprotectant 2. In the latter case expression of FABP4 was similarly elevated in cells obtained from passages 4 and 5. These results vary from those of Zanata and co-workers who did not detect any decrease in the adipogenic potential of the cryopreserved adipose tissue cells (Zanata et al., 2018). In the present study, no significant differences were noted in the chondrogenic potential (as estimated by the expression of the COL10A1 gene) of cells treated with the four cryoprotectants, although there were significant differences between the passage 4 and passage 5 cells. In contrast, James et al. reported that the chondrogenic potential of frozen AT-MSCs was lower than that of “fresh” MSCs (James et al., 2011). With respect to the expression of C-MYC we have observed its inexplicable up-regulation in the cryo2 P4 and the cryo 4 P5 cells. Unfortunately, in our hands the expression of the genes’ tested did not coincide with the cells’ differentiation capacities in vitro; there were no differences between the cells from the experimental groups obtained from the 4th and the 5th passage. Moreover, visualization of the differentiated cells did not reveal any significant differences between the experimental and control groups. At present, we have no plausible explanation for the observed discrepancies between the expression of the selected genes and the capacities of the tested cells to undergo differentiation into adipocytes, chondrocytes, or osteocytes.
In the present investigation, the secretory properties of AT-MSCs appeared to be similar to the those reported by other authors (Kilroy et al., 2007; Krumboeck et al., 2013). We have demonstrated that although the capacity to produce cytokines and growth factors might be compromised in freshly thawed cells, eventually the whole process of freezing, storage and thawing of AT-MSC did not significantly impair the secretory function of these cells. This secretory instability occurring in some experimental groups and in relation to some factors seems to be quite random and it is difficult to see any regularity in this phenomenon. Indeed, the temporarily unstable secretion of almost all of the tested factors by cells obtained from passage 4 was fully restored during the 5th passage, regardless of the cryoprotectant used. Similar results were reported by other authors who showed that impairment of the secretory factors-mediated immunomodulatory properties of MSCs, which occurred immediately after thawing (François et al., 2012; Moll et al., 2014), disappeared quickly in culture (François et al., 2012). According to these authors the early post-thaw drop in the cells’ secretory function is associated with the cryopreservation-induced heat-shock stress. Our present results support this notion, although the secretory function of the cells investigated by us returned to normal after a slightly longer period of time. Nevertheless, the heat-shock stress that occurs in the cell after freezing/thawing procedure seems to be a factor which, although undetectable in classical cellular tests, is clearly visible in the physiology of cells and may quite significantly affect their properties immediately after thawing.
Unfortunately, in the majority of clinical applications of AT-MSC, these cells are administered directly after thawing. Our observations show that this procedure physiologically destabilizes the cells, even if the routine qualitative tests (viability, recovery, phenotype) do not reveal this. It cannot be ruled out that the transient physiological instability may affect the therapeutic effectiveness of the applied cells, especially in terms of their secretory capacities. A simple and low-cost solution to this problem may be stabilitization of the cells in a short culture what, as clearly demonstrated in our investigation, restores their secretion capacity and, to a lesser extent, expression of the selected genes immediately after thawing and later in the culture. The composition of the applied cryoprotective fluid did not seem to affect this phenomenon in any way. All these observation allows us to draw two basic conclusions:
- judging by the dynamics of changes of the secretory function of the thawed AT-MSCs we suggest that before their clinical application the cells should be (if possible) cultured for at least one passage to recover their physiological stability and thus assure their optimal therapeutic potential;
- DMSO-based cryoprotectants are safe and efficient agents for freezing of AT-MSCs and as such can be used in many variants.
Data Availability Statement
The original contributions presented in the study are included in the article/Supplementary Material, further inquiries can be directed to the corresponding author.
Author Contributions
ES, TO, EKM - the conception and design of the study, ES, MT, IMS, MM - acquisition of the data, ES, MPG, KB, EKM - analysis and interpretation of the data, ES, MPG, KB, EKM - drafting of the article; ES, MCK, ISG, DB, TO, EKM – revision and critical evaluation of the draft for important intellectual content, ES, TO, EKM – final approval of the version to be submitted. All authors approved the manuscript for publication.
Funding
This work was supported by the ABC therapy, STRATEGMED2/267976/13/NCBR/2015 grant from the Polish National Centre for Research and Development.
Conflict of Interest
Author ES, MPG, KB, MT, IMS, MM, DB, TO and EKM were employed by the company Polish Stem Cell Bank, FamiCord Group.
The remaining authors declare that the research was conducted in the absence of any commercial or financial relationships that could be construed as a potential conflict of interest.
Publisher’s Note
All claims expressed in this article are solely those of the authors and do not necessarily represent those of their affiliated organizations, or those of the publisher, the editors and the reviewers. Any product that may be evaluated in this article, or claim that may be made by its manufacturer, is not guaranteed or endorsed by the publisher.
Abbreviations
AT-MSCs, adipose tissue-derived mesenchymal stromal cells; ATP, adenosine triphosphate; BM-MSCs, bone marrow-derived mesenchymal stromal cells; CFU-F, colony-forming unit fibroblast; Cryo, cryoprotectant; DAPI, 4′,6-diamidino-2-phenylindole; DMSO, dimethyl sulfoxide; FITC, fluorescein isothiocyanate; MSCs, mesenchymal stromal cells; P, passage; PDT, population doubling time; PE, phycoerythrin; SVF, stromal vascular fraction; TRITC, tetramethylrhodamine.
References
Baer, P. C., Kuçi, S., Krause, M., Kuçi, Z., Zielen, S., Geiger, H., et al. (2013). Comprehensive Phenotypic Characterization of Human Adipose-Derived Stromal/stem Cells and Their Subsets by a High Throughput Technology. Stem Cell Develop. 22 (2), 330–339. doi:10.1089/scd.2012.0346
Benkhalifa, M., Ferreira, Y. J., Chahine, H., Louanjli, N., Miron, P., Merviel, P., et al. (2014). Mitochondria: Participation to Infertility as Source of Energy and Cause of Senescence. Int. J. Biochem. Cel Biol. 55, 60–64. doi:10.1016/j.biocel.2014.08.011
Broxmeyer, H. E., Lee, M.-R., Hangoc, G., Cooper, S., Prasain, N., Kim, Y.-J., et al. (2011). Hematopoietic Stem/progenitor Cells, Generation of Induced Pluripotent Stem Cells, and Isolation of Endothelial Progenitors from 21- to 23.5-year Cryopreserved Cord Blood. Blood 117 (18), 4773–4777. doi:10.1182/blood-2011-01-330514
Broxmeyer, H. E., Srour, E. F., Hangoc, G., Cooper, S., Anderson, S. A., and Bodine, D. M. (2003). High-efficiency Recovery of Functional Hematopoietic Progenitor and Stem Cells from Human Cord Blood Cryopreserved for 15 Years. Proc. Natl. Acad. Sci. 100 (2), 645–650. doi:10.1073/pnas.0237086100
Cantero Peral, S., Burkhart, H. M., Oommen, S., Yamada, S., Nyberg, S. L., Li, X., et al. (2015). Safety and Feasibility for Pediatric Cardiac Regeneration Using Epicardial Delivery of Autologous Umbilical Cord Blood-Derived Mononuclear Cells Established in a Porcine Model System. Stem Cell Transl. Med. 4, 195–206. doi:10.5966/sctm.2014-0195
Carmeliet, P., and Jain, R. K. (2011). Molecular Mechanisms and Clinical Applications of Angiogenesis. Nature 473, 298–307. doi:10.1038/nature10144
Davis, J., Rowley, S., Braine, H., Piantadosi, S., and Santos, G. (1990). Clinical Toxicity of Cryopreserved Bone Marrow Graft Infusion. Blood 75 (3), 781–786. doi:10.1182/blood.v75.3.781.bloodjournal753781
Dominici, M., Le Blanc, K., Mueller, I., Slaper-Cortenbach, I., Marini, F. C., Krause, D. S., et al. (2006). Minimal Criteria for Defining Multipotent Mesenchymal Stromal Cells. The International Society for Cellular Therapy Position Statement. Cytotherapy 8, 315–317. doi:10.1080/14653240600855905
Doorn, J., Moll, G., Le Blanc, K., van Blitterswijk, C., and de Boer, J. (2012). Therapeutic Applications of Mesenchymal Stromal Cells: Paracrine Effects and Potential Improvements. Tissue Eng. B: Rev. 18, 101–115. doi:10.1089/ten.TEB.2011.0488
François, M., Copland, I. B., Yuan, S., Romieu-Mourez, R., Waller, E. K., and Galipeau, J. (2012). Cryopreserved Mesenchymal Stromal Cells Display Impaired Immunosuppressive Properties as a Result of Heat-Shock Response and Impaired Interferon-γ Licensing. Cytotherapy 14 (2), 147–152. doi:10.3109/14653249.2011.623691
Fuller, R. (2004). Subtle Energies and the American Metaphysical Tradition. Cryo Lett. 25, 375–386. doi:10.1093/acprof:oso/9780195167962.003.0024
Gao, D., and Critser, J. K. (2000). Mechanisms of Cryoinjury in Living Cells. ILAR J. 41, 187–196. doi:10.1093/ilar.41.4.187
Han, Y., Li, X., Zhang, Y., Han, Y., Chang, F., and Ding, J. (2019). Mesenchymal Stem Cells for Regenerative Medicine. Cells 8 (8), 886. doi:10.3390/cells8080886
James, A. W., Levi, B., Nelson, E. R., Peng, M., Commons, G. W., Lee, M., et al. (2011). Deleterious Effects of Freezing on Osteogenic Differentiation of Human Adipose-Derived Stromal Cells In Vitro and In Vivo. Stem Cell Develop. 20 (3), 427–439. doi:10.1089/scd.2010.0082
J. Braga Osorio Gomes Salgado, A., L. Goncalves Reis, R., Jorge Carvalho Sousa, N., M. Gimble, J., J. Salgado, A., L. Reis, R., et al. (2010). Adipose Tissue Derived Stem Cells Secretome: Soluble Factors and Their Roles in Regenerative Medicine. Cscr 5, 103–110. doi:10.2174/157488810791268564
Kang, M.-H., Das, J., Gurunathan, S., Park, H.-W., Song, H., Park, C., et al. (2017). The Cytotoxic Effects of Dimethyl Sulfoxide in Mouse Preimplantation Embryos: a Mechanistic Study. Theranostics 7, 4735–4752. doi:10.7150/thno.21662
Kilroy, G. E., Foster, S. J., Wu, X., Ruiz, J., Sherwood, S., Heifetz, A., et al. (2007). Cytokine Profile of Human Adipose-Derived Stem Cells: Expression of Angiogenic, Hematopoietic, and Pro-inflammatory Factors. J. Cel. Physiol. 212 (3), 702–709. doi:10.1002/jcp.21068
Kim, G.-H., Kwak, J., Kim, S. H., Kim, H. J., Hong, H. K., Jin, H. J., et al. (2021). High Integrity and Fidelity of Long-Term Cryopreserved Umbilical Cord Blood for Transplantation. Jcm 10 (2), 293. doi:10.3390/jcm10020293
Kim, N., and Cho, S.-G. (2013). Clinical Applications of Mesenchymal Stem Cells. Korean J. Intern. Med. 28 (4), 387–402. doi:10.3904/kjim.2013.28.4.387
Krumboeck, A., Giovanoli, P., and Plock, J. A. (2013). Fat Grafting and Stem Cell Enhanced Fat Grafting to the Breast under Oncological Aspects - Recommendations for Patient Selection. The Breast 22 (5), 579–584. doi:10.1016/j.breast.2013.05.006
Lane, M., and Gardner, D. K. (2005). Understanding Cellular Disruptions during Early Embryo Development that Perturb Viability and Fetal Development. Reprod. Fertil. Dev. 17, 371–378. doi:10.1071/rd04102
Luetzkendorf, J., Nerger, K., Hering, J., Moegel, A., Hoffmann, K., Hoefers, C., et al. (2015). Cryopreservation Does Not Alter Main Characteristics of Good Manufacturing Process-Grade Human Multipotent Mesenchymal Stromal Cells Including Immunomodulating Potential and Lack of Malignant Transformation. Cytotherapy 17 (2), 186–198. doi:10.1016/j.jcyt.2014.10.018
Mitchell, J. B., McIntosh, K., Zvonic, S., Garrett, S., Floyd, Z. E., Kloster, A., et al. (2006). Immunophenotype of Human Adipose-Derived Cells: Temporal Changes in Stromal-Associated and Stem Cell-Associated Markers. Stem Cells 24 (2), 376–385. doi:10.1634/stemcells.2005-0234
Moll, G., Alm, J. J., Davies, L. C., von Bahr, L., Heldring, N., Stenbeck-Funke, L., et al. (2014). Do cryopreserved Mesenchymal Stromal Cells Display Impaired Immunomodulatory and Therapeutic Properties? Stem Cells 32 (9), 2430–2442. doi:10.1002/stem.1729
Oja, S., Kaartinen, T., Ahti, M., Korhonen, M., Laitinen, A., and Nystedt, J. (2019). The Utilization of Freezing Steps in Mesenchymal Stromal Cell (MSC) Manufacturing: Potential Impact on Quality and Cell Functionality Attributes. Front. Immunol. 10, 1627. doi:10.3389/fimmu.2019.01627
Pittenger, M. F., Discher, D. E., Péault, B. M., Phinney, D. G., Hare, J. M., and Caplan, A. I. (2019). Mesenchymal Stem Cell Perspective: Cell Biology to Clinical Progress. Npj Regen. Med. 4, 22. doi:10.1038/s41536-019-0083-6
Pollock, K., Sumstad, D., Kadidlo, D., McKenna, D. H., and Hubel, A. (2015). Clinical Mesenchymal Stromal Cell Products Undergo Functional Changes in Response to Freezing. Cytotherapy 17 (1), 38–45. doi:10.1016/j.jcyt.2014.06.008
Reinders, M. E., Leuning, D. G., de Fijter, J. W., Hoogduijn, M. J., and Rabelink, T. J. (2013). Mesenchymal Stromal Cell Therapy for Cardio Renal Disorders. Curr. Pharm. Des. 20 (14), 2412–2429. doi:10.2174/13816128113199990477
Rustad, K. C., and Gurtner, G. C. (2012). Mesenchymal Stem Cells Home to Sites of Injury and Inflammation. Adv. Wound Care 1 (4), 147–152. doi:10.1089/wound.2011.0314
Schepers, K., and Fibbe, W. E. (2016). Unraveling Mechanisms of Mesenchymal Stromal Cell-Mediated Immunomodulation through Patient Monitoring and Product Characterization. Ann. N.Y. Acad. Sci. 1370, 15–23. doi:10.1111/nyas.12984
Semenova, E., Chroscinska-Krawczyk, M., Grudniak, M., Oldak, T., and Machaj, E. (2018). Clinical Application of AD-MSCs - A Review. J. Pre Clin. Clin. Res. 12 (3), 100–105. doi:10.26444/jpccr/94910
Shaik, S., Wu, X., Gimble, J., and Devireddy, R. (2018). Effects of Decade Long Freezing Storage on Adipose Derived Stem Cells Functionality. Sci. Rep. 8 (1), 8162. doi:10.1038/s41598-018-26546-7
Sharma, R. R., Pollock, K., Hubel, A., and McKenna, D. (2014). Mesenchymal Stem or Stromal Cells: a Review of Clinical Applications and Manufacturing Practices. Transfusion 54 (5), 1418–1437. doi:10.1111/trf.12421
Spaeth, E., Klopp, A., Dembinski, J., Andreeff, M., and Marini, F. (2008). Inflammation and Tumor Microenvironments: Defining the Migratory Itinerary of Mesenchymal Stem Cells. Gene Ther. 15 (10), 730–738. doi:10.1038/gt.2008.39
Stroncek, D., Fautsch, S., Lasky, L., Hurd, D., Ramsay, N., and McCullough, J. (1991). Adverse Reactions in Patients Transfused with Cryopreserved Marrow. Transfusion 31 (6), 521–526. doi:10.1046/j.1537-2995.1991.31691306250.x
Truong, T. H., Moorjani, R., Dewey, D., Guilcher, G. M. T., Prokopishyn, N. L., and Lewis, V. A. (2016). Adverse Reactions during Stem Cell Infusion in Children Treated with Autologous and Allogeneic Stem Cell Transplantation. Bone Marrow Transpl. 51 (5), 680–686. doi:10.1038/bmt.2015.331
Ullah, M., Liu, D. D., and Thakor, A. S. (2019). Mesenchymal Stromal Cell Homing: Mechanisms and Strategies for Improvement. iScience 15, 421–438. doi:10.1016/j.isci.2019.05.004
Wang, S., Qu, X., and Zhao, R. C. (2012). Clinical Applications of Mesenchymal Stem Cells. J. Hematol. Oncol. 5, 19. doi:10.1186/1756-8722-5-19
Keywords: cryopreservation, banking, cryoprotectant, ADSC, application
Citation: Semenova E, Grudniak MP, Bocian K, Chroscinska-Krawczyk M, Trochonowicz M, Stepaniec IM, Murzyn M, Szablowska-Gadomska I, Boruczkowski D, Oldak T and Machaj EK (2021) Banking of AT-MSC and its Influence on Their Application to Clinical Procedures. Front. Bioeng. Biotechnol. 9:773123. doi: 10.3389/fbioe.2021.773123
Received: 09 September 2021; Accepted: 11 November 2021;
Published: 30 November 2021.
Edited by:
Ornella Parolini, Catholic University of the Sacred Heart, Rome, ItalyReviewed by:
Francisco José Nicolás, Biomedical Research Institute of Murcia (IMIB), SpainDiletta Trojan, Fondazione Banca dei Tessuti di Treviso Onlus, Italy
Copyright © 2021 Semenova, Grudniak, Bocian, Chroscinska-Krawczyk, Trochonowicz, Stepaniec, Murzyn, Szablowska-Gadomska, Boruczkowski, Oldak and Machaj. This is an open-access article distributed under the terms of the Creative Commons Attribution License (CC BY). The use, distribution or reproduction in other forums is permitted, provided the original author(s) and the copyright owner(s) are credited and that the original publication in this journal is cited, in accordance with accepted academic practice. No use, distribution or reproduction is permitted which does not comply with these terms.
*Correspondence: Eugeniusz K. Machaj, S3J6eXN6dG9mLk1hY2hhakBwYmttLnBs