- 1The First Affiliated Hospital, Medical Imaging Centre, Hengyang Medical School, University of South China, Hengyang, China
- 2Institute of Medical Imaging, Hengyang Medical School, University of South China, Hengyang, China
- 3Laboratory of Ultrasound Molecular Imaging, The Third Affiliated Hospital of Guangzhou Medical University, Guangzhou, China
Applying nanosized ultrasound contrast agents (nUCAs) in molecular imaging has received considerable attention. nUCAs have been instrumental in ultrasound molecular imaging to enhance sensitivity, identification, and quantification. nUCAs can achieve high performance in molecular imaging, which was influenced by synthetic formulations and size. This review presents an overview of nUCAs from different synthetic formulations with a discussion on imaging and detection technology. Then we also review the progress of nUCAs in preclinical application and highlight the recent challenges of nUCAs.
Introduction
Nanosized ultrasound contrast agents (nUCAs), as a complementary of microbubbles, are developed as contrast enhancers for ultrasound molecular imaging with the ability of penetrating through vasculature for extravascular imaging (Krupka et al., 2010; Wang et al., 2017). High accumulation of nUCAs in an examined area can enhance the signal of target regions with prolonging persistence time compared with microsized UCAs, especially in tumors with the effect of enhanced permeability and retention effect (EPR) (Omata et al., 2020; Perera et al., 2020). Furthermore, the persistence time of the nUCAs lasted obviously longer by binging targeting ligands with receptors in target regions (Jiang et al., 2016; Wang et al., 2018). nUCAs can be modified at their surfaces with specific targeting ligands to improve accumulation in tissues, reduce off-target effect, and improve safety, which is considered as a promising approach in clinical practice.
To date, the existing formulations of nUCAs are post formulations of microbubbles, such as centrifuge and filtration. However, post formulations may influence the stability of nUCAs (Tong et al., 2013). Notably, the echogenicity of nUCAs under ultrasound may decrease and show low ultrasound signal because of low backscatter (Gorce et al., 2000; Sheeran et al., 2013). A variety of nUCAs have been developed to improve stability and echogenicity. In this review, the imaging and detection technology is first discussed to further explain the low echogenicity of nUCAs. Then different synthetic formulations of nUCAs will be introduced. While many of these contrast agents are employed for both imaging and therapeutic applications in preclinic, the focus of this review will be more toward their utility and potential as molecular ultrasound imaging agents (Figure 1).
Imaging and detection technology
Ultrasound imaging has been widely used in clinical fields because of relatively low price, no need of radiation, and noninvasiveness. The principle of early ultrasound imaging is linear imaging, but ultrasonic pulse through tissues and contrast agents would, respectively, produce nonlinear propagation and nonlinear vibration to produce nonlinear acoustic signal (harmonic signal). In the late 1990s, harmonic signals generated by the nonlinear effects of tissues and contrast agents have been used in ultrasound imaging. According to the different sources of harmonic generation, harmonic imaging can be divided into tissue harmonic imaging and contrast harmonic imaging. A variety of experimental studies show that harmonic imaging has better spatial resolution, higher contrast, and clearer image sharpness.
When the pressure of ultrasonic pulse has reached a certain threshold, the frequency of the emitted wave is double than that of ultrasound contrast agents, which can produce a second harmonic signal and ultraharmonic signal to achieve a better imaging. If the frequency fails to achieve threshold, subharmonics and ultraharmonics will still be produced, but the effect is not obvious. Notably, tissue does not produce subharmonics and ultraharmonics. This further improves the contrast between tissue and contrast agents. However, the size of contrast agents has significant influence on the signal; a smaller size, such as NBs, may produce a low signal. In clinical practice, harmonic imaging contains fundamental wave and harmonic wave. The intensity of harmonic wave is much weaker than the fundamental wave, and the signal-to-noise ratio is low. Thus, it requires high sensitivity of equipment to detect and increase the difficulty of equipment development.
Harmonic imaging can be used to detect the signal of contrast agents, but it has a low value to differentiate the signal of nontargeted and targeted contrast agents. Lindner et al. showed that ultrasound signals first contain tissue signals, targeted and freely circulating UCAs, and freely circulating UCAs could be cleared after the cycle time (5–10 min later), and then a high powered ultrasound pulse was applied to destroy the UCAs in the examined area. The difference in signals before and after destruction would be expressed as targeted UCA signals (Lindner et al., 2001; Yu et al., 2019). Another approach to identify targeted contrast agents is evaluating the residence time in an examined area by algorithm. Only ultrasound contrast agents with a dwell time longer than time threshold were regarded as targeted contrast agents. This technology has the advantages of real-time image acquisition without the need to apply high-powered ultrasound (Pysz et al., 2012; Zlitni and Gambhir, 2018). In addition, sensitive particle acoustic quantification technology has been used to quantify receptor expression levels in vivo (Wei et al., 1998).
Chemical synthesis of nanosized ultrasound contrast agents
Nanobubbles (NBs) are nanoparticles (in the nanometer range) that are commonly composed of an encapsulating shell and gas core (Wang et al., 2021). According to different diameters, NBs can be divided into surface and bulk nanobubbles (Craig, 2010; Azevedo et al., 2019). NBs have inferior oscillation behavior relative to microbubbles but are of interest in therapeutic approaches (Ignee et al., 2016). Thus, phase-change contrast agents (PCCAs) have been developed to overcome the size limitation of microbubbles, which can change liquid core into gas core to improve signal-to-noise ratio after activating by ultrasound.
The encapsulating shell mainly influences stability and durability, while the gas determines solubility and acoustic properties (Paefgen et al., 2015). The shell consists mostly of surfactants, polymers, or proteins, while the gas core components are comprised of elevated molecular weights and low solubility filling gases, such as SF6 or C3F8 (Abenojar et al., 2020). To overcome the weak echogenicity of nanobubbles, novel methods are constantly emerging (Wang et al., 2021).
Encapsulating shell
The shell serves as a barrier to the dissipation of gas between the encapsulated gas and the underlying aqueous medium (Unga and Hashida, 2014). The shell materials are mostly phospholipids or proteins, which are more susceptible to acoustic waves than the hard shells of polymers (Pote et al., 2021). In addition, chitosan is a material of choice for the nanobubble shell because of its low toxicity, low immunogenicity, and excellent biocompatibility (Liu et al., 2021). NBs can be bioconjugated with different forms of drugs or proteins/DNA for selective delivery. Poly(lactic-co-glycolic) acid (PLGA) becomes the preferred choice of pharmaceutical carrier material because of high stability, biodegradability, decreased systemic toxicity, and in vivo biocompatibility (Yan et al., 2018). It is a type of polymer synthesized by the polymerization of lactic and glycolic acid in a certain bimolecular weight of the two polymers. In ultrasound molecular imaging, adequate selection of shell materials is critical for various applications with different rigidities, charges, thicknesses, and functional groups. To improve the echogenicity of nanobubbles for extravascular imaging, Exner et al. have reported on the formulation of echogenic perfluoropropane gas nanobubbles stabilized by a lipid–pluronic surfactant shell (Hernandez et al., 2017). Furthermore, they describe a novel nanobubble of perfluoropropane gas stabilized by a surfactant and lipid membrane and a crosslinked network of N,N-diethylacrylamide (Perera et al., 2017; de Leon et al., 2019). These results demonstrate the capabilities and advantages of a new, more stable, nanometer-scale ultrasound contrast agent that can be utilized in future work for diagnostic scans and molecular imaging.
Gas core
The type of gas core determines its residence time in systemic circulation. In ultrasound molecular imaging, hydrophobic gases are typically employed because they are immiscible in the aqueous environment, which prevents them from leaking out fast and leads to a longer bubble half-life. Among hydrophobic gases, those with higher molecular weight, higher gas density, and lower diffusivity coefficient are expected to give more stable bubbles (Kanbar et al., 2017; Omata et al., 2019). Perfluorocarbons (PFCs) are biocompatible, biologically inert, and highly stable chemicals that are not metabolized in the body after injection (Schutt et al., 2003; Cheng, 2004). In addition, increasing the chain length of PFCs by CF2 leads to an order of magnitude decrease in solubility in water (Chomas et al., 2001; Klibanov, 2002). PFCs also reduce interfacial tension, which can further improve bubble performance (Nguyen et al., 2011; Hernandez et al., 2018). Thus, a large amount of research has focused on using heavier perfluorocarbons, such as C4F10, C5F12, and C6F14 (Sheeran and Dayton, 2012).
Biogenic synthesis of nanosized ultrasound contrast agents
The stability of nanobubbles has raised questions because of their surface tension forces. In addition, the safety of nanobubbles is also controversial because of their synthetic materials and chemical formulation. Shapiro et al. obtained biogenic gas nanobubbles derived from two different microorganism species for molecular imaging, which are named as gas vesicles (GVs) (Shapiro et al., 2014). Gas vesicles were encoded in many bacteria and phyla of archaea, which were illustrated in previous reviews (Pfeifer, 2012; Garrute and Machado, 2020). For this reason, we chose to review the research that used GVs as ultrasound molecular reporters.
Gas vesicles have a protein shell with a hollow gas-filled core, with a dimension of ∼200 nm and a thickness of ∼2 nm. GVs are encoded by 8–14 genes, including the primary structural proteins GvpA and GvpC, and several secondary proteins that function as essential minor constituents or chaperones (Pfeifer, 2012). GvpA is a 7.4-kDa amphiphilic protein that serves as the main structural backbone of the GV shell by forming 4.6-nm-wide ribs, while GvpC is a protein that provides structural reinforcement (Walsby and Hayes, 1989; Buchholz et al., 1993). Shapiro et al. found that the removal, addition, or modification of GvpC would alter the acoustic properties of GVs (Lakshmanan et al., 2016). With the development of genetic engineering, plasmids with gvp genes were transferred into engineered bacteria to produce GVs, which can signify cellular location and function (Bourdeau et al., 2018; Huang et al., 2019) (Table 1).

TABLE 1. The production of gas vesicles (GVs) from engineered bacteria in ultrasound imaging (Bourdeau et al., 2018; Huang et al., 2019).
Targeted strategies of nanosized ultrasound contrast agents
Ultrasound molecular imaging relies on delivering UCAs to a specific site. There are two different classification methods of strategies involving the coupling of UCAs for targeting: 1) passive targeting or 2) active targeting (Table 2).
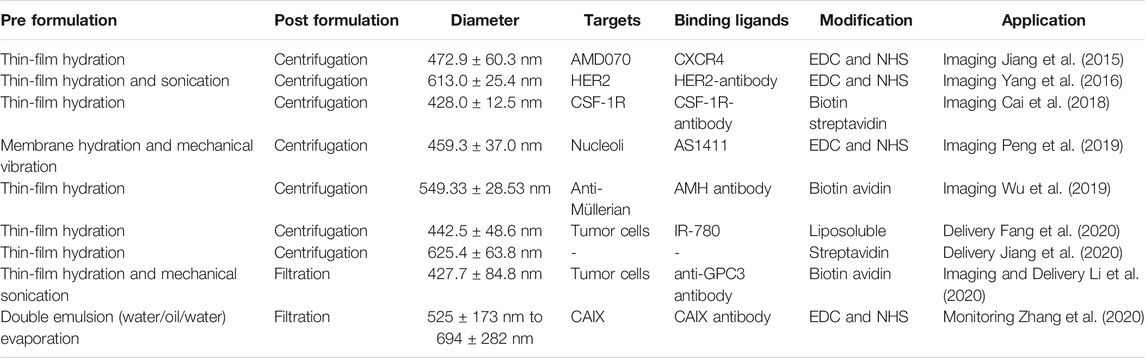
TABLE 2. Different modifications of nanobubbles (Jiang et al., 2015; Yang et al., 2016; Cai et al., 2018; Peng et al., 2019; Wu et al., 2019; Fang et al., 2020; Jiang et al., 2020; Li et al., 2020; Zhang et al., 2020).
Passive targeting
Passive targeting is related to the so-called enhanced permeability and retention (EPR) effect, which is a feature of many tumors and diverse inflammation sites. The pathological changes in blood vessels and normal blood vessels on the structure and morphology is different, the gap between the endothelial cells can be widened to 800 nm, and lymph is blocked at the same time. These two major reasons prompt nUCAs to seep in the enhancement of the lesion site and retention.
Active targeting
Active targeting includes noncovalent conjugation and covalent conjugation. Noncovalent conjugation is similar to the modification and conjugation of ligands. Incorporation of phosphatidylserine in the shell of NBs results in activation and surface attachment of complement fragments because of a highly negative charged shell (Lindner et al., 2000a). In addition, covalent conjugation needs EDC and NHS to activate functional groups and active-carboxyl groups (Jiang et al., 2015; Peng et al., 2019; Fang et al., 2020; Li et al., 2020), while coupling can also be accomplished via thiol-maleimide and coupled to a thiol-activated ligand. Albumin-shelled NBs also can bind to activated leukocytes via β2-integrins on leukocytes (Lindner et al., 2000b; Lindner et al., 2000c) and complement fragments (Anderson et al., 2007). In a more specific approach to targeting, different ligands such as antibody, peptides, and glycoproteins are conjugated to the shell surface. Additionally, the properties of targeted agent, hemodynamic, and target molecule are the major determinants for targeted UCA retention in areas of diseases (Brown and Lindner, 2019). To reduce the recognition by the immune system and increase half-life in circulation, the PEG spacer is used to conjugate with the targeting agent (Borden et al., 2013).
Preclinical application of nanosized ultrasound contrast agents
Ultrasound molecular imaging could potentially be used in early detection, monitoring treatment effects, and delivery of drugs. To date, there are various preclinical applications to explore the effect of targeted nUCAs (Wei et al., 1998; Yin et al., 2012).
Early detection and tumor characteristic
New effective targets overexpressed on tumor tissue and neovasculature are regarded as a breakthrough for early accurate diagnosis and characteristic. Guo et al. constructed lipid nanobubbles combined with AS1411 to highly target nucleolin in triple-negative breast cancer, which can realize molecular imaging of tumor tissues and neovasculature to provide an early detection method (Li et al., 2020). In addition, CA-125-targeted echogenic lipid and surfactant-stabilized nanobubbles were developed to enhance tumor accumulation, which may contribute to improved diagnosis of epithelial ovarian cancer (Gao et al., 2017). Additionally, targeted NBs linked to CSF-1R have been shown to successfully target the margin of hepatocarcinoma, thereby improving the efficiency of radiofrequency ablation (Wu et al., 2019). In addition, targeted NBs have been shown to target a variety of skin-derived tumors in vitro using PLGA-linked NBs to target residual tumors (Xu et al., 2010; Zhang et al., 2014), as an adjunct to synergistic radiofrequency ablation under high-intensity focused ultrasound (HIFU) (Watanabe et al., 2010; Perera et al., 2014). Using the energy of contrast agent rupture can significantly enhance the apoptosis of tumor cells. Some scholars successfully detected the early inflammatory response stage of atherosclerotic plaques by preparing a nanoscale UCA with magnetic targeting of VCAM-1 (Deshpande et al., 2016).
Monitoring treatment response
The expression level of the target molecules, which reflects the diseased or abnormal status, can be evaluated in vivo by quantitative analysis of the ultrasound contrast signal intensity. In order to monitor the survival of ovarian cells in the early transplantation, MU et al. developed AMH-targeted nanobubbles by integrating an AMH antibody onto the surface of NBs. Evidence showed that the ultrasound signal was relative with the expression of AMH in transplant time (Lindner et al., 2000b). In addition, targeted nanobubbles have been used to monitor tamoxifen resistance through the expression of carbonic anhydrase IX in breast cancer (Borden et al., 2013).
Imaging-guided delivery of therapy
Recently, NBs have been researched with regard to the efficient delivery of exogenous genes and drugs noninvasively (Maxim et al., 2019). A recent trend is that ultrasound-targeted NB destruction (UTND) plays a crucial role in improving the efficient delivery by sonoporation of NBs (Roberta et al., 2013; Li et al., 2021). Under ultrasound, cell membrane permeabilization and enhancing drug uptake can be caused by NBs. Notably, nontargeted NBs are readily swallowed by the reticuloendothelial system, thereby reducing the aggregation of target areas. Therefore, it is necessary to develop targeted and drug-loaded NBs to improve therapeutic effect and reduce off-target effect (Pote et al., 2021). Drug-loaded phase-transformation lipid nanoparticles are a promising drug carrier that can provide both physical and chemical therapy in combination with ultrasound for molecular imaging and therapy (Li et al., 2018; Zhu et al., 2018). Zhu et al. have prepared phase-transformation lipid nanoparticles with paclitaxel loaded and anti-LHRHR targeted. This drug carrier can actively target and specifically kill ovarian-3 cells. At the same time, it can occur in liquid–gas phase-transformation under low-intensity focused ultrasound to enhance the ultrasound imaging (Gao et al., 2018). Additionally, nanobubbles combined with ultrasound-targeted destruction (UTD) have become potential carriers for gene delivery (Cai et al., 2018). The NB-siRNA nanoparticle was used to target NB-siRNA to improve siRNA transfection under ultrasound irradiation, which is effectively enhancing the effect of siRNA transfection and in vitro silencing of targeted genes (Maxim et al., 2019).
Challenges of nanosized ultrasound contrast agents
Microsized ultrasound contrast agents have been commercialized and used in diagnosis and adjuvant treatment with less and mild adverse reactions. In contrast, the research of nUCAs have been widely explored (Liufu et al., 2020), while rarely used in clinical application. The main problems of the research are as follows. The decrease in signal intensity, the decrease in inner diameter of contrast agents, and the decrease in backscattering ability are urgent problems to be solved in the research of nUCAs. It is difficult to accurately control the size of nUCAs that not only cross the vasculature but also have a strong scattering performance. In addition, the shell and core of the nUCAs will affect the stability and residence time. Thus, how to reduce the size of nUCAs and increase the signal becomes the focus of future research. The low concentration of target tissue aggregation has been confirmed by many experiments, and how to choose the best ligand and receptor needs to be further explored. Besides the influence of nUCAs materials on normal tissue, the accuracy of lesion location and the optimization of ultrasonic instrument parameters need to be further explored. With the continuous cross fusion of biomedical and clinical medical technology, the existing problems of nano contrast agents will be solved continuously, and the safety will be improved. It is believed that ultrasound contrast agents will play an irreplaceable role in the early diagnosis and accurate treatment of clinical diseases in the future.
Future perspective
The feasibility of ultrasound molecular imaging studies has been demonstrated in numerous preclinical studies and used in different disease models. Therefore, ultrasound molecular imaging has been used in understanding the progression of disease mechanisms, which has also been used in preclinical testing of the efficacy of new drugs. This field is expected to expand with better standardization of target contrast agents and ultrasound imaging protocols. As more and more targeted nanosized ultrasound contrast agents are developed, the ability to characterize specific disease phenotypes will become critical. However, the widespread use of nUCAs in the clinic will depend on additional, extensive, and larger clinical trials that demonstrate safety. In addition, the integration of clinical information obtained from molecular imaging will need to be integrated into diagnostic and therapeutic pathways to improve diagnostic accuracy.
Executive summary
Nanosized ultrasound contrast agents have been successfully used in early diagnosis and response to therapy.
Nanosized ultrasound contrast agents have several challenges, such as the decrease in signal intensity, complex preparation process, the low concentration in the targeted area, and so on.
Author Contributions
ZC is responsible for the design and guidance of the review. FZ is responsible for the literature research and analysis, and article preparation. MD is responsible for language polishing and article modification.
Funding
This work was supported by the National Key R&D Program of China (2019YFE0110400), National Natural Science Foundation of China (81971621), Natural Science Foundation of Guangdong Province (No. 2021A1515011177), Natural Science Foundation of Guangdong Province (No. 2020A1515110628), and Natural Science Foundation of Guangdong Province (No. 2019A1515012212).
Conflict of Interest
The authors declare that the research was conducted in the absence of any commercial or financial relationships that could be construed as a potential conflict of interest.
Publisher’s Note
All claims expressed in this article are solely those of the authors and do not necessarily represent those of their affiliated organizations, or those of the publisher, the editors, and the reviewers. Any product that may be evaluated in this article, or claim that may be made by its manufacturer, is not guaranteed or endorsed by the publisher.
References
Abenojar, E. C., Bederman, I., de Leon, A. C., Zhu, J., Hadley, J., Kolios, M. C., et al. (2020). Theoretical and Experimental Gas Volume Quantification of Micro- and Nanobubble Ultrasound Contrast Agents. Pharmaceutics 12 (3), 208. doi:10.3390/pharmaceutics12030208
Anderson, D., Tsutsui, J., Xie, F., Radio, S., and Porter, T. (2007). The Role of Complement in the Adherence of Microbubbles to Dysfunctional Arterial Endothelium and Atherosclerotic Plaque. Cardiovasc. Res. 73, 597–606. doi:10.1016/j.cardiores.2006.11.029
Azevedo, A., Oliveira, H., and Rubio, J. (2019). Bulk Nanobubbles in the mineral and Environmental Areas: Updating Research and Applications. Adv. Colloid Interf. Sci. 271, 101992. doi:10.1016/j.cis.2019.101992
Borden, M. A., Streeter, J. E., Sirsi, S. R., and Dayton, P. A. (2013). In Vivo demonstration of Cancer Molecular Imaging with Ultrasound Radiation Force and Buried-Ligand Microbubbles. Mol. Imaging 12, 357–363. doi:10.2310/7290.2013.00052
Bourdeau, R. W., Lee-Gosselin, A., Lakshmanan, A., Farhadi, A., Kumar, S. R., Nety, S. P., et al. (2018). Acoustic Reporter Genes for Noninvasive Imaging of Microorganisms in Mammalian Hosts. Nature 553 (7686), 86–90. doi:10.1038/nature25021
Brown, E., and Lindner, J. R. (2019). Ultrasound Molecular Imaging: Principles and Applications in Cardiovascular Medicine. Curr. Cardiol. Rep. 21 (5), 30. doi:10.1007/s11886-019-1117-9
Buchholz, B. E. E., Hayes, P. k., and Walsby, A. E. (1993). The Distribution of the Outer Gas Vesicle Protein, Gvpc, on the Anabaena Gas Vesicle, and its Ratio to Gvpa. J. Gen. Microbiol. 139 (10), 2353–2363. doi:10.1099/00221287-139-10-2353
Cai, W., Lv, W., Feng, Y., Yang, H., Zhang, Y., Yang, G., et al. (2018). The Therapeutic Effect in Gliomas of Nanobubbles Carrying siRNA Combined with Ultrasound-Targeted Destruction. Int. J. Nano. 13, 6791–6807. doi:10.2147/ijn.s164760
Cheng, K. T. (2004). Perflutren Lipid Microspheres. Bethesda: National Center for Biotechnology Information.
Chomas, J. E., Dayton, P., Allen, J., Morgan, K., and Ferrara, K. W. (2001). Mechanisms of Contrast Agent Destruction. IEEE Trans. Ultrason. Ferroelect., Freq. Contr. 48, 232–248. doi:10.1109/58.896136
Craig, V. (2010). Very Small Bubbles at Surfaces—The Nanobubble Puzzle. Soft Matter 7 (1), 40–48. doi:10.1039/C0SM00558D
de Leon, Al., Perera, R., and Hernandez, C. (2019). Contrast Enhanced Ultrasound Imaging by Nature-Inspired Ultrastable Echogenic Nanobubbles. Nanoscale 11 (33), 15647–15658. doi:10.1039/c9nr04828f
Deshpande, D., Kethireddy, S., Janero, D. R., and Amiji, M. M. (2016). Therapeutic Efficacy of an ω-3-Fatty Acid-Containing 17-β Estradiol Nano-Delivery System against Experimental Atherosclerosis. PLoS One 11 (2), e0147337. doi:10.1371/journal.pone.0147337
Fang, K., Wang, L., Huang, H., Lan, M., Shen, D., Dong, S., et al. (2020). Construction of Nucleolin-Targeted Lipid Nanobubbles and Contrast-Enhanced Ultrasound Molecular Imaging in Triple-Negative Breast Cancer. Pharm. Res. 37, 145. doi:10.1007/s11095-020-02873-1
Gao, Y., Chen, S., Li, W., Wang, H., Xiao, K., Wu, L., et al. (2018). An Experimental Study of Ovarian Cancer Imaging and Therapy by Paclitaxel-Loaded Phase-Transformation Lipid Nanoparticles Combined with Low-Intensity Focused Ultrasound. Biochem. Biophys. Res. Commun. 504, 203–210. doi:10.1016/j.bbrc.2018.08.156
Gao, Y., Hernandez, C., Yuan, H. X., Lilly, J., Kota, P., Zhou, H., et al. (2017). Ultrasound Molecular Imaging of Ovarian Cancer with CA-125 Targeted Nanobubble Contrast Agents. Nanomedicine 13, 2159–2168. doi:10.1016/j.nano.2017.06.001
Garrute, F. V., and Machado, J. C. (2020). Gas Vesicle Isolated from Microorganisms to Act as Ultrasound Contrast Agent. Res. Biomed. Eng. 37, 1–7. doi:10.1007/s42600-020-00093-5
Gorce, J.-M., Arditi, M., and Schneider, M. (2000). Influence of Bubble Size Distribution on the Echogenicity of Ultrasound Contrast Agents. Invest. Radiol. 35, 661–671. doi:10.1097/00004424-200011000-00003
Hernandez, C., Nieves, L., De Leon, C., et al. (2018). Role of Surface Tension in Gas Nanobubble Stability under Ultrasound. ACS Appl. Mater. Inter. doi:10.1021/acsami.7b19755
Hernandez, C., Gulati, S., Fioravanti, G., Stewart, P. L., and Exner, A. A. (2017). Cryo-EM Visualization of Lipid and Polymer-Stabilized Perfluorocarbon Gas Nanobubbles - A Step towards Nanobubble Mediated Drug Delivery. Sci. Rep. 7, 13517. doi:10.1038/s41598-017-13741-1
Huang, R., Lin, J., Gao, D., Zhang, F., Yi, L., Huang, Y., et al. (2019). Discovery of Gas Vesicles in Streptomyces Sp. CB03234-S and Potential Effects of Gas Vesicle Gene Overexpression on Morphological and Metabolic Changes in Streptomycetes. Appl. Microbiol. Biotechnol. 103 (14), 5751–5761. doi:10.1007/s00253-019-09891-z
Ignee, A., Atkinson, N., and Schuessler, G. (2016). Ultrasound Contrast Agents. Endoscopic Ultrasound 5 (6), 355–362. doi:10.4103/2303-9027.193594
Jiang, Q., Zeng, Y., Xu, Y., Xiao, X., Liu, H., Zhou, B., et al. (2020). Ultrasound Molecular Imaging as a Potential Non-invasive Diagnosis to Detect the Margin of Hepatocarcinoma via CSF-1R Targeting. Front. Bioeng. Biotechnol. 8, 783. doi:10.3389/fbioe.2020.00783
Jiang, Q. C., Hao, S. Y., and Xiao, X. Y. (2015). Production and Characterization of a Novel Long-Acting Herceptin-Targeted Nanobubble Contrast Agent Specific for Her-2-Positive Breast Cancers, 23. Tokyo, Japan: Breast cancer. doi:10.1007/s12282-014-0581-8
Jiang, Q., Hao, S., Xiao, X., Yao, J., Ou, B., Zhao, Z., et al. (2016). Production and Characterization of a Novel Long-Acting Herceptin-Targeted Nanobubble Contrast Agent Specific for Her-2-Positive Breast Cancers. Breast Cancer 23, 445–455. doi:10.1007/s12282-014-0581-8
Kanbar, E., Fouan, D., Sennoga, C. A., Doinikov, A. A., and Bouakaz, A. (2017). Impact of Filling Gas on Subharmonic Emissions of Phospholipid Ultrasound Contrast Agents. Ultrasound Med. Biol. 43, 1004–1015. doi:10.1016/j.ultrasmedbio.2016.12.013
Klibanov, A. L. (2002). Ultrasound Contrast Agents: Development of the Field and Current Status. Topics in Current Chemistry, 222, 73–106. doi:10.1007/3-540-46009-8_3
Krupka, T. M., Solorio, L., Wilson, R. E., Wu, H., Azar, N., and Exner, A. A. (2010). Formulation and Characterization of Echogenic Lipid−Pluronic Nanobubbles. Mol. Pharmaceutics 7 (1), 49–59. doi:10.1021/mp9001816
Lakshmanan, A., Farhadi, A., Nety, S. P., Lee-Gosselin, A., Bourdeau, R. W., Maresca, D., et al. (2016). Molecular Engineering of Acoustic Protein Nanostructures. ACS Nano 10 (10), 7314–7322. doi:10.1021/acsnano.6b03364
Li, M., Luo, H., Zhang, W., He, K., Chen, Y., Liu, J., et al. (2018). Phase-shift, Targeted Nanoparticles for Ultrasound Molecular Imaging by Low Intensity Focused Ultrasound Irradiation. Ijn Vol. 13, 3907–3920. doi:10.2147/ijn.s166200
Li, Y., Chen, X., Zhou, Z., Li, Q., Westover, K. D., Wang, M., et al. (2020). Dynamic Surveillance of Tamoxifen-Resistance in ER-Positive Breast Cancer by CAIX-Targeted Ultrasound Imaging. Cancer Med. 9, 2414–2426. doi:10.1002/cam4.2878
Li, Y., Chen, Z., and Ge, S. (2021). Sonoporation: Underlying Mechanisms and Applications in Cellular Regulation. BIO Integration 2 (1), 29–36. doi:10.15212/bioi-2020-0028
Lindner, J. R., Coggins, M. P., Kaul, S., Klibanov, A. L., Brandenburger, G. H., and Ley, K. (2000). Microbubble Persistence in the Microcirculation during Ischemia/reperfusion and Inflammation Is Caused by Integrin- and Complement-Mediated Adherence to Activated Leukocytes. Circulation 101, 668–675. doi:10.1161/01.cir.101.6.668
Lindner, J. R., Dayton, P. A., Coggins, M. P., Ley, K., Song, J., Ferrara, K., et al. (2000). Noninvasive Imaging of Inflammation by Ultrasound Detection of Phagocytosed Microbubbles. Circulation 102, 531–538. doi:10.1161/01.cir.102.5.531
Lindner, J. R., Song, J., Christiansen, J., Klibanov, A. L., Xu, F., and Ley, K. (2001). Ultrasound Assessment of Inflammation and Renal Tissue Injury with Microbubbles Targeted to P-Selectin. Circulation 104, 2107–2112. doi:10.1161/hc4201.097061
Lindner, J. R., Song, J., Xu, F., Klibanov, A. L., Singbartl, K., Ley, K., et al. (2000). Noninvasive Ultrasound Imaging of Inflammation Using Microbubbles Targeted to Activated Leukocytes. Circulation 102, 2745–2750. doi:10.1161/01.cir.102.22.2745
Liu, X. Q., Zhou, L. Y., and Zhang, T. A. (2021). Review on Preparation and Adsorption Properties of Chitosan and Chitosan Composites. Polym. Bull., 1–33. doi:10.1007/s00289-021-03626-9
Liufu, C., Li, Y., Lin, Y., Yu, J., Du, M., Chen, Y., et al. (2020). Synergistic Ultrasonic Biophysical Effect-Responsive Nanoparticles for Enhanced Gene Delivery to Ovarian Cancer Stem Cells. Drug Deliv. 27 (1), 1018–1033. doi:10.1080/10717544.2020.1785583
Maxim, B., Josquin, F., and Galina, S. (2019). Nonviral Ultrasound-Mediated Gene Delivery in Small and Large Animal Models. Nat. Protoc. 14 (4), 1015–1026. doi:10.1038/s41596-019-0125-y
Nguyen, P. N., Trinh Dang, T. T., Waton, G., Vandamme, T., and Krafft, M. P. (2011). A Nonpolar, Nonamphiphilic Molecule Can Accelerate Adsorption of Phospholipids and Lower Their Surface Tension at the Air/water Interface. ChemPhysChem 12, 2646–2652. doi:10.1002/cphc.201100425
Omata, D., Maruyama, T., Unga, J., Hagiwara, F., Munakata, L., Kageyama, S., et al. (2019). Effects of Encapsulated Gas on Stability of Lipid-Based Microbubbles and Ultrasound-Triggered Drug Delivery. J. Controlled Release 311-312, 65–73. doi:10.1016/j.jconrel.2019.08.023
Omata, D., Unga, J., Suzuki, R., and Maruyama, K. (2020). Lipid-based Microbubbles and Ultrasound for Therapeutic Application. Adv. Drug Deliv. Rev. 154-155, 236–244. doi:10.1016/j.addr.2020.07.005
Paefgen, V., Doleschel, D., and Kiessling, F. (2015). Evolution of Contrast Agents for Ultrasound Imaging and Ultrasound-Mediated Drug Delivery. Front. Pharmacol. 6, 197. doi:10.3389/fphar.2015.00197
Peng, Y., Zhu, L., Wang, L., Liu, Y., Fang, K., Lan, M., et al. (2019). Preparation of Nanobubbles Modified with A Small-Molecule CXCR4 Antagonist for Targeted Drug Delivery to Tumors and Enhanced Ultrasound Molecular Imaging. Int. J. Nano. 14, 9139–9157. doi:10.2147/ijn.s210478
Perera, R., Wu, H. P., Peiris, P., Hernandez, C., Burke, A., and Zhang, H. I. (2017). Improving Performance of Nano-Scale Ultrasound Contrast Agents Using N,N Diethylacrylamide Stabilization. Nanomedicine 13 (1), 59–67. doi:10.1016/j.nano.2016.08.020
Perera, R. H., de Leon, A., Wang, X., Wang, Y., Ramamurthy, G., Peiris, P., et al. (2020). Real Time Ultrasound Molecular Imaging of Prostate Cancer with PSMA-Targeted Nanobubbles. Nanomedicine: Nanotechnology, Biol. Med. 28, 102213. doi:10.1016/j.nano.2020.102213
Perera, R. H., Solorio, L., Wu, H., Gangolli, M., Silverman, E., Hernandez, C., et al. (2014). Nanobubble Ultrasound Contrast Agents for Enhanced Delivery of thermal Sensitizer to Tumors Undergoing Radiofrequency Ablation. Pharm. Res. 31 (6), 1407–1417. doi:10.1007/s11095-013-1100-x
Pfeifer, F. (2012). Distribution, Formation and Regulation of Gas Vesicles. Nat. Rev. Microbiol. 10, 705–715. doi:10.1038/nrmicro2834
Pote, A., Giri, M., and Vishal, P. (2021). State of Art Review on Nanobubbles. Adv. Mater. Lett. 12, 1–9. doi:10.5185/amlett.2021.031608
Pysz, M. A., Guracar, I., Tian, L., and Willmann, J. K. (2012). Fast Microbubble Dwell-Time Based Ultrasonic Molecular Imaging Approach for Quantification and Monitoring of Angiogenesis in Cancer. Quant. Imaging Med. Surg. 2, 68–80. doi:10.3978/j.issn.2223-4292.2012.06.05
Roberta, C., Agnese, B., and David, L. (2013). Micro- and Nanobubbles: A Versatile Non-viral Platform for Gene Delivery. Int. J. Pharmaceutics 456 (2), 437–445. doi:10.1016/j.ijpharm.2013.08.041
Sheeran, P., and Dayton, P. (2012). Phase-Change Contrast Agents for Imaging and Therapy. Cpd 18, 2152–2165. doi:10.2174/138161212800099883
Schutt, E. G., Klein, D. H., Mattrey, R. M., and Riess, J. G. (2003). Injectable Microbubbles as Contrast Agents for Diagnostic Ultrasound Imaging: The Key Role of Perfluorochemicals. Angew. Chem. Int. Ed. 42, 3218–3235. doi:10.1002/anie.200200550
Shapiro, M. G., Goodwill, P. W., Neogy, A., Yin, M., Foster, F. S., Schaffer, D. V., et al. (2014). Biogenic Gas Nanostructures as Ultrasonic Molecular Reporters. Nat. Nanotech. 9 (4), 311–316. doi:10.1038/nnano.2014.32
Sheeran, P. S., Streeter, J. E., Mullin, L. B., Matsunaga, T. O., and Dayton, P. A. (2013). Toward Ultrasound Molecular Imaging with Phase-Change Contrast Agents: an In Vitro Proof of Principle. Ultrasound Med. Biol. 39, 893–902. doi:10.1016/j.ultrasmedbio.2012.11.017
Tong, H.-P., Wang, L.-F., Guo, Y.-L., Li, L., Fan, X.-Z., Ding, J., et al. (2013). Preparation of Protamine Cationic Nanobubbles and Experimental Study of Their Physical Properties and In Vivo Contrast Enhancement. Ultrasound Med. Biol. 39, 2147–2157. doi:10.1016/j.ultrasmedbio.2013.05.016
Unga, J., and Hashida, M. (2014). Ultrasound Induced Cancer Immunotherapy. Adv. Drug Deliv. Rev. 72, 144–153. doi:10.1016/j.addr.2014.03.004
Walsby, A. E., and Hayes, P. K. (1989). Gas Vesicle Proteins. Biochem. J. 264 (2), 313–322. doi:10.1042/bj2640313
Wang, J.-P., Zhou, X.-L., Yan, J.-P., Zheng, R.-Q., and Wang, W. (2017). Nanobubbles as Ultrasound Contrast Agent for Facilitating Small Cell Lung Cancer Imaging. Oncotarget 8, 78153–78162. doi:10.18632/oncotarget.18155
Wang, L., Chen, S., Zhu, Y., Zhang, M., Tang, S., Li, J., et al. (2018). Triple-modal Imaging-Guided Chemo-Photothermal Synergistic Therapy for Breast Cancer with Magnetically Targeted Phase-Shifted Nanoparticles. ACS Appl. Mater. Inter. 10, 42102–42114. doi:10.1021/acsami.8b16323
Wang, Y., De Leon, A. C., Perera, R., Abenojar, E., Gopalakrishnan, R., Basilion, J. P., et al. (2021). Molecular Imaging of Orthotopic Prostate Cancer with Nanobubble Ultrasound Contrast Agents Targeted to PSMA. Sci. Rep. 11 (1), 4726. doi:10.1038/s41598-021-84072-5
Watanabe, Y., Horie, S., Funaki, Y., Kikuchi, Y., Yamazaki, H., Ishii, K., et al. (2010). Delivery of Na/I Symporter Gene into Skeletal Muscle Using Nanobubbles and Ultrasound: Visualization of Gene Expression by PET. J. Nucl. Med. 51 (6), 951–958. doi:10.2967/jnumed.109.074443
Wei, K., Jayaweera, A. R., Firoozan, S., Linka, A., Skyba, D. M., and Kaul, S. (1998). Quantification of Myocardial Blood Flow with Ultrasound-Induced Destruction of Microbubbles Administered as a Constant Venous Infusion. Circulation 97, 473–483. doi:10.1161/01.cir.97.5.473
Wu, B., Shang, H., Liang, X., Sun, Y., Jing, H., Han, X., et al. (2019). Preparation of Novel Targeting Nanobubbles Conjugated with Small Interfering RNA for Concurrent Molecular Imaging and Gene Therapy In Vivo. FASEB J. 33, 14129–14136. doi:10.1096/fj.201900716RR
Xu, J. S., Huang, J., Qin, R., Hinkle, G. H., Povoski, S. P., Martin, E. W., et al. (2010). Synthesizing and Binding Dual-Mode Poly (Lactic-co-glycolic Acid) (PLGA) Nanobubbles for Cancer Targeting and Imaging. Biomaterials 31 (7), 1716–1722. doi:10.1016/j.biomaterials.2009.11.052
Yan, J., Yang, H., Shen, X., Zhang, C., Wu, C., and Liu, Y. (2018). Co-delivery of Doxorubicin and P-Glycoprotein siRNA Using pH-Sensitive PLGA Nanobubbles for Synergistic Tumor Therapy. Nanomedicine: Nanotechnology, Biol. Med. 14, 1815. doi:10.1016/j.nano.2017.11.207
Yang, H., Zhou, T., Cai, W., Yi, X., Liu, X., Wang, Y., et al. (2016). Novel Dual-Mode Nanobubbles as Potential Targeted Contrast Agents for Female Tumors Exploration. Tumour Biol. 37, 14153–14163. doi:10.1007/s13277-016-5238-0
Yin, T., Wang, P., Zheng, R., Zheng, B., Cheng, D., Zhang, X., et al. (2012). Nanobubbles for Enhanced Ultrasound Imaging of Tumors. Int. J. Nanomedicine 7, 895–904. doi:10.2147/IJN.S28830
Yu, J., Chen, Z., and Yan, F. (2019). Advances in Mechanism Studies on Ultrasonic Gene Delivery at Cellular Level. Prog. Biophys. Mol. Biol. 142, 1–9. doi:10.1016/j.pbiomolbio.2018.07.012
Zhang, S., Di, N., Tayier, B., Guan, L., Wang, G., Lu, H., et al. (2020). Early Evaluation of Survival of the Transplanted Ovaries through Ultrasound Molecular Imaging via Targeted Nanobubbles. Biomater. Sci. 8, 5402–5414. doi:10.1039/d0bm01125h
Zhang, X., Zheng, Y., Wang, Z., Huang, S., Chen, Y., Jiang, W., et al. (2014). Methotrexate-loaded PLGA Nanobubbles for Ultrasound Imaging and Synergistic Targeted Therapy of Residual Tumor during HIFU Ablation. Biomaterials 35 (19), 5148–5161. doi:10.1016/j.biomaterials.2014.02.036
Zhu, L., Zhao, H., Zhou, Z., Xia, Y., Wang, Z., Ran, H., et al. (2018). Peptide-Functionalized Phase-Transformation Nanoparticles for Low Intensity Focused Ultrasound-Assisted Tumor Imaging and Therapy. Nano Lett. 18, 1831–1841. doi:10.1021/acs.nanolett.7b05087
Keywords: nanosized, contrast agents, ultrasound molecular imaging, nanobubbles, gas vesicles
Citation: Zeng F, Du M and Chen Z (2021) Nanosized Contrast Agents in Ultrasound Molecular Imaging. Front. Bioeng. Biotechnol. 9:758084. doi: 10.3389/fbioe.2021.758084
Received: 13 August 2021; Accepted: 08 October 2021;
Published: 29 November 2021.
Edited by:
Laura Mezzanotte, Erasmus Medical Center, NetherlandsReviewed by:
Alessandra Quarta, Italian National Research Council, ItalyEhsan Nazarzadeh Zare, Damghan University, Iran
Copyright © 2021 Zeng, Du and Chen. This is an open-access article distributed under the terms of the Creative Commons Attribution License (CC BY). The use, distribution or reproduction in other forums is permitted, provided the original author(s) and the copyright owner(s) are credited and that the original publication in this journal is cited, in accordance with accepted academic practice. No use, distribution or reproduction is permitted which does not comply with these terms.
*Correspondence: Zhiyi Chen, zhiyi_chen@usc.edu.cn