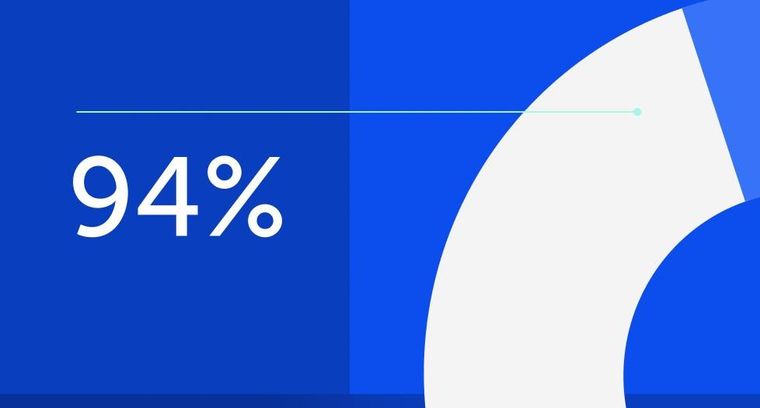
94% of researchers rate our articles as excellent or good
Learn more about the work of our research integrity team to safeguard the quality of each article we publish.
Find out more
ORIGINAL RESEARCH article
Front. Bioeng. Biotechnol., 19 November 2021
Sec. Synthetic Biology
Volume 9 - 2021 | https://doi.org/10.3389/fbioe.2021.757953
This article is part of the Research TopicElectrobiotechnology towards Sustainable Bioeconomy: Fundamental, Optimization and ApplicationsView all 6 articles
Background: Microbial fuel cells (MFCs) are a novel bioelectrochemical devices that can use exoelectrogens as biocatalyst to convert various organic wastes into electricity. Among them, acetate, a major component of industrial biological wastewater and by-product of lignocellulose degradation, could release eight electrons per mole when completely degraded into CO2 and H2O, which has been identified as a promising carbon source and electron donor. However, Shewanella oneidensis MR-1, a famous facultative anaerobic exoelectrogens, only preferentially uses lactate as carbon source and electron donor and could hardly metabolize acetate in MFCs, which greatly limited Coulombic efficiency of MFCs and the capacity of bio-catalysis.
Results: Here, to enable acetate as the sole carbon source and electron donor for electricity production in S. oneidensis, we successfully constructed three engineered S. oneidensis (named AceU1, AceU2, and AceU3) by assembling the succinyl-CoA:acetate CoA-transferase (SCACT) metabolism pathways, including acetate coenzyme A transferase encoded by ato1 and ato2 gene from G. sulfurreducens and citrate synthase encoded by the gltA gene from S. oneidensis, which could successfully utilize acetate as carbon source under anaerobic and aerobic conditions. Then, biochemical characterizations showed the engineered strain AceU3 generated a maximum power density of 8.3 ± 1.2 mW/m2 with acetate as the sole electron donor in MFCs. In addition, when further using lactate as the electron donor, the maximum power density obtained by AceU3 was 51.1 ± 3.1 mW/m2, which approximately 2.4-fold higher than that of wild type (WT). Besides, the Coulombic efficiency of AceU3 strain could reach 12.4% increased by 2.0-fold compared that of WT, which demonstrated that the engineered strain AceU3 can further utilize acetate as an electron donor to continuously generate electricity.
Conclusion: In the present study, we first rationally designed S. oneidensis for enhancing the electron generation by using acetate as sole carbon source and electron donor. Based on synthetic biology strategies, modular assembly of acetate metabolic pathways could be further extended to other exoelectrogens to improve the Coulombic efficiency and broaden the spectrum of available carbon sources in MFCs for bioelectricity production.
During the last few decades, energy exhaustion, water scarcity and environmental pollution have been among the greatest challenges of our time. Therefore, researchers are increasingly interested in the development of cost-effective methods for capturing potential energy from high-concentration saline wastewater. Bioelectrochemical systems (BESs), such as microbial electrolysis cells (Luo et al., 2011; Zhang and Angelidaki, 2014; Jafary et al., 2015) (MECs) and microbial fuel cells (Chaudhuri and Lovley, 2003; Logan, 2009; Logan and Rabaey, 2012; Zhao et al., 2020) (MFCs) are promising technologies for sustainable power generation and contaminants degradation (Xie et al., 2013; Wang et al., 2015; Butti et al., 2016; Mohan et al., 2016; Kronenberg et al., 2017; Kumar et al., 2017; Santoro et al., 2017; Zhen et al., 2017; Ilamathi and Jayapriya, 2018). In particular, MFCs can directly harvest electrical power from wastewater by employing electroactive microorganisms (Li et al., 2018a; Li et al., 2018b). Compared with traditional electrocatalysis systems, much variety of substrates which include organic acids (lactate (Chae et al., 2009), acetate (You et al., 2015), pyruvate (Pillot et al., 2020) etc.) and various carbohydrates (glucose (Chae et al., 2009), starch (Han et al., 2020) etc.) in wastewater and marine sediment could be used as electron donors/acceptors for BESs due to diversified electrochemically active bacteria (EAB). As a major component of industrial biological wastewater and lignocellulosic biomass hydrolysate, acetate has been paid great attention to bio-manufacturing and has a strong potential to compete with sugar-based carbon source (Lim et al., 2018; Novak and Pflügl, 2018). However, utilization of acetate is limited in many EAB by some biological factors which like lack of uptake transporters (Enerson and Drewes, 2003; Gimenez et al., 2003; Morris and Felmlee, 2008), energetic requirements for transport (Lin et al., 2006), or the availability of enzymes to oxidize substrates (Brown et al., 1977; Kumari et al., 1995; Dittrich et al., 2005; Shi et al., 2005).
Shewanella oneidensis MR-1, one of the most widely studied dissimilatory metal-reducing bacterium, can conduct extracellular electrons transfer (EET) through its electroactive biofilm. It has been extensively studied for dissecting inward EET mechanism (Pirbadian et al., 2014; Kumar et al., 2016; Xiao et al., 2017; Light et al., 2018), developing gene editing tools (Yu et al., 2011; Liu et al., 2015; Yang et al., 2015), exploring novel technologies for polymer and nanoparticle synthesis (Yong et al., 2012; Yong et al., 2014; Yu et al., 2015), bioremediations of toxic metals (Vikrant et al., 2018), and electro-fermentations (Logan et al., 2008; Varrone et al., 2014; Kitching et al., 2017) in recent decades. However, S. oneidensis MR-1 has great limitation in substrate utilization, which hinder its practical applications in BESs. Lactate, as a preferential electron donor, could only be consumed and converted to acetate, which could not to be metabolized to CO2 and H2O under anaerobic conditions in MFCs, resulting insufficient utilization of lactate and leaving eight electrons in acetate. Even to enhance the efficiency of electron donor utilization, constructing a synthetic microbial consortium does not completely release electrons. Thus, the incomplete utilization of electron donors of S. oneidensis extremely decreases the Coulombic efficiency of MFCs and restricts the development and applications of various BES.
In microorganisms, acetate is usually metabolized to acetyl-CoA via three pathways catalyzed by: 1) acetate kinase phosphotransacetylase (ACKA-PTA) (Rose et al., 1954; Dittrich et al., 2005; Shi et al., 2005) encoded by ackA and pta, 2) acetyl-CoA synthetase (ACS) (Jogl and Tong, 2004) encoded by acs or 3) acetate coenzyme A transferase, encoded by ato1 and ato2 gene, respectively. Both of ACKA-PTA and ACS pathway need to consume ATP to drive acetate utilization, but acetate could be efficiently converted to acetyl-CoA by assistance of succinyl-CoA without ATP consumption in the third pathway in Geobacter sulfurreducens. ACKA-PTA pathway in S. oneidensis could metabolize acetate under aerobic conditions. However, acetate is hardly utilized for S. oneidensis due to insufficient energetic requirements by the interruption of tricarboxylic acid (TCA) cycle under anaerobic conditions. Thus, the crucial problem is how to broaden and strengthen lactate utilization efficiency of S. oneidensis to thoroughly release electrons.
Recently, many strategies were used for improving bioelectricity production and Coulombic efficiency in Shewanella-inoculated MFCs that focus on increasing intracellular electrons generation and enhancing the extracellular electrons transfer rate of exoelectrogens (Li et al., 2019; Zhang et al., 2019). For example, redirecting metabolic flux towards NAD+ biosynthesis resulted more electrons from the increased oxidation of electron donor to EET pathways of S. oneidensis (Li et al., 2018c; Li et al., 2018d). Furthermore, enhancing flavins biosynthesis and transportation in a hydrophobic chassis of S. oneidensis could significantly boost its EET rate and performance (Lin et al., 2018). However, increasing EET rate and Coulombic efficiency via broadening substrate spectrum and enhancing utilization rate, which are the rate-limiting steps of whole bioelectricity transfer process, have been largely neglected in the past.
Herein, to enable S. oneidensis to completely utilize acetate for the bioelectricity production in MFCs under anaerobic condition, we successfully constructed engineered S. oneidensis by assembling the succinyl-CoA:acetate CoA-transferase (SCACT) metabolism pathway (Figure 1). At the beginning, to broaden the substrate spectrum of S. oneidensis, acetate coenzyme A transferase encoded by ato1 and ato2 gene from G. sulfurreducens was heterologously expressed in S. oneidensis, which could catalyse acetate to acetyl-CoA without ATP consumption. Then, to further accelerate the acetate assimilation rate, we also overexpressed a citrate synthase encoded by the gltA gene in engineered S. oneidensis. Finally, an engineered strain was constructed and its ability to generate electricity using acetate or lactate was compared with wild-type strain. To the best of our knowledge, this is the first report on the rationally designed Shewanella that could use acetate as the carbon source and electron donor to produce electricity. Furthermore, this engineering strategy also offered the possibility of other exoelectrogens to broaden the spectrum of available carbon sources and improve the Coulombic efficiency in MFCs.
FIGURE 1. Construction of engineered S. oneidensis strains to enable acetate utilization and electricity generation based on synthetic biology strategies. A unique acetate utilization way constructing by ato1 and ato2 (the genes encoding succinyl: acetate coenzyme A transferase from G. sulfurreducens and gltA (the gene encoding citrate synthase) from S. oneidensis, which is free of energy-consuming and restores vitality of TCA in anaerobic conditions.
In S. oneidensis, acetate is usually converted to acetyl coenzyme A via ACS and ACKA/PTA pathways under aerobic conditions, and then enters the main metabolic pathway (Figure 1). However, under anaerobic condition in MFCs, both ACS and ACKA/PTA pathways have remarkably limited capacities for acetate assimilation and large accumulation of acetate can cause disturbances of intracellular pH homeostasis. Conversely, SCACT from G. sulfurreducens is a new pathway of acetate metabolism that enables acetate to obtain CoA directly from succinate-CoA to generate acetyl-CoA without consuming ATP and converts succinyl-CoA to succinate accelerating the TCA cycle (Ueki and Zhou, 2021). To realize S. oneidensis to continually utilize acetate as sole carbon source for the bioelectricity production in MFCs, we firstly adopted the synthetic biology strategies to heterologously express the acetate coenzyme A transferase encoded by ato1 and ato2 gene from G. sulfurreducens to catalyse acetate converted in to acetyl-CoA, which not only enhanced the assimilation capacity of acetate without energy consumption, but also accelerated the conversion of succinate-CoA to succinate of the TCA cycle. And then to further improve the flux of acetyl-CoA into the TCA cycle, a citrate synthase encoded by the gltA gene from S. oneidensis was further overexpressed to avoid excessive accumulation of acetyl-CoA, thus constructing acetate-fed S. oneidensis from reconstructing the SCACT pathway and enhancing the rate of acetate utilization.
The multi-module gene assembly was carried out as previously reported in S. oneidensis, a BiobrickTM compatible vector named pYYDT was inductively expressed by kanamycin (Yang et al., 2015). Furthermore, to enhance codon fitness, we used in vitro chemical synthesis of codon-optimized genes, rather than direct molecular cloning from other bacteria. We finally constructed engineered strains AceU1 (including ato1 and ato2 genes for succinyl-CoA:acetate CoA-transferase), AceU2 (including gltA gene for the citrate synthase) and AceU3 (including ato1, ato2 and gltA gene) for further improving the Coulombic efficiency of classical lactate fed MFCs, plasmid construction as shown in Supplementary Figure S1. Expression level of mRNA in each of these strains was quantified by real-time PCR (RT-PCR). Under the conditions of adding 0.75 mM IPTG as inducer, the transcriptional expression levels of ato1, ato2 and gltA were 5.0, 4.1 and 5.1 folds higher than the normalization gene, respectively (Supplementary Figure S2). This result indicated that the three target genes could be well expressed in AceUs.
The cell growth activity and acetate consumption of wild-type (WT, harboring the empty vector pYYDT) and three engineering S. oneidensis strains AceU1, AceU2 and AceU3, were cultured in SBM medium added with 10 mM acetate as sole carbon source under aerobic and anaerobic conditions, respectively.
Under aerobic condition, the growth curves of these engineered strains demonstrate that AceU1, AceU2 and AceU3 have better ability to grow in SBM medium with acetate as the sole carbon source than the WT (Figure 2A) Additionally, the engineering strain AceU3 showed a higher growth biomass than AceU1 and AceU2. The acetate consumption rate of engineered strains AceU3 is 0.40 mM/h, which is faster than that of AceU1 and AceU2 (0.25 mM/h and 0.30 mM/h) (Figure 2B). This indicated that the constructed acetate metabolic pathway has a positive effect on cell growth under aerobic conditions.
FIGURE 2. Growth curves and acetate consumption of the WT and the recombinant S. oneidensis strains under aerobic or anaerobic conditions. (A) Aerobic growth curve (OD600 ∼ t) in SBM supplemented with 10 mM acetate. (B) Acetate consumption under aerobic condition. (C) Anaerobic growth curve (OD600 ∼ t) in SBM supplemented with 10 mM acetate. (D) Acetate consumption under anaerobic condition. The error bars were calculated from triplicate experiments.
The respiratory of WT and engineered strains were also determined under anaerobic condition with acetate as unique carbon source. Unlike aerobic condition, WT strain was barely able to use acetate while engineered strains had significant growth advantages, indicated the substrate spectrum of S. oneidensis had been broadened. Growth rates of engineered strains AceU1 and AceU3 were similar to aerobic condition (Figure 2C). Acetate was consumed by AceU3 at a rate of ∼0.23 mM/h, which was faster than that of AceU1 and AceU2 (∼0.17 mM/h and ∼0.0022 mM/h) (Figure 2D). These results showed that the SCACT pathway is able to convert acetate to acetyl-CoA in one step with less energy consumption compared with the native ACS and ACKA/PTA pathways. Moreover, the AceU2 showed significantly different of acetate consumption rate under both culture conditions, which is 0.30 mM/h and 0.0022 mM/h, respectively. This result further suggested that inadequate downstream carbon flux of acetyl-CoA was a major limitation of acetate metabolism under aerobic condition, whereas the limited synthesis of ATP was insufficient to supply acetate metabolic equivalents under anaerobic condition, which limiting the ability to utilize acetate. In addition, the comparison of the three engineered strains showed that AceU3 had the highest capacity for acetate utilization, indicating that combination of reconstitution of the SCACT pathway and expression of gltA to enhance acetyl-coA conversion can accelerate the utilization of acetate. In conclusion, we broadened the substrate spectrum of S. oneidensis with enhanced acetate assimilation and tolerance capacity.
Under anaerobic condition, Dual-chamber MFCs were used to evaluate the electrochemical performance of engineered strains AceU1, AceU2, and AceU3 using acetate as the sole electron donor or carbon sources. The engineered strains and WT were inoculated into anodic chamber of MFCs, respectively. And output voltages were real-time recorded every 30 min with a data acquisition system.
Initially, 20 mM acetate was added as electron donor to evaluate the power output capacity of each engineered S. oneidensis strain in MFCs (Figure 3A). The WT and AceU2 strains could barely generate any voltage output with acetate as sole carbon source, which illustrated that WT and AceU2 strains could not utilize acetate. Whereas the engineered strains AceU1 and AceU3 could generate a maximum output voltage of∼35 ± 3.5 mV and∼50.2 ± 1.3 mV (n = 3), suggesting that genetically programmed SCACT pathway genes and citrate synthase genes could activate acetate metabolism and enabled acetate as the sole electron donor for electricity production. The electron transfer efficiency also was analyzed by linear sweep voltammetry (LSV) and cyclic voltammetry (CV) as shown in Figures 3B,C. The AceU3 strain had the best electrochemical performance with a peak power density of 8.3 ± 1.2 mW/m2 (Figure 3B). Among the three engineered strains, AceU3 is the combination of AceU1 and AceU2 functions, which showed better electrochemical properties. Current density of AceU3 was obviously improved compared to other strains, indicating a higher electron transfer efficiency (Figure 3C). Meanwhile, there were typical redox peaks in CV curves starting around −0.4 V (vs Ag/AgCl), which belongs to flavins-mediated extracellular electron transfer. It suggested that the novel degradation pathway not only accelerated acetate assimilation, but also in some way promoted the flavin-mediated electron transfer process. As shown in Figure 3D, the acetate metabolite concentrations in the anolyte during MFC discharge were quantified to evaluate electrochemical performance. Acetate consumption of AceU3 strains was significantly better than AceU1 and AceU2, and these results were consistent with the power generation. Thus, it proved the development of bioelectricity performances of engineered strains was brought out by the accelerated acetate utilization rate.
FIGURE 3. Electrochemical analysis of the electrical production properties of WT and engineered S. oneidensis strains (AceU1, AceU2 and AceU3) with acetate as carbon sources. (A) MFCs discharge curve of four strains WT, AceU1, AceU2 and AceU3, using acetate as carbon source. 20 mM acetate was added at the initiation of MFC operations. (B) MFCs power density output curves obtained by linear sweep voltammetry (LSV) with a slow scan rate of 0.1 mV/s. (C) Cyclic voltammetry (CV) analysis of four strains. (D) The acetate consumption curve in MFC.
It is well known that acetate is a secondary metabolite produced by incomplete oxidation of lactate which is one of the most favorable carbon sources under anaerobic conditions in S. oneidensis. Although we have successfully constructed engineered strains using acetate as a carbon source and electron donor, it is unknown whether the electrons in lactate can be fully obtained and then enhanced coulomb efficiency when using lactate as a substrate, which contains a higher number of electrons. To verify the changes of lactate utilization capacity and coulomb efficiency of the engineered strain, the multiple cycles of voltage output of AceU3 were measured in MFCs to assess multicycle operation (Figure 4A). After 760 h when output voltage was firstly decreased to baseline level, another 20 mM lactate was added to anode chamber. Both MFCs of WT and AceU3 showed stable electricity generation during two consecutively discharged cycles, but AceU3 duration in steady state was longer than that of WT in each cycle. Moreover, the output voltage of AceU3 (328.2 ± 6.0 mV and 375.4 ± 4.2 mV) in each cycle was higher than of that of WT (272.1 ± 5.5 mV and 298 ± 4.6 mV), indicating that some new redox reactions joined into the system and changed to anodic electrodynamic potential providing a more powerful driving force. Interestingly, the output voltage of AceU3 in second cycle was obviously higher than the first cycle, indicated that electroactive biofilms were formed on the surface of the electrodes during the first cycle. It suggested that the novel acetate metabolism pathway could promote the formation of electroactive biofilm. Another evidence for the engineered metabolism system facilitating electron transfer is the performance of power density. The power output showed that AceU3 had the highest power density (51.0 ± 3.1 mW/m2), which is 2.4 times higher than that of WT (21.1 ± 2.4 mW/m2) (Figure 4B).
FIGURE 4. Electrochemical performances analysis of WT and ET S. oneidensis in MFCs with lactate as carbon source. (A) Output voltage curves changed a part of anolyte with fresh anolyte when output voltage was completely declined. Catholyte was changed whenever needed. (B) MFCs power density output curves obtained by linear sweep voltammetry (LSV) with a fixed scan rate of 0.1 mV/s. (C) Coulombic efficiency of MFCs which inoculated WT and AceU3. (D) Cyclic voltammetry (CV) analysis of four strains. All error bars were calculated from triplicate experiments.
Furthermore, the Coulombic efficiency was also used to calculate the Coulombic rate between the actual recovered and the total amount in the substrate. And the Coulombic efficiency calculations showed that AceU3 had the highest Coulombic efficiency in MFC (12.4%) which was 2 times that of the WT strain (Figure 4C). It illustrated that the novel pathway could enhance lactate utilization efficiency and avoid more electrons lost in other processes. Besides, the dropping slope of polarization curves obtained from the strain AceU3 was smaller than one obtained from WT, implying that the internal charge transfer resistance of the MFC inoculated with AceU3 was relatively smaller (Supplementary Figure S3). Furthermore, to further study the EET efficiency of engineered strain AceU3, the cyclic voltammetry (CV) at 1 mV/s was applied to reveal the redox reaction kinetics. As shown in Figure 4D, there were typical redox peaks of flavin in the CV curves at ∼ −0.4V (vs Ag/AgCl), which indicated that the EET of AceU3 is similar with WT but with higher current density than that of WT. Meanwhile, biochemical characterization showed that the biosynthesis of flavin was increased in AceU3, confirming to the analysis results of CV (Figure 5A). In addition, the amount of attached biomass was assessed, and the formation of biofilm on the electrode surface was observed by confocal microscope (Figure 5B; Supplementary Figure S4). This result indicated that engineered strain AceU3 possessed a stronger drive to fully assimilate lactate, and accelerated the TCA cycle. Furthermore, it also illustrated that enhanced acetate consumption could enhance biofilm formation and improve cellular activity. It is speculated that this may be due to the enhanced synthesis and regulation of c-di-GMP by acetate metabolism, which could promote biofilm formation and EET in Shewanella (Mukherjee et al., 2020; Ng et al., 2020). In addition, the efficient biofilm formation on anodes in the engineered S. oneidensis strains enabled an accumulation of flavin which eventually enhanced EET efficiency and electricity generation.
FIGURE 5. Biochemical analyses of the engineered S. oneidensis and WT. (A) Determination of flavin concentration in the anolytes of MFCs, and (B) the attached biomass of each strain on anode surfaces. All error bars were calculated from triplicate experiments.
In the present study, we first rationally designed S. oneidensis for enhancing the electron generation by using acetate as sole carbon source and electron donor. To enable S. oneidensis to continually utilize acetate as the sole carbon source for bioelectricity generation in MFCs after lactate was completely consumed, we rationally constructed engineered S. oneidensis (namely AceUs) by heterologously overexpressing the citrate synthase (encoding by the gltA gene) and acetyl-CoA transferase (encoding by ato1 and ato2 gene) from Geobacter sulfurreducens. For the first time we broadened the substrate spectrum of S. oneidensis that could degrade acetate in MFCs and present an accelerated electricity transfer rate. Moreover, when using lactate as sole electron donor for MFCs, the maximum power density of AceU3 strain could reach 51.1 mW/m2, which was 2.4 times than that of WT strain. In addition, the Coulombic efficiency of AceU3 strain could reach 12.4%, surprisingly higher than that of the control strain (6.1%). Our work rationally engineered the metabolism of acetate after lactate was fully consumed and significantly enlarged the spectrum of carbon sources that could be taken by S. oneidensis.
The gltA gene encoding the citrate synthase, ato1 and ato2 genes encoding the acetyl-CoA transferase enzymes originated from Geobacter sulfurreducens PCA were identified in the NCBI database. Subsequently, the gene codon sequences were optimized for S. oneidensis MR-1 in Java codon adaption tool (JCAT) in order to replace rare codons of tRNAs to ensure the translation (Supplementary Table S1). The restriction enzyme sites of EcoRI, XbaI, SpeI and SbfI were avoided in the optimized sequences.
The plasmid construction was performed in E. coli Trans T1 that was cultured in the LB (Luria–Bertani) medium at 37°C with 220 rpm. The gene biobrick including gltA, ato1 and ato2 was inserted into the vector pYYDT to form the expression plasmid pYYDT-AceUs (see Supplementary Table S2 for primer sequences) by BioBrick® Assembly Kit (New England BioLabs inc., United States). The pYYDT- AceUs was firstly transformed into the plasmid donor strain E. coli WM3064 (a dap auxotroph) which was needed to add 100
Quantitative real-time reverse transcription polymerase chain reaction (qRT-PCR) can be used to analyze the expression of genes by quantifying the genes cDNA abundance. To analyze the expression of gltA, ato1 and ato2 genes, the recombinant Shewanella was induced by 0.75 mM IPTG. When in mid-log-phase cultures, total RNA was isolated by a bacterium total RNA extraction kit (APEXBIO, China). Then, the GoScript reverse transcription system (Promega, WI, United States) was used to synthesize the cDNA. Gene gyrB was used as normalization. Quantitative analyses of target gene expression were performed using SsoAdvanced SYBR Green supermix (Bio-Rad, CA, United States) (Cao et al., 2017). Samples were tested in triplicate using the listed primers (Supplementary Table S2).
To determine situation of cell growth, 2 ml culture suspension of the wild-type (WT) or engineered acetate-utilizing S. oneidensis strain was inoculated into 100 ml SBM medium. The cell cultures were incubated at 30°C 200 rpm, and samples were withdrawn periodically for the determination of cell density (optical density at 600 nm, i.e. OD600), and OD600 was measured by ultraviolet and visible spectrophotometer (TU-1810, Beijing, China) (Li et al., 2017). Both WT and AceU strain were cultured in 10 ml LB broth overnight at 30°C 200 rpm. Then, 3% inoculation amount of each suspension was transferred to SBM (pH 7.2) supplemented with 10 mM acetate as substrates. Whenever needed, 50 μg/ml kanamycin, 40 mM Fumarate and 0.75 mM IPTG were supplemented. The metabolites in the shake flasks were analyzed by a high-performance liquid chromatography (HPLC) system, which was equipped with a UV detector. All fermentation samples and standard solutions were pretreated by a 0.22 μm filter before HPLC testing. Acetate concentration was analyzed using HPLC with an Aminex HPX-87H column (Bio-Rad) at 65°C, using 5 mM H2SO4 as eluent, at a flow rate of 0.6 ml/min by UV spectrophotometer at 210 nm.
The WT strain and AceUs strain (harboring the empty vector pYYDT and pYYDT-AceU, respectively) from −80°C freezer stock were inoculated into 3 ml LB broth supplemented with 50 μg/ml kanamycin shaking at 30°C 200 rpm overnight. Then, 2 ml suspension was transferred to 100 ml LB broth with 50 μg/ml kanamycin and 0.75 mM IPTG as an inducer. When cultured around 12 h, the suspension was subsequently centrifuged 5,000 rpm for 10 min at 4°C. Finally, the cell pellets were adjusted to OD600 0.5 and dispersed into 140 ml anolyte. Carbon cloth was used as the electrodes for anode (2.5 cm
The total coulombs, where nx is the number of moles of substrate, bx is the moles of electrons per mole of substrate, and F is Faraday’s constant. The general procedure of lactate and acetate completely degradation can be described as:
which means each mole of lactate and acetate could contribute 12 and 8 mol electrons (
where MS (g/mol) is the molecular weight of the substrate, F is Faraday’s constant (98,485 C/mol of electrons), C is the symbol of Coulomb, I (A) is the current, tb (s) is the time period of a batch cycle, bES is the stoichiometric number of moles of electrons produced per mole of substrate, VAn (L) is the volume of liquid in the anode compartment, and Δc (g/L) is the substrate concentration change over the bath cycle time (Yan et al., 2015; Li et al., 2018e).
Electrochemical analysis is carried out at the steady state of the MFCs when the output voltage of the MFCs is at its maximum. Cyclic voltammetry (CV) analysis was conducted on a three-electrode mode with a scan rate of 1 mV/s, in which the Ag/AgCl as reference electrode by a CHI1000C multichannel electrochemical workstation (CH Instrument, Shanghai, China). To obtain the polarization curves, linear sweep voltammetry (LSV) analysis with a slow scan rate of 0.1 mV/s was conducted on a two-electrode mode by an electrochemical workstation CHI1000C (CH Instrument, Shanghai, China).
For determination of riboflavin content in MFC, the 5 ml of anode solution was collected, then centrifuged at 1,200 rpm/min for 5 min and filtered through a 0.22 μm filter membrane to remove bacteria. All standard solutions and samples were determined by HPLC with reverse-phase C18 column (10 cm × 2.1 mm, 5 μm) (Yang et al., 2015). Anodic attached biofilm measurement, the anode carbon cloth was collected and placed in a 50 ml test tube. Allow the cells to lyse and assay using the pierceTM BCA protein assay kit (made by Thermo) (Li et al., 2017). Biofilms biovolume and thickness in the anode carbon cloth were monitored using a CLSM (Nikon, Ti2-E full electric inverted microscope) (Mukherjee et al., 2018).
The raw data supporting the conclusion of this article will be made available by the authors, without undue reservation.
JZ, ZC and CL have contributed equally to this work, designed the project, performed experiments, analyzed data, and drafted the article. JL and XA helped to perform some experiments and drafted the manuscript; DW, XS, BZ and LF provided some reagents, helped design the experiment and drafted the article. FL and HS designed and supervised the project, analyzed data, and critically revised the article.
This research was supported by the National Key Research and Development Program of China (No. 2018YFA0901300), the National Natural Science Foundation of China (NSFC 32071411, 32001034 and 31701569), Tianjin Science and Technology Plan Project (20JCQNJC00830), Foundation of Basic Research Program (Foundation) of Guizhou Province (20201Y150), the Young Science and Technology Talents Growth Project of Education Department of Guizhou Province (KY2018445), Key Laboratory of Wuliangye-flavor Liquor Solid-state Fermentation, China National Light Industry (2021JJ013), and Tianjin Research Innovation Project for Postgraduate Students (2020YJSB045).
The authors declare that the research was conducted in the absence of any commercial or financial relationships that could be construed as a potential conflict of interest.
All claims expressed in this article are solely those of the authors and do not necessarily represent those of their affiliated organizations, or those of the publisher, the editors, and the reviewers. Any product that may be evaluated in this article, or claim that may be made by its manufacturer, is not guaranteed or endorsed by the publisher.
The Supplementary Material for this article can be found online at: https://www.frontiersin.org/articles/10.3389/fbioe.2021.757953/full#supplementary-material
BES, bioelectrochemical systems; CV, cyclic voltammetry; ET, engineered type; EET, extracellular electron transfer; LSV, linear sweep voltammetry; MECs, microbial electrolytic cells; MFCs, microbial fuel cells; WT, wild type; TCA, tricarboxylic acid cycle.
Brown, T. D. K., Jones-Mortimer, M. C., and Kornberg, H. L. (1977). The Enzymic Interconversion of Acetate and Acetyl-Coenzyme A in Escherichia coli. J. Gen. Microbiol. 102 (2), 327–336. doi:10.1099/00221287-102-2-327
Butti, S. K., Velvizhi, G., Sulonen, M. L. K., Haavisto, J. M., Oguz Koroglu, E., Yusuf Cetinkaya, A., et al. (2016). Microbial Electrochemical Technologies with the Perspective of Harnessing Bioenergy: Maneuvering towards Upscaling. Renew. Sustain. Energ. Rev. 53, 462–476. doi:10.1016/j.rser.2015.08.058
Cao, Y., Li, X., Li, F., and Song, H. (2017). CRISPRi-sRNA: Transcriptional-Translational Regulation of Extracellular Electron Transfer in Shewanella Oneidensis. ACS Synth. Biol. 6 (9), 1679–1690. doi:10.1021/acssynbio.6b00374
Chae, K.-J., Choi, M.-J., Lee, J.-W., Kim, K.-Y., and Kim, I. S. (2009). Effect of Different Substrates on the Performance, Bacterial Diversity, and Bacterial Viability in Microbial Fuel Cells. Bioresour. Technol. 100 (14), 3518–3525. doi:10.1016/j.biortech.2009.02.065
Chaudhuri, S. K., and Lovley, D. R. (2003). Electricity Generation by Direct Oxidation of Glucose in Mediatorless Microbial Fuel Cells. Nat. Biotechnol. 21 (10), 1229–1232. doi:10.1038/nbt867
Dittrich, C. R., Vadali, R. V., Bennett, G. N., and San, K. Y. (2005). Redistribution of Metabolic Fluxes in the central Aerobic Metabolic Pathway of E. coli Mutant Strains with Deletion of the ackA-Pta and poxB Pathways for the Synthesis of Isoamyl Acetate. Biotechnol. Prog. 21 (2), 627–631. doi:10.1021/bp049730r
Enerson, B. E., and Drewes, L. R. (2003). Molecular Features, Regulation, and Function of Monocarboxylate Transporters: Implications for Drug Delivery. J. Pharm. Sci. 92 (8), 1531–1544. doi:10.1002/jps.10389
Gimenez, R., Nuñez, M. F., Badia, J., Aguilar, J., and Baldoma, L. (2003). The Gene yjcG , Cotranscribed with the Gene Acs , Encodes an Acetate Permease in Escherichia coli. J. Bacteriol. 185 (21), 6448–6455. doi:10.1128/jb.185.21.6448-6455.2003
Han, W., Liu, Y., Xu, X., He, H., Chen, L., Tian, X., et al. (2020). A Novel Combination of Enzymatic Hydrolysis and Microbial Fuel Cell for Electricity Production from Bakery Waste. Bioresour. Technol. 297, 122387. doi:10.1016/j.biortech.2019.122387
Ilamathi, R., and Jayapriya, J. (2018). Microbial Fuel Cells for Dye Decolorization. Environ. Chem. Lett. 16 (1), 239–250. doi:10.1007/s10311-017-0669-4
Jafary, T., Daud, W. R. W., Ghasemi, M., Kim, B. H., Md Jahim, J., Ismail, M., et al. (2015). Biocathode in Microbial Electrolysis Cell; Present Status and Future Prospects. Renew. Sustain. Energ. Rev. 47, 23–33. doi:10.1016/j.rser.2015.03.003
Jogl, G., and Tong, L. (2004). Crystal Structure of Yeast Acetyl-Coenzyme A Synthetase in Complex with AMP. Biochemistry 43 (6), 1425–1431. doi:10.1021/bi035911a
Kitching, M., Butler, R., and Marsili, E. (2017). Microbial Bioelectrosynthesis of Hydrogen: Current Challenges and Scale-Up. Enzyme Microb. Technol. 96, 1–13. doi:10.1016/j.enzmictec.2016.09.002
Kronenberg, M., Trably, E., Bernet, N., and Patureau, D. (2017). Biodegradation of Polycyclic Aromatic Hydrocarbons: Using Microbial Bioelectrochemical Systems to Overcome an Impasse. Environ. Pollut. 231, 509–523. doi:10.1016/j.envpol.2017.08.048
Kumar, R., Singh, L., and Zularisam, A. W. (2016). Exoelectrogens: Recent Advances in Molecular Drivers Involved in Extracellular Electron Transfer and Strategies Used to Improve it for Microbial Fuel Cell Applications. Renew. Sustain. Energ. Rev. 56, 1322–1336. doi:10.1016/j.rser.2015.12.029
Kumar, A., Hsu, L. H.-H., Kavanagh, P., Barriere, F., Lens, P. N. L., Lapinsonniere, L., et al. (2017). The Ins and Outs of Microorganism-Electrode Electron Transfer Reactions. Nat. Rev. Chem. 1 (3), 1–13. doi:10.1038/s41570-017-0024
Kumari, S., Tishel, R., Eisenbach, M., and Wolfe, A. J. (1995). Cloning, Characterization, and Functional Expression of Acs, the Gene Which Encodes Acetyl Coenzyme A Synthetase in Escherichia coli. J. Bacteriol. 177 (10), 2878–2886. doi:10.1128/jb.177.10.2878-2886.1995
Li, F., Li, Y., Sun, L., Li, X., Yin, C., An, X., et al. (2017). Engineering Shewanella Oneidensis Enables Xylose-Fed Microbial Fuel Cell. Biotechnol. Biofuels 10, 196. doi:10.1186/s13068-017-0881-2
Li, F., Wang, L., Liu, C., Wu, D., and Song, H. (2018). Engineering Exoelectrogens by Synthetic Biology Strategies. Curr. Opin. Electrochem. 10, 37–45. doi:10.1016/j.coelec.2018.03.030
Li, F., Yin, C., Sun, L., Li, Y., Guo, X., and Song, H. (2018). Synthetic Klebsiella Pneumoniae-Shewanella Oneidensis Consortium Enables Glycerol-Fed High-Performance Microbial Fuel Cells. Biotechnol. J. 13 (5), e1700491–8. doi:10.1002/biot.201700491
Li, F., Li, Y. X., Cao, Y. X., Wang, L., Liu, C. G., Shi, L., et al. (2018). Modular Engineering to Increase Intracellular NAD(H/+) Promotes Rate of Extracellular Electron Transfer of Shewanella Oneidensis. Nat. Commun. 9, 3637–3713. doi:10.1038/s41467-018-05995-8
Li, F., Li, Y., Sun, L., Chen, X., An, X., Yin, C., et al. (2018). Modular Engineering Intracellular NADH Regeneration Boosts Extracellular Electron Transfer of Shewanella Oneidensis MR-1. ACS Synth. Biol. 7 (3), 885–895. doi:10.1021/acssynbio.7b00390
Li, W.-C., Li, X., Zhu, J.-Q., Qin, L., Li, B.-Z., and Yuan, Y.-J. (2018). Improving Xylose Utilization and Ethanol Production from Dry Dilute Acid Pretreated Corn stover by Two-Step and Fed-Batch Fermentation. Energy 157, 877–885. doi:10.1016/j.energy.2018.06.002
Li, F., An, X., Wu, D., Xu, J., Chen, Y., Li, W., et al. (2019). Engineering Microbial Consortia for High-Performance Cellulosic Hydrolyzates-Fed Microbial Fuel Cells. Front. Microbiol. 10, 409. doi:10.3389/fmicb.2019.00409
Light, S. H., Su, L., Rivera-Lugo, R., Cornejo, J. A., Louie, A., Iavarone, A. T., et al. (2018). A Flavin-Based Extracellular Electron Transfer Mechanism in Diverse Gram-Positive Bacteria. Nature 562 (7725), 140–144. doi:10.1038/s41586-018-0498-z
Lim, H. G., Lee, J. H., Noh, M. H., and Jung, G. Y. (2018). Rediscovering Acetate Metabolism: Its Potential Sources and Utilization for Biobased Transformation into Value-Added Chemicals. J. Agric. Food Chem. 66 (16), 3998–4006. doi:10.1021/acs.jafc.8b00458
Lin, H., Castro, N. M., Bennett, G. N., and San, K.-Y. (2006). Acetyl-CoA Synthetase Overexpression in Escherichia coli Demonstrates More Efficient Acetate Assimilation and Lower Acetate Accumulation: A Potential Tool in Metabolic Engineering. Appl. Microbiol. Biotechnol. 71 (6), 870–874. doi:10.1007/s00253-005-0230-4
Lin, T., Ding, W., Sun, L., Wang, L., Liu, C.-G., and Song, H. (2018). Engineered Shewanella Oneidensis-reduced Graphene Oxide Biohybrid with Enhanced Biosynthesis and Transport of Flavins Enabled a Highest Bioelectricity Output in Microbial Fuel Cells. Nano Energy 50, 639–648. doi:10.1016/j.nanoen.2018.05.072
Liu, T., Yu, Y.-Y., Deng, X.-P., Ng, C. K., Cao, B., Wang, J.-Y., et al. (2015). EnhancedShewanellabiofilm Promotes Bioelectricity Generation. Biotechnol. Bioeng. 112 (10), 2051–2059. doi:10.1002/bit.25624
Logan, B. E., and Rabaey, K. (2012). Conversion of Wastes into Bioelectricity and Chemicals by Using Microbial Electrochemical Technologies. Science 337 (6095), 686–690. doi:10.1126/science.1217412
Logan, B. E., Call, D., Cheng, S., Hamelers, H. V. M., Sleutels, T. H. J. A., Jeremiasse, A. W., et al. (2008). Microbial Electrolysis Cells for High Yield Hydrogen Gas Production from Organic Matter. Environ. Sci. Technol. 42 (23), 8630–8640. doi:10.1021/es801553z
Logan, B. E. (2009). Exoelectrogenic Bacteria that Power Microbial Fuel Cells. Nat. Rev. Microbiol. 7 (5), 375–381. doi:10.1038/nrmicro2113
Luo, H., Jenkins, P. E., and Ren, Z. (2011). Concurrent Desalination and Hydrogen Generation Using Microbial Electrolysis and Desalination Cells. Environ. Sci. Technol. 45 (1), 340–344. doi:10.1021/es1022202
Mohan, S. V., Butti, S. K., Amulya, K., Dahiya, S., and Modestra, J. A. (2016). Waste Biorefinery: A New Paradigm for a Sustainable Bioelectro Economy. Trends Biotechnol. 34 (11), 852–855. doi:10.1016/j.tibtech.2016.06.006
Morris, M. E., and Felmlee, M. A. (2008). Overview of the Proton-Coupled MCT (SLC16A) Family of Transporters: Characterization, Function and Role in the Transport of the Drug of Abuse γ-Hydroxybutyric Acid. Aaps J. 10 (2), 311. doi:10.1208/s12248-008-9035-6
Mukherjee, M., Hu, Y., Tan, C. H., Rice, S. A., and Cao, B. (2018). Engineering a Light-Responsive, Quorum Quenching Biofilm to Mitigate Biofouling on Water Purification Membranes. Sci. Adv. 4 (12), eaau1459. doi:10.1126/sciadv.aau1459
Mukherjee, M., Zaiden, N., Teng, A., Hu, Y., and Cao, B. (2020). Shewanella Biofilm Development and Engineering for Environmental and Bioenergy Applications. Curr. Opin. Chem. Biol. 59, 84–92. doi:10.1016/j.cbpa.2020.05.004
Ng, C. K., Xu, J., Cai, Z., Yang, L., Thompson, I. P., Huang, W. E., et al. (2020). Elevated Intracellular cyclic‐di‐GMP Level in Shewanella Oneidensis Increases Expression of C ‐type Cytochromes. Microb. Biotechnol. 13 (6), 1904–1916. doi:10.1111/1751-7915.13636
Novak, K., and Pflügl, S. (2018). Towards Biobased Industry: Acetate as a Promising Feedstock to Enhance the Potential of Microbial Cell Factories. FEMS Microbiol. Lett. 365 (20), 1–11. doi:10.1093/femsle/fny226
Pillot, G., Davidson, S., Auria, R., Combet-Blanc, Y., Godfroy, A., and Liebgott, P.-P. (2020). Production of Current by Syntrophy between Exoelectrogenic and Fermentative Hyperthermophilic Microorganisms in Heterotrophic Biofilm from a Deep-Sea Hydrothermal Chimney. Microb. Ecol. 79 (1), 38–49. doi:10.1007/s00248-019-01381-z
Pirbadian, S., Barchinger, S. E., Leung, K. M., Byun, H. S., Jangir, Y., Bouhenni, R. A., et al. (2014). Shewanella Oneidensis MR-1 Nanowires Are Outer Membrane and Periplasmic Extensions of the Extracellular Electron Transport Components. Proc. Natl. Acad. Sci. 111 (35), 12883–12888. doi:10.1073/pnas.1410551111
Rose, I. A., Grunberg-Manago, M., Korey, S. R., and Ochoa, S. (1954). Enzymatic Phosphorylation of Acetate. J. Biol. Chem. 211 (2), 737–756. doi:10.1016/s0021-9258(18)71161-7
Santoro, C., Arbizzani, C., Erable, B., and Ieropoulos, I. (2017). Microbial Fuel Cells: From Fundamentals to Applications. A Review. J. Power Sourc. 356, 225–244. doi:10.1016/j.jpowsour.2017.03.109
Shi, I. Y., Stansbury, J., and Kuzminov, A. (2005). A Defect in the Acetyl Coenzyme A↔Acetate Pathway Poisons Recombinational Repair-Deficient Mutants of Escherichia coli. J. Bacteriol. 187 (4), 1266–1275. doi:10.1128/jb.187.4.1266-1275.2005
Ueki, T., and Zhou, N.-Y. (2021). Key Enzymes for Anaerobic Lactate Metabolism in Geobacter Sulfurreducens. Appl. Environ. Microbiol. 87 (2), e01968–20. doi:10.1128/AEM.01968-20
Varrone, C., Van Nostrand, J. D., Liu, W., Zhou, B., Wang, Z., Liu, F., et al. (2014). Metagenomic-based Analysis of Biofilm Communities for Electrohydrogenesis: From Wastewater to Hydrogen. Int. J. Hydrogen Energ. 39 (9), 4222–4233. doi:10.1016/j.ijhydene.2014.01.001
Vikrant, K., Giri, B. S., Raza, N., Roy, K., Kim, K.-H., Rai, B. N., et al. (2018). Recent Advancements in Bioremediation of Dye: Current Status and Challenges. Bioresour. Technol. 253, 355–367. doi:10.1016/j.biortech.2018.01.029
Wang, H., Luo, H., Fallgren, P. H., Jin, S., and Ren, Z. J. (2015). Bioelectrochemical System Platform for Sustainable Environmental Remediation and Energy Generation. Biotechnol. Adv. 33 (3-4), 317–334. doi:10.1016/j.biotechadv.2015.04.003
Xiao, Y., Zhang, E., Zhang, J., Dai, Y., Yang, Z., Christensen, H. E. M., et al. (2017). Extracellular Polymeric Substances Are Transient media for Microbial Extracellular Electron Transfer. Sci. Adv. 3 (7), e1700623–8. doi:10.1126/sciadv.1700623
Xie, X., Ye, M., Hsu, P.-C., Liu, N., Criddle, C. S., and Cui, Y. (2013). Microbial Battery for Efficient Energy Recovery. Proc. Natl. Acad. Sci. 110 (40), 15925–15930. doi:10.1073/pnas.1307327110
Yan, D., Yang, X., and Yuan, W. (2015). Electricity and H2 Generation from Hemicellulose by Sequential Fermentation and Microbial Fuel/electrolysis Cell. J. Power Sourc. 289, 26–33. doi:10.1016/j.jpowsour.2015.04.164
Yang, Y., Ding, Y., Hu, Y., Cao, B., Rice, S. A., Kjelleberg, S., et al. (2015). Enhancing Bidirectional Electron Transfer of Shewanella Oneidensis by a Synthetic Flavin Pathway. ACS Synth. Biol. 4 (7), 815–823. doi:10.1021/sb500331x
Yong, Y.-C., Dong, X.-C., Chan-Park, M. B., Song, H., and Chen, P. (2012). Macroporous and Monolithic Anode Based on Polyaniline Hybridized Three-Dimensional Graphene for High-Performance Microbial Fuel Cells. Acs Nano 6 (3), 2394–2400. doi:10.1021/nn204656d
Yong, Y.-C., Yu, Y.-Y., Zhang, X., and Song, H. (2014). Highly Active Bidirectional Electron Transfer by a Self-Assembled Electroactive Reduced-Graphene-Oxide-Hybridized Biofilm. Angew. Chem. Int. Ed. 53 (17), 4480–4483. doi:10.1002/anie.201400463
You, J., Walter, X. A., Greenman, J., Melhuish, C., and Ieropoulos, I. (2015). Stability and Reliability of Anodic Biofilms under Different Feedstock Conditions: Towards Microbial Fuel Cell Sensors. Sens. Bio-Sens. Res. 6, 43–50. doi:10.1016/j.sbsr.2015.11.007
Yu, Y.-Y., Chen, H.-l., Yong, Y.-C., Kim, D.-H., and Song, H. (2011). Conductive Artificial Biofilm Dramatically Enhances Bioelectricity Production in Shewanella-Inoculated Microbial Fuel Cells. Chem. Commun. 47 (48), 12825–12827. doi:10.1039/c1cc15874k
Yu, Y.-Y., Guo, C. X., Yong, Y.-C., Li, C. M., and Song, H. (2015). Nitrogen Doped Carbon Nanoparticles Enhanced Extracellular Electron Transfer for High-Performance Microbial Fuel Cells Anode. Chemosphere 140, 26–33. doi:10.1016/j.chemosphere.2014.09.070
Zhang, Y., and Angelidaki, I. (2014). Microbial Electrolysis Cells Turning to Be Versatile Technology: Recent Advances and Future Challenges. Water Res. 56, 11–25. doi:10.1016/j.watres.2014.02.031
Zhang, Z., Li, F., Cao, Y., Tian, Y., Li, J., Zong, Y., et al. (2019). Electricity-driven 7α-Hydroxylation of a Steroid Catalyzed by a Cytochrome P450 Monooxygenase in Engineered Yeast. Catal. Sci. Technol. 9 (18), 4877–4887. doi:10.1039/c9cy01288e
Zhao, J., Li, F., Cao, Y., Zhang, X., Chen, T., Song, H., et al. (2020). Microbial Extracellular Electron Transfer and Strategies for Engineering Electroactive Microorganisms. Biotechnol. Adv. [Epub ahead of print], 107682. doi:10.1016/j.biotechadv.2020.107682
Zhen, G., Lu, X., Kumar, G., Bakonyi, P., Xu, K., and Zhao, Y. (2017). Microbial Electrolysis Cell Platform for Simultaneous Waste Biorefinery and Clean Electrofuels Generation: Current Situation, Challenges and Future Perspectives. Prog. Energ. Combust. Sci. 63, 119–145. doi:10.1016/j.pecs.2017.07.003
Keywords: acetate, microbial fuel cell, synthetic biology, Shewanella oneidensis, coulombic efficiency
Citation: Zhang J, Chen Z, Liu C, Li J, An X, Wu D, Sun X, Zhang B, Fu L, Li F and Song H (2021) Construction of an Acetate Metabolic Pathway to Enhance Electron Generation of Engineered Shewanella oneidensis. Front. Bioeng. Biotechnol. 9:757953. doi: 10.3389/fbioe.2021.757953
Received: 13 August 2021; Accepted: 08 October 2021;
Published: 19 November 2021.
Edited by:
Yuan Lu, Tsinghua University, ChinaReviewed by:
Manuel Porcar, University of Valencia, SpainCopyright © 2021 Zhang, Chen, Liu, Li, An, Wu, Sun, Zhang, Fu, Li and Song. This is an open-access article distributed under the terms of the Creative Commons Attribution License (CC BY). The use, distribution or reproduction in other forums is permitted, provided the original author(s) and the copyright owner(s) are credited and that the original publication in this journal is cited, in accordance with accepted academic practice. No use, distribution or reproduction is permitted which does not comply with these terms.
*Correspondence: Feng Li, bWVzc2lsaWZlbmdAMTYzLmNvbQ==; Hao Song, aHNvbmdAdGp1LmVkdS5jbg==
†These authors have contributed equally to this work
Disclaimer: All claims expressed in this article are solely those of the authors and do not necessarily represent those of their affiliated organizations, or those of the publisher, the editors and the reviewers. Any product that may be evaluated in this article or claim that may be made by its manufacturer is not guaranteed or endorsed by the publisher.
Research integrity at Frontiers
Learn more about the work of our research integrity team to safeguard the quality of each article we publish.