- 1Department of Cell Biology–Inspired Tissue Engineering, MERLN Institute for Technology-Inspired Regenerative Medicine, Maastricht University, Maastricht, Netherlands
- 2Department of Food Innovation and Health at the Centre of Healthy Eating and Food Innovation, Maastricht University, Maastricht, Netherlands
In regenerative medicine and tissue engineering, many materials are developed to mimic the extracellular matrix (ECM). However, these ECM-mimicking materials do not yet completely recapitulate the diversity and complexity of biological tissue-specific ECM. In this review, an alternative strategy is proposed to generate ECM, namely synthesizing a material that functions as a drug delivery system, releasing molecules that target cellular metabolic pathways and thereby stimulate the local cells to create their own ECM. This is based on the fact that ECM synthesis, modification, composition, signaling, stiffness, and degradation are modulated by cellular metabolism. Metabolism can be targeted at different levels, ranging from modulating the availability of substrates or co-factors to regulating the activity of essential transcription factors. Depending on the drug of interest, its characteristics, mechanism of action, cellular target, and application, a different drug delivery system should be designed. Metabolic drugs modulating the ECM require cellular uptake for their function, therefore reversible linkers are recommended. Preferably the metabolic modulators are only released when needed, which will be upon a specific metabolic state, a change in ECM stiffness, or ECM remodeling. Therefore, reversible linkers that respond to an environmental stimulus could be incorporated. All in all, a novel strategy is suggested to develop a tissue-specific ECM by generating a synthetic material that releases metabolic molecules modulating the ECM. Various ways to modulate the ECM properties via the metabolism are reviewed and guidelines for the development of these materials are provided.
Introduction
In regenerative medicine and tissue engineering, a variety of approaches have been taken to engineer the extracellular matrix (ECM) using synthetic or natural building blocks. The aim of these efforts is to generate a matrix that closely mimics the tissue-specific matrix properties including its stiffness, strength, dynamicity, and cell–matrix interactions to enhance cell behavior specific to the tissue of interest (Geckil et al., 2010; Hong et al., 2011; Burdick and Murphy, 2012; Lam et al., 2014).
Despite significant advances in engineering the ECM, synthetic materials have yet to fully capture its diversity and complexity (Kyburz and Anseth, 2015). Some scientists have turned to using biological tissue as a source of ECM proteins. For example, following decellularization, these proteins can be used as a scaffold or as a bioink for 3D printing (Uriel et al., 2009; Dzobo et al., 2019; Fernández-Pérez and Ahearne, 2019). Other approaches have embraced a combined synthetic and natural approach, for example by coating synthetic materials with biological motifs to enhance cell–matrix interactions. The best known example of this approach is the addition of an RGD peptide to a scaffold, which cells can use for integrin-mediated adhesion and subsequent signaling (Ingavle et al., 2014; Xing et al., 2020). However, while these approaches may capture some of the important features of the ECM, it remains challenging to produce tissue-specific ECM with the correct biological (e.g., expression of specific ECM proteins and post-translational modifications) and physical (e.g., stiffness, viscoelasticity) properties. Many approaches are also hindered by additional disadvantages such as the lack of reproducibility and tunability.
Here, an alternative strategy for generating tissue-specific ECM is reviewed. In this strategy, instead of directly engineering an ECM, synthetic materials can be used to modulate cellular metabolism and thus stimulate cells to synthesize and remodel their own ECM (Ma et al., 2019). A synthetic material is chosen for this strategy because it offers a blank-slate, whereas a naturally-derived ECM can possess tissue-specific cues, which is seen in examples where it induces tissue-specific differentiation (da Mata Martins et al., 2020; Parmaksiz et al., 2020). From a synthetic material, the ECM stiffness can be tuned and bioactive molecules can easily be incorporated (Kyburz and Anseth, 2015). The synthetic material therefore functions as a drug delivery system to target metabolic pathways in the cells (Tiwari et al., 2012; Tibbitt et al., 2016; Li et al., 2019), which also offers the benefit of local drug delivery to enhance the therapeutic effect (Hwang et al., 2020). These metabolic pathways leading to changes in ECM production and remodeling are consistently expressed in all different cell types and can be modulated on demand. In this mini-review, the mechanisms by which cellular metabolism affects the ECM, the various ways to modulate the ECM by targeting the metabolism, and guidelines for the development of materials that employ this approach are reviewed.
Cellular Metabolism Affects the ECM Synthesis, Composition, and Physical Properties
Every cell needs energy to perform its function. They are therefore equipped with various metabolic pathways to generate energy from substrates ranging from glucose to lipids. These metabolic pathways are regulated based on the available substrates and oxygen levels. Some pathways generate more energy (e.g., oxidative phosphorylation, the aerobic oxygen-dependent pathway) than others (glycolysis, the anaerobic oxygen-independent pathway).
Every tissue has a specific ECM in terms of its structure and composition (Frantz et al., 2010). Because ECM synthesis requires a lot of energy, its synthesis and the composition of the resulting matrix can be affected by the metabolism. Furthermore, modulating the cellular metabolism can change the physical properties of the ECM, such as its stiffness and degradation. All of these naturally occurring metabolic pathways that affect ECM properties can be targets of a synthetic materials-based approach to generate tissue-specific ECM (Romani et al., 2021).
Different ECM Components are Expressed due to Changes in Metabolism
Different metabolic targets exist to modulate ECM expression. In general, when there is an excess of nutrients, AMP-activated protein kinase (AMPK) is decreased and mammalian target of rapamycin complex 1 (mTORC1) is increased, which subsequently increases fibronectin assembly and integrin activation (Table 1). AMPK has also been shown to regulate hyaluronan synthesis by phosphorylating and inhibiting hyaluronan synthase 2 (Xing et al., 2020). In addition, another in fibroblasts and epithelial cells from the lung, kidney, liver, and skin, it has been shown that the Sterol regulatory element-binding proteins (SREBP)/mevalonate metabolism regulates the Yes-associated protein 1 (YAP1)/transcriptional coactivator with PDZ-binding motif (TAZ) signaling (Sorrentino et al., 2014; Noguchi et al., 2018). This activation of YAP/TAZ signaling induces the synthesis of (profibrotic) ECM proteins like collagen I (Table 1). Conversely, when nutrients are in short supply, fibronectin assembly is decreased and integrin internalization and ECM degradation are subsequently increased (Dornier and Norman, 2017).
This relationship between nutrients and ECM also exists in differentiating stem cells. For example, during adipocyte differentiation, cellular metabolism changes due to the uptake and storage of fatty acids (Mariman and Wang, 2010). This change affects the ECM composition through peroxisome proliferator-activated receptor gamma (PPARγ), a key transcription factor in adipocyte differentiation, which upregulates the synthesis ECM components collagen VI and thrombospondin 1 (Okuno et al., 2002; Table 1). All in all, the energetic substrate leads to metabolic changes that can affect the expression of ECM proteins like fibronectin or collagen.
Hypoxia Induces a Stiffer Matrix
Another means by which the ECM can be modulated is through oxygen availability, which can also affect the induction of metabolic pathways, leading to mechanical modulation of the ECM. In hypoxia, which is a low level of oxygen, more anaerobic metabolic pathways are upregulated, and the expression of collagen I is reduced while the expression of collagen IV is increased (Tajima et al., 2001; McKay et al., 2017). However, it is not just the ECM synthesis and composition that are affected by metabolism; the physical properties of the ECM are also influenced. When the metabolism of chondrocytes is affected by hypoxia and there is a subsequent diminished glucose oxidation, the collagen synthesis is also reduced (Stegen et al., 2019). As the cells switch from a metabolism based on glucose and fatty acids to glutamine, the enhanced α-ketoglutarate formation from glutamine leads to increased proline and lysine hydroxylation of collagen (Stegen et al., 2019; Table 2). This hydroxylation of collagen increases ECM stiffness and prevents matrix degradation. As another example, in hepatic stellate spheroids, the addition of the glycolytic metabolite phosphoenolpyruvate has been shown to increase α-smooth muscle actin, thereby stiffening the matrix (Fujisawa et al., 2020; Table 2).
Metabolism can also affect the degradability of the ECM through, for example, matrix metalloproteinases (MMPs). Briefly, MMPs can be classified in specific groups, one of which are the collagenases. These MMPs cleave collagen at specific sites, except when the collagen is hydroxylated at a nearby proline or lysine residue (Taddese et al., 2010). An example of how metabolism affects the degradability of the ECM is the activation of PPARγ, which increases the expression of MMP-1 (François et al., 2004; Table 2). Similar relationships are seen in medicine, where obesity leads to adipocyte hypertrophy and overgrowth, leading to hypoxia and decreased energy production via the tricarboxylic acid cycle. This inhibits proline hydroxylation, which increases ECM degradation by MMPs and leads to ECM instability (Mariman and Wang, 2010). Finally, in breast cancer cells, it has been shown that pyruvate metabolism induces collagen hydroxylation by activating prolyl-4-hydroxylase, which decreases MMP8 degradation and subsequently decreases metastatic growth of the cancer cells (Elia et al., 2019; Table 2). Overall, due to low oxygen, alternative metabolic pathways are induced that affect ECM crosslinking or release enzymes that are involved in ECM remodeling affecting its mechanical properties.
Other Interactions Between Cellular Metabolism and ECM Properties
It is also interesting to note that in addition to the cellular metabolism determining ECM properties, it is also the case that the ECM conversely affects cellular metabolism. However, this is outside of the scope of this mini-review and has been extensively described elsewhere (Mah et al., 2018; Fernie et al., 2020). For example, when cells detach from the ECM that leads to decreased glucose uptake, glycolysis and oxidative phosphorylation (Mason et al., 2017). In addition, a low level of hyaluronan leads to diminished internalization of the glucose transporter GLUT1 that subsequently decreases all glucose-related cellular metabolism (Sullivan et al., 2018). Decreased hyaluronan activates receptor tyrosine kinase (RTK), which subsequently induces ZFP36. ZFP36 leads to thioredoxin interacting protein (TXNIP) degradation and decreases GLUT1 internalization (Katsu-Jiménez et al., 2019). ECM detachment of ECM expression can both affect substrate uptake or increase metabolism.
In summary, cellular metabolism can affect the ECM synthesis, modification, composition, signaling, stiffness, and degradation. In addition, the ECM can also influence the cellular metabolism. These studies indicate interesting metabolic targets that can be used to modulate the ECM. Next, we will describe how (bio)materials scientists and tissue engineers can harness this information.
A Toolbox for (Bio)material Scientists to Influence Metabolism and Thereby Affect ECM
Methods to modulation the ECM by influencing cellular metabolism can be incorporated in the design of materials or scaffolds for tissue engineering. Material scientists have previously developed various effective tools for drug delivery that can be applied (Li et al., 2019). Depending on the drug of interest, its characteristics, mechanism of action, cellular target and application, different materials, and strategies to modify the material can be chosen. ECM-like hybrid materials are very suitable for the addition of a drug-delivery element and application for regenerative medicine purposes (Hinderer et al., 2016; Setayeshmehr et al., 2019; Zhu et al., 2019). All in all, synthetic materials could be combined with a drug delivery element depending on the drug of choice.
Different metabolic drugs exist to target different metabolic pathways. A metabolic pathway can be targeted at different levels, for example an essential co-factor can be scavenged or an inhibitory analog of an essential substrate with higher affinity can be provided (Park et al., 2008; Zhdanov et al., 2015; Zhang et al., 2016). Furthermore, a transcription factor can be inhibited directly by keeping it in its inactive form (Gu et al., 2016). We will first discuss the selection of a drug, which will be followed by an overview of the delivery strategies that (bio)materials scientists can employ.
Drugs can Target Metabolism by Affecting Essential Co-Factors or Metabolic Transcription Factors
There are many drugs that can target metabolism, for example, by targeting a co-factor for a metabolic pathway or directly targeting a transcription factor. Examples of drugs that target an essential co-factor for a metabolic pathway are deferoxamine (DFO) and dimethyloxalylglycine (DMOG), which both affect the metabolic transcription factor HIF1α. DFO is an iron chelator, while DMOG is a synthetic analogue of α-ketoglutarate. Both iron and α-ketoglutarate are essential substrates for prolyl-4-hydroxylase, which hydroxylates HIF1α in normoxia to target HIF1α for proteosomal degradation (Iommarini et al., 2017; Donneys et al., 2019). However, during hypoxia or when adding DFO or the competitive inhibitor DMOG, prolyl-hydroxylases do not hydroxylate HIF1α. This leads to HIF1α-mediated signaling, but also decreases proline and lysine hydroxylation of collagen, which decreases matrix stiffness and leads to a higher sensitivity of the matrix for degradation (Rappu et al., 2019; Stegen et al., 2019).
An example of a metabolic drug that directly targets a metabolic transcription factor is the inhibitor AS1842856 that inhibits FOXO1 by binding to its active non-phosphorylated form. FOXO1 mediates the activation of Glucose 6-phosphatase and inhibits glucokinase. In hepatocytes, insulin represses glucose production and enhances lipogenesis by inhibiting FOXO1 activation (Langlet et al., 2017). Depending on the metabolic pathway that needs to be activated, a different metabolic drug can be chosen.
Criteria for Metabolic Drugs are Mechanism of Action, No Therapeutic Toxicity and Potential for Clinical Use
In this strategy, it is important that the characteristics of the drug of interest be taken into account. There are numerous considerations that should be given to selecting a drug for the purpose of modulating cellular metabolism and thereafter the ECM. Firstly, it is essential that the drug does not have any toxic effects on the cells at its effective concentration and exposure time (Suzman et al., 2019; Hoy, 2020). Preferably, the drug is FDA/EMA-approved like DFO, which has been approved for iron overload (Kontoghiorghes and Kontoghiorghe, 2016; Sun et al., 2020; Xu et al., 2020; Takpradit et al., 2021). After selecting the drug of interest based on characteristics, mechanism of action and cellular target, the next step will be to include it in the right material and apply the right release strategy.
The Mechanism of Action of the Metabolic Drug Determines Which Material and Coupling Strategy will be Chosen.
The physical properties of the tissue of interest is important for the choice of the material, while the mechanism of action of the metabolism-modulating drug defines the coupling strategy. For example, depending on the stiffness of the ECM of the target tissue, a different material could be selected, ranging from solid materials to soft hydrogels. PEG, PVA, and PHEMA are frequently used for engineering the ECM, and they can be co-polymerized with different polymers to tune the physical properties (Unal and West, 2020), which can also be an advantage in 3D printing applications (Da Silva et al., 2020). For an optimal mechanical strength, dynamic viscoelastic hydrogels are of particular interest because of the tunability of their stiffness and their resemblance to the ECM (Hafeez et al., 2018; Table 3).
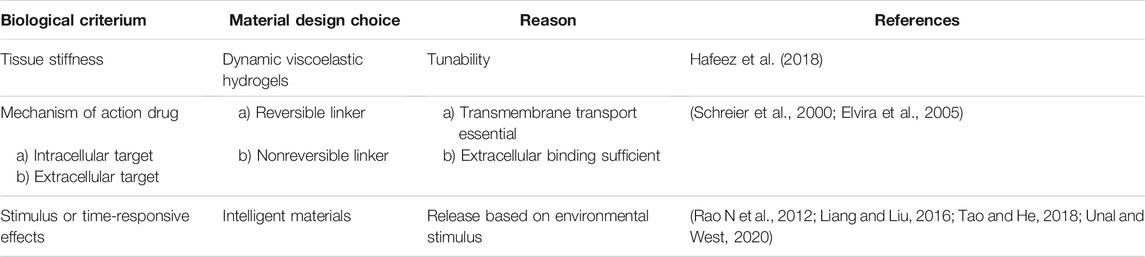
TABLE 3. Design criteria for synthetic materials that affect the extracellular matrix via cellular metabolism.
The drug coupling or delivery method needs to be chosen based on the drug of interest and its cellular target (Table 3). For example, if the metabolic drug needs to be released to be functional or taken up by the cell to induce its biological effect, it is essential to include a reversible linker into the material design. Biodegradable linkers can be used, including: carbonates, anhydrides, esters, urethanes, amides, or orthoesters (Elvira et al., 2005). If the drug of interest is of amphiphilic or hydrophobic nature, it can self-associate in surfactant materials (Schreier et al., 2000). In order to control drug release, linkers that depend on the environmental stimulus could be applied or designed to modulate the timing of drug release (Table 3).
For some metabolic modulators that regulate the ECM, a more sustained release is required. Many strategies are available to induce a sustained release, ranging from coupling, host-guest chemistry to encapsulating in cellulose capsules (Bakker et al., 2018a; Bakker et al., 2018b; Sun et al., 2019). Furthermore, many different intelligent biomaterials have been developed that respond to the environment, including cues such as pH, reactive oxygen species (ROS), MMPs, or even mechanotransduction. Depending on the degradation rate by MMPs, a different polymer can be chosen (Unal and West, 2020). An example of how environmental cues can be used is in the setting of an engineered tissue that lacks vasculature. This will lead to hypoxia and enhanced glycolysis and lactic acidosis in the surrounding tissue that decreases the pH. This pH decrease can be used to prevent associated changes in the surrounding ECM by releasing a drug that influences the metabolism and subsequent ECM remodeling. A hydrazine linker is a commonly used pH-inducible release linker, since it is cleaved in an acidic environment (Rao N et al., 2012). Similarly, the decreased oxidative phosphorylation present in hypoxia also leads to incomplete mitochondrial respiration and the formation of ROS. These ROS could be another stimulus that releases metabolic drugs influencing ECM in response to metabolic changes. ROS can reduce disulfide bonds inducing the release of the coupled drug molecule. Next to the disulfide bonds, there exist many different ROS or redox-responsive linkers (Liang and Liu, 2016; Tao and He, 2018).
In summary, the tissue of interest defines the material choice. Depending on the local ECM stiffness and presence of local cells, more or less metabolic modulator will be released, making synthesis and stiffness of the ECM tissue-specific. In addition, depending on the characteristics of the metabolic drug modulating ECM and the local ECM, different drug delivery strategies should be used. Because most metabolic drugs that affect the ECM require cellular uptake for their function, reversible linkers should be applied. Intelligent materials that respond to the pH, ROS, MMPs, or mechanical signals from the environment enhance the biological application of these materials.
Discussion
To promote both the formation of a naturally complex ECM, while preventing the variability in production, studies have shown the potential of a synthetic material that functions as a drug delivery system to release metabolic modulators that influence the synthesis and modification of ECM in resident cells (Figure 1).
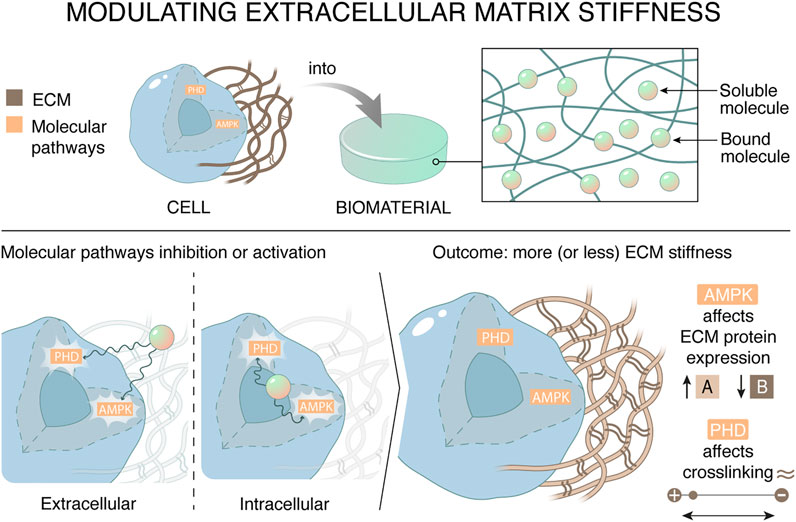
FIGURE 1. Materials can affect the stiffness of the extracellular matrix by regulating cellular metabolism. The synthetic drug delivery system targets metabolic mediators AMP-activated protein kinase (AMPK) and prolyl hydroxylases in tissue resident cells, which in their turn decreases ECM stiffness. There are two ways to regulate the outcome of ECM stiffness, namely by affecting ECM expression or by affecting ECM crosslinking. This can be achieved by targeting metabolic pathways by scavenging essential cofactors extracellularly or directly inhibiting metabolic targets.
AMPK, PPARγ, SREBP, or prolyl hydroxylases are metabolic targets known to influence the composition and/or stiffness of the ECM. Different metabolic drugs exist that target metabolic pathways and thereby influence the ECM synthesis and/or modification. The metabolic drugs can target co-factors or substrates or interact with a metabolic transcription factor. The characteristics, mechanism of action, cellular target and application of the drug of interest inform the choices for the synthetic materials and the drug-coupling strategy. If the metabolic molecule needs to bind the intracellular enzymes or transcription factors for performing its function, a reversible linker could be used. These reversible linkers could respond to metabolic (pH, ROS) or ECM-related (MMP-mediated degradation or mechanical forces) microenvironmental stimuli.
In conclusion, we have described the potential of a synthetic material with a drug-delivery element to modulate cellular metabolism and thereby regulate the ECM. This strategy confers reproducibility in production while capturing the complexity of the tissue-specific ECM.
In the future, multidrug spatiotemporal controlled hydrogels could contribute to induce a tunable time or concentration-specific drug release. This is especially important because biological systems are complex with multiple (negative) feedback loops. During physiological metabolism, a metabolic pathway is activated, which leads to a new cue influencing the next pathway. This could be the inspiration for the next step in material engineering. Multidrug-responsive hydrogels could be designed that contain multiple drug delivery elements that are also capable of responding to a second cellular mediators, also called intelligent multicommunicating materials (Brown and Anseth, 2017; Leijten et al., 2017). These multidrug spatiotemporal controlled hydrogels could be the next generation of cell–material communication.
Author Contributions
All authors have read and approved the final article. MS and VL wrote the article with input from CvB.
Funding
This research has received funding from the Gravitation Program “Materials‐Driven Regeneration” by the Netherlands Organization for Scientific Research. This research has received funding from the European Research Council (ERC) under the European Union’s Horizon 2020 research and innovation program (grant agreement No. 694801).
Conflict of Interest
The authors declare that the research was conducted in the absence of any commercial or financial relationships that could be construed as a potential conflict of interest.
Publisher’s Note
All claims expressed in this article are solely those of the authors and do not necessarily represent those of their affiliated organizations, or those of the publisher, the editors and the reviewers. Any product that may be evaluated in this article, or claim that may be made by its manufacturer, is not guaranteed or endorsed by the publisher.
References
Bakker, M. H., Grillaud, M., Wu, D. J., Fransen, P. K. H., de Hingh, I. H., and Dankers, P. Y. W. (2018). Cholesterol modification of an anticancer drug for efficient incorporation into a supramolecular hydrogel system. Macromol Rapid Commun. 39, e1800007. doi:10.1002/marc.201800007
Bakker, M. H., van Rooij, E., and Dankers, P. Y. W. (2018). Controlled release of RNAi molecules by tunable supramolecular hydrogel carriers. Chem. Asian J. 13, 3501–3508. doi:10.1002/asia.201800582
Brown, T. E., and Anseth, K. S. (2017). Spatiotemporal hydrogel biomaterials for regenerative medicine. Chem. Soc. Rev. 46, 6532–6552. doi:10.1039/c7cs00445a
Burdick, J. A., and Murphy, W. L. (2012). Moving from static to dynamic complexity in hydrogel design. Nat. Commun. 3, 1269. doi:10.1038/ncomms2271
da Mata Martins, T. M., da Silva Cunha, P., Rodrigues, M. A., de Carvalho, J. L., de Souza, J. E., de Carvalho Oliveira, J. A., et al. (2020). Epithelial basement membrane of human decellularized cornea as a suitable substrate for differentiation of embryonic stem cells into corneal epithelial-like cells. Mater. Sci. Eng. C 116, 111215. doi:10.1016/j.msec.2020.111215
Da Silva, K., Kumar, P., Choonara, Y. E., du Toit, L. C., and Pillay, V. (2020). Three‐dimensional printing of extracellular matrix ( ECM )‐mimicking scaffolds: A critical review of the current ECM materials. J. Biomed. Mater. Res. 108, 2324–2350. doi:10.1002/jbm.a.36981
Donneys, A., Yang, Q., Forrest, M. L., Nelson, N. S., Zhang, T., Ettinger, R., et al. (2019). Implantable hyaluronic acid-deferoxamine conjugate prevents nonunions through stimulation of neovascularization. NPJ Regen. Med. 4, 11. doi:10.1038/s41536-019-0072-9
Dornier, E., and Norman, J. C. (2017). Tensin links energy metabolism to extracellular matrix assembly. J. Cel Biol 216, 867–869. doi:10.1083/jcb.201702025
Dzobo, K., Motaung, K. S. C. M., and Adesida, A. (2019). Recent trends in decellularized extracellular matrix bioinks for 3D printing: an updated review. Int. J. Mol. Sci. 20. doi:10.3390/ijms20184628
Elia, I., Rossi, M., Stegen, S., Broekaert, D., Doglioni, G., van Gorsel, M., et al. (2019). Breast cancer cells rely on environmental pyruvate to shape the metastatic niche. Nature 568, 117–121. doi:10.1038/s41586-019-0977-x
Elvira, C., Gallardo, A., Roman, J., and Cifuentes, A. (2005). Covalent polymer-drug conjugates. Molecules 10, 114–125. doi:10.3390/10010114
Fernández-Pérez, J., and Ahearne, M. (2019). The impact of decellularization methods on extracellular matrix derived hydrogels. Sci. Rep. 9, 14933. doi:10.1038/s41598-019-49575-2
Fernie, A. R., Zhang, Y., and Sampathkumar, A. (2020). Cytoskeleton architecture regulates glycolysis coupling cellular metabolism to mechanical cues. Trends Biochem. Sci. 45, 637–638. doi:10.1016/j.tibs.2020.04.003
Frantz, C., Stewart, K. M., and Weaver, V. M. (2010). The extracellular matrix at a glance. J. Cel Sci 123, 4195–4200. doi:10.1242/jcs.023820
François, M., Richette, P., Tsagris, L., Raymondjean, M., Fulchignoni-Lataud, M.-C., Forest, C., et al. (2004). Peroxisome Proliferator-activated Receptor-γ Down-regulates Chondrocyte Matrix Metalloproteinase-1 via a Novel Composite Element. J. Biol. Chem. 279, 28411–28418. doi:10.1074/jbc.m312708200
Fujisawa, K., Takami, T., Sasai, N., Matsumoto, T., Yamamoto, N., and Sakaida, I. (2020). Metabolic alterations in spheroid-cultured hepatic stellate cells. Int. J. Mol. Sci. 21. doi:10.3390/ijms21103451
Geckil, H., Xu, F., Zhang, X., Moon, S., and Demirci, U. (2010). Engineering hydrogels as extracellular matrix mimics. Nanomedicine 5, 469–484. doi:10.2217/nnm.10.12
Gu, W., Yang, Y., Zhang, C., Zhang, Y., Chen, L., Shen, J., et al. (2016). Caffeic acid attenuates the angiogenic function of hepatocellular carcinoma cells via reduction in JNK-1-mediated HIF-1α stabilization in hypoxia. RSC Adv. 6, 82774–82782. doi:10.1039/c6ra07703j
Hafeez, S., Ooi, H. W., Morgan, F. L. C., Mota, C., Dettin, M., Van Blitterswijk, C., et al. (2018) Viscoelastic oxidized alginates with reversible imine type crosslinks: self-healing, injectable, and bioprintable hydrogels. Gels 4. doi:10.3390/gels4040085
Hinderer, S., Layland, S. L., and Schenke-Layland, K. (2016). ECM and ECM-like materials - Biomaterials for applications in regenerative medicine and cancer therapy. Adv. Drug Deliv. Rev. 97, 260–269. doi:10.1016/j.addr.2015.11.019
Hong, Y., Huber, A., Takanari, K., Amoroso, N. J., Hashizume, R., Badylak, S. F., et al. (2011). Mechanical properties and in vivo behavior of a biodegradable synthetic polymer microfiber-extracellular matrix hydrogel biohybrid scaffold. Biomaterials 32, 3387–3394. doi:10.1016/j.biomaterials.2011.01.025
Hwang, J., Sullivan, M. O., and Kiick, K. L. (2020). Targeted drug delivery via the use of ECM-mimetic materials. Front. Bioeng. Biotechnol. 8, 69. doi:10.3389/fbioe.2020.00069
Ingavle, G. C., Gehrke, S. H., and Detamore, M. S. (2014). The bioactivity of agarose-PEGDA interpenetrating network hydrogels with covalently immobilized RGD peptides and physically entrapped aggrecan. Biomaterials 35, 3558–3570. doi:10.1016/j.biomaterials.2014.01.002
Iommarini, L., Porcelli, A. M., Gasparre, G., and Kurelac, I. (2017). Non-Canonical Mechanisms Regulating Hypoxia-Inducible Factor 1 Alpha in Cancer. Front. Oncol. 7, 286. doi:10.3389/fonc.2017.00286
Katsu-Jiménez, Y., Vázquez-Calvo, C., Maffezzini, C., Halldin, M., Peng, X., Freyer, C., et al. (2019). Absence of TXNIP in humans leads to lactic acidosis and low serum methionine linked to deficient respiration on pyruvate. Diabetes 68, 709–723. doi:10.2337/db18-0557
Kontoghiorghes, G. J., and Kontoghiorghe, C. N. (2016). Efficacy and safety of iron-chelation therapy with deferoxamine, deferiprone, and deferasirox for the treatment of iron-loaded patients with non-transfusion-dependent thalassemia syndromes. Dddt 10, 465–481. doi:10.2147/dddt.s79458
Kyburz, K. A., and Anseth, K. S. (2015). Synthetic mimics of the extracellular matrix: how simple is complex enough. Ann. Biomed. Eng. 43, 489–500. doi:10.1007/s10439-015-1297-4
Lam, J., Truong, N. F., and Segura, T. (2014). Design of cell-matrix interactions in hyaluronic acid hydrogel scaffolds. Acta Biomater. 10, 1571–1580. doi:10.1016/j.actbio.2013.07.025
Langlet, F., Haeusler, R. A., Lindén, D., Ericson, E., Norris, T., Johansson, A., et al. (2017). Selective inhibition of FOXO1 activator/repressor balance modulates hepatic glucose handling. Cell 171, 824–835. doi:10.1016/j.cell.2017.09.045
Leijten, J., Seo, J., Yue, K., Trujillo-de Santiago, G., Tamayol, A., Ruiz-Esparza, G. U., et al. (2017). Spatially and temporally controlled hydrogels for tissue engineering. Mater. Sci. Eng. R: Rep. 119, 1–35. doi:10.1016/j.mser.2017.07.001
Li, C., Wang, J., Wang, Y., Gao, H., Wei, G., Huang, Y., et al. (2019). Recent progress in drug delivery. Acta Pharmaceutica Sinica B 9, 1145–1162. doi:10.1016/j.apsb.2019.08.003
Liang, J., and Liu, B. (2016). ROS‐responsive drug delivery systems. Bioeng. Translational Med. 1, 239–251. doi:10.1002/btm2.10014
Ma, C., Kuzma, M. L., Bai, X., and Yang, J. (2019). Biomaterial‐Based Metabolic Regulation in Regenerative Engineering. Adv. Sci. 6, 1900819. doi:10.1002/advs.201900819
Mah, E. J., Lefebvre, A. E. Y. T., McGahey, G. E., Yee, A. F., and Digman, M. A. (2018). Collagen density modulates triple-negative breast cancer cell metabolism through adhesion-mediated contractility. Sci. Rep. 8, 17094. doi:10.1038/s41598-018-35381-9
Mariman, E. C. M., and Wang, P. (2010). Adipocyte extracellular matrix composition, dynamics and role in obesity. Cell. Mol. Life Sci. 67, 1277–1292. doi:10.1007/s00018-010-0263-4
Mason, J. A., Hagel, K. R., Hawk, M. A., and Schafer, Z. T. (2017). Metabolism during ECM detachment: Achilles heel of cancer cells. Trends Cancer 3, 475–481. doi:10.1016/j.trecan.2017.04.009
McKay, T. B., Hjortdal, J., Priyadarsini, S., and Karamichos, D. (2017). Acute hypoxia influences collagen and matrix metalloproteinase expression by human keratoconus cells in vitro. PLoS One 12, e0176017. doi:10.1371/journal.pone.0176017
Noguchi, S., Saito, A., and Nagase, T. (2018). YAP/TAZ Signaling as a molecular link between fibrosis and cancer. Int. J. Mol. Sci., 19. doi:10.3390/ijms19113674
Okuno, M., Arimoto, E., Nishizuka, M., Nishihara, T., and Imagawa, M. (2002). Isolation of up- or down-regulated genes in PPARγ-expressing NIH-3T3 cells during differentiation into adipocytes. FEBS Lett. 519, 108–112. doi:10.1016/s0014-5793(02)02720-5
Park, S.-S., Bae, I., and Lee, Y. J. (2008). Flavonoids-induced accumulation of hypoxia-inducible factor (HIF)-1α/2α is mediated through chelation of iron. J. Cel. Biochem. 103, 1989–1998. doi:10.1002/jcb.21588
Parmaksiz, M., Elçin, A. E., and Elçin, Y. M. (2020). Decellularized cell culture ECMs act as cell differentiation inducers. Stem Cel Rev Rep 16, 569–584. doi:10.1007/s12015-020-09963-y
Rao N, V., Mane, S., Kishore, A., Das Sarma, J., and Shunmugam, R. (2012). Norbornene derived doxorubicin copolymers as drug carriers with pH responsive hydrazone linker. Biomacromolecules 13, 221–230. doi:10.1021/bm201478k
Rappu, P., Salo, A. M., Myllyharju, J., and Heino, J. (2019). Role of prolyl hydroxylation in the molecular interactions of collagens. Essays Biochem. 63, 325–335. doi:10.1042/ebc20180053
Romani, P., Valcarcel-Jimenez, L., Frezza, C., and Dupont, S. (2021). Crosstalk between mechanotransduction and metabolism. Nat. Rev. Mol. Cel Biol 22, 22–38. doi:10.1038/s41580-020-00306-w
Schreier, S., Malheiros, S. V. P., and de Paula, E. (2000). Surface active drugs: self-association and interaction with membranes and surfactants. Physicochemical and biological aspects. Biochim. Biophys. Acta (Bba) - Biomembranes 1508, 210–234. doi:10.1016/s0304-4157(00)00012-5
Setayeshmehr, M., Esfandiari, E., Rafieinia, M., Hashemibeni, B., Taheri-Kafrani, A., Samadikuchaksaraei, A., et al. (2019). Hybrid and composite scaffolds based on extracellular matrices for cartilage tissue engineering. Tissue Eng. B: Rev. 25, 202–224. doi:10.1089/ten.teb.2018.0245
Sorrentino, G., Ruggeri, N., Specchia, V., Cordenonsi, M., Mano, M., Dupont, S., et al. (2014). Metabolic control of YAP and TAZ by the mevalonate pathway. Nat. Cel Biol 16, 357–366. doi:10.1038/ncb2936
Stegen, S., Laperre, K., Eelen, G., Rinaldi, G., Fraisl, P., Torrekens, S., et al. (2019). HIF-1α metabolically controls collagen synthesis and modification in chondrocytes. Nature 565, 511–515. doi:10.1038/s41586-019-0874-3
Sullivan, W. J., Mullen, P. J., Schmid, E. W., Flores, A., Momcilovic, M., Sharpley, M. S., et al. (2018). Extracellular matrix remodeling regulates glucose metabolism through TXNIP destabilization. Cell 175, 117–132. doi:10.1016/j.cell.2018.08.017
Sun, B., Zhang, M., Shen, J., He, Z., Fatehi, P., and Ni, Y. (2019). Applications of cellulose-based materials in sustained drug delivery systems. Cmc 26, 2485–2501. doi:10.2174/0929867324666170705143308
Sun, T., Guo, X., Zhong, R., Wang, C., Liu, H., Li, H., et al. (2020). Interactions of alginate-deferoxamine conjugates with blood components and their antioxidation in the hemoglobin oxidation model. Front. Bioeng. Biotechnol. 8, 53. doi:10.3389/fbioe.2020.00053
Suzman, D. L., Agrawal, S., Ning, Y. m., Maher, V. E., Fernandes, L. L., Karuri, S., et al. (2019). FDA Approval Summary: Atezolizumab or Pembrolizumab for the Treatment of Patients with Advanced Urothelial Carcinoma Ineligible for Cisplatin‐Containing Chemotherapy. Oncol. 24, 563–569. doi:10.1634/theoncologist.2018-0084
Taddese, S., Jung, M. C., Ihling, C., Heinz, A., Neubert, R. H. H., and Schmelzer, C. E. H. (2010). MMP-12 catalytic domain recognizes and cleaves at multiple sites in human skin collagen type I and type III. Biochim. Biophys. Acta (Bba) - Proteins Proteomics 1804, 731–739. doi:10.1016/j.bbapap.2009.11.014
Tajima, R., Kawaguchi, N., Horino, Y., Takahashi, Y., Toriyama, K., Inou, K., et al. (2001). Hypoxic enhancement of type IV collagen secretion accelerates adipose conversion of 3T3-L1 fibroblasts. Biochim. Biophys. Acta (Bba) - Mol. Cel Res. 1540, 179–187. doi:10.1016/s0167-4889(01)00114-8
Takpradit, C., Viprakasit, V., Narkbunnam, N., Vathana, N., Phuakpet, K., Pongtanakul, B., et al. (2021). Using of deferasirox and deferoxamine in refractory iron overload thalassemia. Pediatr. Int. 63, 404–409. doi:10.1111/ped.14444
Tao, W., and He, Z. (2018). ROS-responsive drug delivery systems for biomedical applications. Asian J. Pharm. Sci. 13, 101–112. doi:10.1016/j.ajps.2017.11.002
Tibbitt, M. W., Dahlman, J. E., and Langer, R. (2016). Emerging frontiers in drug delivery. J. Am. Chem. Soc. 138, 704–717. doi:10.1021/jacs.5b09974
Tiwari, G., Tiwari, R., Bannerjee, S., Bhati, L., Pandey, S., Pandey, P., et al. (2012). Drug delivery systems: an updated review. Int. J. Pharma Investig. 2, 2–11. doi:10.4103/2230-973x.96920
Unal, A. Z., and West, J. L. (2020). Synthetic ECM: bioactive synthetic hydrogels for 3D tissue engineering. Bioconjug. Chem. 31, 2253–2271. doi:10.1021/acs.bioconjchem.0c00270
Uriel, S., Labay, E., Francis-Sedlak, M., Moya, M. L., Weichselbaum, R. R., Ervin, N., et al. (2009). Extraction and assembly of tissue-derived gels for cell culture and tissue engineering. Tissue Eng. C: Methods 15, 309–321. doi:10.1089/ten.tec.2008.0309
Xing, H., Lee, H., Luo, L., and Kyriakides, T. R. (2020). Extracellular matrix-derived biomaterials in engineering cell function. Biotechnol. Adv. 42, 107421. doi:10.1016/j.biotechadv.2019.107421
Xu, J., Sun, T., Zhong, R., You, C., and Tian, M. (2020). PEGylation of deferoxamine for improving the stability, cytotoxicity, and iron-overload in an experimental stroke model in rats. Front. Bioeng. Biotechnol. 8, 592294. doi:10.3389/fbioe.2020.592294
Zhang, S., Ma, K., Liu, Y., Pan, X., Chen, Q., Qi, L., et al. (2016). Stabilization of hypoxia-inducible factor by DMOG inhibits development of chronic hypoxia-induced right ventricular remodeling. J. Cardiovasc. Pharmacol. 67, 68–75. doi:10.1097/fjc.0000000000000315
Zhdanov, A. V., Okkelman, I. A., Collins, F. W. J., Melgar, S., and Papkovsky, D. B. (2015). A novel effect of DMOG on cell metabolism: direct inhibition of mitochondrial function precedes HIF target gene expression. Biochim. Biophys. Acta (Bba) - Bioenerg. 1847, 1254–1266. doi:10.1016/j.bbabio.2015.06.016
Keywords: extracellar matrix, metabolism, materials, regenerative medicine, tissue engineering
Citation: Sthijns MM, van Blitterswijk CA and LaPointe VL (2021) Synthetic Materials that Affect the Extracellular Matrix via Cellular Metabolism and Responses to a Metabolic State. Front. Bioeng. Biotechnol. 9:742132. doi: 10.3389/fbioe.2021.742132
Received: 15 July 2021; Accepted: 28 September 2021;
Published: 11 October 2021.
Edited by:
Tim B.F. Woodfield, University of Otago, New ZealandReviewed by:
Atakan Tevlek, Middle East Technical University, TurkeyPayam Zarrintaj, University of Montana, United States
Copyright © 2021 Sthijns, van Blitterswijk and LaPointe. This is an open-access article distributed under the terms of the Creative Commons Attribution License (CC BY). The use, distribution or reproduction in other forums is permitted, provided the original author(s) and the copyright owner(s) are credited and that the original publication in this journal is cited, in accordance with accepted academic practice. No use, distribution or reproduction is permitted which does not comply with these terms.
*Correspondence: Vanessa L.S. LaPointe, di5sYXBvaW50ZUBtYWFzdHJpY2h0dW5pdmVyc2l0eS5ubA==