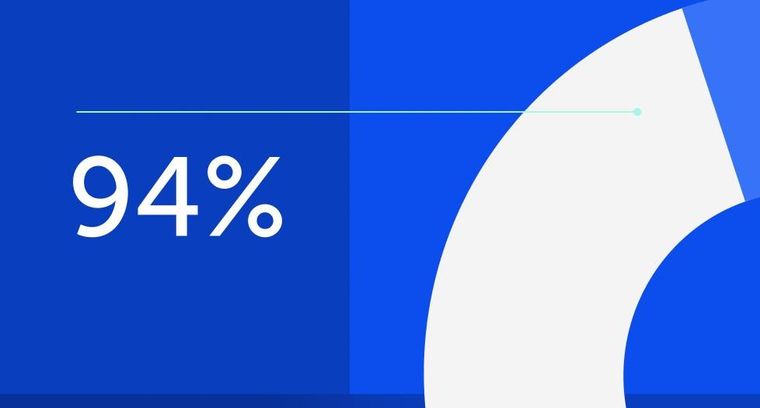
94% of researchers rate our articles as excellent or good
Learn more about the work of our research integrity team to safeguard the quality of each article we publish.
Find out more
REVIEW article
Front. Bioeng. Biotechnol., 12 January 2021
Sec. Biomaterials
Volume 8 - 2020 | https://doi.org/10.3389/fbioe.2020.631616
This article is part of the Research TopicAdvanced Materials for the Restoration and Reconstruction of Dental FunctionsView all 19 articles
In recent years, polyetheretherketone (PEEK) has been increasingly employed as an implant material in clinical applications. Although PEEK is biocompatible, chemically stable, and radiolucent and has an elastic modulus similar to that of natural bone, it suffers from poor integration with surrounding bone tissue after implantation. To improve the bioactivity of PEEK, numerous strategies for functionalizing the PEEK surface and changing the PEEK structure have been proposed. Inspired by the components, structure, and function of bone tissue, this review discusses strategies to enhance the biocompatibility of PEEK implants and provides direction for fabricating multifunctional implants in the future.
Bone defects caused by aging, trauma, disease, congenital abnormalities, and surgical resections are widespread all over the world. It's reported that the number of fractures is estimated to rise from 2.1 million to over 3 million during 2005–2025 in the United States (Quarto and Giannoni, 2016). In the past decades, numerous biomaterials have been employed to repair bone defects. Conventional implants used in bone tissue engineering are commonly made of titanium (Ti) or its alloys due to their good biocompatibility, chemical stability, and mechanical properties. However, these materials suffer from limitations such as the release of harmful metal ions, osteolysis, allergenic effects, and radiopaqueness (Niki et al., 2001; Fage et al., 2016). In particular, Ti possesses an elastic modulus of more than 100 GPa, which contributes to stress shielding and the resorption of surrounding bone (Huiskes et al., 1992). To overcome these drawbacks and reduce negative post-implantation biological reactions, polyetheretherketone (PEEK) and PEEK-based compounds have emerged as viable alternatives to Ti and its alloys.
PEEK, which is a dominant member of the polyaryletherketone (PAEK) family, was first synthesized by British scientists via nucleophilic displacement in 1972 (Eschbach, 2000). In the late 1990s, PEEK was approved by the U.S. Food and Drug Administration as an implantable biomaterial (Kurtz and Devine, 2007). Since then, PEEK has been extensively employed in the fields of orthopedics, trauma, spinal and dental implants. Another important member of PAEK named polyetherketoneketone (PEKK), has also been extensively studied as a promising orthopedic implant recently (Wang et al., 2017; Yuan et al., 2018). PAEK has numerous beneficial characteristics such as non-toxicity, excellent mechanical properties, natural radiolucency, and good chemical and sterilization resistance (Wenz et al., 1990; Katzer et al., 2002; Godara et al., 2007). However, smooth PEEK implants can lead to poor osseointegration characterized by fibrous encapsulation, potentially leading to clinical failure (Walsh et al., 2015). This phenomenon can be explained by the hydrophobic and chemically inert properties of PEEK. Consequently, considerable efforts should focus on modifying the surfaces of PEEK implants. It is expected that the reinforced PEEK implants can result in enhanced ingrowth of osteoblasts (osteoconduction), direct contact with surrounding bone (osseointegration) and stimulation of immature cells into osteogenic cells (osteoinduction).
This review presents an overview of the properties of PEEK and multiple strategies for the modification of PEEK implants inspired by the constituents, structure, and function of human bone (Figure 1). Subsequently, we discuss several aspects in need of further exploration and highlight future directions in the field of multifunctional implants.
Bulk properties are determined by the atomic composition and structure of a material (Binyamin et al., 2006), including the mechanical, chemical, thermal, and radiation characteristics, which are fundamental to the performance of an implant. PEEK is a semi-crystalline linear polycyclic aromatic thermoplastic that has an aromatic molecular backbone interconnected by ketone and ether functional groups between the aryl rings (Kurtz and Devine, 2007). Regarding its mechanical behavior, the tensile modulus, bending modulus, and compressive modulus of PEEK are ~3.8, 3.6, and 2.8 GPa, respectively (Han et al., 2019b). At room temperature, PEEK is chemically stable in all conventional solvents except for 98% sulfuric acid (Ha et al., 1997). At high temperatures, PEEK maintains stability and displays resistance to damage from chemical components and radiation. The glass transition temperature of PEEK is ~143°C, whereas the crystalline melt transition occurs at around 343°C (Kurtz and Devine, 2007). In addition, gamma irradiation can be used to sterilize PEEK in air at doses of 25–40 kGy in clinical practice (Kurtz and Devine, 2007).
The interactions that occur at the interface of a material define its surface properties (Binyamin et al., 2006). For PEEK implants, one of the key surface properties is biocompatibility. A previous study showed that PEEK has no cytotoxic or mutagenic effects (Katzer et al., 2002). Moreover, human gingival fibroblasts (HGFs) and osteoblasts adhered on the surface of PEEK displayed increased proliferation and viability compared to those on Ti, indicating that PEEK exhibits desirable performance with both soft and hard tissues (da Cruz et al., 2019). Other surface properties, including surface topography, chemistry, energy, and wettability, should also be considered. Surface topography and chemistry can influence the tissue response to an implant by altering protein adsorption and subsequent cell adhesion and differentiation. The surface energy has been confirmed to affect cell maturation, differentiation, and osseointegration (Kilpadi and Lemons, 1994; Zhao et al., 2005). Surfaces with higher surface energy exhibit more rapid cell activation and differentiation than those with lower surface energy. Unmodified PEEK with a water contact angle of 80–90° is inherently hydrophobic and bioinert. When the surface of an implant becomes hydrophilic, plasma proteins and cells are more likely to adhere to it (Jimbo et al., 2010). Surface wettability is related to surface roughness, Elawadly et al. proposed that the water contact angle of PEEK-based materials was below 90° when the surface average roughness (Ra) value was either <1.0 or> 1.7 μm (Elawadly et al., 2017).
Human bone is mainly composed of inorganic and organic phases. Calcium phosphate (CaP), which is the primary inorganic constituent of human bone, has been extensively studied in bone tissue engineering owing to its superb biocompatibility, osseointegration, osteoconduction, and osteoinductivity (Eliaz and Metoki, 2017). CaP can enhance the local concentrations of calcium and phosphate ions and form apatite on the surface of the substrate. Meanwhile, CaP can adsorb extracellular matrix (ECM) proteins and activate osteoblastic differentiation via cell–ECM interactions (Eliaz and Metoki, 2017). CaP coatings have been shown to remarkably enhance the proliferation of MC3T3-E1 cells and apatite-forming ability in simulated body fluid (SBF) (Jabbari et al., 2018). In another study, surface functionalization with a combination of phosphate and calcium remarkably promoted the osteogenic activity of rabbit bone marrow-derived mesenchymal stem cells in vitro and the osseointegration ability in vivo (Sunarso et al., 2019). It is worth noting that amorphous calcium phosphate can be fabricated on the PEEK surface within 1 day, and strong adhesion with PEEK can be obtained using a novel PrA treatment (involving three steps: H2SO4 treatment, O2 plasma treatment, and alkaline SBF treatment) (Yabutsuka et al., 2017, 2018). Further study revealed that the PrA treatment resulted in zero cytotoxicity and produced excellent bone-bonding properties (Masamoto et al., 2019). However, the use of CaP-based materials in high-load-bearing areas is limited due to the poor mechanical properties of CaP (Ambard and Mueninghoff, 2006).
Hydroxyapatite (HA) is widely used in bone regeneration since its composition is close to that of natural bone (Yoshikawa and Myoui, 2005). HA is the most stable and least soluble CaP ceramic and has the molecular formula Ca10(PO4)6 (OH)2, in which the Ca/P ratio is approximately 1.67 (Klein et al., 1990; He et al., 2003). HA is considered to be osteoconductive but not osteoinductive (Samavedi et al., 2013). HA can be used as either a coating or a reinforcement component to produce bioactive PEEK composites. Coating PEEK with HA can impart PEEK with a rough and hydrophilic surface, which is beneficial for cell growth (Figure 2A; Lee et al., 2013). Numerous treatments including cold spraying, plasma spraying, ion beam-assisted deposition, and electrophoretic co-deposition have been adopted to deposit HA coatings (Lee et al., 2013; Suska et al., 2014; Durham et al., 2016; Baştan et al., 2018). Unfortunately, plasma-sprayed HA coatings suffer from insufficient adhesion strength to PEEK and tend to delaminate, resulting in the infiltration of multinucleated giant cells (Reigstad et al., 2011). In addition, the detachment of thick, layered apatite coatings was found to cause severe inflammatory response and bone resorption (Røkkum et al., 2003). To suppress such negative responses, a thin nanosized HA (nHA) layer was applied to the PEEK surface. In vivo studies indicated that this nHA coating significantly improved the removal torque, early bone integration, and osteoconductive properties of PEEK implants (Barkarmo et al., 2013; Johansson et al., 2014, 2015, 2016, 2018). Chemical modifications such as the grafting of phosphonate groups have also performed positive effects on the adhesion strength between HA and PEEK implants (Mahjoubi et al., 2017).
Figure 2. Examples and biological effects of modifications inspired by the components of bone. (A) Micro-CT images of new bone formed on HA-coated PEEK and on uncoated PEEK at 4 and 8 weeks after implantation. (B) Fluorescence micrographs of actin (red) and nucleus (blue) in rBMSCs grown on PEEK, PEEK-MeHA, and PEEK-MeHA-TiO2 for 24 h. (C) Immunofluorescence analysis of actin (red), collagen I (green), and nucleus (blue) in rBMSCs grown on PEEK-MeHA and PEEK-MeHA-TiO2 for 7 days. (D) HUVEC tube formation ability in extracts of sulfonated PEEK (sPEEK), sPEEK-HA, sPEEK-Ni, and sPEEK-Ni-HA at 6 and 18 h. Reproduced with permission from Dong et al. (2020). Copyright (2020) American Chemical Society.
Subsequent studies attempted to develop bioactive PEEK composites by adding HA, nHA, or ion-substituted HA to PEEK (Wong et al., 2009; Deng et al., 2015b; Liu et al., 2016; Ma and Guo, 2019; Bastan, 2020; Dong et al., 2020). For instance, the addition of strontium-containing HA not only improved the bending modulus of PEEK, it also enhanced apatite formation in SBF and cell-mediated mineralization in vitro (Wong et al., 2009). Moreover, increasing the HA content can improve the elastic modulus, compressive strength, and hardness of the composite but not the tensile strength. A HA/PEEK composite containing 30 wt% HA exhibited a higher elastic modulus and slightly lower tensile strength than that of pure PEEK (Ma and Guo, 2019). To optimize the mechanical properties of HA/PEEK hybrid materials, researchers have focused on incorporating reinforcement components [e.g., carbon fiber (CF) and carbon nanotubes (CNTs)] and identifying the optimal content of HA (Deng et al., 2015b; Liu et al., 2016).
To evaluate the biological properties of PEEK implants, it is crucial to choose biomaterials with good osteogenesis ability for bone repair. Metal ions are strong candidates as coating materials for PEEK implants. Han et al. proposed that electron beam deposition could be used to deposit a Ti layer on the surface of PEEK with strong adhesion and enhanced wettability. Compared to PEEK alone, the Ti-coated PEEK implants exhibited two times greater proliferation and differentiation of MC3T3-E1 cells along with better osseointegration ability (Han et al., 2010). Ti plasma spraying, another coating process, increased the microscale surface area by 40% and accelerated cement line formation in human osteoprogenitor cells (hFOB 1.19) while also increasing the shear strength and bone integration at the implant surface (Walsh et al., 2015; Hickey et al., 2019). Moreover, the addition of Ti increased the compressive strength and stiffness of PEEK (Jung et al., 2016).
Titanium dioxide (TiO2) can also be deposited onto the surface of PEEK using several coating methods. Before sol-gel coating, pretreatment with UV, O2 plasma, or sandblasting can enhance the bonding strength of the coating layer, while post-treatment with HCl can induce apatite formation, which is related to the bone-bonding ability in vivo (Kizuki et al., 2015; Shimizu et al., 2016). Different TiO2 nanostructures such as nanoparticles, nanotubes, and nanofibers can also be employed to improve the cytocompatibility, soft tissue integration, and osseointegration of PEEK implants due to their favorable bioactivity and surface morphology (Figures 2B,C; Wu et al., 2012; Lu et al., 2014; Wang X. et al., 2016; Liu et al., 2018).
Other coating materials such as tantalum, tantalum pentoxide, and niobium pentoxide can not only elevate the surface energy, surface roughness, hydrophilicity, protein absorption, and mechanical properties of PEEK implants, they can also induce positive cellular responses and osteointegration (Lu et al., 2015; Mei et al., 2019; Ge et al., 2020). Silicon-based compounds have been found to reinforce the mechanical properties, surface hydrophilicity, apatite mineralization, and cell and bone tissue responses (Ma et al., 2014; Wen et al., 2016; Monich et al., 2017; Zhang et al., 2018a). Other additives such as phosphonate, amorphous magnesium phosphate, graphene, and diamond-like carbon were found to exert similar effects (Wang et al., 2010; Mahjoubi et al., 2017; Ren et al., 2018; Yan J. H. et al., 2018; Sikder et al., 2020).
The organic phase of bone tissue includes a plethora of growth factors and proteins that play a prominent role in osteogenesis and angiogenesis. Bone morphogenetic protein-2 (BMP-2), which is one of the strongest osteoinductive factors, can initiate the differentiation of mesenchymal stem cells (MSCs) into osteoblasts and promote osteogenesis (Ryoo et al., 2006). Therefore, to improve the biological behavior of PEEK, researchers have attempted to immobilize BMP-2 on PEEK through several methods, including the deposition of nanoporous TiO2 layers, polyelectrolyte multilayer films, and phosphorylated gelatin coatings along with sulfonation treatment (Han et al., 2014; Guillot et al., 2016; Sun et al., 2018; Wu et al., 2018). It was reported that BMP-2-coated PEEK with a dose of 9.3 μg could lead to localized and temporary bone impairment (Guillot et al., 2016). Thereby, further studies should focus on determining the optimal dose of BMP-2 to maximize osteogenic activity. Functionalizing cell-interfacing surfaces with cell-modulatory proteins can also improve the biological properties of the implant. For example, the ECM protein tropoelastin has been shown to promote the biological behavior of human osteoblast-like osteosarcoma cells (SAOS-2) on PEEK via plasma immersion ion implantation (PIII) treatment (Wakelin et al., 2018). Adiponectin (APN), which is adipocyte-secreted adipokine, has been confirmed to increase the osteogenic ability in vitro and osseointegration in vivo of sPEEK implants (Wang et al., 2019; Deng et al., 2020). Out of these proteins, bone forming peptide (BFP) can also be utilized to improve the osteogenic differentiation and maturation of MG-63 cells (Wang et al., 2018).
In addition to the aforementioned osteoinductive agents, angiogenic growth factors such as vascular endothelial growth factor (VEGF), transforming growth factor-β, placental growth factor, and fibroblast growth factor can regulate angiogenesis, which involves endothelial cell proliferation, migration, and tube formation (Stegen et al., 2015). However, the short half-lives and complicated structures of these growth factors make them easy to degrade and deactivate. Alternatively, nickel hydroxide nanoparticles immobilized on the micro-/nanostructured surfaces of PEEK implants can facilitate the migration, tube formation, and angiogenic gene expression of human umbilical vein endothelial cells (HUVECs; Figure 2D; Dong et al., 2020). Apart from applying these osteogenic and angiogenic organic constituents, a recent study provided a novel direction for future research on genes such as human bone morphogenetic protein-4 (hBMP-4) to enhance bone regeneration (Cui et al., 2020).
Bone is an inhomogeneous 3D structure with complicated topography. Surface roughness plays a pivotal role in topographical modification due to its effect on bone formation and implant fixation. Proper surface roughness can promote the absorption extra-cellular matrix (ECM) proteins (e.g., vitronectin, fibronectin and collagen I) and benefit the adhesion of osteoblasts (Pashkuleva et al., 2010). Subsequently, the ECM proteins provide a RGD sequence which performs as a receptor for osteoblast membrane proteins (mainly Integrins) and then initiate cell spreading, proliferation and differentiation (Mavropoulos et al., 2013). For instance, untreated PEEK with high surface roughness formed via fused filament fabrication displayed remarkably higher osteoblast proliferation and metabolic activity compared to grit-blasted PEEK with a smooth surface (Figure 3A; Han et al., 2019a). However, when the surface roughness exceeded 2.19 μm, osteoblast adhesion was inhibited because it is difficult to form osteoblastic pseudopodia between the larger crests and grooves (Anselme et al., 2000).
Figure 3. Biological evaluation of PEEK implants with different topographies. (A) SEM image of the surface of PEEK formed via fused filament fabrication and the cell proliferation of different groups on days one, three, and five. U, untreated; P, polished, GB (50), 50 μm grit-blasted; GB (120), 120 μm grit-blasted; and GB (250), 250 μm grit-blasted (Han et al., 2019a). (B) SEM image of the surface of sPEEK-WA and Giemsa-stained hard tissue around the implant at 8 weeks after implantation. Red arrows represent the newly formed bone, yellow arrows represent bone ingrowth, blue arrows represent osteoblasts, white arrows represent the sPEEK layer, and orange arrows represent the PEEK implant. (a) PEEK, (b) sPEEK-W, (c) sPEEK-A (low magnification), and (d) sPEEK-WA (high magnification). Scale bar is 200 μm. Reproduced from Zhao et al. (2013). Copyright (2013), with permission from Elsevier. (C) SEM image, cell spreading (a,b) and ALP activity (c,d) of the PEEK/cHAp scaffold after cultivation for 14 days: (a) magnification of 300 μm, (b) magnification of 50 μm, (c) magnification of 100 μm, and (d) magnification of 20 μm. Reproduced from Oladapo et al. (2020). Copyright (2020), with permission from Elsevier.
Microscale to nanoscale surface topography has been shown to affect osteoblastic cell adhesion. Micrometer-scale surface modifications (e.g., sandblasting) of PEEK implants have been used to enhance MG-63 cell behavior and bone-bonding ability (Deng et al., 2015a). Spraying PEEK surfaces with Ti plasma generated hierarchical roughness and increased the surface area (by 40% at the microscale), resulting in enhanced cell proliferation and accelerated cement line formation in hFOB 1.19 cells (Hickey et al., 2019). Compared to micro-roughness surfaces, nano-roughness surfaces showed greater initial protein (e.g., vitronectin and fibronectin) absorption, which subsequently mediates cell adhesion (Khang et al., 2007).
To date, several processes have been used to make nanoscale modifications to the surfaces of PEEK implants. The incorporation of nanosized particles like nHA via spin-coating can enhance the wettability, removal torque, and biocompatibility of PEEK (Johansson et al., 2014). The addition of nanoscale TiO2 (nTiO2) to PEEK via powder mixing and compression molding demonstrated that the enhanced surface roughness effectively promoted cell attachment and new bone regeneration (Wu et al., 2012). Other modifications including treatment with nitrogen, water, and ammonia PIII also displayed similar biological effects on PEEK (Lu et al., 2015; Zhao et al., 2016). Furthermore, the combination of oxygen plasma treatment and sandblasting imparted the PEEK surface with a micro/nano-topographical structure, which triggered the osteogenic differentiation of MG-63 cells and new bone formation (Xu et al., 2015).
Mimicking the morphology of trabecular bone is a crucial concept in the development of porous implant surfaces. Compared to rough surfaces, porous PEEK surfaces exhibit increased osteoblastic differentiation and bonding strength with bone (Torstrick et al., 2018, 2020). Numerous techniques have been applied to develop porous structures on the PEEK surface, including sulfonation, melt extrusion, porogen templating, and PIII technique (Zhao et al., 2013; Lu et al., 2014; Evans et al., 2015; Torstrick et al., 2016; Hieda et al., 2017; Yabutsuka et al., 2017; Deng L.-J. et al., 2018; Wu et al., 2018; Yuan et al., 2018; Conrad and Roeder, 2020; Swaminathan et al., 2020; Wan et al., 2020). Cell viability evaluations revealed that the optimal sulfonation treatment time was 5 min (Ma R. et al., 2020). Sulfonation can generate a 3D porous structure along with -SO3H groups, which are beneficial for pre-osteoblast functions, apatite formation, and bone growth (Figure 3B; Zhao et al., 2013). It's worth noting that sulfonation treatment can introduce more micropores and -SO3H groups on PEKK than PEEK for the reason that PEKK prossesses more ketone groups (Yuan et al., 2018). To further reinforce the bioactivity of porous PEEK, promising drug loading platforms were created by adding gelatin, hydrogel, chitosan, or polydopamine on the surface of PEEK (Deng L.-J. et al., 2018; Ouyang et al., 2018; Wu et al., 2018; Wang et al., 2019). For instance, poly(L-lactic acid)/simvastatin-loaded PEEK coated with a hyaluronic acid hydrogel enhanced the osteogenic differentiation of MC3T3-E1 and the expression of VEGF mRNA compared to uncoated PEEK (Deng L.-J. et al., 2018).
In recent years, clinical interest in 3D printing has grown rapidly. Techniques such as selective laser sintering (SLS) and fused filament fabrication have been adopted to construct PEEK scaffolds with controlled pore size (Roskies et al., 2016; Shuai et al., 2016; Deng et al., 2017; Peng et al., 2017; Oladapo et al., 2020; Spece et al., 2020). Up to now, there has been little consensus on the optimal pore size of scaffolds. Results revealed that porous PEEK with pore sizes ranged 200 to 508 μm exhibited higher proliferation and mineralization compared with smooth PEEK and Ti6Al4V (Torstrick et al., 2016). Another study presented that PEEK scaffold with 450 μm pore size displayed improved ingrowth of new bone and vascular perfusion (Feng et al., 2020). Although developed pore formation processes provide the ability to control the pore structures of PEEK implants, a deeper understanding of how pore size affects cell and tissue responses is needed. To further strengthen the biological properties of scaffolds for bone tissue engineering applications, HA and calcium HA were introduced into the PEEK matrix (Figure 3C; Vaezi et al., 2016; Oladapo et al., 2020). To reinforce the interfacial bonding between HA and PEEK, graphene oxide (GO) can be employed as an interfacial phase (Peng et al., 2017). Furthermore, the addition of graphene nanosheets, CNTs, or CF can boost the mechanical properties of PEEK/HA scaffolds (Shuai et al., 2016; Uddin et al., 2019; Swaminathan et al., 2020).
Bone mainly consists of outer cortical bone and inner cancellous bone. The high modulus (16–23 GPa) of cortical bone endows it with stability and allows it to support the inner porous structure (Augat and Schorlemmer, 2006). The elastic modulus of PEEK (3–4 GPa) is close to that of human cortical bone, reducing the risk of high stress peaks and stress shielding effects during load transfer at the implant–bone interface. The stress shielding is a phenomenon which depicts stiff implants (e.g., titanium) cannot strain the surrounding bone adequately and then lead to bone resorption (Huiskes et al., 2000).
In consideration of the above, the mechanical properties of PEEK implants need to be elevated under specific clinical conditions. It's reported that CFs, CNTs, glass fibers (GFs), and graphene nanosheets (GNS) can be introduced into the PEEK matrix to satisfy the requirements and broaden its applications in load-bearing areas (Ji et al., 2015; Shuai et al., 2016; Yang et al., 2018; Han et al., 2019b). For instance, the stress distribution of a PEEK implant with 60% endless carbon fibers was similar to that of a titanium implant in dental application (Schwitalla et al., 2015). Mechanical property evaluations suggested that the elastic modulus of CF/PEEK and GF/PEEK could reach to 18 and 12 GPa, respectively (Lee et al., 2012). Furthermore, the mechanical properties of PEEK can be controlled by adding different amounts or lengths of fibers to the composite. For example, the bending strengths of CF/PEEK containing 25, 30, 35, and 40 wt% CF were 230.1–264.6 MPa, and the compressive strengths were 191.2–215.8 MPa (Qin et al., 2019). Meanwhile, the mechanical strengths of CF/PEEK composites containing CFs with lengths of 2–3 mm were more than two times those of the composites containing CFs with lengths of 150–200 μm (Li et al., 2019b). While fiber-reinforced PEEK has been manufactured through the traditional injection molding process, a novel technique called fused deposition modeling (FDM) has been applied currently. CF/PEEK formed via FDM was found to possess similar strength and toughness as the injection-molded samples along with better tensile and bending strengths compared to pure PEEK (Han et al., 2019b; Li et al., 2019a).
Although reinforced PEEK performs excellent mechanical properties, further investigations are needed to increase the bioactivity of hybrid PEEK materials. Researchers have proposed numerous hybrid materials containing different bioactive components, their mechanical and biological properties are summarized in Table 1. Moreover, the modification of CF-reinforced PEEK surfaces via chemical treatment, graphene functionalization, and PrA treatment has resulted in similar biological properties (Miyazaki et al., 2017; Yabutsuka et al., 2018; Yan J. H. et al., 2018; Ma J. et al., 2020).
Upon implantation, the innate immune response of bone tissue is mainly initiated by macrophages, which release a series of cytokines and growth factors. Macrophages can be divided into the pro-inflammatory M1 phenotype and anti-inflammatory M2 phenotype (Mosser and Edwards, 2008). To modulate the inflammatory response to PEEK implants, Gao et al. proposed a layer-by-layer self-assembly technique that facilitates the polarization of macrophages into the M2 phenotype and contributes to the upregulation of osteogenesis and the downregulation of osteoclastogenesis (Figure 4; Gao et al., 2020). Fukuda et al. found that the plasma treatment and subsequent phosphorylation on the PEEK surface attenuated the phenotypic polarization of RAW264.7 macrophages to an inflammatory phenotype (Fukuda et al., 2019). A recent study revealed that PEEK modified with hydrofluoric acid and nitric acid could promote the polarization of macrophages (M2 phenotype) and inhibit the expression of proinflammatory factors via the NF-κB pathway (Huo et al., 2020).
Figure 4. Evaluation of the acute inflammatory responses of RAW264.7 cells on different samples. (A) Heat map of the RT-PCR results of M1- and M2-related genes after incubation for 1, 3, and 5 days. (B) Level of TNF-α released by RAW264.7 cells after culturing for three days. (C) TRAP activity after culturing for 8 days to evaluate the osteoclastic differentiation of RAW264.7 cells. *p < 0.05, **p < 0.01. Reproduced from Gao et al. (2020). Copyright (2020), with permission from Elsevier.
Researchers have also focused on surface modifications that target microbial infections. To the best of our knowledge, there are two ways to fabricate an anti-biofilm surface: depositing antibacterial coatings that release antimicrobial constituents or depositing antiadhesive coatings that restrict the adhesion of bacteria. Various antibiotics (e.g., lawsone, dexamethasone, minocycline, hinokitiol, tobramycin, gentamicin sulfate, and antimicrobial peptides) have been adopted to treat infections caused by implants (Ur Rehman et al., 2018; Zhang et al., 2018b; Deng et al., 2019; Xu et al., 2019; Yuan et al., 2019; Xue et al., 2020; Yin et al., 2020). Since the abuse of antibiotics has contributed to the emergence of bacterial resistance, several ions and their nanoparticles have emerged as promising alternatives to antibiotics (Figures 5A,B). The details of the techniques involved and the biological effects of these ions are summarized in Table 2. It is worth noting that the uncontrolled release of bactericides will exacerbate bacterial resistance. To avoid this phenomenon, silk fibroin, which was reported to cause the pH-responsive controlled release of bactericides, has been introduced into PEEK implants (Yan J. et al., 2018).
Figure 5. Assessment of the antibacterial ability of modified PEEK. (A) Inhibition zones of 3D PEEK and 3D PEEK/Ag against E. coli and S. aureus. (B) Bacterial dynamic curves of E. coli and S. aureus on 3D PEEK and 3D PEEK/Ag at OD600. Reproduced from Deng et al. (2017). Copyright (2017), with permission from Elsevier. (C) E. coli and (D) S. aureus seeded on the PEEK, SP (sulfonated only), SPW25 (sulfonated and hydrothermal treated at 25°C), and SPW120 (sulfonated and hydrothermal treated at 120°C) at a bacteria concentration of 107 cfu/ml. The green arrows indicate the morphology of E. coli (high magnification), while the yellow arrows show the morphology of S. aureus (low magnification). Reproduced from Ouyang et al. (2016). Copyright (2016), with permission from Elsevier.
Surface roughness and hydrophobicity are the dominant factors affecting bacterial adhesion and biofilm formation. Bollen et al. suggested that enhancing the surface roughness can improve the attachment of bacteria when Ra >0.2 μm (Bollen et al., 1996). In addition, it has been reported that bacteria tend to adhere on implants with hydrophilic surfaces (Das et al., 2010). To prepare porous surfaces, sulfonation followed by hydrothermal treatment which can remove residues are applied (Ouyang et al., 2016; Yuan et al., 2019). Moreover, a porous surface can limit the adhesion of bacteria with different shapes and sizes. For instance, porous structures are considered to be more easily to trap spherical S. aureus compared to elongated E. coli (Figures 5C,D; Ouyang et al., 2016).
Except for microbial infections, bone tumor like osteosarcoma should also be taken into consideration. In order to reduce the risk of tumor recurrence after a bone removal surgery, applying an implant with anti-cancer agents is an effective method. Studies confirmed that the anti-cancer drugs (e.g., methotrexate, and 5-fluorouracil) loaded in ceramic cements could inhibit the growth of osteosarcoma cell line (Prasad et al., 2018; Wu et al., 2020). Currently, light-assisted photothermal therapy (PTT) based on photothermal conversion agent (e.g., MXenes) was introduced to PEEK implants to defect osteosarcoma cells (Yin et al., 2020).
After decades of research, PEEK implants in bone tissue engineering have experienced three stages of development: (1) first-generation implants possessing outstanding mechanical properties; (2) second-generation implants with prominent cytocompatibility and osteogenic activity; and (3) third-generation implants equipped with outstanding osseointegration and anti-inflammation/-infection ability. According to previous studies, we have grouped PEEK modifications into four categories based on their inspiration: the constituents (inorganic phase, mineral ions, and organic phase), structure (surface roughness and porosity), mechanical function, and immune function of human bone.
Although the techniques for modifying PEEK have matured, numerous remaining obstacles must be overcome before modified PEEK implants can be applied in clinical practice: (1) the ratio of different constituents must be optimized to maximize bone regeneration and the mechanical properties; (2) methods must be developed to control the release of biomolecules and achieve the desired long-term effects; and (3) experiments are needed to identify the ideal pore structure to optimize biological performance. Thus, extensive studies are still needed to observe the long-term implantation of PEEK in vivo and elucidate which strategies are appropriate to modify PEEK in clinical settings. In this respect, the complexity, reproducibility, stability and cost-effectiveness of the manufacturing process should also be considered. In the future, researchers will focus on PEEK implants possessing the optimal combination of osteointegration, vascularization, anti-inflammation/-infection, and mechanical properties. It is worth noting that modifications that mimic the components, structure, and function of human bone are expected to be the key to fabricating multifunctional implants. Also, it can provide inspirations for researchers to modify other PAEK materials and expand their applications in the field of orthopedic, spinal and dental impalnts.
XG conceptualized and designed the manuscript. XG and XS drafted the manuscript. YS and JW designed the table. YL, KY, and YW designed the figure. YZ revised the manuscript. All authors approved the final version.
This work was supported by Department of Finance of Jilin Province (jcsz2020304-1) and the Department of Science and Technology of Jilin Province (20200201302JC).
The authors declare that the research was conducted in the absence of any commercial or financial relationships that could be construed as a potential conflict of interest.
The reviewer YZ declared a shared affiliation, with no collaboration, with the authors to the handling Editor at the time of the review.
Ambard, A. J., and Mueninghoff, L. (2006). Calcium phosphate cement: review of mechanical and biological properties. J. Prosthodont. 15, 321–328. doi: 10.1111/j.1532-849X.2006.00129.x
Anselme, K., Bigerelle, M., Noel, B., Dufresne, E., Judas, D., Iost, A., et al. (2000). Qualitative and quantitative study of human osteoblast adhesion on materials with various surface roughnesses. J. Biomed. Mater. Res. 49, 155–166. doi: 10.1002/(SICI)1097-4636(200002)49:2<155::AID-JBM2>3.0.CO;2-J
Augat, P., and Schorlemmer, S. (2006). The role of cortical bone and its microstructure in bone strength. Age Ageing 35 (Suppl. 2):ii27–ii31. doi: 10.1093/ageing/afl081
Barkarmo, S., Wennerberg, A., Hoffman, M., Kjellin, P., Breding, K., Handa, P., et al. (2013). Nano-hydroxyapatite-coated PEEK implants: a pilot study in rabbit bone. J. Biomed. Mater. Res. Part A 101A, 465–471. doi: 10.1002/jbm.a.34358
Bastan, F. E. (2020). Fabrication and characterization of an electrostatically bonded PEEK- hydroxyapatite composites for biomedical applications. J. Biomed. Mater. Res. Part B Appl. Biomater. 108, 2513–2527. doi: 10.1002/jbm.b.34583
Baştan, F. E., Atiq Ur Rehman, M., Avcu, Y. Y., Avcu, E., Üstel, F., and Boccaccini, A.R. (2018). Electrophoretic co-deposition of PEEK-hydroxyapatite composite coatings for biomedical applications. Colloids Surf. B Biointerfaces 169, 176–182. doi: 10.1016/j.colsurfb.2018.05.005
Binyamin, G., Shafi, B. M., and Mery, C. M. (2006). Biomaterials: a primer for surgeons. Semin. Pediatr. Surg. 15, 276–283. doi: 10.1053/j.sempedsurg.2006.07.007
Bollen, C. M., Papaioanno, W., Van Eldere, J., Schepers, E., Quirynen, M., and van Steenberghe, D. (1996). The influence of abutment surface roughness on plaque accumulation and peri-implant mucositis. Clin. Oral Implants Res. 7, 201–211. doi: 10.1034/j.1600-0501.1996.070302.x
Chen, M., Ouyang, L., Lu, T., Wang, H., Meng, F., Yang, Y., et al. (2017). Enhanced bioactivity and bacteriostasis of surface fluorinated polyetheretherketone. ACS Appl. Mater. Interf. 9, 16824–16833. doi: 10.1021/acsami.7b02521
Conrad, T. L., and Roeder, R. K. (2020). Effects of porogen morphology on the architecture, permeability, and mechanical properties of hydroxyapatite whisker reinforced polyetheretherketone scaffolds. J. Mech. Behav. Biomed. Mater. 106:103730. doi: 10.1016/j.jmbbm.2020.103730
Cui, L., Zhang, J., Zou, J., Yang, X., Guo, H., Tian, H., et al. (2020). Electroactive composite scaffold with locally expressed osteoinductive factor for synergistic bone repair upon electrical stimulation. Biomaterials 230:119617. doi: 10.1016/j.biomaterials.2019.119617
da Cruz, M. B., Marques, J. F., Penarrieta-Juanito, G. M., Costa, M., Souza, J. C., Magini, R. S., et al. (2019). Hard and soft tissue cell behavior on polyetheretherketone, zirconia, and titanium implant materials. Int. J. Oral Maxillofac. Implants 34, 39–46. doi: 10.11607/jomi.6926
Das, T., Sharma, P. K., Busscher, H. J., van der Mei, H. C., and Krom, B. P. (2010). Role of extracellular DNA in initial bacterial adhesion and surface aggregation. Appl. Environ. Microbiol. 76, 3405–3408. doi: 10.1128/AEM.03119-09
Deng, L., Deng, Y., and Xie, K. (2017). AgNPs-decorated 3D printed PEEK implant for infection control and bone repair. Colloids Surf B Biointerf. 160, 483–492. doi: 10.1016/j.colsurfb.2017.09.061
Deng, L., He, X., Xie, K., Xie, L., and Deng, Y. (2019). Dual therapy coating on micro/nanoscale porous polyetheretherketone to eradicate biofilms and accelerate bone tissue repair. Macromol. Biosci. 19:e1800376. doi: 10.1002/mabi.201800376
Deng, L.-J., Wu, Y.-L., He, X.-H., Xie, K.-N., Xie, L., and Deng, Y. (2018). Simvastatin delivery on PEEK for bioactivity and osteogenesis enhancements. J. Biomater. Sci. Polym. Ed. 29, 2237–2251. doi: 10.1080/09205063.2018.1534668
Deng, Y., Gao, X., Shi, X.-L., Lu, S., Yang, W., Duan, C., et al. (2020). Graphene oxide and adiponectin-functionalized sulfonated poly(etheretherketone) with effective osteogenicity and remotely repeatable photodisinfection. Chem. Mater. 32, 2180–2193. doi: 10.1021/acs.chemmater.0c00290
Deng, Y., Liu, X., Xu, A., Wang, L., Luo, Z., Zheng, Y., et al. (2015a). Effect of surface roughness on osteogenesis in vitro and osseointegration in vivo of carbon fiber-reinforced polyetheretherketone-nanohydroxyapatite composite. Int. J. Nanomedicine 10, 1425–1447. doi: 10.2147/IJN.S75557
Deng, Y., Yang, L., Huang, X., Chen, J., Shi, X., Yang, W., et al. (2018). Dual Ag/ZnO-decorated micro-/nanoporous sulfonated polyetheretherketone with superior antibacterial capability and biocompatibility via layer-by-layer self-assembly strategy. Macromol. Biosci. 18:e1800028. doi: 10.1002/mabi.201800028
Deng, Y., Zhou, P., Liu, X., Wang, L., Xiong, X., Tang, Z., et al. (2015b). Preparation, characterization, cellular response and in vivo osseointegration of polyetheretherketone/nano-hydroxyapatite/carbon fiber ternary biocomposite. Colloids Surf. B Biointerfaces 136, 64–73. doi: 10.1016/j.colsurfb.2015.09.001
Díez-Pascual, A. M., and Díez-Vicente, A. L. (2014). Development of nanocomposites reinforced with carboxylated poly(ether ether ketone) grafted to zinc oxide with superior antibacterial properties. ACS Appl. Mater. Interfaces 6, 3729–3741. doi: 10.1021/am500171x
Dong, T., Duan, C., Wang, S., Gao, X., Yang, Q., Yang, W., et al. (2020). Multifunctional surface with enhanced angiogenesis for improving long-term osteogenic fixation of poly(ether ether ketone) implants. ACS Appl. Mater. Interfaces 12, 14971–14982. doi: 10.1021/acsami.0c02304
Durham, J. W., Montelongo, S. A., Ong, J. L., Guda, T., Allen, M. J., and Rabiei, A. (2016). Hydroxyapatite coating on PEEK implants: biomechanical and histological study in a rabbit model. Mater. Sci. Eng. C 68, 723–731. doi: 10.1016/j.msec.2016.06.049
Elawadly, T., Radi, I. A. W., El Khadem, A., and Osman, R. B. (2017). Can PEEK be an implant material? Evaluation of surface topography and wettability of filled versus unfilled peek with different surface roughness. J. Oral. Implantol. 43, 456–461. doi: 10.1563/aaid-joi-D-17-00144
Eliaz, N., and Metoki, N. (2017). Calcium phosphate bioceramics: a review of their history, structure, properties, coating technologies and biomedical applications. Materials 10:334. doi: 10.3390/ma10040334
Eschbach, L. (2000). Nonresorbable polymers in bone surgery. Injury 31 (Suppl. 4): 22–27. doi: 10.1016/S0020-1383(00)80019-4
Evans, N. T., Torstrick, F. B., Lee, C. S., Dupont, K. M., Safranski, D. L., Chang, W. A., et al. (2015). High-strength, surface-porous polyether-ether-ketone for load-bearing orthopedic implants. Acta Biomater. 13, 159–167. doi: 10.1016/j.actbio.2014.11.030
Fage, S. W., Muris, J., Jakobsen, S. S., and Thyssen, J. P. (2016). Titanium: a review on exposure, release, penetration, allergy, epidemiology, and clinical reactivity. Contact Derm. 74, 323–345. doi: 10.1111/cod.12565
Feng, X., Ma, L., Liang, H., Liu, X., Lei, J., Li, W., et al. (2020). Osteointegration of 3D-printed fully porous polyetheretherketone scaffolds with different pore sizes. ACS Omega 5, 26655–26666. doi: 10.1021/acsomega.0c03489
Fukuda, N., Tsuchiya, A., Sunarso, Toita, R., Tsuru, K., Mori, Y., et al. (2019). Surface plasma treatment and phosphorylation enhance the biological performance of poly(ether ether ketone). Colloids Surf. B Biointerfaces 173, 36–42. doi: 10.1016/j.colsurfb.2018.09.032
Gan, K., Liu, H., Jiang, L., Liu, X., Song, X., Niu, D., et al. (2016). Bioactivity and antibacterial effect of nitrogen plasma immersion ion implantation on polyetheretherketone. Dent. Mater. 32, e263–e274. doi: 10.1016/j.dental.2016.08.215
Gao, A., Liao, Q., Xie, L., Wang, G., Zhang, W., Wu, Y., et al. (2020). Tuning the surface immunomodulatory functions of polyetheretherketone for enhanced osseointegration. Biomaterials 230:119642. doi: 10.1016/j.biomaterials.2019.119642
Ge, J., Wang, F., Xu, Z., Shen, X., Gao, C., Wang, D., et al. (2020). Influences of niobium pentoxide on roughness, hydrophilicity, surface energy and protein absorption, and cellular responses to PEEK based composites for orthopedic applications. J. Mater. Chem. B 8, 2618–2626. doi: 10.1039/C9TB02456E
Godara, A., Raabe, D., and Green, S. (2007). The influence of sterilization processes on the micromechanical properties of carbon fiber-reinforced PEEK composites for bone implant applications. Acta Biomater. 3, 209–220. doi: 10.1016/j.actbio.2006.11.005
Guillot, R., Pignot-Paintrand, I., Lavaud, J., Decambron, A., Bourgeois, E., Josserand, V., et al. (2016). Assessment of a polyelectrolyte multilayer film coating loaded with BMP-2 on titanium and PEEK implants in the rabbit femoral condyle. Acta Biomater. 36, 310–322. doi: 10.1016/j.actbio.2016.03.010
Ha, S. W., Kirch, M., Birchler, F., Eckert, K. L., Mayer, J., Wintermantel, E., et al. (1997). Surface activation of polyetheretherketone (PEEK) and formation of calcium phosphate coatings by precipitation. J. Mater. Sci. Mater. Med. 8, 683–690. doi: 10.1023/A:1018535923173
Han, C. M., Jang, T. S., Kim, H. E., and Koh, Y. H. (2014). Creation of nanoporous TiO2 surface onto polyetheretherketone for effective immobilization and delivery of bone morphogenetic protein. J. Biomed. Mater. Res. A 102, 793–800. doi: 10.1002/jbm.a.34748
Han, C. M., Lee, E. J., Kim, H. E., Koh, Y. H., Kim, K. N., Ha, Y., et al. (2010). The electron beam deposition of titanium on polyetheretherketone (PEEK) and the resulting enhanced biological properties. Biomaterials 31, 3465–3470. doi: 10.1016/j.biomaterials.2009.12.030
Han, X., Sharma, N., Xu, Z., Scheideler, L., Geis-Gerstorfer, J., Rupp, F., et al. (2019a). An in vitro study of osteoblast response on fused-filament fabrication 3D printed PEEK for dental and cranio-maxillofacial implants. J. Clin. Med. 8:771. doi: 10.3390/jcm8060771
Han, X., Yang, D., Yang, C., Spintzyk, S., Scheideler, L., Li, P., et al. (2019b). Carbon fiber reinforced PEEK composites based on 3D-printing technology for orthopedic and dental applications. J. Clin. Med. 8:240. doi: 10.3390/jcm8020240
He, G., Dahl, T., Veis, A., and George, A. (2003). Nucleation of apatite crystals in vitro by self-assembled dentin matrix protein 1. Nat. Mater. 2, 552–558. doi: 10.1038/nmat945
Hickey, D. J., Lorman, B., and Fedder, I. L. (2019). Improved response of osteoprogenitor cells to titanium plasma-sprayed PEEK surfaces. Colloids Surf. B Biointerfaces 175, 509–516. doi: 10.1016/j.colsurfb.2018.12.037
Hieda, A., Uemura, N., Hashimoto, Y., Toda, I., and Baba, S. (2017). In vivo bioactivity of porous polyetheretherketone with a foamed surface. Dent. Mater. J. 36, 222–229. doi: 10.4012/dmj.2016-277
Huiskes, R., Ruimerman, R., van Lenthe, G. H., and Janssen, J. D. (2000). Effects of mechanical forces on maintenance and adaptation of form in trabecular bone. Nature 405, 704–706. doi: 10.1038/35015116
Huiskes, R., Weinans, H., and van Rietbergen, B. (1992). The relationship between stress shielding and bone resorption around total hip stems and the effects of flexible materials. Clin. Orthop. Relat. Res. 274, 124–134. doi: 10.1097/00003086-199201000-00014
Huo, S., Meng, X., Zhang, S., Yue, B., Zhao, Y., Long, T., et al. (2020). Hydrofluoric acid and nitric acid cotreatment for biofunctionalization of polyetheretherketone in M2 macrophage polarization and osteogenesis. J. Biomed. Mater. Res. Part A. 108, 2473–2483. doi: 10.1002/jbm.a.37079
Jabbari, E., Oyane, A., Nakamura, M., Sakamaki, I., Shimizu, Y., Miyata, S., et al. (2018). Laser-assisted wet coating of calcium phosphate for surface-functionalization of PEEK. PLoS ONE 13:e0206524. doi: 10.1371/journal.pone.0206524
Ji, S., Sun, C., Zhao, J., and Liang, F. (2015). Comparison and analysis on mechanical property and machinability about polyetheretherketone and carbon-fibers reinforced polyetheretherketone. Materials 8, 4118–4130. doi: 10.3390/ma8074118
Jimbo, R., Ivarsson, M., Koskela, A., Sul, Y. T., and Johansson, C. B. (2010). Protein adsorption to surface chemistry and crystal structure modification of titanium surfaces. J. Oral Maxillofac. Res. 1:e3. doi: 10.5037/jomr.2010.1303
Johansson, P., Barkarmo, S., Hawthan, M., Peruzzi, N., Kjellin, P., and Wennerberg, A. (2018). Biomechanical, histological, and computed X-ray tomographic analyses of hydroxyapatite coated PEEK implants in an extended healing model in rabbit. J. Biomed. Mater. Res. A 106, 1440–1447. doi: 10.1002/jbm.a.36345
Johansson, P., Jimbo, R., Kjellin, P., Currie, F., Chrcanovic, B. R., and Wennerberg, A. (2014). Biomechanical evaluation and surface characterization of a nano-modified surface on PEEK implants: a study in the rabbit tibia. Int. J. Nanomedicine 9, 3903–3911. doi: 10.2147/IJN.S60387
Johansson, P., Jimbo, R., Kozai, Y., Sakurai, T., Kjellin, P., Currie, F., et al. (2015). Nanosized hydroxyapatite coating on PEEK implants enhances early bone formation: a histological and three-dimensional investigation in rabbit bone. Materials 8, 3815–3830. doi: 10.3390/ma8073815
Johansson, P., Jimbo, R., Naito, Y., Kjellin, P., Currie, F., and Wennerberg, A. (2016). Polyether ether ketone implants achieve increased bone fusion when coated with nano-sized hydroxyapatite: a histomorphometric study in rabbit bone. Int. J. Nanomedicine 11, 1435–1442. doi: 10.2147/IJN.S100424
Jung, H. D., Park, H. S., Kang, M. H., Li, Y., Kim, H. E., Koh, Y. H., et al. (2016). Reinforcement of polyetheretherketone polymer with titanium for improved mechanical properties and in vitro biocompatibility. J. Biomed. Mater. Res. Part B Appl. Biomater. 104, 141–148. doi: 10.1002/jbm.b.33361
Kakinuma, H., Ishii, K., Ishihama, H., Honda, M., Toyama, Y., Matsumoto, M., et al. (2015). Antibacterial polyetheretherketone implants immobilized with silver ions based on chelate-bonding ability of inositol phosphate: processing, material characterization, cytotoxicity, and antibacterial properties. J. Biomed. Mater. Res. Part A 103, 57–64. doi: 10.1002/jbm.a.35157
Katzer, A., Marquardt, H., Westendorf, J., Wening, J. V., and von Foerster, G. (2002). Polyetheretherketone–cytotoxicity and mutagenicity in vitro. Biomaterials 23, 1749–1759. doi: 10.1016/S0142-9612(01)00300-3
Khang, D., Kim, S. Y., Liu-Snyder, P., Palmore, G. T., Durbin, S. M., and Webster, T. J. (2007). Enhanced fibronectin adsorption on carbon nanotube/poly(carbonate) urethane: independent role of surface nano-roughness and associated surface energy. Biomaterials 28, 4756–4768. doi: 10.1016/j.biomaterials.2007.07.018
Kilpadi, D. V., and Lemons, J. E. (1994). Surface energy characterization of unalloyed titanium implants. J. Biomed. Mater. Res. 28, 1419–1425. doi: 10.1002/jbm.820281206
Kizuki, T., Matsushita, T., and Kokubo, T. (2015). Apatite-forming PEEK with TiO2 surface layer coating. J. Mater. Sci. Mater. Med. 26:5359. doi: 10.1007/s10856-014-5359-1
Klein, C. P., de Blieck-Hogervorst, J. M., Wolke, J. G., and de Groot, K. (1990). Studies of the solubility of different calcium phosphate ceramic particles in vitro. Biomaterials 11, 509–512. doi: 10.1016/0142-9612(90)90067-Z
Kurtz, S. M., and Devine, J. N. (2007). PEEK biomaterials in trauma, orthopedic, and spinal implants. Biomaterials 28, 4845–4869. doi: 10.1016/j.biomaterials.2007.07.013
Lee, J. H., Jang, H. L., Lee, K. M., Baek, H. R., Jin, K., Hong, K. S., et al. (2013). In vitro and in vivo evaluation of the bioactivity of hydroxyapatite-coated polyetheretherketone biocomposites created by cold spray technology. Acta Biomater. 9, 6177–6187. doi: 10.1016/j.actbio.2012.11.030
Lee, W.-T., Koak, J.-Y., Lim, Y.-J., Kim, S.-K., Kwon, H.-B., and Kim, M.-J. (2012). Stress shielding and fatigue limits of poly-ether-ether-ketone dental implants. J. Biomed. Mater. Res. Part B Appl. Biomater. 100, 1044–1052. doi: 10.1002/jbm.b.32669
Li, Q., Zhao, W., Li, Y., Yang, W., and Wang, G. (2019a). Flexural properties and fracture behavior of CF/PEEK in orthogonal building orientation by FDM: microstructure and mechanism. Polymers 11:656. doi: 10.3390/polym11040656
Li, Y., Wang, D., Qin, W., Jia, H., Wu, Y., Ma, J., et al. (2019b). Mechanical properties, hemocompatibility, cytotoxicity and systemic toxicity of carbon fibers/poly(ether-ether-ketone) composites with different fiber lengths as orthopedic implants. J. Biomater. Sci. Polym. Ed 30, 1709–1724. doi: 10.1080/09205063.2019.1659711
Liu, C., Chan, K., Shen, J., Liao, C., Yeung, K., and Tjong, S. (2016). Polyetheretherketone hybrid composites with bioactive nanohydroxyapatite and multiwalled carbon nanotube fillers. Polymers 8:425. doi: 10.3390/polym8120425
Liu, S., Zhu, Y., Gao, H., Ge, P., Ren, K., Gao, J., et al. (2018). One-step fabrication of functionalized poly(etheretherketone) surfaces with enhanced biocompatibility and osteogenic activity. Mater. Sci. Eng. C Mater. Biol. Appl. 88, 70–78. doi: 10.1016/j.msec.2018.03.003
Liu, W., Li, J., Cheng, M., Wang, Q., Qian, Y., Yeung, K. W. K., et al. (2019). A surface-engineered polyetheretherketone biomaterial implant with direct and immunoregulatory antibacterial activity against methicillin-resistant staphylococcus aureus. Biomaterials 208, 8–20. doi: 10.1016/j.biomaterials.2019.04.008
Liu, X., Gan, K., Liu, H., Song, X., Chen, T., and Liu, C. (2017). Antibacterial properties of nano-silver coated PEEK prepared through magnetron sputtering. Dental Mater. 33, e348–e360. doi: 10.1016/j.dental.2017.06.014
Lu, T., Liu, X., Qian, S., Cao, H., Qiao, Y., Mei, Y., et al. (2014). Multilevel surface engineering of nanostructured TiO2 on carbon-fiber-reinforced polyetheretherketone. Biomaterials 35, 5731–5740. doi: 10.1016/j.biomaterials.2014.04.003
Lu, T., Wen, J., Qian, S., Cao, H., Ning, C., Pan, X., et al. (2015). Enhanced osteointegration on tantalum-implanted polyetheretherketone surface with bone-like elastic modulus. Biomaterials 51, 173–183. doi: 10.1016/j.biomaterials.2015.02.018
Ma, J., Liang, Q., Qin, W., Lartey, P. O., Li, Y., and Feng, X. (2020). Bioactivity of nitric acid and calcium chloride treated carbon-fibers reinforced polyetheretherketone for dental implant. J. Mech. Behav. Biomed. Mater. 102:103497. doi: 10.1016/j.jmbbm.2019.103497
Ma, R., and Guo, D. (2019). Evaluating the bioactivity of a hydroxyapatite-incorporated polyetheretherketone biocomposite. J. Orthop. Surg. Res. 14:32. doi: 10.1186/s13018-019-1069-1
Ma, R., Tang, S., Tan, H., Qian, J., Lin, W., Wang, Y., et al. (2014). Preparation, characterization, in vitro bioactivity, and cellular responses to a polyetheretherketone bioactive composite containing nanocalcium silicate for bone repair. ACS Appl. Mater. Interfaces 6, 12214–12225. doi: 10.1021/am504409q
Ma, R., Wang, J., Li, C., Ma, K., Wei, J., Yang, P., et al. (2020). Effects of different sulfonation times and post-treatment methods on the characterization and cytocompatibility of sulfonated PEEK. J. Biomater. Appl. 35, 342–352. doi: 10.1177/0885328220935008
Mahjoubi, H., Buck, E., Manimunda, P., Farivar, R., Chromik, R., Murshed, M., et al. (2017). Surface phosphonation enhances hydroxyapatite coating adhesion on polyetheretherketone and its osseointegration potential. Acta Biomater. 47, 149–158. doi: 10.1016/j.actbio.2016.10.004
Masamoto, K., Fujibayashi, S., Yabutsuka, T., Hiruta, T., Otsuki, B., Okuzu, Y., et al. (2019). In vivo and in vitro bioactivity of a “precursor of apatite” treatment on polyetheretherketone. Acta Biomater. 91, 48–59. doi: 10.1016/j.actbio.2019.04.041
Mavropoulos, E., Hausen, M., Costa, A. M., Alves, G., Mello, A., Ospina, C. A., et al. (2013). The impact of the RGD peptide on osteoblast adhesion and spreading on zinc-substituted hydroxyapatite surface. J. Mater. Sci. Mater. Med. 24, 1271–1283. doi: 10.1007/s10856-013-4851-3
Mei, S., Yang, L., Pan, Y., Wang, D., Wang, X., Tang, T., et al. (2019). Influences of tantalum pentoxide and surface coarsening on surface roughness, hydrophilicity, surface energy, protein adsorption and cell responses to PEEK based biocomposite. Colloids Surf. B Biointerfaces 174, 207–215. doi: 10.1016/j.colsurfb.2018.10.081
Miyazaki, T., Matsunami, C., and Shirosaki, Y. (2017). Bioactive carbon-PEEK composites prepared by chemical surface treatment. Mater. Sci. Eng. C Mater. Biol. Appl. 70, 71–75. doi: 10.1016/j.msec.2016.08.058
Monich, P. R., Berti, F. V., Porto, L. M., Henriques, B., Novaes de Oliveira, A. P., Fredel, M. C., et al. (2017). Physicochemical and biological assessment of PEEK composites embedding natural amorphous silica fibers for biomedical applications. Mater. Sci. Eng. C 79, 354–362. doi: 10.1016/j.msec.2017.05.031
Mosser, D. M., and Edwards, J. P. (2008). Exploring the full spectrum of macrophage activation. Nat. Rev. Immunol. 8, 958–969. doi: 10.1038/nri2448
Niki, Y., Matsumoto, H., Otani, T., Suda, Y., and Toyama, Y. (2001). Metal ion concentrations in the joint fluid immediately after total knee arthroplasty. Mod. Rheumatol. 11, 192–196. doi: 10.3109/s101650170003
Oladapo, B. I., Ismail, S. O., Bowoto, O. K., Omigbodun, F. T., Olawumi, M. A., and Muhammad, M. A. (2020). Lattice design and 3D-printing of PEEK with Ca10(OH)(PO4)3 and in-vitro bio-composite for bone implant. Int. J. Biol. Macromol. 165, 50–62. doi: 10.1016/j.ijbiomac.2020.09.175
Ouyang, L., Sun, Z., Wang, D., Qiao, Y., Zhu, H., Ma, X., et al. (2018). Smart release of doxorubicin loaded on polyetheretherketone (PEEK) surface with 3D porous structure. Colloids Surf. B Biointerfaces 163, 175–183. doi: 10.1016/j.colsurfb.2017.12.045
Ouyang, L., Zhao, Y., Jin, G., Lu, T., Li, J., Qiao, Y., et al. (2016). Influence of sulfur content on bone formation and antibacterial ability of sulfonated PEEK. Biomaterials 83, 115–126. doi: 10.1016/j.biomaterials.2016.01.017
Pashkuleva, I., Marques, A. P., Vaz, F., and Reis, R. L. (2010). Surface modification of starch based biomaterials by oxygen plasma or UV-irradiation. J. Mater. Sci. Mater. Med. 21, 21–32. doi: 10.1007/s10856-009-3831-0
Peng, S., Feng, P., Wu, P., Huang, W., Yang, Y., Guo, W., et al. (2017). Graphene oxide as an interface phase between polyetheretherketone and hydroxyapatite for tissue engineering scaffolds. Sci. Rep. 7:46604. doi: 10.1038/srep46604
Pezzotti, G., Marin, E., Adachi, T., Lerussi, F., Rondinella, A., Boschetto, F., et al. (2018). Incorporating Si3N4 into PEEK to produce antibacterial, osteocondutive, and radiolucent spinal implants. Macromol. Biosci. 18:1800033. doi: 10.1002/mabi.201800033
Prasad, S. R., Kumar, T. S. S., and Jayakrishnan, A. (2018). Ceramic core with polymer corona hybrid nanocarrier for the treatment of osteosarcoma with co-delivery of protein and anti-cancer drug. Nanotechnology 29:015101. doi: 10.1088/1361-6528/aa9a21
Qin, W., Li, Y., Ma, J., Liang, Q., Cui, X., Jia, H., et al. (2020). Osseointegration and biosafety of graphene oxide wrapped porous CF/PEEK composites as implantable materials: the role of surface structure and chemistry. Dental Mater. 36, 1289–1302. doi: 10.1016/j.dental.2020.06.004
Qin, W., Li, Y., Ma, J., Liang, Q., and Tang, B. (2019). Mechanical properties and cytotoxicity of hierarchical carbon fiber-reinforced poly (ether-ether-ketone) composites used as implant materials. J. Mech. Behav. Biomed. Mater. 89, 227–233. doi: 10.1016/j.jmbbm.2018.09.040
Quarto, R., and Giannoni, P. (2016). “Bone tissue engineering: past-present-future,” in Mesenchymal Stem Cells: Methods and Protocols, ed. M. Gnecchi (New York, NY: Springer New York), 21–33. doi: 10.1007/978-1-4939-3584-0_2
Reigstad, O., Johansson, C., Stenport, V., Wennerberg, A., Reigstad, A., and Røkkum, M. (2011). Different patterns of bone fixation with hydroxyapatite and resorbable CaP coatings in the rabbit tibia at 6, 12, and 52 weeks. J. Biomed. Mater. Res. Part B Appl. Biomater. 99, 14–20. doi: 10.1002/jbm.b.31866
Ren, Y., Sikder, P., Lin, B., and Bhaduri, S. B. (2018). Microwave assisted coating of bioactive amorphous magnesium phosphate (AMP) on polyetheretherketone (PEEK). Mater. Sci. Eng. C 85, 107–113. doi: 10.1016/j.msec.2017.12.025
Røkkum, M., Reigstad, A., Johansson, C. B., and Albrektsson, T. (2003). Tissue reactions adjacent to well-fixed hydroxyapatite-coated acetabular cups. Histopathology of ten specimens retrieved at reoperation after 0.3 to 5.8 years. J. Bone Joint Surg. Br. 85, 440–447. doi: 10.1302/0301-620X.85B3.12834
Roskies, M., Jordan, J. O., Fang, D., Abdallah, M. N., Hier, M. P., Mlynarek, A., et al. (2016). Improving PEEK bioactivity for craniofacial reconstruction using a 3D printed scaffold embedded with mesenchymal stem cells. J. Biomater. Appl. 31, 132–139. doi: 10.1177/0885328216638636
Ryoo, H. M., Lee, M. H., and Kim, Y. J. (2006). Critical molecular switches involved in BMP-2-induced osteogenic differentiation of mesenchymal cells. Gene 366, 51–57. doi: 10.1016/j.gene.2005.10.011
Samavedi, S., Whittington, A. R., and Goldstein, A. S. (2013). Calcium phosphate ceramics in bone tissue engineering: a review of properties and their influence on cell behavior. Acta Biomater. 9, 8037–8045. doi: 10.1016/j.actbio.2013.06.014
Schwitalla, A. D., Abou-Emara, M., Spintig, T., Lackmann, J., and Muller, W. D. (2015). Finite element analysis of the biomechanical effects of PEEK dental implants on the peri-implant bone. J. Biomech. 48, 1–7. doi: 10.1016/j.jbiomech.2014.11.017
Shimizu, T., Fujibayashi, S., Yamaguchi, S., Yamamoto, K., Otsuki, B., Takemoto, M., et al. (2016). Bioactivity of sol-gel-derived TiO2 coating on polyetheretherketone: in vitro and in vivo studies. Acta Biomater. 35, 305–317. doi: 10.1016/j.actbio.2016.02.007
Shuai, C., Peng, S., Wu, P., Gao, C., Huang, W., Deng, Y., et al. (2016). A nano-sandwich construct built with graphene nanosheets and carbon nanotubes enhances mechanical properties of hydroxyapatiteandndash;polyetheretherketone scaffolds. Int. J. Nanomedicine 11, 3487–3500. doi: 10.2147/IJN.S110920
Sikder, P., Ferreira, J. A., Fakhrabadi, E. A., Kantorski, K. Z., Liberatore, M. W., Bottino, M. C., et al. (2020). Bioactive amorphous magnesium phosphate-polyetheretherketone composite filaments for 3D printing. Dent. Mater. 36, 865–883. doi: 10.1016/j.dental.2020.04.008
Spece, H., Yu, T., Law, A. W., Marcolongo, M., and Kurtz, S. M. (2020). 3D printed porous PEEK created via fused filament fabrication for osteoconductive orthopaedic surfaces. J. Mech. Behav. Biomed. Mater. 109:103850. doi: 10.1016/j.jmbbm.2020.103850
Stegen, S., van Gastel, N., and Carmeliet, G. (2015). Bringing new life to damaged bone: the importance of angiogenesis in bone repair and regeneration. Bone 70, 19–27. doi: 10.1016/j.bone.2014.09.017
Sun, Z., Ouyang, L., Ma, X., Qiao, Y., and Liu, X. (2018). Controllable and durable release of BMP-2-loaded 3D porous sulfonated polyetheretherketone (PEEK) for osteogenic activity enhancement. Colloids Surf. B Biointerfaces 171, 668–674. doi: 10.1016/j.colsurfb.2018.08.012
Sunarso, T. A., Toita, R., Tsuru, K., and Ishikawa, K. (2019). Enhanced osseointegration capability of poly(ether ether ketone) via combined phosphate and calcium surface-functionalization. Int. J. Mol. Sci. 21:198. doi: 10.3390/ijms21010198
Suska, F., Omar, O., Emanuelsson, L., Taylor, M., Gruner, P., Kinbrum, A., et al. (2014). Enhancement of CRF-PEEK osseointegration by plasma-sprayed hydroxyapatite: a rabbit model. J. Biomater. Appl. 29, 234–242. doi: 10.1177/0885328214521669
Swaminathan, P. D., Uddin, M. N., Wooley, P., and Asmatulu, R. (2020). Fabrication and biological analysis of highly porous PEEK bionanocomposites incorporated with carbon and hydroxyapatite nanoparticles for biological applications. Molecules 25:3572. doi: 10.3390/molecules25163572
Tang, X., Dai, J., Sun, H., Nabanita, S., Petr, S., Wang, D., et al. (2019). Mechanical strength, surface properties, cytocompatibility and antibacterial activity of nano zinc-magnesium silicate/polyetheretherketone biocomposites. J. Nanosci. Nanotechnol. 19, 7615–7623. doi: 10.1166/jnn.2019.16727
Torstrick, F. B., Evans, N. T., Stevens, H. Y., Gall, K., and Guldberg, R. E. (2016). Do surface porosity and pore size influence mechanical properties and cellular response to PEEK? Clin. Orthop. Relat. Res. 474, 2373–2383. doi: 10.1007/s11999-016-4833-0
Torstrick, F. B., Lin, A. S. P., Potter, D., Safranski, D. L., Sulchek, T. A., Gall, K., et al. (2018). Porous PEEK improves the bone-implant interface compared to plasma-sprayed titanium coating on PEEK. Biomaterials 185, 106–116. doi: 10.1016/j.biomaterials.2018.09.009
Torstrick, F. B., Lin, A. S. P., Safranski, D. L., Potter, D., Sulchek, T., Lee, C. S. D., et al. (2020). Effects of surface topography and chemistry on polyether-ether-ketone (PEEK) and titanium osseointegration. Spine 45, E417–E424. doi: 10.1097/BRS.0000000000003303
Uddin, M. N., Dhanasekaran, P. S., and Asmatulu, R. (2019). Mechanical properties of highly porous PEEK bionanocomposites incorporated with carbon and hydroxyapatite nanoparticles for scaffold applications. Progress Biomater. 8, 211–221. doi: 10.1007/s40204-019-00123-1
Ur Rehman, M. A., Bastan, F. E., Nawaz, Q., Goldmann, W. H., Maqbool, M., Virtanen, S., et al. (2018). Electrophoretic deposition of lawsone loaded bioactive glass (BG)/chitosan composite on polyetheretherketone (PEEK)/BG layers as antibacterial and bioactive coating. J. Biomed. Mater. Res. Part A 106, 3111–3122. doi: 10.1002/jbm.a.36506
Ur Rehman, M. A., Ferraris, S., Goldmann, W. H., Perero, S., Bastan, F. E., Nawaz, Q., et al. (2017). Antibacterial and bioactive coatings based on radio frequency co-sputtering of silver nanocluster-silica coatings on PEEK/bioactive glass layers obtained by electrophoretic deposition. ACS Appl. Mater. Interf. 9, 32489–32497. doi: 10.1021/acsami.7b08646
Vaezi, M., Black, C., Gibbs, D. M., Oreffo, R. O., Brady, M., Moshrefi-Torbati, M., et al. (2016). Characterization of new PEEK/HA composites with 3D HA network fabricated by extrusion freeforming. Molecules 21:687. doi: 10.3390/molecules21060687
Wakelin, E. A., Yeo, G. C., McKenzie, D. R., Bilek, M. M. M., and Weiss, A. S. (2018). Plasma ion implantation enabled bio-functionalization of PEEK improves osteoblastic activity. APL Bioeng 2:026109. doi: 10.1063/1.5010346
Walsh, W. R., Bertollo, N., Christou, C., Schaffner, D., and Mobbs, R. J. (2015). Plasma-sprayed titanium coating to polyetheretherketone improves the bone-implant interface. Spine J. 15, 1041–1049. doi: 10.1016/j.spinee.2014.12.018
Wan, T., Jiao, Z., Guo, M., Wang, Z., Wan, Y., Lin, K., et al. (2020). Gaseous sulfur trioxide induced controllable sulfonation promoting biomineralization and osseointegration of polyetheretherketone implants. Bioact. Mater. 5, 1004–1017. doi: 10.1016/j.bioactmat.2020.06.011
Wang, C., Wang, S., Yang, Y., Jiang, Z., Deng, Y., Song, S., et al. (2018). Bioinspired, biocompatible and peptide-decorated silk fibroin coatings for enhanced osteogenesis of bioinert implant. J. Biomater. Sci. Polym. Ed. 29, 1595–1611. doi: 10.1080/09205063.2018.1477316
Wang, H., Xu, M., Zhang, W., Kwok, D. T., Jiang, J., Wu, Z., et al. (2010). Mechanical and biological characteristics of diamond-like carbon coated poly aryl-ether-ether-ketone. Biomaterials 31, 8181–8187. doi: 10.1016/j.biomaterials.2010.07.054
Wang, M., Bhardwaj, G., and Webster, T. J. (2017). Antibacterial properties of PEKK for orthopedic applications. Int. J. Nanomedicine 12, 6471–6476. doi: 10.2147/IJN.S134983
Wang, Q., Mejía Jaramillo, A., Pavon, J. J., and Webster, T. J. (2016). Red selenium nanoparticles and gray selenium nanorods as antibacterial coatings for PEEK medical devices. J. Biomed. Mater. Res. Part B Appl. Biomater. 104, 1352–1358. doi: 10.1002/jbm.b.33479
Wang, S., Yang, Y., Li, Y., Shi, J., Zhou, J., Zhang, L., et al. (2019). Strontium/adiponectin co-decoration modulates the osteogenic activity of nano-morphologic polyetheretherketone implant. Colloids Surf. B Biointerfaces 176, 38–46. doi: 10.1016/j.colsurfb.2018.12.056
Wang, X., Lu, T., Wen, J., Xu, L., Zeng, D., Wu, Q., et al. (2016). Selective responses of human gingival fibroblasts and bacteria on carbon fiber reinforced polyetheretherketone with multilevel nanostructured TiO2. Biomaterials 83, 207–218. doi: 10.1016/j.biomaterials.2016.01.001
Wen, J., Lu, T., Wang, X., Xu, L., Wu, Q., Pan, H., et al. (2016). In vitro and in vivo evaluation of silicate-coated polyetheretherketone fabricated by electron beam evaporation. ACS Appl. Mater. Interfaces 8, 13197–13206. doi: 10.1021/acsami.5b10229
Wenz, L. M., Merritt, K., Brown, S. A., Moet, A., and Steffee, A. D. (1990). In vitro biocompatibility of polyetheretherketone and polysulfone composites. J. Biomed. Mater. Res. 24, 207–215. doi: 10.1002/jbm.820240207
Wong, K. L., Wong, C. T., Liu, W. C., Pan, H. B., Fong, M. K., Lam, W. M., et al. (2009). Mechanical properties and in vitro response of strontium-containing hydroxyapatite/polyetheretherketone composites. Biomaterials 30, 3810–3817. doi: 10.1016/j.biomaterials.2009.04.016
Wu, J., Li, L., Fu, C., Yang, F., Jiao, Z., Shi, X., et al. (2018). Micro-porous polyetheretherketone implants decorated with BMP-2 via phosphorylated gelatin coating for enhancing cell adhesion and osteogenic differentiation. Colloids Surf. B Biointerfaces 169, 233–241. doi: 10.1016/j.colsurfb.2018.05.027
Wu, X., Liu, X., Wei, J., Ma, J., Deng, F., and Wei, S. (2012). Nano-TiO2/PEEK bioactive composite as a bone substitute material: in vitro and in vivo studies. Int. J. Nanomedicine 7, 1215–1225. doi: 10.2147/IJN.S28101
Wu, Y., Woodbine, L., Carr, A. M., Pillai, A. R., Nokhodchi, A., and Maniruzzaman, M. (2020). 3D printed calcium phosphate cement (CPC) scaffolds for anti-cancer drug delivery. Pharmaceutics 12:1077. doi: 10.3390/pharmaceutics12111077
Xu, A., Liu, X., Gao, X., Deng, F., Deng, Y., and Wei, S. (2015). Enhancement of osteogenesis on micro/nano-topographical carbon fiber-reinforced polyetheretherketone-nanohydroxyapatite biocomposite. Mater. Sci. Eng. C Mater. Biol. Appl. 48, 592–598. doi: 10.1016/j.msec.2014.12.061
Xu, X., Li, Y., Wang, L., Li, Y., Pan, J., Fu, X., et al. (2019). Triple-functional polyetheretherketone surface with enhanced bacteriostasis and anti-inflammatory and osseointegrative properties for implant application. Biomaterials 212, 98–114. doi: 10.1016/j.biomaterials.2019.05.014
Xue, Z., Wang, Z., Sun, A., Huang, J., Wu, W., Chen, M., et al. (2020). Rapid construction of polyetheretherketone (PEEK) biological implants incorporated with brushite (CaHPO4.2H2O) and antibiotics for anti-infection and enhanced osseointegration. Mater. Sci. Eng. C Mater. Biol. Appl. 111:110782. doi: 10.1016/j.msec.2020.110782
Yabutsuka, T., Fukushima, K., Hiruta, T., Takai, S., and Yao, T. (2017). Effect of pores formation process and oxygen plasma treatment to hydroxyapatite formation on bioactive PEEK prepared by incorporation of precursor of apatite. Mater. Sci. Eng. C 81, 349–358. doi: 10.1016/j.msec.2017.07.017
Yabutsuka, T., Fukushima, K., Hiruta, T., Takai, S., and Yao, T. (2018). Fabrication of Bioactive Fiber-reinforced PEEK and MXD6 by Incorporation of Precursor of Apatite. J. Biomed. Mater. Res. Part B Appl. Biomater. 106, 2254–2265. doi: 10.1002/jbm.b.34025
Yan, J., Zhou, W., Jia, Z., Xiong, P., Li, Y., Wang, P., et al. (2018). Endowing polyetheretherketone with synergistic bactericidal effects and improved osteogenic ability. Acta Biomater. 79, 216–229. doi: 10.1016/j.actbio.2018.08.037
Yan, J. H., Wang, C. H., Li, K. W., Zhang, Q., Yang, M., Di-Wu, W. L., et al. (2018). Enhancement of surface bioactivity on carbon fiber-reinforced polyether ether ketone via graphene modification. Int. J. Nanomedicine 13, 3425–3440. doi: 10.2147/IJN.S160030
Yang, X., Wu, Y., Wei, K., Fang, W., and Sun, H. (2018). Non-isothermal crystallization kinetics of short glass fiber reinforced poly (Ether Ether Ketone) composites. Materials 11:2094. doi: 10.3390/ma11112094
Yin, J., Han, Q., Zhang, J., Liu, Y., Gan, X., Xie, K., et al. (2020). MXene-based hydrogels endow polyetheretherketone with effective osteogenicity and combined treatment of osteosarcoma and bacterial infection. ACS Appl. Mater. Interfaces 12, 45891–45903. doi: 10.1021/acsami.0c14752
Yoshikawa, H., and Myoui, A. (2005). Bone tissue engineering with porous hydroxyapatite ceramics. J. Artif. Organs 8, 131–136. doi: 10.1007/s10047-005-0292-1
Yu, W., Zhang, H., A, L., Yang, S., Zhang, J., Wang, H., et al. (2020). Enhanced bioactivity and osteogenic property of carbon fiber reinforced polyetheretherketone composites modified with amino groups. Colloids Surf. B Biointerfaces 193:111098. doi: 10.1016/j.colsurfb.2020.111098
Yu, X., Ibrahim, M., Liu, Z., Yang, H., Tan, L., and Yang, K. (2018). Biofunctional Mg coating on PEEK for improving bioactivity. Bioact. Mater. 3, 139–143. doi: 10.1016/j.bioactmat.2018.01.007
Yuan, B., Cheng, Q., Zhao, R., Zhu, X., Yang, X., Yang, X., et al. (2018). Comparison of osteointegration property between PEKK and PEEK: Effects of surface structure and chemistry. Biomaterials 170, 116–126. doi: 10.1016/j.biomaterials.2018.04.014
Yuan, X., Ouyang, L., Luo, Y., Sun, Z., Yang, C., Wang, J., et al. (2019). Multifunctional sulfonated polyetheretherketone coating with beta-defensin-14 for yielding durable and broad-spectrum antibacterial activity and osseointegration. Acta Biomater. 86, 323–337. doi: 10.1016/j.actbio.2019.01.016
Zhang, J., Cai, L., Wang, T., Tang, S., Li, Q., Tang, T., et al. (2018a). Lithium doped silica nanospheres/poly(dopamine) composite coating on polyetheretherketone to stimulate cell responses, improve bone formation and osseointegration. Nanomedicine 14, 965–976. doi: 10.1016/j.nano.2018.01.017
Zhang, J., Wei, W., Yang, L., Pan, Y., Wang, X., Wang, T., et al. (2018b). Stimulation of cell responses and bone ingrowth into macro-microporous implants of nano-bioglass/polyetheretherketone composite and enhanced antibacterial activity by release of hinokitiol. Colloids Surf. B Biointerfaces 164, 347–357. doi: 10.1016/j.colsurfb.2018.01.058
Zhao, G., Schwartz, Z., Wieland, M., Rupp, F., Geis-Gerstorfer, J., Cochran, D. L., et al. (2005). High surface energy enhances cell response to titanium substrate microstructure. J. Biomed. Mater. Res. A 74, 49–58. doi: 10.1002/jbm.a.30320
Zhao, Y., Wong, H. M., Lui, S. C., Chong, E. Y., Wu, G., Zhao, X., et al. (2016). Plasma surface functionalized polyetheretherketone for enhanced osseo-integration at bone-implant interface. ACS Appl. Mater. Interfaces 8, 3901–3911. doi: 10.1021/acsami.5b10881
Keywords: polyetheretherketone, surface modification, implants, bioactivity, osseointegration
Citation: Gu X, Sun X, Sun Y, Wang J, Liu Y, Yu K, Wang Y and Zhou Y (2021) Bioinspired Modifications of PEEK Implants for Bone Tissue Engineering. Front. Bioeng. Biotechnol. 8:631616. doi: 10.3389/fbioe.2020.631616
Received: 20 November 2020; Accepted: 10 December 2020;
Published: 12 January 2021.
Edited by:
Jianxun Ding, Chinese Academy of Sciences, ChinaReviewed by:
Yunhe Zhang, Jilin University, ChinaCopyright © 2021 Gu, Sun, Sun, Wang, Liu, Yu, Wang and Zhou. This is an open-access article distributed under the terms of the Creative Commons Attribution License (CC BY). The use, distribution or reproduction in other forums is permitted, provided the original author(s) and the copyright owner(s) are credited and that the original publication in this journal is cited, in accordance with accepted academic practice. No use, distribution or reproduction is permitted which does not comply with these terms.
*Correspondence: Yanmin Zhou, emhvdXltQGpsdS5lZHUuY24=
Disclaimer: All claims expressed in this article are solely those of the authors and do not necessarily represent those of their affiliated organizations, or those of the publisher, the editors and the reviewers. Any product that may be evaluated in this article or claim that may be made by its manufacturer is not guaranteed or endorsed by the publisher.
Research integrity at Frontiers
Learn more about the work of our research integrity team to safeguard the quality of each article we publish.