- 1School of Biological Engineering, Dalian Polytechnic University, Dalian, China
- 2Key Laboratory of Systems Microbial Biotechnology, Chinese Academy of Sciences, Tianjin, China
- 3Tianjin Institute of Industrial Biotechnology, Chinese Academy of Sciences, Tianjin, China
- 4National Technology Innovation Center of Synthetic Biology, Tianjin, China
- 5University of Chinese Academy of Sciences, Beijing, China
Vitamins are a group of essential nutrients that are necessary to maintain normal metabolic activities and optimal health. There are wide applications of different vitamins in food, cosmetics, feed, medicine, and other areas. The increase in the global demand for vitamins has inspired great interest in novel production strategies. Chemical synthesis methods often require high temperatures or pressurized reactors and use non-renewable chemicals or toxic solvents that cause product safety concerns, pollution, and hazardous waste. Microbial cell factories for the production of vitamins are green and sustainable from both environmental and economic standpoints. In this review, we summarized the vitamins which can potentially be produced using microbial cell factories or are already being produced in commercial fermentation processes. They include water-soluble vitamins (vitamin B complex and vitamin C) as well as fat-soluble vitamins (vitamin A/D/E and vitamin K). Furthermore, metabolic engineering is discussed to provide a reference for the construction of microbial cell factories. We also highlight the current state and problems encountered in the fermentative production of vitamins.
Introduction
Vitamins are essential for proper growth and health of animals, that cannot produce vitamins by themselves or that synthesize insufficient amount to cover all their needs (Capone and Sentongo, 2019; Suter, 2020). The methods of producing vitamins are based either on chemical synthesis or fermentative production (Yuan et al., 2020).
There are at least 30 kinds of different compounds considered “vitamins,” more than 20 vitamins of which are known to be necessary for biological health. Vitamins are either water-soluble or fat-soluble. As the name suggests, a water-soluble vitamin dissolves in water easily and insoluble in organic solvents. After absorption, the body stores very little of such proteins, and most are excreted with urine (Berdanier and Adkins, 2019). Fat-soluble vitamins are dissolved in fats but not in water, and which are stored in the liver or fatty tissues for future use. While vitamins are essential nutrients for all living things, many plants and microorganisms can synthesize them naturally by themselves. By contrast, humans and other animals need to acquire sufficient vitamins with their diet or through supplements to maintain optimal health (Blake and Konings, 2019).
Traditionally, vitamin production strains have been improved through mutagenesis and metabolic engineering, which can be conducted either through chemical or biological means (Vandamme and Revuelta, 2016b). The main chemical strategies include chemical mutagenesis, application of N+ ion beam, ultraviolet radiation or laser mutagenesis. The biological methods mainly include the construction and mutagenesis of the starting strain, genetic modification, synthetic biotechnology, optimization of media and culture conditions, construction of biofilm reactors, etc. (Nie et al., 2013; Song et al., 2014). A series of biotechnological methods are used to transform the metabolic network of cells to construct a programmable “chassis” and “programmable” whole, which can be used to develop an effective assembly strategy, test the adaptability of external components and modules after loading, forming a fine-tuned and customized biological application system. To drive the iterative evolution of other industrial strains, and effectively promote the transformation and renewal of high vitamin producing strains. Chemical methods are usually expensive, environment-unfriendly, waste-prone, and the costly waste disposal. However, the microbial fermentation method has attracted much attention due to low cost, low energy consumption and easy waste recycling. At present, the fermentation method has been recognized by researchers, and it is more environment-friendly and safe than chemical methods. As the fermentation technology matures, this approach is increasingly being used in industry to increase the production of different vitamins. For example, fermentation processes for the production of vitamin B2 (VB2), vitamin B12 (VB12), vitamin C, and vitamin K2 have all been industrialized successfully.
Acevedo-Rocha et al. (2019) reviewed the fermentation of B vitamins from the aspect of sustainability. In this review, we mainly discuss vitamins that can be produced by green fermentation processes. It covers water-soluble vitamins, including vitamin C and vitamin B complex (thiamine, riboflavin, niacin, pantothenic acid, pyridoxine, biotin, folate, and cobalamin) as well as the fat-soluble vitamin E and vitamin K. Here, we discussed the producing microorganisms, advanced biological methods and metabolic bottlenecks of different vitamins.
Water-Soluble Vitamins
B Vitamins
The global demand for B vitamins is growing due to wide applications in food, pharmaceuticals, feed, and other fields. Although most vitamins are manufactured by chemical synthesis, successful industrial bioprocesses have been established for the production of VB2 and VB12. The underlying extraordinary achievement in metabolic engineering is discussed in this article.
Vitamin B1
Vitamin B1, which is also known as thiamine, was the first B vitamin to be identified. Thiamine pyrophosphate (TPP), the active form of thiamine, can inhibit the activity of cholinesterase, reduce skin inflammation, prevent seborrheic dermatitis, or eczema, and improve skin health. Thiamine biosynthesis results from the coupling of the pyrimidine and the thiazole moieties to form thiamine phosphate (Dorrestein et al., 2004; Jurgenson et al., 2009; Cea et al., 2020). Escherichia coli, Salmonella typhimurium, and Bacillus subtilis are the most thoroughly studied thiamine production organisms (Begley et al., 1999).
In chassis cell S. typhimurium, the thiamine pyrimidine moiety can be produced through de novo purine biosynthesis or independently of the purF gene through the alternative pyrimidine biosynthesis (APB) pathway (Downs and Roth, 1991; Downs, 1992). According to the phenotypic characteristics of the abpA mutant, follow-up studies concluded that the functional APB pathway is essential for thiamine synthesis when S. typhimurium grows in the presence of exogenous purines (Downs and Petersen, 1994). Research has shown that overexpression of thiA, nmtA, and thiP in Aspergillus oryzae can increase the vitamin B1 yield fourfold compared to the wild-type (Tokui et al., 2011). Based on the riboswitch mechanism, mutations in the genes of thiamine pyrophosphate kinase activity (thiN) and thiamine-related transport proteins (YkoD and YuaJ) were introduced in B. subtilis TH95. It was recently reported that thiamine biosynthesis is strictly regulated by TPP riboswitches in bacteria/eukaryotes and transcriptional repressors in archaea (Hwang et al., 2017). E. coli has emerged as the preferred cell factory for TPP production after a riboswitch-based biosensors enabled the discovery of thiamine transporters, combined with overexpression of the native thiFSGHCE and thiD genes, which are closely related to Fe-S metabolism (Figure 1A and Table 1; Cardinale et al., 2017).
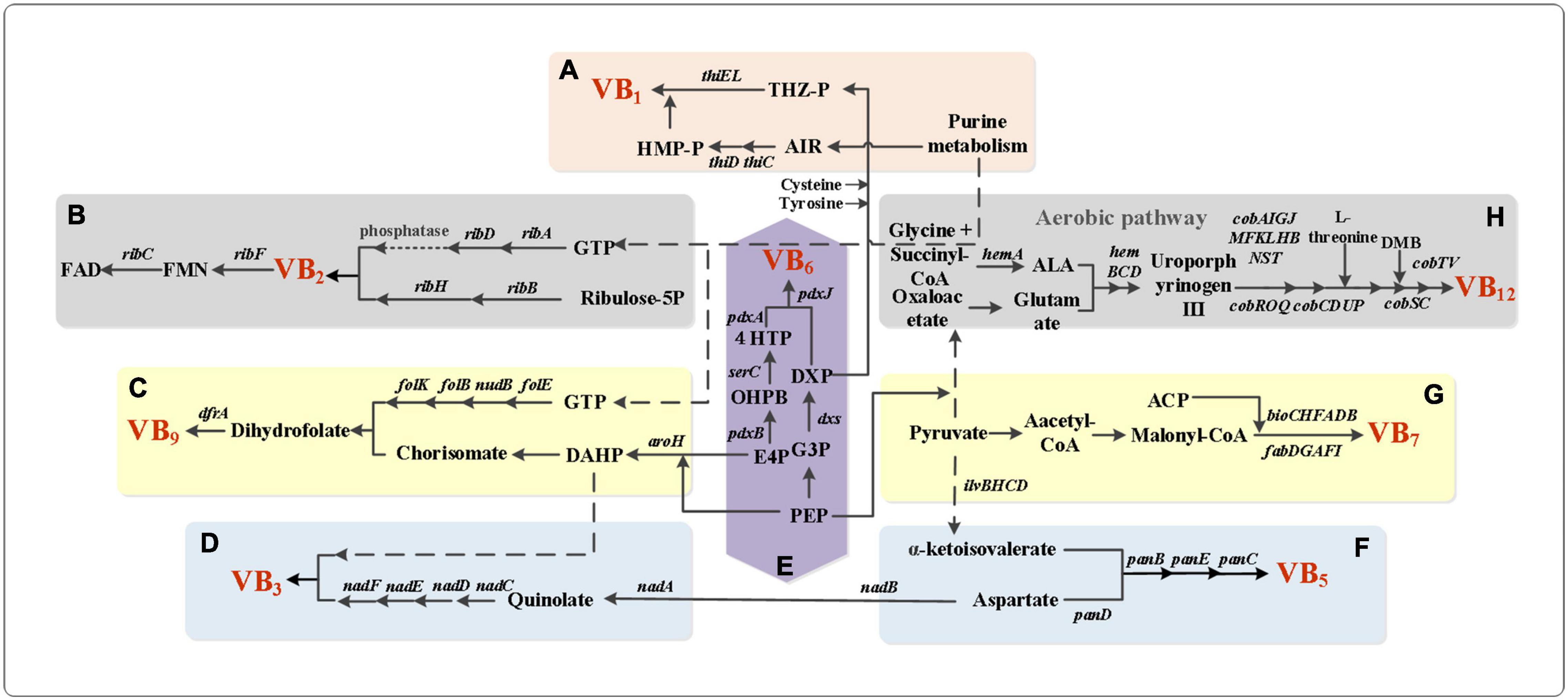
Figure 1. Metabolic network pathway of B vitamins. (A) Biosynthesis pathway of thiamine in E. coli. ThiC/ThiD, phosphomethylpyrimidine synthase; ThiE, thiamine-phosphate pyrophosphorylase; ThiL, thiamine-monophosphate kinase. (B) Biosynthesis pathway of riboflavin in B. subtilis. RibA, GTP cyclohydrolase II; RibB, 3,4-dihydroxy 2-butanone 4-phosphate synthase; RibD, diaminohydroxyphosphoribosylaminopyrimidine deaminase; RibH, 6,7-dimethyl-8-ribityllumazine synthase. RibF, FMN adenylyltransferase; RibC, riboflavin synthase. (C) Vitamin B9 biosynthesis pathway in B. subtilis. AroH, chorismate mutase; FolE, GTP cyclohydrolase IA; NudB, dihydroneopterin triphosphate diphosphatase; FolB, 7,8-dihydroneopterin aldolase/epimerase/oxygenase; FolK, 2-amino-4-hydroxy-6-hydroxymethyldihydropteridine diphosphokinase; DfrB, dihydrofolate reductase. (D) Vitamin B3 biosynthesis pathway in E. coli. NadB, L-aspartate oxidase; NadA, quinolinate synthase; NadC, nicotinate-nucleotide pyrophosphorylase; NadD, nicotinate-nucleotide adenylyltransferase; NadE/NadF, NAD + synthase. (E) De novo biosynthesis pathway of vitamin B6. PdxB, erythronate-4-phosphate dehydrogenase; SerC, phosphoserine aminotransferase; PdxA, 4-hydroxythreonine-4-phosphate dehydrogenase; PdxJ, pyridoxine 5-phosphate synthase; Dxs, 1-deoxy-D-xylulose-5-phosphate synthase. (F) Pathway for de novo synthesis of vitamin B5. ilvBHCD, increased the transcription levels of the ilv genes; panDBEC, pantothenate biosynthetic genes. (G) Biosynthesis pathway of Vitamin B7 in E. coli. BioC, malonyl-CoA O-methyltransferase; BioH, pimeloyl-[acyl-carrier protein] methyl ester esterase; BioF, 8-amino-7-oxononanoate synthase; BioA, 8-amino-7-oxononanoate aminotransferase; BioD, dethiobiotin synthetase; BioB, biotin synthase; FabD, S-malonyltransferase; FabG, 3-oxoacyl-(acyl-carrier protein) reductase; FabA, 3-hydroxyacyl-(acyl-carrier protein) dehydratase; FabF, 3-oxoacyl-(acyl-carrier-protein) synthase II; FabI, enoyl-(acyl-carrier protein) reductase I. (H) The aerobic pathway in the synthesis pathway of cobalamin. HemA, glutamyl-tRNA reductase; ALA, δ-aminolevulinate; HemB, porphobilinogen synthase; HemC, hydroxymethylbilane synthase; HemD, uroporphyrinogen-III synthase; CobA, uroporphyrin-III C-methyltransferase; CobI, precorrin-2 C(20)-methyltransferase; CobG, precorrin-3B synthase; CobJ, precorrin-3B C17-methyltransferase; CobF, precorrin-6A synthase; CobK, precorrin-6A/cobalt-precorrin-6A reductase; CobL, precorrin-6B methyltransferase; CobH, precorrin-8X/cobalt-precorrin-8 methylmutase; CobB, cobyrinic acid a,c-diamide synthase; cobNST, hydrogenobyrinic-acid-a,c-diamide:cobalt cobalt-ligase; CobR, cob(II)yrinic acid a,c-diamide reductase; CobO/CobP, corrinoid adenosyltransferase; CobQ, adenosylcobyric acid synthase; CobS/CobV, adenosylcobinamide-GDP ribazoletransferase; CobC, cobalamin biosynthesis protein; CobD, adenosylcobinamide-phosphate synthase; CobU/CobT, nicotinate-nucleotide–dimethylbenzimidazole phosphoribosyltransferase.
However, thiC/thiH in the thiamine biosynthetic pathway is involved in Fe–S metabolism and is inhibited by S-adenosylmethionine (SAM) metabolites, and the catalytic activity of ThiC enzyme (Figure 2) is very low (kcat = 0.002 s–1) which is one of the main metabolic bottlenecks (Palmer and Downs, 2013). In addition, the cost of chemical production of thiamine is very low, and the production of engineered strains needs to be increased to be expected to be industrialized.
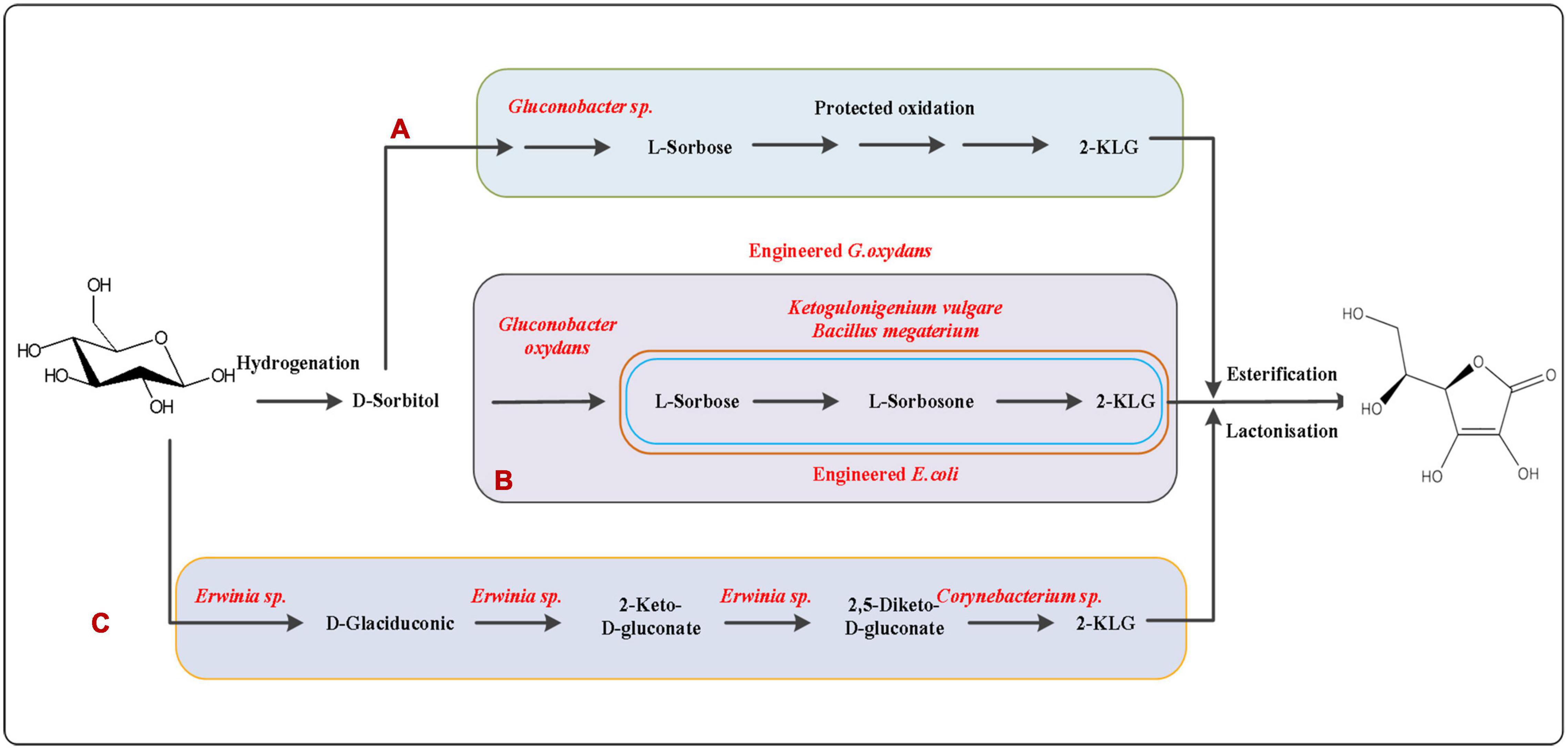
Figure 2. L-ascorbic acid production pathway. (A) Classical Reichstein process: the seven-step Reichstein process involving six chemical transformations and one fermentation by Gluconobacter oxydans. (B) Two steps fermentation by mixed bacteria: G. oxydans, Bacillus megaterium and Ketogulonicigenium vulgare; (C) 2,5-Diketo-D-gluconic acid pathway: mixed or two stage fermentations with Erwinia sp. and Corynebacterium sp. or other species for the direct synthesis of 2-KLG.
Vitamin B2
Riboflavin is an important precursor of flavin mononucleotide (FMN) and flavin adenine dinucleotide (FAD) (Balasubramaniam et al., 2019; Andreieva et al., 2020). Riboflavin insufficiency manifests as persistent anemia (Shi et al., 2014). The biosynthesis of riboflavin begins with guanosine triphosphate and ribose-5-phosphate, followed by six enzymatic steps (Fischer and Bacher, 2005). Burgess et al. (2004) found that overexpression of the ribABCGH genes can increase riboflavin production. Later, it was found that there were both nucleotide substitutions and deletions in the regulatory region of the rib operon. By deregulating the rib operon and purine pathway of B. subtilis, riboflavin production was greatly improved. The specific genetic engineering steps included overexpression of the ribA gene and deletion of the purR gene, after which maximum output of riboflavin reached more than 826.52 mg/L (Figure 1B; Shi et al., 2014). In Candida famata overexpression of sef1 and imh3 was combined with classic mutagenesis methods to construct the high riboflavin-producing strain AF-4. As a result, 1026 ± 50 mg/L of riboflavin can be produced during a fed-batch cultivation in a lab-scale fermenter. This research has made a great contribution to industrial production of riboflavin (Dmytruk et al., 2011, 2014). The two most important industrial producers are Ashbya gossypii and B. subtilis.
In A. gossypii, malate synthase in the glyoxylate cycle is essential for riboflavin production. Deletion of the malate synthase gene (ACR268C) decreased riboflavin production 10-fold compared to the wild-type strain. Conversely, overexpression of the ACR286C gene significantly increased the yield of riboflavin by 70%. These results demonstrated that malate synthase is a new target for improving the production of riboflavin (Sugimoto et al., 2009). Abbas and Sibirny (2011) introduced the icl gene, overexpressed the gly1, prs2,4, and prs3 genes, as well as knocking out the vma4, shm2, and bas1 genes, resulting in riboflavin production of more than 20 g/L.
In B. subtilis, Schwechheimer et al. (2016) overexpressed riboflavin biosynthesis genes, decreased the activity of the flavin kinase RibCF, and improved the de novo purine synthesis and pentose supply, after which the riboflavin yield reached more than 26 g/L. At present, the bottleneck of riboflavin production is mainly due to the poor genetic stability of the engineered strain, and more by-products produced by fermentation, which restrict the high yield of riboflavin.
Vitamin B3
Niacin is the precursor in the synthesis of the pyridine coenzymes NAD (nicotinamide adenine dinucleotide) and NADP (nicotinamide adenine dinucleotide phosphate) (Chand and Savitri, 2016; Chauhan and Poddar, 2019; Tannous et al., 2020). It is found at relatively high concentrations in internal organs of animal, muscle tissues, and fruits. Currently, niacin is mainly used as a feed additive to increase the utilization of feed protein, or as a pharmaceutical intermediate in the synthesis of various drugs. So far, there is no systematic description of a commercial fermentation process of nicotinic acid (NA) or nicotinamide (NAM). Industrial production methods are mainly ammonia oxidation and electrolytic oxidation, but the former has high production costs and needs to be above 300°C during the reaction, and the latter has low costs of production, however, the efficiency of electrolysis is not high, which limits the industrial production of niacin (Chand and Savitri, 2016).
Recent reports describe the use of recombinant E. coli expressing Rhodococcus rhodochrous nitrile hydratase for vitamin B3 production. At low cell density, nicotinamide was produced in fed-batch mode, and the product concentration reached 390 g/L. After high-density culture in 5 L bioreactor, the concentration of nicotinamide reached 508 g/L in 60 min (Figure 1D; Wang et al., 2017). Belenky et al. (2011) showed that the disruption of nrt1 results in increased export of nicotinamide riboside (NR). Moreover, disruption of the niacin transporter Tna1 can also increase the output of niacin, revealing that cells regulate the intracellular NAD+ metabolic process by balancing the transport of niacin, the precursor of NAD+. On the basis of adding 5 mM niacin, yeast cells can produce 8 mg/L nicotinamide mononucleotide (Belenky et al., 2011).
Vitamin B5
Vitamin B5, also known as pantothenic acid, is composed of pantoic acid and β-alanine (β-Ala), which is a precursor of coenzyme A (Leonardi and Jackowski, 2007). It plays an important role in maintaining the health of skin and blood. Its general function is to participate in the production of energy in the body, but it can also control the fat metabolism, and is also an essential nutrient for the brain and nerves. There are chemical and microbial synthesis methods for the synthesis of pantothenic acid, whereby microbial methods can be used to directly synthesize optically pure D-pantothenic acid.
Sahm and Eggeling (1999) adopted a series of methods to increase the production of pantothenic acid, including the deletion of the ilvA gene and the overexpression of the ilvBNCD and panBC genes. The pantothenic acid production of the best strain reached 1000 mg/L (Figure 1F; Sahm and Eggeling, 1999). Huser et al. (2005) also used Corynebacterium glutamicum to produce pantothenic acid. They deleted the ilvA gene, inhibited the expression of the ilvE gene and overexpressed the ilvBNCD gene. The final titer of pantothenate reached 1.75 g/L (Huser et al., 2005). Studies have shown that the specific activity of pantothenic acid synthase PanC of C. glutamicum is 205.10 U/mg. Adding substrates (D-pantothenic acid and β-Ala) to E. coli containing the enzyme can be produced 97.10 U/mg within 32 h, the conversion rate of pantothenic acid was 99.10%. However, the reported work had production defects, which required the addition of exogenous substrate pantothenic acid, and the high market price of pantothenic acid seriously restricted the industrialization of this method. Another chassis organism that is commonly used to produce pantothenic acid is B. subtilis. Hohmann et al. (2016) clarified the highest production of pantothenic acid by overexpressing ilvBHCD, panBCDE, serA, and glyA, as well as the enzymes of the glycine cleavage cycle the purpose is to increase the number of precursors for pantothenic acid synthesis (Figure 1F). The maximal output of the best strain reached 82–86 g/L during a 48 h fed-batch fermentation, opening up a new chapter of vitamin production in the biological world (Hohmann et al., 2016).
Vitamin B6
There are six forms of vitamin B6, including pyridoxine (PN), pyridoxal (PL), and pyridoxamine (PM), as well as their respective phosphate derivatives. It is a water-soluble vitamin, which exists in the form of phosphate in the body. The most versatile form of vitamin B6 is pyridoxal 5′-phosphate (PLP), which is a cofactor of many proteins and enzymes in all organisms. As the most widely available commercial form, PN hydrochloride is extensively used in the pharmaceutical and food industries (Eliot and Kirsch, 2004).
Two de novo synthesis routes have been reported the 1-deoxyxylulose 5-phosphate (DXP)-dependent pathway and the DXP-independent pathway (Tanaka et al., 2005). From large-scale screening studies of different strains, found that the Gram-negative bacterium Sinorhizobium meliloti is the best producer of vitamin B6, reaching a titer of 103 mg/L of B6 isoforms within 168 h. Vitamin B6 production was further increased to 1.30 g/L by expressing the E. coli epd gene and the native dxs gene in this S. meliloti strain (Figure 1E; Hoshino et al., 2007). E. coli and B. subtilis were also engineered to produce vitamin B6. The vitamin B6 production was enhanced to 78 mg/L within 31 h in E. coli, and B. subtilis produced 65 mg/L of PN when supplied with the precursor 4-hydroxy-L-threonine (4HT) (Hoshino et al., 2004). At present, the industry mainly adopts the oxazole method to produce vitamin B6, and the current research also focuses on the improvement of the oxazole method synthesis process. In the process of biosynthesis, the PdxJ enzyme activity is very low (kcat = 0.07 s–1), and the reaction step catalyzed by this enzyme is the rate-limiting step in the VB6 biosynthetic pathway. The intermediate metabolite 4-phosphate hydroxy-threonine (4HTP) is cytotoxic and is also the main bottleneck of biosynthesis. Therefore, the fermentative production of vitamin B6 requires more effort to meet the commercial demand.
Vitamin B7
Biotin is indispensable for the normal metabolism of fats and proteins (Lin and Cronan, 2011; Selvam et al., 2019). It is a nutrient necessary for human growth, development and normal function. Biotin combines with enzymes to participate in the process of carbon dioxide fixation and carboxylation in the body. The current large-scale production of D-biotin is mainly based on the Sternbach synthetic route, and the current industrial production method were improved on this basis. Unless biosynthetic methods can obtain high output at low cost, it is difficult to shake the position of chemical synthesis technology in industrial production. Nevertheless, it was recently reported that some microorganisms can overproduce biotin, which has been elaborated in C. glutamicum, Mesorhizobium loti, and S. meliloti.
In Agrobacterium and Rhizobium HK40, overexpression of the biotin operon from E. coli driven by the powerful tac promoter and introducing a modified RBS in front of bioB resulted in a biotin yield of 110 mg/L (Figure 1G; Streit and Entcheva, 2003). If the native biotin operon is overexpressed in B. subtilis, most enzymes will be strongly inhibited by the by-product of SAM. However, the high demand for SAM by biotin synthase and 7,8-diaminononanoate synthase is still a bottleneck that must be addressed in future research. If lysine is supplied to B. subtilis, BioK will use lysine as the amino donor of the biotin precursor to promote the production of biotin precursor (dephosphorization biotin), and the fermentation process used carbon-limited fed-batch growth conditions with computer control of dissolved oxygen concentrations, but the maximal titer can only reach 21 mg/L biotin. Therefore, improving the catalytic mechanism of biotin synthase is also a challenge for future research (Van Arsdell et al., 2005; Lin and Cronan, 2011).
Vitamin B9
Naturally occurring folic acid is mostly found in the form of polyglutamic acid, and the biologically active form of folic acid is tetrahydrofolate (Myszczyszyn et al., 2019). Deficiency can lead to reduced hemoglobin content in red blood cells, impaired cell maturation and megaloblastic anemia (Lucock, 2000). B. subtilis or A. gossypii were successfully engineered to produce folic acid.
Jagerstad and Jastrebova (2013) achieved a 5-methyltetrahydrofolate (THF) titer of 0.95 mg/L by increasing the supply of precursor substances and blocking the catabolic pathway of THF in B. subtilis. With the continuous research progress, A. gossypii has attracted increasing interest as the chassis strain for folic acid production. A. gossypii can synthesize 0.04 mg/L of folic acid naturally, which can reach 6.59 mg/L after metabolic engineering treatment. This is also the highest production value reported to date (Figure 1C; Serrano-Amatriain et al., 2016). Since the commercial chemical synthesis of folic acid is cheap, unless the environmentally unfriendly part of the chemical synthesis process is restricted, there is still a long way to go for the fermentation of this product.
Vitamin B12
Cobalamin is the only vitamin containing metal elements. Cobalamin is the general term for a class of corrin compounds containing cobalt (Osman et al., 2021). It is the largest and most complex vitamin molecule discovered so far. Vitamin B12 deficiency leads to increased formation of ring sideroblasts in pre-myelodysplastic syndromes (Kitago et al., 2020).
Vitamin B12 is synthesized by microorganisms through de novo synthesis or salvage synthesis in nature, but higher-animals and plants cannot produce it (Figure 1H; Fang et al., 2017). Although in the 19th century, researchers have completed the full chemical synthesis of vitamin B12, the chemical synthesis method is too complicated and expensive, so the world’s major suppliers rely on microbial fermentation to produce vitamins. Pseudomonas denitrificans and Propionibacterium freudenreichii being widely used in industrial fermentation to produce vitamin B12. In order to improve the productivity of vitamin B12, researchers have adopted a random mutagenesis method to construct a vitamin B12 overproducing strains by ultraviolet rays, nitrosoguanidine (NTG), nitrosomethylurethane and ethyleneimine (Blanche et al., 1992, 1995a,b). Propionibacterium shermanii was reported to produce vitamin B12 with a maximum titer of 200 mg/L (Sych et al., 2016). However, the aerobic P. denitrificans remains the most used industrial host, and the effect is obvious. Moreover, P. denitrificans has stronger production capacity than the anaerobic strain that produces vitamin B12 and is widely used in industrial production. Xia et al. (2015) increased the production of vitamin B12 to 198 ± 4.60 mg/L by optimizing the fermentation medium using response surface method. Our research group used E. coli strain MG1655 (DE3) as the starting strain to achieve de novo synthesis of vitamin B12 (Fang et al., 2018). Cai et al. (2018) later used riboswitch elements in S. meliloti for the first time, and successfully developed a flow cytometry high-throughput screening system for high-yield VB12 strains. The vitamin B12 titer of the best strain, S. meliloti MC5–2, reached 156 ± 4.20 mg/L, but the yield was still relatively low. At the same time, they also emphasized that the titer of vitamin B12 is greatly dependent on the medium composition (Cai et al., 2018).
Vitamin C
Vitamin C, also known as L-ascorbic acid (LAA), is an important cofactor for multiple enzyme reaction in the body (Paciolla et al., 2019; Kawahori et al., 2020). It can act as an antioxidant to scavenge free radicals and reduce oxidative stress, so a rapidly expanding market is the application of LAA as an additive to cosmetic products (Timoshnikov et al., 2020). Vitamin C deficiency can result in scurvy. Recently, researchers used biochemical methods combined with DNA recombination technology to produce vitamin C.
At present, L-AA is commercially manufactured via the classic seven-step Reichstein process using D-glucose as the initial substrate. The process involves six chemical steps and one fermentation steps for the oxidation of D-sorbitol to 2-keto-L-gulonic acid (2-KGA) by Gluconobacter oxydans and Bacillus megaterium (Figure 2A). Sugisawa et al. (2005) reported for the first time that Ketogulonigenium vulgare DSM 4025 can produce 1.37 g/L of L-AA under static culture conditions. Kim et al. (1996, 1998) reported that the respective enzymes from Candida albicans and S. cerevisiae convert not only D-arabinose to D-arabinono-1,4-lactone but also L-galactose to L-galactono-1,4-lactone in vitro. Experiments have shown that budding yeast cells overexpressing the endogenous D-arabinono-1,4-lactone oxidase and L-galactose dehydroge-nase can produce about 100 mg/L of L-ascorbic acid (Sauer et al., 2004). A microbiological consortium composed of G. oxydans, K. vulgare, and B. endophyticus was constructed to produce 2-KGA, and a final yield of 73.70 g/L was obtained within 30 h (Figure 2B; Ma et al., 2019). This result holds promise for the construction of a microbial cell factory for the production of vitamin C. However, it has been reported that mixed-bacteria fermentation can be unstable due to competition between the individual strains for nutrients and other factors. Therefore, mixed-bacteria fermentation technology has poor stability and low efficiency, which also hinders the pace of industrial production of vitamin C. Nevertheless, fermentation is expected to become the mainstream way of vitamin C production in the future if stable single strains can be used instead of mixed bacteria fermentation, while also shortening the production cycle.
Fat-Soluble Vitamins
Vitamin A
Vitamin A mainly includes β-carotene, α-carotene, and β-cryptoxanthin (Wise et al., 2021). β-carotene, a provitamin A carotenoid, is divided into all-trans and cis isomers (Yang et al., 2021). All-trans-β-carotene is the major isomer found in unprocessed carotene-rich plant foods, followed by its 9- and 13-cis isomers. β-carotene is an antioxidant, which not only inhibits singlet oxygen but also inhibits lipid peroxidation, thereby playing an important role in the prevention of disease (Kawata et al., 2018).
Carotene is mainly produced by fungi, some bacteria, and algae. For example, Yoon et al. increased the supply of IPP (isopentenyl diphosphate) and DMAPP (dimethylallyl diphosphate) through the introduction of foreign MVA (mevalonate) pathway (Figure 3D), thereby enhancing the production of carotenoids. The final engineered E. coli with a whole MVA pathway and β-carotene synthesis gene can produce β-carotene of 465 mg/L (Figure 3C; Yoon et al., 2009). Adenosine-triphosphate (ATP) and nicotinamide adenine dinucleotide phospha (NADPH) are two important cofactors in β-carotene biosynthesis pathway. Zhao et al. (2013) used E. coli as host cells, constructed and optimized a central metabolic module to increase the supply of ATP and NADPH in β- carotene synthesis pathway, thereby improving the yield of the β-carotene. Finally, the best strain CAR005 increased the β-carotene production to 2.1 g/L with a yield of 60 mg/g DCW in fed-batch fermentation (Zhao et al., 2013). Larroude et al. (2018) overexpressed heterologous carotene synthase (Crt) in Yarrowia lipolytica to make it produce high β-carotene. The fermentation yield of the engineered strain obtained by screening the best promoter was 1.5 g/L. By optimizing the fermentation conditions and using fed-batch fermentation, the yield of β-carotene was further increased production titer of 6.5 g/L and 90 mg/g DCW (Larroude et al., 2018). However, the insufficient number of precursors seriously hindered the industrialization process of β-carotene in the process of β-carotene synthesis in the future.
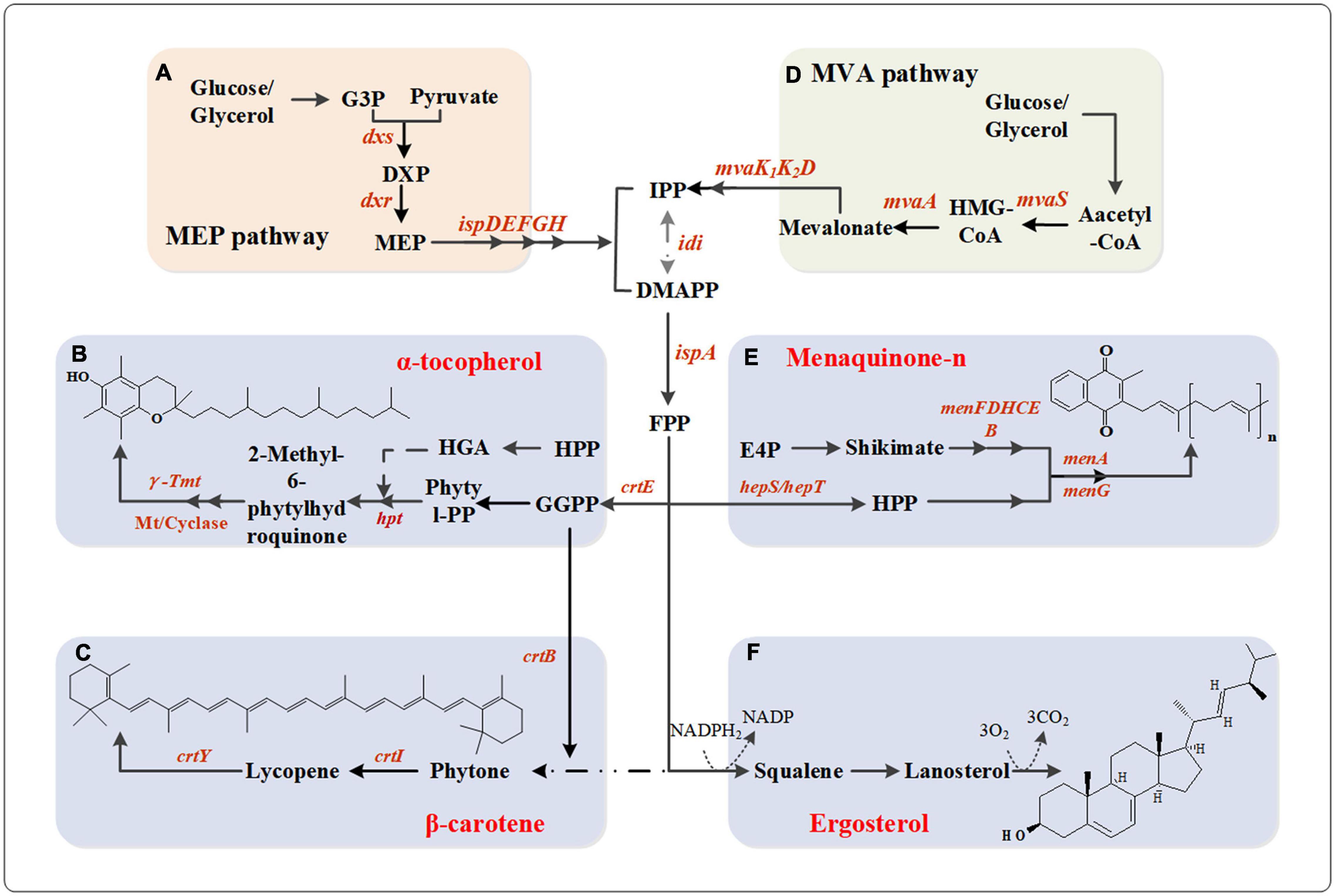
Figure 3. Metabolic network pathway of vitamin A/D/E and vitamin K. (A) MEP pathway. Dxs, 1-deoxy-D-xylulose-5-phosphate synthase; Dxr, 1-deoxy-D-xylulose-5-phosphate reductoisomerase; IspD, 2-C-methyl-D-erythritol 4-phosphate cytidylyltransferase; IspE, 4-diphosphocytidyl-2-C-methyl-D-erythritol kinase; IspF, 2-C-methyl-D-erythritol 2,4-cyclodiphosphate synthase; IspG, 1-hydroxy-2-methyl-2-(E)-butenyl 4-diphosphate synthase; IspH, 4-hydroxy-3-methylbut-2-enyl diphosphate reductase; (B) α-tocopherol biosynthesis pathway. Idi, isopentenyl diphosphate isomerase; IspA, geranyltranstransferase; CrtE, GGPP synthase; hpt, hypoxanthine phosphoribosyltransferase; γ-Tmt, γ-tocopherol methyl-transferase; Mt, methyl-transferase. (C) β-carotene biosynthesis pathway. CrtB, phytoene synthase; CrtI, phytoene desaturase; CrtY, lycopene cyclase. (D) MVA pathway. MvaS, HMG-CoA synthase; MvaA, HMG-CoA reductase; MvaK1, mevalonate kinase; MvaK2, phosphomevalonate kinase; MvaD, diphosphomevalonate decarboxylase; (E) Menaquinone-n biosynthesis pathway. HepS/HepT, heptaprenyl diphosphate synthase component I/II; MenF, isochorismate synthase; MenD, 2-succinyl-5-enolpyruvyl-6-hydroxy-3-cyclohexene-1-carboxylate synthase; MenH, demethylmenaquinone methyltransferase; MenC, o-succinylbenzoate synthase; MenE, o-succinylbenzoate-CoA ligase; MenB, 1,4-dihydroxy-2-naphthoyl-CoA synthase; MenA, 1,4-dihydroxy-2-naphthoate heptaprenyltransferase; MenG, demethylmenaquinone methyltransferase. (F) Ergosterol biosynthesis pathway.
Vitamin D
Vitamin D refers to a group of fat-soluble secosteroids responsible for increasing intestinal absorption of magnesium, calcium, and phosphate, and many other biological effects. The most important compounds are vitamin D2 (ergocalciferol) and vitamin D3 (cholecalciferol) in vitamin D. Vitamin D can increase intestinal absorption of calcium, magnesium, and phosphate, and can prevent many diseases (Yuan et al., 2020).
It is well known that the precursor of vitamin D2 is ergosterol (Papoutsis et al., 2020). Vitamin D2 is widely used in medical, food and other industries. The current commercial production of ergosterol is mainly produced by yeast fermentation. Tan et al. improved the production of ergosterol by optimizing the fermentation medium and screening high ergosterol producing strains (Figure 3F). The results show that dissolved oxygen (DO) can be used as the effective control parameter for yeast fed-batch fermentation. The total yield of ergosterol can be increased to 1.16 g/L when DO was controlled at 12% (±1%) and pulse fed-batch was used (Tan et al., 2003).
Vitamin D3 cannot play a direct role in human and animals, but it can produce the physiologically active form 25-hydroxyvitamin D3 (25-OH-VD3) through the metabolism in liver. At present, the production process of 25-OH-VD3 mainly includes chemical synthesis and light irradiation. The chemical reaction steps are cumbersome, some of them need halogen reagents, and the racemates are generated during the reaction, which makes the separation difficult. Therefore, more and more researchers pay attention to the fermentation of 25-OH-VD3 by microorganisms. The strains used in microbial biosynthesis mainly include Rhodococcus, Streptomyces, Pseudonocardia sp., and Mycobacterium. Vitamin D3 hydroxylase (Vdh) is a kind of cytochrome P450 monooxygenase, which can catalyze the two-step hydroxylation of vitamin D3 (VD3) to produce 25-OH-VD3 and 1α,25-dihydroxyvitamin D3. Yasutake et al. (2013) used nisin, a natural bioactive antimicrobial peptide, to treat Rhodococcus cells containing hydroxylase, and they found that 573 mg/L 25-OH-VD3 can be synthesized. Although the current industrial production of vitamin D3 is mainly dominated by chemical synthesis, microbial synthesis methods are more sustainable and do not produce impurities during the biosynthesis process, thus it will be taken priority in the future industrial production.
Vitamin E
Vitamin E is a group of lipid-soluble antioxidants, including tocopherols and tocotrienols (Muñoz and Munné-Bosch, 2019; Zeng Z. et al., 2020). These compounds are composed of an oxygen-containing double ring system with a hydrophobic prenyl side chain (Blake and Konings, 2019). Lack of vitamin E affects the function of T and B immune cells (Moriguchi and Muraga, 2000). Additionally, patients with severe impairment due to Alzheimer’s disease improved significantly after receiving α-tocopherol (Sano et al., 1997). Considering various physiological effects of tocopherols, they are widely used in the manufacture of human dietary supplements, food preservatives and cosmetics. There are four different tocopherol compounds, named α, β, γ, and δ tocopherol. Among the four forms of vitamin E, α-tocopherol is the most biologically active (Kaiser et al., 1990). In nature, α-tocopherol is produced by photosynthetic organisms, e.g., eukaryotic algae and green plants, some prokaryotic cyanobacteria, such as Synechocystis, which can accumulate vitamin E in large amounts (Figure 3B; Taketomi et al., 1983).
Recently, Euglena gracilis was found to be suitable for the production of high-value products, such as amino acids and ascorbic acid (Schwarzhans et al., 2015). E. gracilis is the most promising host for the commercial production of α-tocopherol, with a high growth rate and α-tocopherol content, which accounts for more than 97% of the total tocopherol accumulated by E. gracilis. Tani and Tsumura added precursors such as homogentisate and L-tyrosine to E. gracilis growth medium, which increased the accumulation of α- tocopherol to 143.60 mg/L corresponding to 5.1 mg/g dry cell weight (DCW) (Tani and Tsumura, 1989). Durmaz (2007). explored the effect of nitrogen source and concentration on the accumulation of α-tocopherol in Nannochloropsis oculata. When sodium nitrate and ammonium chloride were used as inorganic nitrogen source, the highest content of α-tocopherol reached 2.32 ± 0.04 mg/g dry weight (DW) (Table 2). The research showed that higher concentrations of nitrogen in the form of NO3+ and NH4+ can promote production the of α-tocopherol (Durmaz, 2007).
To balance cell growth and product synthesis, Shen et al. (2020) recently combined heterologous genes from photosynthetic organisms with the endogenous shikimate and mevalonate pathways (MEP) to construct a strain of S. cerevisiae that produces tocotrienols (Figure 3A). By incorporating a newly designed cold-shock-triggered temperature control system, the phased control of cell biomass and tocotrienol accumulation by the engineered strains was successfully realized. The final total tocotrienol titer reached 320 mg/L in a 5 L fermenter, which laid the foundation for the production of natural vitamin E in a fully fermentative process (Figure 3B; Shen et al., 2020).
In general, compared with chemical total synthesis, the method of obtaining vitamin E directly through biotechnology has low yield and high cost, and is not suitable for large-scale production. Although chemical total synthesis is currently the main production method of vitamin E, there are still many problems with this technology, such as complex synthesis routes, high technical barriers, etc. Therefore, the development of safer and more efficient synthesis technology has become the main problem to improve the current situation of vitamin E.
Vitamin K
Vitamin K is a fat-soluble vitamin, which also called blood coagulation vitamin in virtue of the function of promoting blood coagulation and preventing osteoporosis (Henrik, 1973; Schwalfenberg, 2017; Zhou et al., 2019). There are two naturally occurring types of vitamin K, called vitamin K1 (phylloquinone/phytomenadione) and vitamin K2 (menaquinone, MK) (Holvik et al., 2019). Vitamin K1 is synthesized by plants, while vitamin K2 is synthesized by microorganisms and can be divided into 14 isoforms depending on the number of isoprenoid units connected to the menaquinone ring (Figure 3E; Schwalfenberg, 2017). Among them, menaquinone-7 (MK-7) is the most effective subtype of vitamin K with a very long half-life in circulation. Notably, MK-7 can be synthesized in the cis, trans, and cis/trans forms, but only the all-trans form is biologically active (Szterk et al., 2018).
The biosynthesis of MK-7 from the embden-meyerhof-parnas (EMP) pathway, pentose phosphate pathway (PPP), MVA pathway and menadione synthesis (MK) pathway (Figure 3C). A number of microorganisms have been used to produce MK-7, including B. subtilis, E. coli, lactic acid bacteria, Flavobacterium sp., and B. amyloliquefaciens (Sharma et al., 1993; Morishita et al., 1999; Sato et al., 2001; Wu, 2011; Taguchi et al., 2014).
Bacillus subtilis isolated from natto (a traditional Japanese food), the strain found in the eponymous fermented Japanese beans, has been certified by the FDA as a food-safe and has a strong ability to produce MK-7. Accordingly, B. subtilis natto was used as a parent strain to develop some of the industrial strains currently on the market. In industrial production, B. subtilis natto fermentation broth was sprayed and dried, and the dry powder from the fermentation broth was subjected to solvent extraction. The obtained extract was condensed into a paste and then purified by chromatography. Using this process, the final yield of MK-7 can reach 200–300 mg/L in the fermentation cycle of 16–24 h (Chen et al., 2016).
Cui et al. (2019) developed a bifunctional quorum-sensing system in B. subtilis 168 to engineer the synthesis modules of MK-7. The resulting strain was capable of producing 360 mg/L MK-7 in shak flasks and 200 mg/L MK-7 in 15-L bioreactor (Cui et al., 2019). Recently, comparative transcriptomics revealed that cell membrane and electron transfer engineering in B. subtilis can improve the synthesis of MK-7. The resulting strain reached a product titer of 410 mg/L after 6 days in shake-flask culture, which is the highest value reported to date (Cui et al., 2020). In the current market environment, the production of natural all-trans MK-7 is via liquid fermentation of B. subtilis natto, which is safe, natural and controllable, and occupies the mainstream position in the market. Compared with the chemically synthesized of trans-MK-7, it has a higher yield and fewer impurities.
Conclusion
The fermentative production of vitamins using bacteria, yeasts or microalgae has many advantages over traditional chemical synthesis methods. From the aspects of safety, biological activity, absorption rate, etc., vitamins manufactured by biological methods can be more suitable for both internal and external applications (Yuan et al., 2020). Although the fermentation of VB2 and VB12 has technologically matured and is being applied in industrial production, fermentation methods for the remaining B-group vitamins have yet to be developed or require significant yield improvement.
Vitamin C has a large market, and its production method is mainly based on single-bacteria fermentation, which eliminates the dependency of associated bacteria by replacing accompanying bacteria with associated active agents (Vandamme and Revuelta, 2016a). However, the current market situation indicates that vitamin C production has overcapacity, the downstream processing is complicated, and the market demand is concentrated in the field of medicine and food. For these reasons, the momentum of price increase will remain slow in the future. Vitamin C fermentation technology can explore the mechanism of a variety of accompanying bacteria, establish their anabolism database, or use isotope technology to label and trace the individual metabolites. It is also possible to design heterologous assembly modules for 2-KGA synthesis, and study adaptation mechanisms in microbial chassis cells, so as to achieve higher productivity (Liu et al., 2011).
The current biosynthesis product of vitamin A is mainly focused on β-carotene. The biosynthesis of β-carotene has successfully established a large-scale production process through classical and reasonable microbial metabolic engineering. However, due to the high barriers of intermediate industry and the complex process of synthesis and metabolism, the future research will face more difficult challenges. At present, the industrial production of vitamin D is mainly through the chemical synthesis of active 25-OH-VD3 and 1α,25-dihydroxyvitamin D3, but the biggest obstacle is the assured quality and security of supply for raw materials, which must be cholesterol with purity greater than 95% (NF grade). Therefore, the key to solving the problem of raw materials is to develop more production bacteria, optimize their metabolic pathways and make them highly productive. With the continuous optimization and technological progress of vitamin E fermentation, the overall cost of the industry has fallen, which promoted the growth of the industry. Unfortunately, although studies have shown that photosynthetic microorganisms have considerable potential for the production of tocopherols, light-driven fermentation is costly, which makes commercialization difficult. However, due to the considerable potential E. gracilis and the conditions of the cultivation environment, the construction of a specifically designed photo-bioreactor may be a feasible research direction for the production of tocopherol. Moreover, the controllable temperature-sensitive control system may also be a key control technology for vitamin E production. Among vitamin K producing bacteria, B. subtilis natto seems to be the most promising candidate for MK production. Many researchers have optimized the design of fermentation modes, medium components, and culture conditions. They have also applied genetic engineering and other means to increase MK production (Szterk et al., 2018). However, to achieve higher industrial output, the technology needs to be further improved. Some studies have used biofilm reactors, which may become a promising new area for future research.
Recently, our research group used E. coli MG1655 (DE3) as chassis strains and achieved the de novo synthesis of vitamin B12 via metabolic engineering and optimization of fermentation conditions. In addition, we have not only proved that E. coli is a microbial biosynthesis platform for the production of vitamin B12, also provides an encouraging example of how the dozens of proteins in a complex biosynthetic pathway can be transferred between organisms to promote industrial production (Fang et al., 2018). In addition, our research group is also doing metabolism research on vitamin B2, B6, B7, and vitamin K.
In general, the development of synthetic biotechnology provides new opportunities for the construction of vitamin cell factories. First, high-throughput screening of high-yield strains, the CRISPR/Cas9 genome editing technology, and automatic gene assembly technology provide important technical means for the mining and genetic modification of chassis cells (Chang et al., 2019; Zeng W. et al., 2020; Zhang and Showalter, 2020). Further, the output of vitamin products in different dimensions will be increased by transforming the complex and multi-enzyme pathways required for the production of vitamins, establishing microbial flora with controllable functions and stability, and application of some advanced engineering technology, such as the cold-shock-triggered temperature control system, dynamic control of gene expression systems, different types of biosensors, cell-free systems and computer-aided design, etc. (Koo et al., 2020; Marucci et al., 2020; Sachsenhauser et al., 2020; Shen et al., 2020; Glasscock et al., 2021). Additionally, the modular and orthogonal strategies are increasingly supporting the construction of vitamin cell factories (Liu et al., 2015). The mining and design of biological components, the assembly and integration of elements and modules, and the optimization and adaptation of the fermentation system are also important for efficient production of vitamins (Santos-Merino et al., 2019). However, there will be many challenges in the field of synthetic biotechnology in the future, including the compatibility between flexible biological systems and rigid engineering systems, or the universality of biological system reconstruction. It will be necessary and important to advance the existing technology, combine it with new strategies, and conduct interdisciplinary research to establish novel microbial cell factories for the industrial fermentation of most vitamins. All in all, we firmly believe that the industrialization of the fermentation production of vitamins is expected to become a broader, safer and more sustainable manufacturing with the continuous advancement of synthetic biotechnology and metabolic engineering.
Author Contributions
YW and LL: manuscript planning, writing, and revision. ZJ and DZ: manuscript revision and writing. All authors contributed to the article and approved the submitted version.
Funding
This study was funded by the National Key R&D Program of China (no. 2019YFA0905300), the National Natural Science Foundation of China under grants nos. 31670604 and 31970324, the Natural Science Foundation of Liaoning Province of China under grant no. 2019020758, the Science and Technology Project of Liaoning Education Department under grant no. 819001110761, and the Tianjin Synthetic Biotechnology Innovation Capacity Improvement Project (no. TSBICIP-CXRC-004).
Conflict of Interest
The authors declare that the research was conducted in the absence of any commercial or financial relationships that could be construed as a potential conflict of interest.
References
Abbas, C. A., and Sibirny, A. A. (2011). Genetic control of biosynthesis and transport of riboflavin and flavin nucleotides and construction of robust biotechnological producers. Microbiol. Mol. Biol. Rev. 75, 321–360. doi: 10.1128/MMBR.00030-10
Acevedo-Rocha, C. G., Gronenberg, L. S., Mack, M., Commichau, F. M., and Genee, H. J. (2019). Microbial cell factories for the sustainable manufacturing of B vitamins. Curr. Opin. Biotechnol 56, 18–29. doi: 10.1016/j.copbio.2018.07.006
Andreieva, Y., Petrovska, Y., Lyzak, O., Liu, W., and Sibirny, A. (2020). Role of the regulatory genes SEF1, VMA1 and SFU1 in riboflavin synthesis in the flavinogenic yeast Candida famata (Candida flareri). Yeast 37, 497–504. doi: 10.1002/yea.3503
Balasubramaniam, S., Christodoulou, J., and Rahman, S. (2019). Disorders of riboflavin metabolism. J. Inherited Metab. Dis. 42, 608–619.
Begley, T. P., Downs, D. M., Ealick, S. E., Mclafferty, F. W., Loon, A. P. G. M. V., Taylor, S., et al. (1999). Thiamin biosynthesis in prokaryotes. Arch. Microbiol. 171, 293–300. doi: 10.1007/s002030050713
Belenky, P., Stebbins, R., Bogan, K. L., Evans, C. R., and Brenner, C. (2011). Nrt1 and Tna1-independent export of NAD+ precursor vitamins promotes NAD+ homeostasis and allows engineering of vitamin production. PLoS One 6:e19710. doi: 10.1371/journal.pone.0019710
Berenjian, A., Mahanama, R., Talbot, A., Biffin, R., Regtop, H., Valtchev, P., et al. (2011). Efficient media for high menaquinone-7 production: response surface methodology approach. N. Biotechnol. 28, 665–672. doi: 10.1016/j.nbt.2011.07.007
Blake, C. J., and Konings, E. J. M. (2019). Committee on food nutrition: fat-soluble vitamins: water-soluble Vitamins. J. AOAC Int. 88, 325–330. doi: 10.1093/jaoac/88.1.325
Blanche, F., Cameron, B., Crouzet, J., Debussche, L., and Battersby, A. R. (1995a). Vitamin B12: how the problem of its biosynthesis was solved. Angewandte Chemie Int. Edition 34, 383–411. doi: 10.1002/chin.199527327
Blanche, F., Cameron, B., Crouzet, J., Debussche, L., and Battersby, A. R. (1995b). Vitamin B12: wie das problem seiner biosynthese gelöst wurde. Angewandte Chemie 107, 421–452. doi: 10.1002/ange.19951070404
Blanche, F., Cameron, B., Crouzet, J., Debussche, L., Levy-Schil, S., and Thibaut, D. (1992). Polypeptides involved in the biosynthesis of cobalamines and/or cobamides, dna sequences coding for these polypeptides, and their preparation and use. WO 1991011518:A1.
Burgess, C., O’Connell-Motherway, M., Sybesma, W., Hugenholtz, J., and van Sinderen, D. (2004). Riboflavin production in Lactococcus lactis: potential for in situ production of vitamin-enriched foods. Appl. Environ. Microbiol. 70, 5769–5777. doi: 10.1128/AEM.70.10.5769-5777.2004
Cai, Y., Xia, M., Dong, H., Qian, Y., Zhang, T., Zhu, B., et al. (2018). Engineering a vitamin B12 high-throughput screening system by riboswitch sensor in Sinorhizobium meliloti. BMC Biotechnol. 18:27. doi: 10.1186/s12896-018-0441-2
Capone, K., and Sentongo, T. (2019). The ABCs of nutrient deficiencies and toxicities. Pediatr. Ann. 48, e434–e440. doi: 10.3928/19382359-20191015-01
Cardinale, S., Tueros, F. G., and Sommer, M. O. A. (2017). Genetic-metabolic coupling for targeted metabolic engineering. Cell Rep. 20, 1029–1037. doi: 10.1016/j.celrep.2017.07.015
Cea, P. A., Araya, G., Vallejos, G., Recabarren, R., and Castro-Fernandez, V. (2020). Characterization of hydroxymethylpyrimidine phosphate kinase from mesophilic and thermophilic bacteria and structural insights into their differential thermal stability. Arch. Biochem. Biophys. 688:108389. doi: 10.1016/j.abb.2020.108389
Chang, H., Wang, C., Wang, P., Zhou, J., and Li, B. (2019). DNA assembly technologies: a review. Sheng Wu Gong Cheng Xue Bao 35, 2215–2226. doi: 10.13345/j.cjb.190273
Chauhan, N., and Poddar, R. (2019). In silico pharmacophore modeling and simulation studies for searching potent antileishmanials targeted against Leishmania donovani nicotinamidase. Comp. Biol. Chem. 83:107150. doi: 10.1016/j.compbiolchem.2019.107150
Chen, J., Duan, P. L., Chen, L. H., Qiu, R. X., Cai, L. G., Hong, Z. L., et al. (2016). Bacillus Subtilis Natto and Method for Producting Protein MK-7. CN 104262129B. Beijing: China National Intellectual Property Administration.
Commichau, F. M., Alzinger, A., Sande, R., Bretzel, W., Reuss, D. R., Dormeyer, M., et al. (2015). Engineering Bacillus subtilis for the conversion of the antimetabolite 4-hydroxy-l-threonine to pyridoxine. Metab. Eng. 29, 196–207. doi: 10.1016/j.ymben.2015.03.007
Cui, S., Lv, X., Wu, Y., Li, J., Du, G., Ledesma-Amaro, R., et al. (2019). Engineering a bifunctional Phr60-Rap60-Spo0A quorum-sensing molecular switch for dynamic fine-tuning of menaquinone-7 synthesis in Bacillus subtilis. ACS Synth. Biol. 8, 1826–1837. doi: 10.1021/acssynbio.9b00140
Cui, S., Xia, H., Chen, T., Gu, Y., Lv, X., Liu, Y., et al. (2020). Cell membrane and electron transfer engineering for improved synthesis of menaquinone-7 in Bacillus subtilis. iScience 23:100918. doi: 10.1016/j.isci.2020.100918
Dmytruk, K., Lyzak, O., Yatsyshyn, V., Kluz, M., Sibirny, V., Puchalski, C., et al. (2014). Construction and fed-batch cultivation of Candida famata with enhanced riboflavin production. J. Biotechnol. 172, 11–17. doi: 10.1016/j.jbiotec.2013.12.005
Dmytruk, K. V., Yatsyshyn, V. Y., Sybirna, N. O., Fedorovych, D. V., and Sibirny, A. A. (2011). Metabolic engineering and classic selection of the yeast Candida famata (Candida flareri) for construction of strains with enhanced riboflavin production. Metab. Eng. 13, 82–88. doi: 10.1016/j.ymben.2010.10.005
Dorrestein, P. C., Zhai, H., Mclafferty, F. W., and Begley, T. P. (2004). The biosynthesis of the thiazole phosphate moiety of thiamin. Chem. Biol. 11, 1373–1381. doi: 10.1016/j.chembiol.2004.08.009
Downs, D. M. (1992). Evidence for a new, oxygen-regulated biosynthetic pathway for the pyrimidine moiety of thiamine in Salmonella typhimurium. J. Bacteriol. 174, 1515–1521. doi: 10.1128/jb.174.5.1515-1521.1992
Downs, D. M., and Petersen, L. (1994). apbA, a new genetic locus involved in thiamine biosynthesis in Salmonella typhimurium. J. Bacteriol. 176, 4858–4864. doi: 10.1007/BF02182164
Downs, D. M., and Roth, J. R. (1991). Synthesis of thiamine in Salmonella typhimurium independent of the purF function. J. Bacteriol. 173, 6597–6604. doi: 10.1128/jb.173.20.6597-6604.1991
Durmaz, Y. (2007). Vitamin E (α-tocopherol) production by the marine microalgae Nannochloropsis oculata (Eustigmatophyceae) in nitrogen limitation. Aquaculture 272, 717–722. doi: 10.1016/j.aquaculture.2007.07.213
Eliot, A. C., and Kirsch, J. F. (2004). Pyridoxal phosphate enzymes: mechanistic, structural, and evolutionary considerations. Annu. Rev. Biochem. 73, 383–415. doi: 10.1146/annurev.biochem.73.011303.074021
Fang, H., Kang, J., and Zhang, D. (2017). Microbial production of vitamin B12: a review and future perspectives. Microbial Cell Factories 16:15. doi: 10.1186/s12934-017-0631-y
Fang, H., Li, D., Kang, J., Jiang, P., Sun, J., and Zhang, D. (2018). Metabolic engineering of Escherichia coli for de novo biosynthesis of vitamin B12. Nat. Commun. 9:4917. doi: 10.1038/s41467-018-07412-6
Fischer, M., and Bacher, A. (2005). Biosynthesis of flavocoenzymes. Nat. Prod. Rep. 22, 324–350. doi: 10.1039/b210142b
Glasscock, C. J., Biggs, B. W., Lazar, J. T., Arnold, J. H., Burdette, L. A., Valdes, A., et al. (2021). Dynamic control of gene expression with riboregulated switchable feedback promoters. ACS Synth Biol. doi: 10.1021/acssynbio.1c00015 Online ahead of print.
Grimm, P., Risse, J. M., Cholewa, D., Muller, J. M., Beshay, U., Friehs, K., et al. (2015). Applicability of Euglena gracilis for biorefineries demonstrated by the production of α-tocopherol and paramylon followed by anaerobic digestion. J. Biotechnol. 215, 72–79. doi: 10.1016/j.jbiotec.2015.04.004
Henrik, D. (1973). CLIV. The antihaemorrhagic vitamin of the chick. Nutrition Rev. 31:121. doi: 10.1111/j.1753-4887.1973.tb05050.x
Hohmann, H. P., Dijl, J. M. V., Krishnappa, L., and Prágai, Z. (2016). Host Organisms: Bacillus subtilis. Hoboken, NJ: John Wiley & Sons, Ltd.
Holvik, K., Fryland, L., Haugen, M., Henjum, S., and Parr, C. L. (2019). Assessment of dietary intake of vitamin K and maximum limits for vitamin K in food supplements. Eur. J. Nutrition Food Safety 9, 96–98. doi: 10.9734/ejnfs/2019/v9i230044
Hoshino, T., Ichikawa, K., and Nagahashi, Y. (2007). Microorganism and Process for Preparing Vitamin B6. Washington, DC: U.S. Patent and Trademark Office. U.S. Patent No 20060127992 A1.
Hoshino, T., Ichikawa, K., and Tazoe, M. (2004). Recombinant Microorganism for the Production. Washington, DC: U.S. Patent and Trademark Office. U.S. Patent No EP 1 543 139 B1.
Huser, A. T., Chassagnole, C., Lindley, N. D., Merkamm, M., Guyonvarch, A., Elisakova, V., et al. (2005). Rational design of a Corynebacterium glutamicum pantothenate production strain and its characterization by metabolic flux analysis and genome-wide transcriptional profiling. Appl. Environ. Microbiol. 71, 3255–3268. doi: 10.1128/AEM.71.6.3255-3268.2005
Hwang, S., Cordova, B., Abdo, M., Pfeiffer, F., and Maupin-Furlow, J. A. (2017). ThiN as a versatile domain of transcriptional repressors and catalytic enzymes of thiamine biosynthesis. J. Bacteriol. 199:JB.00810-16. doi: 10.1128/JB.00810-16
Ifuku, O., Koga, N., Haze, S., Kishimoto, J., Arai, T., and Wachi, Y. (1995). Molecular analysis of growth inhibition caused by overexpression of the biotin operon in Escherichia coli. Biosci. Biotechnol. Biochem. 59, 184–189. doi: 10.1271/bbb.59.184
Jagerstad, M., and Jastrebova, J. (2013). Occurrence, stability, and determination of formyl folates in foods. J. Agric. Food Chem. 61, 9758–9768. doi: 10.1021/jf4028427
Jurgenson, C. T., Begley, T. P., and Ealick, S. E. (2009). The structural and biochemical foundations of thiamin biosynthesis. Annu. Rev. Biochem. 78, 569–603. doi: 10.1146/annurev.biochem.78.072407.102340
Kaiser, S., Di, M. P., Murphy, M. E., and Sies, H. (1990). Physical and chemical scavenging of singlet molecular oxygen by tocopherols. Arch. Biochem. Biophys. 277, 101–108. doi: 10.1016/0003-9861(90)90556-E
Kawahori, K., Kondo, Y., Yuan, X., Kawasaki, Y., and Hashimoto, K. (2020). Ascorbic acid during the suckling period is required for proper DNA demethylation in the liver. Sci. Rep. 10:21228. doi: 10.1038/s41598-020-77962-7
Kawata, A., Murakami, Y., Suzuki, S., and Fujisawa, S. (2018). Anti-inflammatory activity of β-carotene, lycopene and tri-n-butylborane, a scavenger of reactive oxygen species. In Vivo 32, 255–264. doi: 10.21873/invivo.11232
Kim, S. T., Huh, W. K., Kim, J. Y., Hwang, S. W., and Kang, S. O. (1996). D-Arabinose dehydrogenase and biosynthesis of erythroascorbic acid in Candida albicans. Biochim. Biophys. Acta 1297, 1–8. doi: 10.1016/0167-4838(96)00077-5
Kim, S. T., Huh, W. K., Lee, B. H., and Kang, S. O. (1998). D-arabinose dehydrogenase and its gene from Saccharomyces cerevisiae. Biochim. Biophys. Acta 1429, 29–39. doi: 10.1016/S0167-4838(98)00217-9
Kitago, M., Kase, Y., Iwata, Y., Suwa, Y., Tsuchiya, H., Hanata, N., et al. (2020). Deteriorating anemia in an 86-year-old man was improved by prednisolone. Geriatr. Gerontol. Int. 20, 1091–1092. doi: 10.1111/ggi.14024
Koo, J., Yang, J., and Park, H. (2020). Cell-free systems: recent advances and future outlook. Biotechnol. Bioprocess Eng. 25, 955–961. doi: 10.1007/s12257-020-0013-x
Larroude, M., Celinska, E., Back, A., Thomas, S., Nicaud, J. M., and Ledesma-Amaro, R. (2018). A synthetic biology approach to transform Yarrowia lipolytica into a competitive biotechnological producer of β-carotene. Biotechnol. Bioeng. 115, 464–472. doi: 10.1002/bit.26473
Leonardi, R., and Jackowski, S. (2007). Biosynthesis of pantothenic acid and coenzyme a. EcoSal Plus 2:10.1128/ecosallus.3.6.3.4. doi: 10.1128/ecosalplus.3.6.3.4
Li, K. T., Liu, D. H., Chu, J., Wang, Y. H., Zhuang, Y. P., and Zhang, S. L. (2008). An effective and simplified pH-stat control strategy for the industrial fermentation of vitamin B(12) by Pseudomonas denitrificans. Bioprocess Biosyst. Eng. 31, 605–610. doi: 10.1007/s00449-008-0209-5
Lin, S., and Cronan, J. E. (2011). Closing in on complete pathways of biotin biosynthesis. Mol. Biosyst. 7, 1811–1821. doi: 10.1039/c1mb05022b
Liu, L., Xu, W., Jia, H., and Wu, J. (2011). A novel and effective multi-constrained QoS routing scheme in WMNs. Front. Electrical Electronic Eng. China 6:507–514. doi: 10.1007/s11460-011-0118-2
Liu, S., Dicker, K. T., and Jia, X. (2015). Modular and orthogonal synthesis of hybrid polymers and networks. Chem. Commun. (Camb) 51, 5218–5237. doi: 10.1039/c4cc09568e
Lucock, M. (2000). Folic acid: nutritional biochemistry, molecular biology, and role in disease processes. Mol. Genet. Metab. 71, 121–138. doi: 10.1006/mgme.2000.3027
Ma, Q., Bi, Y. H., Wang, E. X., Zhai, B. B., Dong, X. T., Qiao, B., et al. (2019). Integrated proteomic and metabolomic analysis of a reconstructed three-species microbial consortium for one-step fermentation of 2-keto-L-gulonic acid, the precursor of vitamin C. J. Ind. Microbiol. Biotechnol. 46, 21–31. doi: 10.1007/s10295-018-2096-3
Mahanama, R., Berenjian, A., Valtchev, P., Talbot, A., Biffin, R., Regtop, H., et al. (2011). Enhanced production of menaquinone 7 via solid substrate fermentation from Bacillus subtilis. Int. J. Food Eng. 7, 1–2. doi: 10.2202/1556-3758.2314
Marucci, L., Barberis, M., Karr, J., Ray, O., Race, P. R., de Souza Andrade, M., et al. (2020). Computer-aided whole-cell design: taking a holistic approach by integrating synthetic with systems biology. Front. Bioeng. Biotechnol. 8:942. doi: 10.3389/fbioe.2020.00942
Moriguchi, S., and Muraga, M. (2000). Vitamin E and immunity. Vitamins Hormones-advances Res. Appl. 59, 305–336.
Morishita, T., Tamura, N., Makino, T., and Kudo, S. (1999). Production of menaquinones by lactic acid bacteria. J. Dairy Sci. 82, 0–1903. doi: 10.3168/jds.S0022-0302(99)75424-X
Muñoz, P., and Munné-Bosch, S. (2019). Vitamin E in plants: biosynthesis, transport, and function. Trends Plant Sci. 24, 1040–1051. doi: 10.1016/j.tplants.2019.08.006
Myszczyszyn, A., Krajewski, R., Ostapów, M., and Hirnle, L. (2019). Folic acid – role in the body, recommendations and clinical significance. Pielegniarstwo XXI wieku / Nursing in the 21st Century 18, 50–59. doi: 10.2478/pielxxiw-2019-0007
Nie, G., Yang, X., Liu, H., Wang, L., and Gong, G. (2013). N+ ion beam implantation of tannase-producing Aspergillus niger and optimization of its process parameters under submerged fermentation. Annals Microbiol. 63, 279–287. doi: 10.1007/s13213-012-0471-2
Osman, D., Cooke, A., Young, T. R., Deery, E., and Warren, M. J. (2021). The requirement for cobalt in vitamin B12: a paradigm for protein metalation. Biochimica et Biophysica Acta (BBA) - Mol. Cell Res. 1868:118896. doi: 10.1016/j.bbamcr.2020.118896
Paciolla, C., Fortunato, S., Dipierro, N., Paradiso, A., and Pinto, M. C. D. (2019). Vitamin C in plants: from functions to biofortification. Antioxidants 8:519. doi: 10.3390/antiox8110519
Palmer, L. D., and Downs, D. M. (2013). The thiamine biosynthetic enzyme ThiC catalyzes multiple turnovers and is inhibited by S-adenosylmethionine (AdoMet) metabolites. J. Biol. Chem. 288, 30693–30699. doi: 10.1074/jbc.M113.500280
Papoutsis, K., Grasso, S., Menon, A., Brunton, N. P., Lyng, J. G., Jacquier, J.-C., et al. (2020). Recovery of ergosterol and vitamin D2 from mushroom waste-potential valorization by food and pharmaceutical industries. Trends Food Sci. Technol. 99, 351–366. doi: 10.1016/j.tifs.2020.03.005
Rao, Y. M., and Sureshkumar, G. K. (2000). Direct biosynthesis of ascorbic acid from glucose by Xanthomonas campestris through induced free-radicals. Biotechnol. Lett. 22, 407–411. doi: 10.1023/B:DOBS.0000033277.54135.71
Sachsenhauser, V., Deng, X., Kim, H. H., Jankovic, M., and Bardwell, J. C. A. (2020). Yeast tripartite biosensors sensitive to protein stability and aggregation propensity. ACS Chem. Biol. 15, 1078–1088. doi: 10.1021/acschembio.0c00083
Sahm, H., and Eggeling, L. (1999). D-Pantothenate synthesis in Corynebacterium glutamicum and use of panBC and genes encoding L-valine synthesis for D-Pantothenate overproduction. Appl. Environ. Microbiol. 65, 1973–1979. doi: 10.1016/S0027-5107(99)00041-X
Sano, M., Ernesto, C., Thomas, R. G., Klauber, M. R., Schafer, K., Grundman, M., et al. (1997). A controlled trial of selegiline, alpha-tocopherol, or both as treatment for Alzheimer’s disease. New England J. Med. 336, 1216–1222. doi: 10.1056/nejm199704243361704
Santos-Merino, M., Singh, A., and Ducat, D. (2019). New applications of synthetic biology tools for cyanobacterial metabolic engineering. Front. Bioeng. Biotechnol. 7:33. doi: 10.3389/fbioe.2019.00033
Sato, T., Yamada, Y., Ohtani, Y., Mitsui, N., Murasawa, H., and Araki, S. (2001). Efficient production of menaquinone (vitamin K2) by a menadione-resistant mutant of Bacillus subtilis. J. Ind. Microbiol. Biotechnol. 26, 115–120. doi: 10.1038/sj/jim/7000089
Sauer, M., Branduardi, P., Valli, M., and Porro, D. (2004). Production of L-ascorbic acid by metabolically engineered Saccharomyces cerevisiae and Zygosaccharomyces bailii. Appl. Environ. Microbiol. 70, 6086–6091. doi: 10.1128/AEM.70.10.6086-6091.2004
Schwalfenberg, G. K. (2017). Vitamins K1 and K2: the emerging group of vitamins required for human health. J. Nutr. Metab. 2017:6254836. doi: 10.1155/2017/6254836
Schwechheimer, S. K., Park, E. Y., Revuelta, J. L., Becker, J., and Wittmann, C. (2016). Biotechnology of riboflavin. Appl. Microbiol. Biotechnol. 100, 2107–2119. doi: 10.1007/s00253-015-7256-z
Schwarzhans, J.-P., Cholewa, D., Grimm, P., Beshay, U., Risse, J.-M., Friehs, K., et al. (2015). Dependency of the fatty acid composition of Euglena gracilis on growth phase and culture conditions. J. Appl. Phycol. 27, 1389–1399. doi: 10.1007/s10811-014-0458-4
Schyns, G., Potot, S., Geng, Y., Barbosa, T. M., Henriques, A., and Perkins, J. B. (2005). Isolation and characterization of new thiamine-deregulated mutants of Bacillus subtilis. J. Bacteriol. 187, 8127–8136. doi: 10.1128/JB.187.23.8127-8136.2005
Selvam, S., Ramaian Santhaseela, A., Ganesan, D., Rajasekaran, S., and Jayavelu, T. (2019). Autophagy inhibition by biotin elicits endoplasmic reticulum stress to differentially regulate adipocyte lipid and protein synthesis. Cell Stress Chaperones 24, 343–350. doi: 10.1007/s12192-018-00967-9
Serrano-Amatriain, C., Ledesma-Amaro, R., Lopez-Nicolas, R., Ros, G., Jimenez, A., and Revuelta, J. L. (2016). Folic acid production by engineered Ashbya gossypii. Metab. Eng. 38, 473–482. doi: 10.1016/j.ymben.2016.10.011
Sharma, V., Meganathan, R., and Hudspeth, M. E. (1993). Menaquinone (vitamin K2) biosynthesis: cloning, nucleotide sequence, and expression of the menC gene from Escherichia coli. J. Bacteriol. 175, 4917–4921. doi: 10.1128/jb.175.15.4917-4921.1993
Shen, B., Zhou, P., Jiao, X., Yao, Z., Ye, L., and Yu, H. (2020). Fermentative production of Vitamin E tocotrienols in Saccharomyces cerevisiae under cold-shock-triggered temperature control. Nat. Commun. 11:5155. doi: 10.1038/s41467-020-18958-9
Shi, Z., Zhen, S., Wittert, G. A., Yuan, B., Zuo, H., and Taylor, A. W. (2014). Inadequate riboflavin intake and anemia risk in a Chinese population: fve-year follow up of the Jiangsu Nutrition Study. PLoS One 9:e88862. doi: 10.1371/journal.pone.0088862
Sivakumar, G., Jeong, K., and Lay, J. O. Jr. (2014). Biomass and RRR-α-tocopherol production in Stichococcus bacillaris strain siva2011 in a balloon bioreactor. Microb. Cell Fact. 13:79. doi: 10.1186/1475-2859-13-79
Song, J., Liu, H., Wang, L., Dai, J., and Zheng, Z. (2014). Enhanced production of vitamin K2 from Bacillus subtilis (natto) by mutation and optimization of the fermentation medium. Braz. Arch. Biol. Technol. 57, 606–612. doi: 10.1590/S1516-8913201402126
Streit, W. R., and Entcheva, P. (2003). Biotin in microbes, the genes involved in its biosynthesis, its biochemical role and perspectives for biotechnological production. Appl. Microbiol. Biotechnol. 61, 21–31. doi: 10.1007/s00253-002-1186-2
Sugimoto, T., Kanamasa, S., Kato, T., and Park, E. Y. (2009). Importance of malate synthase in the glyoxylate cycle of Ashbya gossypii for the efficient production of riboflavin. Appl. Microbiol. Biotechnol. 83, 529–539. doi: 10.1007/s00253-009-1972-1
Sugisawa, T., Miyazaki, T., and Hoshino, T. (2005). Microbial production of L-Ascorbic acid from D-Sorbitol, L-Sorbose, L-Gulose, and L-Sorbosone by Ketogulonicigenium vulgare DSM 4025. Biosci. Biotechnol. Biochem. 69, 659–662. doi: 10.1271/bbb.69.659
Suter, P. M. (2020). The B-vitamins. Essential Toxic Trace Elements Vitamins Hum. Health 2020, 217–239 doi: 10.1016/B978-0-12-805378-2.00017-6
Sych, J. M., Lacroix, C., and Stevens, M. J. A. (2016). Vitamin B12 – Physiology, Production and Application. Weinheim: Wiley-VCH Verlag GmbH & Co. KGaA.
Szterk, A., Zmyslowski, A., and Bus, K. (2018). Identification of cis/trans isomers of menaquinone-7 in food as exemplified by dietary supplements. Food Chem. 243, 403–409. doi: 10.1016/j.foodchem.2017.10.001
Taguchi, H., Shibata, T., Duangmanee, C., and Tani, Y. (2014). Menaquinone-4 production by a sulfonamide-resistant mutant of Flavobacterium sp. Agricultural Biol. Chem. 53, 3017–3023. doi: 10.1080/00021369.1989.10869800
Taketomi, H., Soda, K., and Katsui, G. (1983). Results of screening test in tocopherols in microbial realm. Vitamins 57, 133–138.
Tan, T., Zhang, M., and Gao, H. (2003). Ergosterol production by fed-batch fermentation of Saccharomyces cerevisiae. Enzyme Microbial Technol. 33, 366–370. doi: 10.1016/s0141-0229(03)00132-7
Tanaka, T., Tateno, Y., and Gojobori, T. (2005). Evolution of vitamin B6 (pyridoxine) metabolism by gain and loss of genes. Mol. Biol. Evol. 22, 243–250. doi: 10.1093/molbev/msi011
Tani, Y., and Tsumura, H. (1989). Screening for tocopherol-producing microorganisms and α-tocopherol production by Euglena gracilis Z. Agricultural Biol. Chem. 53, 305–312. doi: 10.1271/bbb1961.53.305
Tannous, C., Booz, G. W., Altara, R., Muhieddine, D. H., and Zouein, F. A. (2020). Nicotinamide adenine dinucleotide: biosynthesis, consumption, and therapeutic role in cardiac diseases. Acta Physiol. 231:e13551. doi: 10.1111/apha.13551
Timoshnikov, V. A., Kobzeva, T. V., Polyakov, N. E., and Kontoghiorghes, G. J. (2020). Redox interactions of vitamin C and iron: inhibition of the pro-oxidant activity by deferiprone. Int. J. Mol. Sci. 21:3967. doi: 10.3390/ijms21113967
Tokui, M., Kubodera, T., Gomi, K., Yamashita, N., and Nishimura, A. (2011). Construction of a thiamine pyrophosphate high-producing strain of Aspergillus oryzae by overexpression of three genes involved in thiamine biosynthesis. J. Biosci. Bioeng. 111, 388–390. doi: 10.1016/j.jbiosc.2010.12.011
Van Arsdell, S. W., Perkins, J. B., Yocum, R. R., Luan, L., Howitt, C. L., Chatterjee, N. P., et al. (2005). Removing a bottleneck in the Bacillus subtilis biotin pathway: BioA utilizes lysine rather than S-adenosylmethionine as the amino donor in the KAPA-to-DAPA reaction. Biotechnol. Bioeng. 91, 75–83. doi: 10.1002/bit.20488
Vandamme, E. J., and Revuelta, J. L. (2016a). “Industrial fermentation of vitamin C,” in Industrial Biotechnology of Vitamins, Biopigments, and Antioxidants, eds E. J. Vandamme and J. L. Revuelta (Weinheim: Wiley-VCH Verlag GmbH & Co. KGaA), 161–192. doi: 10.1002/9783527681754.ch7
Vandamme, E. J., and Revuelta, J. L. (2016b). “Vitamins, biopigments, antioxidants and related compounds: a historical, physiological and (bio)technological perspective,” in Industrial Biotechnology of Vitamins, Biopigments, and Antioxidants, eds E. J. Vandamme and J. L. Revuelta (Weinheim: Wiley-VCH Verlag GmbH & Co. KGaA).
Wang, Z., Liu, Z., Cui, W., and Zhou, Z. (2017). Establishment of bioprocess for synthesis of nicotinamide by recombinant Escherichia coli expressing high-molecular-mass nitrile hydratase. Appl. Biochem. Biotechnol. 182, 1458–1466. doi: 10.1007/s12010-017-2410-y
Wise, L. A., Wesselink, A. K., Bethea, T. N., Brasky, T. M., Wegienka, G., Harmon, Q., et al. (2021). Intake of lycopene and other carotenoids and incidence of uterine leiomyomata: a prospective ultrasound study. J. Acad. Nutr. Diet. 121, 92–104. doi: 10.1016/j.jand.2020.08.013
Wu, W.-J. (2011). Isolation and identification of Bacillus amyloliquefaciens BY01 with high productivity of menaquinone for Cheonggukjang production. J. Korean Soc. Appl. Biol. Chem. 54, 783–789. doi: 10.3839/jksabc.2011.118
Xia, W., Chen, W., Peng, W. F., and Li, K. T. (2015). Industrial vitamin B12 production by Pseudomonas denitrificans using maltose syrup and corn steep liquor as the cost-effective fermentation substrates. Bioprocess Biosyst. Eng. 38, 1065–1073. doi: 10.1007/s00449-014-1348-5
Yang, Y., Li, R., Hui, J., Li, L., and Zheng, X. (2021). β-Carotene attenuates LPS-induced rat intestinal inflammation via modulating autophagy and regulating the JAK2/STAT3 and JNK/p38 MAPK signaling pathways. J. Food Biochem. 45:e13544. doi: 10.1111/jfbc.13544
Yasutake, Y., Nishioka, T., Imoto, N., and Tamura, T. (2013). A single mutation at the ferredoxin binding site of P450 Vdh enables efficient biocatalytic production of 25-hydroxyvitamin D(3). Chembiochem 14, 2284–2291. doi: 10.1002/cbic.201300386
Yoon, S. H., Lee, S. H., Das, A., Ryu, H. K., Jang, H. J., Kim, J. Y., et al. (2009). Combinatorial expression of bacterial whole mevalonate pathway for the production of β-carotene in E. coli. J. Biotechnol. 140, 218–226. doi: 10.1016/j.jbiotec.2009.01.008
Yuan, P., Cui, S., Liu, Y., Li, J., and Liu, L. (2020). Metabolic engineering for the production of fat-soluble vitamins: advances and perspectives. Appl. Microbiol. Biotechnol. 104, 935–951. doi: 10.1007/s00253-019-10157-x
Zeng, W., Guo, L., Xu, S., Chen, J., and Zhou, J. (2020). High-throughput screening technology in industrial biotechnology. Trends Biotechnol. 38, 888–906. doi: 10.1016/j.tibtech.2020.01.001
Zeng, Z., Han, N., Liu, C., Buerte, B., Zhou, C., Chen, J., et al. (2020). Functional dissection of HGGT and HPT in barley vitamin E biosynthesis via CRISPR/Cas9-enabled genome editing. Annals Bot. 126, 929–942. doi: 10.1093/aob/mcaa115
Zhang, Y., and Showalter, A. M. (2020). CRISPR/Cas9 genome editing technology: a valuable tool for understanding plant cell wall biosynthesis and function. Front. Plant Sci. 11:589517. doi: 10.3389/fpls.2020.589517
Zhao, J., Li, Q., Sun, T., Zhu, X., Xu, H., Tang, J., et al. (2013). Engineering central metabolic modules of Escherichia coli for improving β-carotene production. Metab. Eng. 17, 42–50. doi: 10.1016/j.ymben.2013.02.002
Keywords: vitamins, metabolic engineering, microbial cell factory, chemical synthesis, biosynthesis
Citation: Wang Y, Liu L, Jin Z and Zhang D (2021) Microbial Cell Factories for Green Production of Vitamins. Front. Bioeng. Biotechnol. 9:661562. doi: 10.3389/fbioe.2021.661562
Received: 31 January 2021; Accepted: 12 May 2021;
Published: 17 June 2021.
Edited by:
Dipesh Dhakal, University of Florida, United StatesReviewed by:
Ana Margarida Goncalves Carvalho Dias, New University of Lisbon, PortugalAngel León-Buitimea, Universidad Autonoma de Nuevo Leon, Mexico
Copyright © 2021 Wang, Liu, Jin and Zhang. This is an open-access article distributed under the terms of the Creative Commons Attribution License (CC BY). The use, distribution or reproduction in other forums is permitted, provided the original author(s) and the copyright owner(s) are credited and that the original publication in this journal is cited, in accordance with accepted academic practice. No use, distribution or reproduction is permitted which does not comply with these terms.
*Correspondence: Zhaoxia Jin, amluengyMDE4QDE2My5jb20=; Dawei Zhang, emhhbmdfZHdAdGliLmNhcy5jbg==
†These authors have contributed equally to this work