- 1Key Laboratory of Medicinal and Edible Plants Resources Development of Sichuan Education Department, Sichuan Industrial Institute of Antibiotics, Chengdu University, Chengdu, China
- 2Key Laboratory of Industrial Biotechnology, Ministry of Education, Jiangnan University, Wuxi, China
- 3National Engineering Laboratory for Cereal Fermentation Technology, Jiangnan University, Wuxi, China
The compounds 5-aminovalerate and δ-valerolactam are important building blocks that can be used to synthesize bioplastics. The production of 5-aminovalerate and δ-valerolactam in microorganisms provides an ideal source that reduces the cost. To achieve efficient biobased coproduction of 5-aminovalerate and δ-valerolactam in Escherichia coli, a single biotransformation step from L-lysine was constructed. First, an equilibrium mixture was formed by L-lysine α-oxidase RaiP from Scomber japonicus. In addition, by adjusting the pH and H2O2 concentration, the titers of 5-aminovalerate and δ-valerolactam reached 10.24 and 1.82 g/L from 40 g/L L-lysine HCl at pH 5.0 and 10 mM H2O2, respectively. With the optimized pH value, the δ-valerolactam titer was improved to 6.88 g/L at pH 9.0 with a molar yield of 0.35 mol/mol lysine. The ratio of 5AVA and δ-valerolactam was obviously affected by pH value. The ratio of 5AVA and δ-valerolactam could be obtained in the range of 5.63:1–0.58:1 at pH 5.0–9.0 from the equilibrium mixture. As a result, the simultaneous synthesis of 5-aminovalerate and δ-valerolactam from L-lysine in Escherichia coli is highly promising. To our knowledge, this result constitutes the highest δ-valerolactam titer reported by biological methods. In summary, a commercially implied bioprocess developed for the coproduction of 5-aminovalerate and δ-valerolactam using engineered Escherichia coli.
Introduction
Over the years, mounting global environmental, climate change, economic concerns, and fossil fuel sources are leading to a shift in the production of traditional bulk chemicals toward more green, renewable, economic, and sustainable routes (Wang A. et al., 2020; Gordillo Sierra and Alper, 2020; Wendisch, 2020). In many cases, the need has been partially met by biorefineries, in which microbial cell factories convert renewable feedstock resources into high-value and useful chemicals (Gao et al., 2020; Klenk et al., 2020; Youn et al., 2020; Zhang et al., 2021). While many chemicals are being developed via biotechnology, polyamide monomers are an important class of compounds (Li et al., 2020; Prell et al., 2020; Osire et al., 2021). 5-Aminovalerate (5AVA) and δ-valerolactam are attractive monomers for the production of biopolyamides, serving as raw materials for clothes, architecture, and disposable goods.
Plastics are mainly derived from petroleum feedstock. Bioplastics have attracted enormous interest because of their main degradability (Ben Abdallah et al., 2020). The annual output of bioplastics is predicted to exceed 2.43 million tons in 2024 (Haupka et al., 2020). Among microbial bioplastics, biopolyamides are widely applied in chemical, automotive, and textile industries (Ligon et al., 2017). The monomers of polyamides are primarily dicarboxylic acids, diamines, lactams, and ω-amino acids (Radzik et al., 2019). Examples of these main platform chemicals range from succinate (Zhang et al., 2009), glutarate (Zhao et al., 2018), to adipate (Wang F. et al., 2020) for dicarboxylic acids; from putrescine, cadaverine (Rui et al., 2020; Xue et al., 2020), to 1,6-hexanediamine for diamines; from δ-valerolactam (Zhang et al., 2017a), to ε-caprolactam (Thompson et al., 2020) for lactams; from 3-hydroxybutyrate (Atakav et al., 2021; Mierziak et al., 2021; Schmid et al., 2021), 2-hydroxybutyrate (Tian et al., 2021), to 3-hydroxyhexanoate (Harada et al., 2021) for hydroxyl acids; and from 4-aminobutyrate, 5AVA (Cheng et al., 2021b), to 6-aminocaproate (Turk et al., 2016) for ω-amino acids. In this respect, also 5AVA (Adkins et al., 2013) and δ-valerolactam (Xu et al., 2020) are attractive C5 platform chemicals for the production of biopolyamides from renewable biomass.
Four metabolic routes of 5AVA from L-lysine have been developed so far. The first route is the 5-aminovaleramide–mediated pathway that involves L-lysine 2-monooxygenase (DavB) and δ-aminovaleramidase (DavA) (Joo et al., 2017). The engineering WL3110 strain with overexpression of DavA and DavB generated 3.6 g/L 5AVA (Park et al., 2013). Shin et al. reported that 33.1 g/L of 5AVA was successfully formed by promoter optimization (Shin et al., 2016). The second route is the cadaverine-mediated pathway that does not require oxygen involves L-lysine decarboxylase (LdcC), putrescine transaminase (PatA), and γ-aminobutyraldehyde dehydrogenase (PatD) (Haupka et al., 2020). Haupka et al. reported that 3.7 g/L 5AVA was reached, with a yield of 0.09 g/g in shake flasks (Haupka et al., 2020). The third route is 2-keto-6-aminocaproate (2K6AC)–mediated pathway that involves L-lysine α-oxidase (RaiP) from Scomber japonicus (S. japonicus) and H2O2 (Pukin et al., 2010). Pukin et al. found that 13.4 g/L 5AVA was enzymatically produced by RaiP from Trichoderma viride (Pukin et al., 2010). Interestingly, Cheng et al. proposed that the titer of 5AVA could be improved to 29.12 g/L by adding 4% (v/v) ethanol and 10 mM H2O2 (Cheng et al., 2018b). Independently, a three-step route based on RaiP, α-ketoacid decarboxylase (KivD) from Lactococcus lactis, and aldehyde dehydrogenase (PadA) from Escherichia coli (E. coli) was established in E. coli with 5AVA titer up to about 52.24 g/L (Cheng et al., 2021b).
Lactams are important chemicals used in the manufacture of commercial polyamides. However, there are few reports on the direct bioproduction of lactams from engineered microorganisms. Zhang et al. confirmed that 1.1 g/L γ-butyrolactam was formed from L-glutamate by identifying a newly 2-pyrrolidone synthase ORF26 from Streptomyces aizunensis, with a yield of 0.14 g/g (Zhang et al., 2016). Then, Zhang et al. further revealed the catalytic promiscuity of ORF26, which cyclized ω-amino acids to produce of γ-butyrolactam, δ-valerolactam, and ε-caprolactam (Zhang et al., 2017b). However, the titers of δ-valerolactam and ε-caprolactam achieved were relatively low; 705 mg/L δ-valerolactam and 2.02 mg/L ε-caprolactam were produced, respectively. Chae et al. reported that β-alanine CoA transferase could activate ω-amino acids to produce 54.14 g/L γ-butyrolactam, 29 mg/L δ-valerolactam, and 79.6 μg/L ε-caprolactam, respectively (Chae et al., 2017). In addition, a novel route for δ-valerolactam was discovered through the direct oxidative decarboxylation of L-pipecolic acid by DavB in Xu et al.’s research (Xu et al., 2020). 90.3 mg/L δ-valerolactam was achieved from L-pipecolic acid by DavB expressed in E. coli (Xu et al., 2020). However, the titer of δ-valerolactam generated was rather low (Table 1).
Escapin from Aplysia californica (A. californica) is an L-amino acid oxidase, which could oxidize L-lysine to produce an antimicrobial equilibrium mixture (Kamio et al., 2009). This equilibrium mixture contains cyclic form Δ1-piperidine-2-carboxylase (P2C), 2-hydroxy-piperidine-2-carboxylase (2HP2C) and Δ2-piperidine-2-carboxylase (2P2C) and linear form 2K6AC, 6-amino-2-hydroxy-hex-2-enolate (6A2HH2E), and 6-amino-2,2-dihydroxy-caproate (6A2DHC) (Ko et al., 2008). P2C was proved to be the dominant component of this enzymatic product at any pH using mass spectroscopy and NMR. Interestingly, this equilibrium shifts to produce relatively more 2P2C at more alkaline conditions, 2K6AC, 6A2HH2E, and 6A2DHC under more acidic conditions (Ko et al., 2008). The equilibrium mixture could react with H2O2 to produce 5AVA and δ-valerolactam, and its ratios are affected by pH (Kamio et al., 2009). However, the titers of 5AVA and δ-valerolactam and its ratios were not mentioned in their studies.
In this study, 5AVA and δ-valerolactam were coproduced from an equilibrium mixture by adjusting pH and H2O2 in E. coli (Figure 1). The α-amino group of L-lysine was oxidized by RaiP from S. japonicus to form the equilibrium mixture. 2K6AC, P2C, and 2P2C in this equilibrium mixture were oxidized to generate 5AVA, δ-valerolactam, and δ-valerolactam, respectively. In addition, the ratio of 5AVA and δ-valerolactam could be regulated by pH. The route of coproduction of 5AVA and δ-valerolactam was first proposed in this study. As a result, a promising strategy for coproducing 5AVA and δ-valerolactam in a single biotransformation step by adjusting the pH and H2O2 was established.
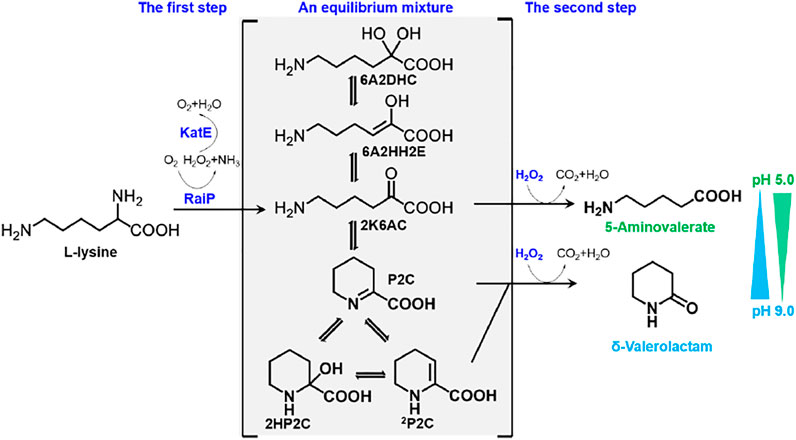
FIGURE 1. Schematic diagram of coproduction routes of 5AVA and δ-valerolactam from L-lysine in this study. RaiP, L-lysine α-oxidase; 5AVA, 5-aminovalerate; P2C, Δ1-piperidine-2-carboxylase; 2HP2C, 2-hydroxy-piperidine-2-carboxylase; 2P2C, Δ2-piperidine-2-carboxylase; 2K6AC, linear form 2-keto-6-aminocaproate; 6A2HH2E, 6-amino-2-hydroxy-hex-2-enolate; 6A2DHC, 6-amino-2,2-dihydroxy-caproate.
Materials and Methods
Strains and Plasmids
The strains and plasmids used in this work are listed in Table 2. The raiP from S. japonicus (Accession No. MG423617) was inserted into pET21a to generate plasmid pET21a-raiP with NdeI and BamHI restriction sites (Cheng et al., 2018b). The gene katE from E. coli MG1655 (Accession No. AAT48137.1) was inserted into pET21a-raiP to generate plasmid pET21a-raiP-katE with SalI and XhoI restriction sites. The engineered E. coli ML03 for knocking out lysine decarboxylase gene cadA was from our previous study (Cheng et al., 2018a). In addition, the plasmid pET21a, pET21a-raiP, and pET21a-raiP-katE were transformed into E. coli BL21 (DE3) or E. coli ML03 to obtain the strains BL21-pET21a, BL21-raiP, BL21-raiP-katE, ML03-pET21a, ML03-raiP, and ML03-raiP-katE, respectively.
Cultivation Conditions
The engineering strains were streaked onto Luria–Bertani (LB) agar plates with 100 mg/L Amp at 37°C for overnight. Engineering strains used for biotransformation in the shake flask were cultured in LB medium with 100 mg/L Amp. After the OD600 reached 0.6, 0.2 mM of isopropyl β-D-thiogalactoside (IPTG) and 6.5 g/L of L-lysine HCl were added. The pH was controlled at 5.0, 6.0, 7.0, 8.0, and 9.0 by NH3·H2O and 10% H2SO4 at 30°C after 12 h. H2O2 was added after 12 h.
Enzyme Assays
RaiP activity was determined as Cheng et al. reported (Cheng et al., 2018b). Briefly, the reaction buffer contained 30 mM L-lysine, 26.5 mM phenol, 0.5 mM 4-aminoantipyrine, and 10 units/ml catalase. Quinoneimine dye formed was measured at 505 nm using SpectraMax M2e. One unit of enzyme activity was defined as the amount of enzyme that catalyzes the formation of 1 μM of H2O2 per minute (Cheng et al., 2018b). The activity of KatE was determined according to Liu et al. (2017); 0.1 ml diluted crude enzyme was incubated with 1 ml 60 mM H2O2 at 30°C for 10 min. The absorbance of a yellow complex formed by molybdate and H2O2 was immediately measured at 405 nm (Liu et al., 2017). One unit of catalase activity was defined as the amount of enzyme decomposing of 1 μmol H2O2 per min.
Biotransformation
Biotransformation was performed in a 5.0-L fermenter. The medium consisted of 55 g/L of glucose, 0.004 g/L of CoCl2·6H2O, 0.02 g/L of Na2SO4, 1.6 g/L of MgSO4·7H2O, 0.0064 g/L of ZnSO4, 0.0006 g/L of Cu2SO4·5H2O, 1.6 g/L of (NH4)2SO4, 0.00756 g/L of FeSO4·7H2O, 2 g/L of citric acid, 7.5 g/L of K2HPO4·3H2O, and 250 μl of antifoam 289. The pH was controlled at 7.0 by the automatic addition of NH3·H2O and 10% H2SO4 at 30°C. After the OD600 reached 20, 0.2 mM IPTG was added to the broth. When the OD600 reached 80, the pH was controlled at 5.0, 6.0, 7.0, 8.0, and 9.0 by the automatic addition of NH3·H2O and 10% H2SO4. L-lysine HCl was added to at an initial concentration of 40 g/L. H2O2 was added after 24 h.
Lysine, 5-Aminovalerate, and δ-Valerolactam Analysis by High-Performance Liquid Chromatography
Lysine, 5AVA, and δ-valerolactam were monitored and quantitated by high-performance liquid chromatography (HPLC). For monitoring L-lysine and 5AVA, samples were derived with phenyl isothiocyanate (PITC) with an Agilent Eclipse XDB-C18 column (4.6 mm × 150 mm × 5 μm), as described by Cheng et al. (2018b). To monitor δ-valerolactam, a Chirex®3126 (D)-penicillamine LC column (4.6 × 250 mm, Phenomenex, USA) was used (Xu et al., 2020).
Results and Discussion
Construction of a Synthetic Route for the Simultaneous Synthesis of 5-Aminovalerate and δ-Valerolactam in E. coli
A synthetic route for the concurrent synthesis of 5AVA and δ-valerolactam from L-lysine was constructed from an equilibrium mixture (Figure 1). The designed route for the coproduction of 5AVA and δ-valerolactam consists of two steps: 1) the deamination of α-amino group in L-lysine to generate an equilibrium mixture by RaiP from S. japonicus, with this equilibrium mixture containing P2C, 2HP2C, 2P2C, 2K6AC, 6A2HH2E, and 6A2DHC; 2) the decarboxylation of 2K6AC, P2C, and 2P2C in this equilibrium mixture to produce 5AVA and δ-valerolactam via H2O2, respectively. First, a plasmid pET21a–raiP was constructed and introduced into E. coli BL21(DE3) to obtain the strain BL21–raiP. As shown in Figure 2, engineering strain BL21-raiP produced 0.26 g/L 5AVA and 0.08 g/L δ-valerolactam in the absence of H2O2, and 0.45 g/L 5AVA and 0.24 g/L δ-valerolactam in pH 7.0 and 5 mM H2O2, respectively. The specific activity of RaiP was 5.14 units/mg. These results demonstrated the feasibility of the coproduction of 5AVA and δ-valerolactam in E. coli. In addition, the strain ML03-raiP with cadA knocked out was constructed. The engineered strain ML03-raiP produced 0.58 g/L 5AVA and 0.29 g/L δ-valerolactam, nearly about 0.29-fold and 0.21-fold increase compared to control strain BL21-raiP at pH 7.0 and 5 mM H2O2 (Figure 2).
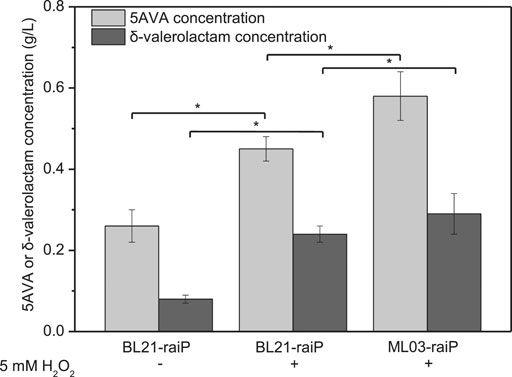
FIGURE 2. Feasibility for the coproduction of 5AVA and δ-valerolactam in a single biotransformation step. pH was controlled at 7.0. 6.5 g/L of L-lysine HCl was added as the substrate. Statistics were performed by two-tailed Student’s t-test. *p < 0.05. All experiments were performed a minimum of three independent sets.
In the past, some studies of the concurrent bioproduction of bulk chemicals were investigated (Li et al., 2017). Few examples are the simultaneous synthesis of 5AVA and glutarate by Corynebacterium glutamicum (Rohles et al., 2016; Haupka et al., 2020), β-glucan and pullulan by engineering Aureobasidium pullulans (Wang G.-L. et al., 2020), acetoin and succinic acid by Enterobacter cloacae (Su et al., 2021), polyhydroxyalkanoates and exopolysaccharides by Yangia sp. ND199 (Romero Soto et al., 2021), and xylitol and ethanol by yeast strains (Shankar et al., 2020). Lopez-Hidalgo et al. reported that the engineered strain increased 30% the coproduction of ethanol and hydrogen used wheat straw and corn stover as feedstock (Lopez-Hidalgo et al., 2021). And 11.0 g/L polyhydroxybutyrate and 1.5 g/L violacein pigment were successfully co-synthesized in Iodobacter sp. PCH194 (Kumar et al., 2021). 7,12-dioxolithocholate and L-tert-leucine were simultaneously produced in a cofactor self-sufficient cascade system for enhancing the atom efficiency (You et al., 2021). Chae et al. found that only 29 mg/L δ-valerolactam was produced by β-alanine CoA transferase (Chae et al., 2017). Xu et al. reported that 90.3 mg/L δ-valerolactam was successfully obtained by an oxidative decarboxylase DavB (Xu et al., 2020). However, the low titers limit the prospect of industrial application.
The Effect of H2O2 on the Simultaneous Synthesis of 5-Aminovalerate and δ-Valerolactam
The effect of H2O2 on the simultaneous synthesis of 5AVA and δ-valerolactam in engineering strain ML03-raiP-katE at pH 7.0 is shown in Figure 3. It showed that the addition of H2O2 had a significant effect on the titers of 5AVA and δ-valerolactam. Engineering E. coli ML03-raiP-katE was cultured in LB medium to form an equilibrium mixture containing P2C, 2HP2C, 2P2C, 2K6AC, 6A2HH2E, and 6A2DHC. At 5 mM H2O2 addition, recombinant ML03-raiP-katE produced 0.58 g/L 5AVA and 0.29 g/L δ-valerolactam after 24 h, respectively, increased about 0.87-fold and 2.22-fold compared to the control group without H2O2. With the continuous increase in H2O2 concentration to 10 mM, the titers of 5AVA and δ-valerolactam both were further increased to 0.96 g/L 5AVA and 0.42 g/L δ-valerolactam, with a yield increase of 2.13-fold and 3.67-fold compared to the control without H2O2 addition, respectively. However, with the increase in H2O2 concentration to 15 mM, the titers of 5AVA and δ-valerolactam decreased dramatically (Figure 3).
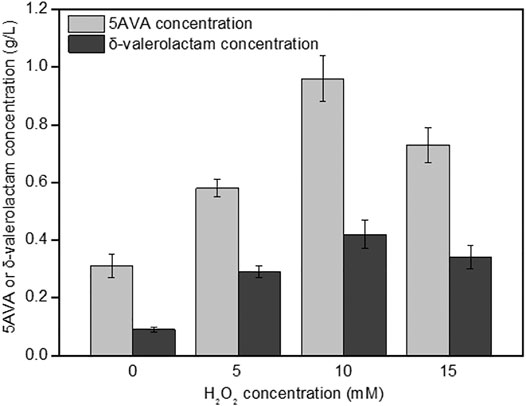
FIGURE 3. Effect of H2O2 on the coproduction of 5AVA and δ-valerolactam by strain ML03-raiP-katE in flasks. pH was controlled at 7.0. 6.5 g/L of L-lysine HCl was added as the substrate. All experiments were performed a minimum of three independent sets.
H2O2 is an important reactive oxygen species in organisms and is produced in response to signal transduction, growth, and development (Oldroyd, 2013; Sies and Jones, 2020). H2O2 enters cells to regulate signaling and cellular processes through aquaporin membrane proteins and covalently modifies cytoplasmic proteins (Sies and Jones, 2020). Wu et al. found that H2O2 sensor HPCAI is a receptor kinase (Wu et al., 2020). However, excess of H2O2 could inhibit cell growth and affect the production of target compounds, resulting in low OD600 (Cheng et al., 2018b). Therefore, in this study, a strategy was proposed that the H2O2 produced by RaiP was decomposed by overexpression of catalase in E. coli in the early stage, and then H2O2 was added in the later stage to produce 5AVA and δ-valerolactam. The specific activity of KatE was 23.58 units/mg. The H2O2 that is generated by RaiP can affect the cell growth and the titers of products (Cheng et al., 2021b). The coexpression of RaiP and KatE in E. coli might provide a more convenient and effective method for the production of 5AVA and δ-valerolactam. As shown in Supplementary Figure S1, the coexpressed E. coli BL21 (DE3) strain harboring pET21a-raiP-katE showed another distinct 84-kDa band on SDS-PAGE, which was consistent with the calculated molecular weight of catalase.
The Effect of pH on the Ratio of 5-Aminovalerate and δ-Valerolactam
The effect of pH on the ratio of 5AVA and δ-valerolactam in engineering strain ML03-raiP-katE with 10 mM H2O2 addition is shown in Table 3. It showed that the pH had a great effect on the ratio of 5AVA and δ-valerolactam; 1.12 g/L 5AVA and 0.25 g/L δ-valerolactam were generated at pH 5.0 after adding H2O2 for 12 h. The maximum ratio of 5AVA and δ-valerolactam was reached 4.48:1 at pH 5.0. With the increase in pH, the titer of δ-valerolactam increased gradually, resulting in a decrease in the ratio of 5AVA and δ-valerolactam; 1.08 g/L 5AVA and 0.33 g/L δ-valerolactam were obtained at pH 6.0. When the pH value was 7.0, recombinant ML03-raiP-katE could produce 0.96 g/L 5AVA and 0.42 g/L δ-valerolactam after 24 h from the equilibrium mixture. In addition, the titer of δ-valerolactam increased significantly to 0.56 g/L at pH 8.0, with a titer increase of 0.33-fold compared with pH 7.0. Interestingly, the titer of δ-valerolactam was higher than 5AVA at pH 9.0, and the ratio of 5AVA and δ-valerolactam was 0.91. As a result, the flux of the equilibrium mixture would shift to 5AVA under acidic condition and to δ-valerolactam under alkaline condition. These findings are consistent with Kamio’s research (Kamio et al., 2009). However, their specific ratio has not been reported (Ko et al., 2008; Kamio et al., 2009).
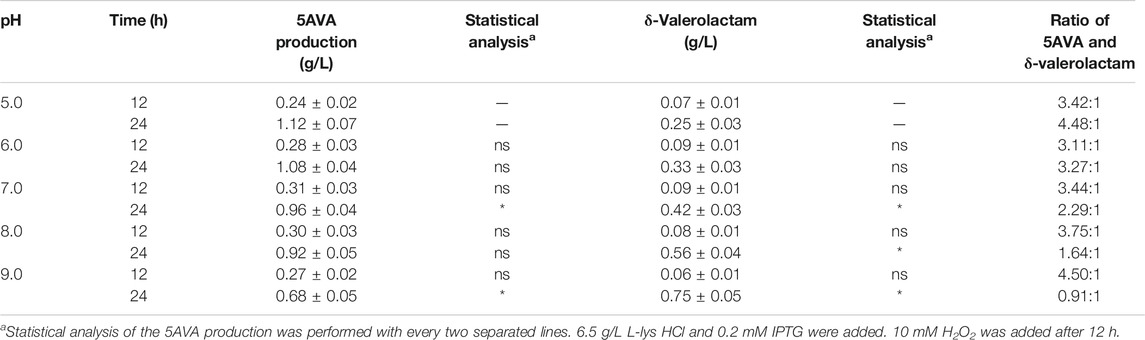
TABLE 3. Effect of pH on the ratio of 5AVA and δ-valerolactam in ML03-raiP-katE. Data are presented as means ± STDV calculated from three replicate biotransformation experiments. Statistics were performed by the two-tailed Student’s t-test. *p < 0.05; ns, not significant.
Biotransformation for the Coproduction of 5-Aminovalerate and δ-Valerolactam
Time profiles for the simultaneous synthesis of 5AVA and δ-valerolactam were investigated by biotransformation of engineered strain ML03-raiP-katE at pH 5.0 (Figure 4A) and pH 9.0 (Figure 4B) in a 5-L fermenter. The catalase KatE was overexpressed to remove H2O2, which significantly improved OD600 and the titer of products in the 5-L fermenter (Cheng et al., 2021b). The titers of 5AVA and δ-valerolactam were very low before the addition of H2O2. In this process, the main accumulation was the equilibrium mixture produced by RaiP from lysine. Although H2O2 was produced by RaiP, its low concentration leads to low production of 5AVA and δ-valerolactam. After adding H2O2 for 12 h, the titers of 5AVA and δ-valerolactam increased significantly to 8.88 and 1.56 g/L at pH 5.0. Finally, 10.24 g/L 5AVA and 1.82 g/L δ-valerolactam were obtained, with a total molar yield of 0.52 mol/mol lysine, and its ratio was 5.63:1 at pH 5.0. The difference was that the titers of 5AVA and δ-valerolactam were 3.42 and 5.12 g/L after adding H2O2 for 12 h at pH 9.0. Finally, 3.98 g/L 5AVA and 6.88 g/L δ-valerolactam were obtained, with a total molar yield of 0.51 mol/mol lysine, and its ratio was 0.58:1 at pH 9.0. The previous results showed that the ratio of 5AVA and δ-valerolactam was significantly regulated by pH. δ-Valerolactam would be the main component in alkaline condition.
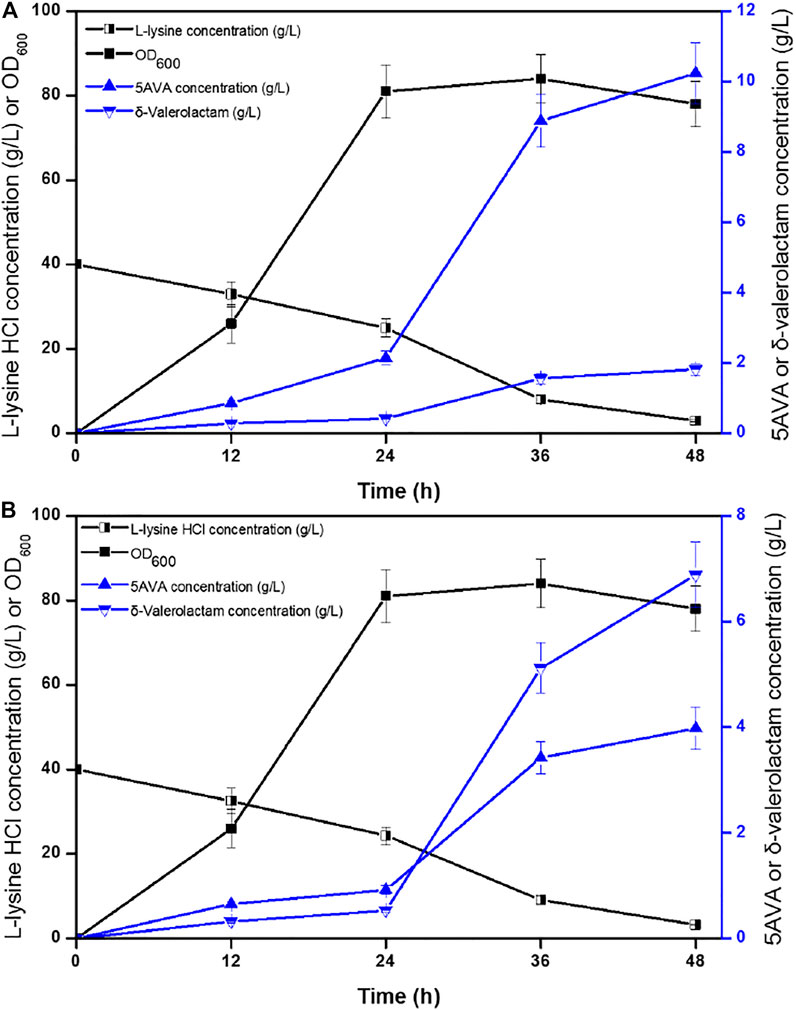
FIGURE 4. Time profiles of 5AVA and δ-valerolactam production were investigated by biotransformation of engineered strain ML03-raiP-katE at pH 5.0 (A) or pH 9.0 (B) in a 5-L fermenter. The experiments were conducted at 40 g/L L-lysine HCl, 37°C and 250 rpm. 10 mM H2O2 was added after reaction 24 h. All experiments were performed a minimum of three independent sets.
We have previously reported the production of 5AVA by overexpression of RaiP, but the titer and change in δ-valerolactam were not noticed in this process. At the same time, the addition of ethanol improved the expression level of RaiP, which increases the cost and leads to uneconomical (Cheng et al., 2018b; Cheng et al., 2020; Cheng et al., 2021a). Xu et al. reported that the expression of DavB from P. putida could synthesize 90.3 mg/L of δ-valerolactam from L-pipecolic acid (Xu et al., 2020). Interestingly, the coexpression of RaiP, glucose dehydrogenase GDH, P2C reductase DpkA, and LysP could produce more δ-valerolactam from lysine, up to 242 mg/L (Xu et al., 2020). This may be due to the fact that part of δ-valerolactam does not originate from the oxidative decarboxylation of L-pipecolic acid but from this equilibrium mixture in this study. Compared with other biotransformation for production of 5AVA, the advantage in this study was to realize the simultaneous synthesis of 5AVA and δ-valerolactam. In terms of biotransformation mechanism, the simultaneous synthesis of 5AVA and δ-valerolactam mainly includes two steps: 1) the formation of an equilibrium mixture by RaiP from lysine and 2) the oxidization of the equilibrium mixture to 5AVA and δ-valerolactam by H2O2 at different pH values.
Conclusion
Many important monomers of polyamides, such as adipate, cadaverine, and 3-hydroxybutyrate, have been extensively studied in microbes. The results presented here demonstrated that engineering E. coli also has the potential to be used as a promising alternative to produce monomers of polyamides derived from petrochemicals. In this study, the strategy for coproducing 5AVA and δ-valerolactam by adjusting the pH and H2O2 in E. coli was proposed. H2O2 was regulated to improve the synthesis efficiency of δ-valerolactam in E. coli in different pH environments, which also increased 5AVA accumulation. The ratio of 5AVA and δ-valerolactam was significantly affected by pH value. δ-Valerolactam would be the main component in alkaline condition. The titers of 5-aminovalerate and δ-valerolactam reached 3.98 and 6.88 g/L from 40 g/L L-lysine HCl at pH 9.0, with a total yield of 0.51 mol/mol lysine. The present findings indicated a promising strategy for the simultaneous synthesis of two commercial products in a single biotransformation step. These strategies could be widely applied for sustainable production of many commercially monomers of polyamides.
Data Availability Statement
The original contributions presented in the study are included in the article/Supplementary Material; further inquiries can be directed to the corresponding authors.
Author Contributions
JC, WT, and ZL performed the experiments and analyzed the data. LL, XG, and XW analyzed data. JC and CL drafted the manuscript. JC, CL, and GZ coordinated the study and finalized the manuscript.
Funding
This work was financially supported by the National Key Research and Development Program of China (2019YFA0904900); the National Natural Science Foundation of China (2210081508); the Open Funding Project of Key Laboratory of Industrial Biotechnology, Ministry of Education, Jiangnan University (KLIB-KF202103); the Natural Science Foundation of Jiangsu Province (BK20190607); the Open Funding Project of the Key Laboratory of Medicinal and Edible Plants Resources Development of Sichuan Education Department (10Y202105).
Conflict of Interest
The authors declare that the research was conducted in the absence of any commercial or financial relationships that could be construed as a potential conflict of interest.
Publisher’s Note
All claims expressed in this article are solely those of the authors and do not necessarily represent those of their affiliated organizations, or those of the publisher, the editors, and the reviewers. Any product that may be evaluated in this article, or claim that may be made by its manufacturer, is not guaranteed or endorsed by the publisher.
Supplementary Material
The Supplementary Material for this article can be found online at: https://www.frontiersin.org/articles/10.3389/fbioe.2021.726126/full#supplementary-material
References
Adkins, J., Jordan, J., and Nielsen, D. R. (2013). EngineeringEscherichia Colifor Renewable Production of the 5-carbon Polyamide Building-Blocks 5-aminovalerate and Glutarate. Biotechnol. Bioeng. 110 (6), 1726–1734. doi:10.1002/bit.24828
Atakav, Y., Pinar, O., and Kazan, D. (2021). Investigation of the Physiology of the Obligate Alkaliphilic Bacillus Marmarensis GMBE 72T Considering its Alkaline Adaptation Mechanism for Poly(3-Hydroxybutyrate) Synthesis. Microorganisms 9 (2), 462. doi:10.3390/microorganisms9020462
Ben Abdallah, M., Karray, F., and Sayadi, S. (2020). Production of Polyhydroxyalkanoates by Two Halophilic Archaeal Isolates from Chott El Jerid Using Inexpensive Carbon Sources. Biomolecules 10 (1), 109. doi:10.3390/biom10010109
Chae, T. U., Ko, Y.-S., Hwang, K.-S., and Lee, S. Y. (2017). Metabolic Engineering of Escherichia coli for the Production of Four-, Five- and Six-Carbon Lactams. Metab. Eng. 41, 82–91. doi:10.1016/j.ymben.2017.04.001
Cheng, J., Luo, Q., Duan, H., Peng, H., Zhang, Y., Hu, J., et al. (2020). Efficient Whole-Cell Catalysis for 5-aminovalerate Production from L-Lysine by Using Engineered Escherichia coli with Ethanol Pretreatment. Sci. Rep. 10 (1), 990. doi:10.1038/s41598-020-57752-x
Cheng, J., Huang, Y., Mi, L., Chen, W., Wang, D., and Wang, Q. (2018a). An Economically and Environmentally Acceptable Synthesis of Chiral Drug Intermediate L-Pipecolic Acid from Biomass-Derived Lysine via Artificially Engineered Microbes. J. Ind. Microbiol. Biot 45, 405–415. doi:10.1007/s10295-018-2044-2
Cheng, J., Luo, Q., Ren, Y., Luo, Z., Liao, W., Wang, X., et al. (2021a). Panorama of Intron Dynamics and Gene Rearrangements in the Phylum Basidiomycota as Revealed by the Complete Mitochondrial Genome of Turbinellus Floccosus. Appl. Microbiol. Biotechnol. 105 (5), 2017–2032. doi:10.1007/s00253-021-11153-w
Cheng, J., Tu, W., Luo, Z., Gou, X., Li, Q., Wang, D., et al. (2021b). A High-Efficiency Artificial Synthetic Pathway for 5-aminovalerate Production from Biobased L-Lysine in Escherichia coli. Front. Bioeng. Biotechnol. 9, 633028. doi:10.3389/fbioe.2021.633028
Cheng, J., Zhang, Y., Huang, M., Chen, P., Zhou, X., Wang, D., et al. (2018b). Enhanced 5-aminovalerate Production in Escherichia coli from L -lysine with Ethanol and Hydrogen Peroxide Addition. J. Chem. Technol. Biotechnol. 93 (12), 3492–3501. doi:10.1002/jctb.5708
Gao, S., Xu, X., Zeng, W., Xu, S., Lyv, Y., Feng, Y., et al. (2020). Efficient Biosynthesis of (2S)-Eriodictyol from (2S)-Naringenin in Saccharomyces cerevisiae through a Combination of Promoter Adjustment and Directed Evolution. ACS Synth. Biol. 9 (12), 3288–3297. doi:10.1021/acssynbio.0c00346
Gordillo Sierra, A. R., and Alper, H. S. (2020). Progress in the Metabolic Engineering of Bio-Based Lactams and Their ω-amino Acids Precursors. Biotechnol. Adv. 43, 107587. doi:10.1016/j.biotechadv.2020.107587
Harada, K., Kobayashi, S., Oshima, K., Yoshida, S., Tsuge, T., and Sato, S. (2021). Engineering of Aeromonas Caviae Polyhydroxyalkanoate Synthase through Site-Directed Mutagenesis for Enhanced Polymerization of the 3-Hydroxyhexanoate Unit. Front. Bioeng. Biotechnol. 9, 627082. doi:10.3389/fbioe.2021.627082
Haupka, C., Delépine, B., Irla, M., Heux, S., and Wendisch, V. F. (2020). Flux Enforcement for Fermentative Production of 5-aminovalerate and Glutarate by Corynebacterium Glutamicum. Catalysts 10 (9), 1065. doi:10.3390/catal10091065
Joo, J. C., Oh, Y. H., Yu, J. H., Hyun, S. M., Khang, T. U., Kang, K. H., et al. (2017). Production of 5-aminovaleric Acid in Recombinant Corynebacterium Glutamicum Strains from a Miscanthus Hydrolysate Solution Prepared by a Newly Developed Miscanthus Hydrolysis Process. Bioresour. Technol. 245 (Pt B), 1692–1700. doi:10.1016/j.biortech.2017.05.131
Jorge, J. M. P., Pérez-García, F., and Wendisch, V. F. (2017). A New Metabolic Route for the Fermentative Production of 5-aminovalerate from Glucose and Alternative Carbon Sources. Bioresour. Technol. 245 (Pt B), 1701–1709. doi:10.1016/j.biortech.2017.04.108
Kamio, M., Ko, K.-C., Zheng, S., Wang, B., Collins, S. L., Gadda, G., et al. (2009). The Chemistry of Escapin: Identification and Quantification of the Components in the Complex Mixture Generated by an L-Amino Acid Oxidase in the Defensive Secretion of the Sea SnailAplysia Californica. Chem. Eur. J. 15 (7), 1597–1603. doi:10.1002/chem.200801696
Klenk, J. M., Ertl, J., Rapp, L., Fischer, M.-P., and Hauer, B. (2020). Expression and Characterization of the Benzoic Acid Hydroxylase CYP199A25 from Arthrobacter Sp. Mol. Catal. 484, 110739. doi:10.1016/j.mcat.2019.110739
Ko, K.-C., Wang, B., Tai, P. C., and Derby, C. D. (2008). Identification of Potent Bactericidal Compounds Produced by Escapin, an L -Amino Acid Oxidase in the Ink of the Sea Hare Aplysia californica. Antimicrob. Agents Chemother. 52 (12), 4455–4462. doi:10.1128/aac.01103-08
Kumar, V., Darnal, S., Kumar, S., Kumar, S., and Singh, D. (2021). Bioprocess for Co-production of Polyhydroxybutyrate and Violacein Using Himalayan Bacterium Iodobacter Sp. PCH194. Bioresour. Technol. 319, 124235. doi:10.1016/j.biortech.2020.124235
Li, L., Zou, D., Ji, A., He, Y., Liu, Y., Deng, Y., et al. (2020). Multilevel Metabolic Engineering of Bacillus Amyloliquefaciens for Production of the Platform Chemical Putrescine from Sustainable Biomass Hydrolysates. ACS Sustain. Chem. Eng. 8 (5), 2147–2157. doi:10.1021/acssuschemeng.9b05484
Li, T., Elhadi, D., and Chen, G.-Q. (2017). Co-production of Microbial Polyhydroxyalkanoates with Other Chemicals. Metab. Eng. 43 (Pt A), 29–36. doi:10.1016/j.ymben.2017.07.007
Li, Z., Xu, J., Jiang, T., Ge, Y., Liu, P., Zhang, M., et al. (2016). Overexpression of Transport Proteins Improves the Production of 5-aminovalerate from L-Lysine in Escherichia coli. Sci. Rep. 6, 30884. doi:10.1038/srep30884
Ligon, S. C., Liska, R., Stampfl, J., Gurr, M., and Mülhaupt, R. (2017). Polymers for 3D Printing and Customized Additive Manufacturing. Chem. Rev. 117 (15), 10212–10290. doi:10.1021/acs.chemrev.7b00074
Liu, Q., Ma, X., Cheng, H., Xu, N., Liu, J., and Ma, Y. (2017). Co-expression of L-Glutamate Oxidase and Catalase in Escherichia coli to Produce α-ketoglutaric Acid by Whole-Cell Biocatalyst. Biotechnol. Lett. 39 (6), 913–919. doi:10.1007/s10529-017-2314-5
Lopez-Hidalgo, A. M., Magaña, G., Rodriguez, F., De Leon-Rodriguez, A., and Sanchez, A. (2021). Co-production of Ethanol-Hydrogen by Genetically Engineered Escherichia coli in Sustainable Biorefineries for Lignocellulosic Ethanol Production. Chem. Eng. J. 406, 126829. doi:10.1016/j.cej.2020.126829
Mierziak, J., Burgberger, M., and Wojtasik, W. (2021). 3-Hydroxybutyrate as a Metabolite and a Signal Molecule Regulating Processes of Living Organisms. Biomolecules 11 (3), 402. doi:10.3390/biom11030402
Oldroyd, G. E. D. (2013). Speak, Friend, and Enter: Signalling Systems that Promote Beneficial Symbiotic Associations in Plants. Nat. Rev. Microbiol. 11 (4), 252–263. doi:10.1038/nrmicro2990
Osire, T., Yang, T., Xu, M., Zhang, X., Long, M., Ngon, N. K. a., et al. (2021). Integrated Gene Engineering Synergistically Improved Substrate-Product Transport, Cofactor Generation and Gene Translation for Cadaverine Biosynthesis in E. coli. Int. J. Biol. Macromolecules 169, 8–17. doi:10.1016/j.ijbiomac.2020.12.017
Park, S. J., Kim, E. Y., Noh, W., Park, H. M., Oh, Y. H., Lee, S. H., et al. (2013). Metabolic Engineering of Escherichia coli for the Production of 5-aminovalerate and Glutarate as C5 Platform Chemicals. Metab. Eng. 16, 42–47. doi:10.1016/j.ymben.2012.11.011
Prell, C., Burgardt, A., Meyer, F., and Wendisch, V. F. (2020). Fermentative Production of L-2-Hydroxyglutarate by Engineered Corynebacterium Glutamicum via Pathway Extension of L-Lysine Biosynthesis. Front. Bioeng. Biotechnol. 8, 630476. doi:10.3389/fbioe.2020.630476
Pukin, A. V., Boeriu, C. G., Scott, E. L., Sanders, J. P. M., and Franssen, M. C. R. (2010). An Efficient Enzymatic Synthesis of 5-aminovaleric Acid. J. Mol. Catal. B: Enzym 65 (1-4), 58–62. doi:10.1016/j.molcatb.2009.12.006
Radzik, P., Leszczyńska, A., and Pielichowski, K. (2019). Modern Biopolyamide-Based Materials: Synthesis and Modification. Polym. Bull. 77 (1), 501–528. doi:10.1007/s00289-019-02718-x
Rohles, C. M., Giesselmann, G., Kohlstedt, M., Wittmann, C., and Becker, J. (2016). Systems Metabolic Engineering of Corynebacterium Glutamicum for the Production of the Carbon-5 Platform Chemicals 5-aminovalerate and Glutarate. Microb. Cel Fact 15 (1), 154. doi:10.1186/s12934-016-0553-0
Romero Soto, L., Thabet, H., Maghembe, R., Gameiro, D., Van-Thuoc, D., Dishisha, T., et al. (2021). Metabolic Potential of the Moderate Halophile Yangia Sp. ND199 for Co-production of Polyhydroxyalkanoates and Exopolysaccharides. Microbiologyopen 10 (1), e1160. doi:10.1002/mbo3.1160
Rui, J., You, S., Zheng, Y., Wang, C., Gao, Y., Zhang, W., et al. (2020). High-efficiency and Low-Cost Production of Cadaverine from a Permeabilized-Cell Bioconversion by a Lysine-Induced Engineered Escherichia coli. Bioresour. Technol. 302, 122844. doi:10.1016/j.biortech.2020.122844
Sasikumar, K., Hannibal, S., Wendisch, V. F., and Nampoothiri, K. M. (2021). Production of Biopolyamide Precursors 5-Amino Valeric Acid and Putrescine from Rice Straw Hydrolysate by Engineered Corynebacterium Glutamicum. Front. Bioeng. Biotechnol. 9, 635509. doi:10.3389/fbioe.2021.635509
Schmid, M., Raschbauer, M., Song, H., Bauer, C., and Neureiter, M. (2021). Effects of Nutrient and Oxygen Limitation, Salinity and Type of Salt on the Accumulation of Poly(3-Hydroxybutyrate) in Bacillus Megaterium Uyuni S29 with Sucrose as a Carbon Source. New Biotechnol. 61, 137–144. doi:10.1016/j.nbt.2020.11.012
Shankar, K., Kulkarni, N. S., Sajjanshetty, R., Jayalakshmi, S. K., and Sreeramulu, K. (2020). Co-production of Xylitol and Ethanol by the Fermentation of the Lignocellulosic Hydrolysates of Banana and Water Hyacinth Leaves by Individual Yeast Strains. Ind. Crops Prod. 155, 112809. doi:10.1016/j.indcrop.2020.112809
Shin, J. H., Park, S. H., Oh, Y. H., Choi, J. W., Lee, M. H., Cho, J. S., et al. (2016). Metabolic Engineering of Corynebacterium Glutamicum for Enhanced Production of 5-aminovaleric Acid. Microb. Cel Fact 15 (1), 174. doi:10.1186/s12934-016-0566-8
Sies, H., and Jones, D. P. (2020). Reactive Oxygen Species (ROS) as Pleiotropic Physiological Signalling Agents. Nat. Rev. Mol. Cel Biol 21 (7), 363–383. doi:10.1038/s41580-020-0230-3
Su, H.-Y., Li, H.-Y., Xie, C.-Y., Fei, Q., and Cheng, K.-K. (2021). Co-production of Acetoin and Succinic Acid by Metabolically Engineered Enterobacter cloacae. Biotechnol. Biofuels 14 (1), 26. doi:10.1186/s13068-021-01878-1
Thompson, M. G., Pearson, A. N., Barajas, J. F., Cruz-Morales, P., Sedaghatian, N., Costello, Z., et al. (2020). Identification, Characterization, and Application of a Highly Sensitive Lactam Biosensor from Pseudomonas Putida. ACS Synth. Biol. 9 (1), 53–62. doi:10.1021/acssynbio.9b00292
Tian, L., Zhou, J., Yang, T., Zhang, X., Xu, M., and Rao, Z. (2021). Cascade Biocatalysis for Production of Enantiopure (S)-2-hydroxybutyric Acid Using Recombinant Escherichia coli with a Tunable Multi-Enzyme-Coordinate Expression System. Syst. Microbiol. Biomanuf 1 (2), 234–244. doi:10.1007/s43393-020-00021-9
Turk, S. C. H. J., Kloosterman, W. P., Ninaber, D. K., Kolen, K. P. A. M., Knutova, J., Suir, E., et al. (2016). Metabolic Engineering toward Sustainable Production of Nylon-6. ACS Synth. Biol. 5 (1), 65–73. doi:10.1021/acssynbio.5b00129
Wang, A., Duncan, S. E., Whalley, N. W., and O'Keefe, S. F. (2020). Interaction Effect of LED Color Temperatures and Light-Protective Additive Packaging on Photo-Oxidation in Milk Displayed in Retail Dairy Case. Food Chem. 323, 126699. doi:10.1016/j.foodchem.2020.126699
Wang, F., Zhao, J., Li, Q., Yang, J., Li, R., Min, J., et al. (2020). One-pot Biocatalytic Route from Cycloalkanes to α,ω‐dicarboxylic Acids by Designed Escherichia coli Consortia. Nat. Commun. 11 (1), 5035. doi:10.1038/s41467-020-18833-7
Wang, G.-L., Din, A. U., Qiu, Y.-S., Wang, C.-L., Wang, D.-H., and Wei, G.-Y. (2020c). Triton X-100 Improves Co-production of β-1,3-D-glucan and Pullulan by Aureobasidium Pullulans. Appl. Microbiol. Biotechnol. 104 (24), 10685–10696. doi:10.1007/s00253-020-10992-3
Wang, X., Cai, P., Chen, K., and Ouyang, P. (2016). Efficient Production of 5-aminovalerate from L -lysine by Engineered Escherichia coli Whole-Cell Biocatalysts. J. Mol. Catal. B: Enzymatic 134, 115–121. doi:10.1016/j.molcatb.2016.10.008
Wendisch, V. F. (2020). Metabolic Engineering Advances and Prospects for Amino Acid Production. Metab. Eng. 58, 17–34. doi:10.1016/j.ymben.2019.03.008
Wu, F., Chi, Y., Jiang, Z., Xu, Y., Xie, L., Huang, F., et al. (2020). Hydrogen Peroxide Sensor HPCA1 Is an LRR Receptor Kinase in Arabidopsis. Nature 578 (7796), 577–581. doi:10.1038/s41586-020-2032-3
Xu, Y., Zhou, D., Luo, R., Yang, X., Wang, B., Xiong, X., et al. (2020). Metabolic Engineering of Escherichia coli for Polyamides Monomer δ-valerolactam Production from Feedstock Lysine. Appl. Microbiol. Biotechnol. 104 (23), 9965–9977. doi:10.1007/s00253-020-10939-8
Xue, Y., Zhao, Y., Ji, X., Yao, J., Busk, P. K., Lange, L., et al. (2020). Advances in Bio-Nylon 5X: Discovery of New Lysine Decarboxylases for the High-Level Production of Cadaverine. Green. Chem. 22 (24), 8656–8668. doi:10.1039/d0gc03100c
You, Z.-N., Zhou, K., Han, Y., Yang, B.-Y., Chen, Q., Pan, J., et al. (2021). Design of a Self-Sufficient Hydride-Shuttling cascade for Concurrent Bioproduction of 7,12-dioxolithocholate and L-Tert-Leucine. Green. Chem. 23, 4125–4133. doi:10.1039/d1gc01120k
Youn, J.-W., Albermann, C., and Sprenger, G. A. (2020). In Vivo cascade Catalysis of Aromatic Amino Acids to the Respective Mandelic Acids Using Recombinant E. coli Cells Expressing Hydroxymandelate Synthase (HMS) from Amycolatopsis Mediterranei. Mol. Catal. 483, 110713. doi:10.1016/j.mcat.2019.110713
Zhang, J., Barajas, J. F., Burdu, M., Ruegg, T. L., Dias, B., and Keasling, J. D. (2017a). Development of a Transcription Factor-Based Lactam Biosensor. ACS Synth. Biol. 6 (3), 439–445. doi:10.1021/acssynbio.6b00136
Zhang, J., Barajas, J. F., Burdu, M., Wang, G., Baidoo, E. E., and Keasling, J. D. (2017b). Application of an Acyl-CoA Ligase from Streptomyces Aizunensis for Lactam Biosynthesis. ACS Synth. Biol. 6 (5), 884–890. doi:10.1021/acssynbio.6b00372
Zhang, J., Kao, E., Wang, G., Baidoo, E. E. K., Chen, M., and Keasling, J. D. (2016). Metabolic Engineering of Escherichia coli for the Biosynthesis of 2-pyrrolidone. Metab. Eng. Commun. 3, 1–7. doi:10.1016/j.meteno.2015.11.001
Zhang, S., Fang, Y., Zhu, L., Li, H., Wang, Z., Li, Y., et al. (2021). Metabolic Engineering of Escherichia coli for Efficient Ectoine Production. Syst. Microbiol. Biomanufacturing. doi:10.1007/s43393-021-00031-1
Zhang, X., Jantama, K., Moore, J. C., Jarboe, L. R., Shanmugam, K. T., and Ingram, L. O. (2009). Metabolic Evolution of Energy-Conserving Pathways for Succinate Production in Escherichia coli. Proc. Natl. Acad. Sci. 106 (48), 20180–20185. doi:10.1073/pnas.0905396106
Keywords: 5-aminovalerate, δ-valerolactam, L-lysine HCl, equilibrium mixture, H2O2
Citation: Cheng J, Tu W, Luo Z, Liang L, Gou X, Wang X, Liu C and Zhang G (2021) Coproduction of 5-Aminovalerate and δ-Valerolactam for the Synthesis of Nylon 5 From L-Lysine in Escherichia coli. Front. Bioeng. Biotechnol. 9:726126. doi: 10.3389/fbioe.2021.726126
Received: 16 June 2021; Accepted: 04 August 2021;
Published: 16 September 2021.
Edited by:
Yi-Rui Wu, Shantou University, ChinaReviewed by:
Bin Zhang, Jiangxi Agricultural University, ChinaShuke Wu, University of Greifswald, Germany
Copyright © 2021 Cheng, Tu, Luo, Liang, Gou, Wang, Liu and Zhang. This is an open-access article distributed under the terms of the Creative Commons Attribution License (CC BY). The use, distribution or reproduction in other forums is permitted, provided the original author(s) and the copyright owner(s) are credited and that the original publication in this journal is cited, in accordance with accepted academic practice. No use, distribution or reproduction is permitted which does not comply with these terms.
*Correspondence: Chao Liu, aGlzdGxpdWNoYW9AMTYzLmNvbQ==; Guoqiang Zhang, Z3F6aGFuZ0BqaWFuZ25hbi5lZHUuY24=