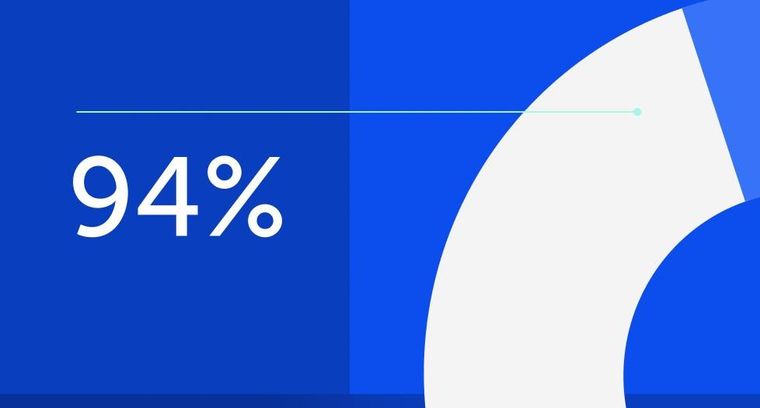
94% of researchers rate our articles as excellent or good
Learn more about the work of our research integrity team to safeguard the quality of each article we publish.
Find out more
REVIEW article
Front. Bioeng. Biotechnol., 14 December 2020
Sec. Tissue Engineering and Regenerative Medicine
Volume 8 - 2020 | https://doi.org/10.3389/fbioe.2020.598607
This article is part of the Research TopicExtracellular Vesicle Treatment, Epigenetic Modification and Cell Reprogramming to Promote Bone and Cartilage RegenerationView all 7 articles
Bone is a dynamic organ with high regenerative potential and provides essential biological functions in the body, such as providing body mobility and protection of internal organs, regulating hematopoietic cell homeostasis, and serving as important mineral reservoir. Bone defects, which can be caused by trauma, cancer and bone disorders, pose formidable public health burdens. Even though autologous bone grafts, allografts, or xenografts have been used clinically, repairing large bone defects remains as a significant clinical challenge. Bone tissue engineering (BTE) emerged as a promising solution to overcome the limitations of autografts and allografts. Ideal bone tissue engineering is to induce bone regeneration through the synergistic integration of biomaterial scaffolds, bone progenitor cells, and bone-forming factors. Successful stem cell-based BTE requires a combination of abundant mesenchymal progenitors with osteogenic potential, suitable biofactors to drive osteogenic differentiation, and cell-friendly scaffold biomaterials. Thus, the crux of BTE lies within the use of cell-friendly biomaterials as scaffolds to overcome extensive bone defects. In this review, we focus on the biocompatibility and cell-friendly features of commonly used scaffold materials, including inorganic compound-based ceramics, natural polymers, synthetic polymers, decellularized extracellular matrix, and in many cases, composite scaffolds using the above existing biomaterials. It is conceivable that combinations of bioactive materials, progenitor cells, growth factors, functionalization techniques, and biomimetic scaffold designs, along with 3D bioprinting technology, will unleash a new era of complex BTE scaffolds tailored to patient-specific applications.
Considered as one of a few organs with high regenerative potential, bone is a dynamic form of connective tissue with complex cellular composition and highly specialized organic-inorganic architecture (Perez et al., 2018). Bone provides essential biological functions in the body, such as providing body mobility and protection of internal organs, regulating hematopoietic cell homeostasis, and serving as important mineral reservoir (Perez et al., 2018; Iaquinta et al., 2019). Repairing large bone defects represents a challenge for musculoskeletal surgeons. Each year, millions suffer from a decreased quality of life as a result of segmental bone defects caused by trauma, tumors, and other musculoskeletal pathologies (Dimitriou et al., 2011). Despite its remarkable remodeling potential of small defects, human bone tissue is unable to bridge and heal large segmental defects. Grafting materials are frequently required in segmental defects. In fact, autologous bone graft has been the gold standard for reconstruction of large bony deficiencies (Herford et al., 2011). However, use of autologous bone graft has several limitations, including donor site morbidity, its finite amount and limitation in terms of shape and structure. Allografts and xenografts may be the first alternatives to autografts, but they have the risk of transmission of infectious agents. Allografts may also be slow to incorporate or can even be rejected (Campana et al., 2014).
Bone tissue engineering (BTE) emerged as a promising solution to overcome the limitations of auto- and allografts. BTE combines concepts from bone biology and engineering techniques to design scaffolds enriched with stem cells to repair large bony defects (Bishop et al., 2017; Coalson et al., 2019; Qasim et al., 2019; Karakuzu-Ikizler et al., 2020; Wang et al., 2020a). However, bone tissue engineering practices have yet to be realized in clinical practice due to numerous limitations or lack of efficacy. Ideal bone tissue engineering is to induce bone regeneration through the synergistic integration of biomaterial scaffolds, bone progenitor cells, and bone-forming factors (Amini et al., 2012; Perez et al., 2018; Iaquinta et al., 2019). Thus, successful stem cell-based BTE would require a combination of abundant mesenchymal progenitors with osteogenic potential, suitable biofactors to drive osteogenic differentiation, and cell-friendly scaffold biomaterials (Bishop et al., 2017; Yan et al., 2018; Budsamongkol et al., 2019; Coalson et al., 2019; Mostafa et al., 2019; Qasim et al., 2019; Zhang et al., 2019; Pakvasa et al., 2021; Wang et al., 2020a).
Biomaterials represent the basic components of the scaffolds and serve a critical function in BTE. An ideal scaffold material should be biocompatible, biodegradable, osteoconductive, osteoinductive, and possess favorable mechanical properties (Babilotte et al., 2019; Lin Y. et al., 2019). Biomaterials used as scaffolds in BTE can be divided into the following categories: Ceramics, natural/synthetic polymers and decellularized extracellular matrix (dECM) or composites of the above (Kim et al., 2019; Rustom et al., 2019; Udomluck et al., 2019; Rothrauff and Tuan, 2020). The purpose of this review is to summarize the properties of these basic biomaterials used in BTE and their utilization in recent studies. We also discuss novel three-dimensional (3D) bioprinting technologies, the newest methods of scaffold fabrication, and 3D bioprinting applications in bone tissue engineering.
Ceramics are non-metallic inorganic compounds with a high level of stiffness and widely used as bone replacing biomaterials (Kaur et al., 2019). Ceramics can be divided into two categories: bioinert and bioactive. The bioinert ceramics, such as alumina and zirconia, induce immunoreaction and can form a thin fibrous layer at the bone interface (Ercal and Pekozer, 2020). Therefore, bioinert ceramics are not considered a usable material for bridging bone defects. On the other hand, bioactive ceramics have the ability of bone-bonding, also known as osteoconductivity. The main bioactive ceramics used as bone substitutes include hydroxyapatite (HA), β-tricalcium phosphate (β-TCP), biphasic calcium phosphate (BCP), whitlockite (WH), octacalcium phosphate (OCP), and bioglass (BG) (Table 1).
HA is biochemically similar to the inorganic matrix of human bone, and is the most stable form of calcium phosphate due to its Ca/P molar ratio of 1.67 (Tuukkanen and Nakamura, 2017). HA is one of the most widely used biomaterials for both research and clinical applications (Shue et al., 2012). Its ideal biocompatibility is related to the ability to chemically bond bone. HA can be dissolved in body fluids and do not incite inflammatory reactions when applied clinically (Patel et al., 2002). HA biocompatibility is improved by promoting the osteogenic differentiation or the proliferation of mesenchymal stem cells (MSCs) and osteoblasts (Douglas et al., 2009). Studies have shown that HA is osteoconductive and osteoinductive (Xiao et al., 2020). Osteoconduction is the ability to promote bone growth on the surface of materials, whereas osteoinduction is the ability to induce progenitor cells into osteoblasts (Ambard and Mueninghoff, 2006). These bioactive characteristics are the result of the release of calcium and phosphate ions from the HA scaffold, which up-regulates the differentiation of osteoblasts, promotes bone formation through calcification, and increases osteoblastic differentiation gene markers (Jeong et al., 2019). Scaffolds composed of HA have no toxic effects on MSCs in vitro, and exhibit good biocompatibility and extensive osteoconductivity in rabbits in vivo, whereas the combination of MSCs with the scaffolds dramatically increased new bone formation (Wang et al., 2007).
Despite HA's many favorable characteristics as a bone biomaterial, it has some shortcomings including low degradability and poor mechanical properties (Siddiqui et al., 2018). As HA is one of the most stable forms of calcium phosphate, HA has been shown to degrade very slowly, even considered to be non-degradable (Nakamura et al., 2013). Its poor mechanical properties include low tensile strength and toughness, precluding its usage for high load-bearing applications (Siddiqui et al., 2018). As a result of its non-degradability and poor mechanical properties, HA is not used as a scaffold by itself. Instead, HA is used mainly in implant coating (Gustavsson et al., 2012). When used as an implant coating, HA improves osteoblast activity and increases the bony contact area (Darimont et al., 2002). Thus, in compound scaffolds HA is often mixed with polymers or tricalcium phosphate (TCP) to improve its degradability and mechanical properties (Dhivya et al., 2015).
TCP is another calcium phosphate biomaterial widely used in BTE. There are two available forms of TCP: α-TCP and β-TCP, both of which have a Ca/P molar ratio of 1.5 (Kim et al., 2017a,b). α-TCP has a monoclinic crystal structure while β-TCP has a rhombohedral crystal structure (Singh et al., 2016). Both can change into the other form at different temperatures (Horch et al., 2006). However, β-TCP is more widely used than α-TCP, because it is more stable and has a higher biodegradation rate (Kim et al., 2014). In addition, β-TCP has been shown to exhibit good biocompatibility in both in vivo and in vitro studies (Horch et al., 2006). β-TCP can bond to the host bone directly (Neo et al., 1993) and it increases the proliferation of osteoblasts and BMSCs (Yao et al., 2004). Furthermore, it promotes osteogenic differentiation of BMSCs (Neamat et al., 2009). Kamitakahara et al. also found that porous β-TCP scaffolds increase cell proliferation and adhesion when used in bone regeneration (Kamitakahara et al., 2008).
In recent years, β-TCP has been shown to have good osteoconductive and osteoinductive activity (Ogose et al., 2002). β-TCP can promote mineralization through calcium and phosphate ion release after degradation (Luginbuehl et al., 2010) and β-TCP scaffolds combined with bone morphogenetic proteins (BMP) can increase bone regeneration (Urist et al., 1987). Yuan et al. also reported new bone formation in the β-TCP scaffolds when implanted in dog muscles (Yuan et al., 2001).
Compared to HA, β-TCP is less stable but has a higher biodegradability, which allows newly formed bone to replace the β-TCP scaffold (Lu et al., 2019). β-TCP has two mechanisms of biodegradation: osteoclast-mediated and biological fluid-mediated biodegradation (Shue et al., 2012). However, the biodegradation rate of the β-TCP scaffolds is too fast to be compensated for by newly formed bone. Moreover, β-TCP scaffolds are brittle with a very low tensile strength (Ercal and Pekozer, 2020). Therefore, in order to counteract these drawbacks, other biomaterials are often mixed with β-TCP.
The term BCP was first introduced in 1985 (Tortelli and Cancedda, 2009). BCP was considered a mixture of HA and β-TCP, although studies have shown that BCP is its own substance entirely rather than a combination with each of its component homogeneously mixed at the submicron level (Dorozhkin, 2012). BCP combines the advantages of HA and β-TCP, the biodegradability of β-TCP, and the biocompatibility, osteoconductivity and osteoinductivity of both (Bouler et al., 2017). The biodegradability of BCP depends on the HA/β-TCP ratio. A lower ratio results in a higher level of biodegradability (Bouler et al., 2017). Therefore, we can fabricate BCP with different levels of biodegradability by adjusting the HA/β-TCP ratio. As a result, there are over 30 commercially-used BCP bone substitution for various orthopedic applications (Ebrahimi et al., 2017).
Multiple studies demonstrated BCP's biocompatibility with osteoconductive and osteoinductive properties (Cordonnier et al., 2010). Cordonnier et al. found that MSCs adhered to BCP biomaterial and proliferated rapidly (Cordonnier et al., 2010). Li et al.'s study showed that BCP granules have good biocompatibility and can promote BMSCs adhesion, spread and proliferation (Li et al., 2019). Other studies reported that the osteoconductivity of BCP is most potent when its size ranges from 40 to 1,500 μm, and that BCP particles of this size could repair bone defects in animal models (Fellah et al., 2006). Li et al. found that porous BCP ceramic could up-regulate the osteogenic gene expression and promote osteogenesis when intramuscularly implanted in dogs (Li et al., 2019). Our previous study also showed that BCP scaffolds provide a cell-friendly environment to support the survival, propagation, and ultimately differentiation of BMP9-expressing osteoblastic progenitor cells (Shui et al., 2014).
Whitlockite (WH) is a calcium phosphate derivative containing magnesium ions in its lattice (Wang et al., 2020b). The Ca/P ratio of WH is 1.43 and it has a rhombohedral morphology similar to β-TCP (Jeong et al., 2019). Compared to HA and β-TCP, the biomechanical properties of WH are more favorable, especially its compressive strength (Jang et al., 2016; Cheng et al., 2018). WH is the second most abundant component in human bone occupying ~20% of bone mineral by weight (Kim et al., 2017b). WH is relatively rare in nature, indicating it is difficult to synthesize a pure phase of WH in a physiological system (Kim et al., 2017b; Cheng et al., 2018). Recently, studies found that WH could be easily synthesized in low-temperature conditions with useful characteristics as a scaffold in BTE (Jang et al., 2015; Kim et al., 2017b; Cheng et al., 2018).
It has been shown that the number of pits induced by osteoclasts on WH scaffold was two times higher than the number of pits induced by osteoclasts on an HA scaffold under the same conditions, rendering WH more biodegradable than HA (Kim et al., 2017b). In addition, WH has a higher solubility than HA in physiological conditions with a continuous release of magnesium and phosphate ions, enhancing the osteogenic activity of stem cells (Kim et al., 2017b; Cheng et al., 2018). When MSCs are cultured in HA/WH scaffolds with different ratios, cellular viability and proliferation were both higher than when they were cultured in HA alone (Cheng et al., 2018), although it was also reported that the cell viability of murine MSCs seeded across either WH or HA scaffolds was equal between the two scaffold environments (Jang et al., 2016). WH has been shown to poss superior osteoconductivity and osteoinductivity as human MSCs cultured on WH scaffolds exhibited significantly increased osteoblast-related gene expression compared to MSCs cultured on an HA scaffold, and WH-embedded cryogel had a higher mineral deposition compared to HA-embedded cryogel (Kim et al., 2017b). Nonetheless, HA and WH may have a synergistic effect on the promotion of growth and osteogenic activity of MSCs (Cheng et al., 2018). Thus, WH is expected to contribute to future research in BTE as a biomaterial due to its high osteogenic activity.
Octacalcium Phosphate (OCP) is a calcium phosphate material and the precursor phase of HA formation. The Ca/P molar ratios of stoichiometric OCP is 1.33 (Yanagisawa et al., 2020). OCP has certain bone regenerative properties superior to HA including osteoinductivity, osteoconductivity, and biodegradability (Yanagisawa et al., 2020). Although OCP has many suitable properties as a bone substitute, its innate brittleness results in difficulty in sustaining its shape and therefore limits its clinical applications. Nonetheless, OCP combined with natural polymers is explored to treat bone defects (Suzuki et al., 2020).
Similar to other biocompatible calcium phosphate materials, the main components of OCP are calcium and phosphate ions (Wang et al., 2015). Bigi et al. found that when human primary osteoblasts were cultured on OCP coatings, the cells showed normal morphology with high rate of proliferation and viability (Bigi et al., 2005). Shelton et al. showed that when rat BMSCs were cultured on OCP scaffolds, cell number increased 40-fold after 20 days (Shelton et al., 2006). OCP is a bioresorbable ceramic and will degrade gradually in a physiological environment (Wang et al., 2015). OCP in a granule form degraded more rapidly than HA when implanted in the subperiosteal area of mouse calvaria (Kikawa et al., 2009). Moreover, recent studies demonstrated that the biodegradability of OCP occurred through osteoclastic cellular resorption, which in turn was enhanced by the intrinsic properties of OCP (Bigi et al., 2005; Suzuki, 2010).
OCP has better osteoconductivity and osteoinductivity than both HA and β-TCP (Kamakura et al., 1999). Calcium and phosphate ions are diffused from the surface of OCP during OCP–HA conversion (Suzuki, 2010). It has been shown that high concentrations of calcium and phosphate ions regulated osteoblastic cell differentiation (Dvorak et al., 2004), as it was reported that when mouse BMSCs cultured on a plate coated with OCP or HA, OCP was superior in inducing osteoblastic differentiation in a dose-dependent manner (Anada et al., 2008), and that OCP granules were able to repair critical-sized bone defects (Honda et al., 2009).
BG is a form of bioactive ceramic widely used as scaffolds in BTE. The first form of BG (45S5) was created by Larry Hench in 1969 (Baino et al., 2018). Initially, Hench and his coworkers designed different glass formulations based on the SiO2-Na2O–CaO–P2O5 oxide system, and found that the composition 45SiO2-24.5Na2O−24.5CaO−6P2O5 (wt %) made the surface of the material reactive in physiological environments (Wilson et al., 1981), which was thus designated as 45S5. The 45S5 BG is the first example of the third generation biomaterial, which can induce genetic activation of specific cell pathways due to its released ionic dissolution products (Xynos et al., 2000). Over the past 40 years, many new types of BG have been proposed for use in specific BTE clinical applications (Rottensteiner-Brandl et al., 2018). In fact, from 1985 to 2016 the 45S5 BG-based scaffolds have been implanted in approximately 1.5 million patients to repair bone and dental defects (Baino et al., 2018).
The overall mechanism underlying BG degradation involves ion release and a surface reaction. First, sodium ions in the BG and hydrogen ions in the environment exchange and breakdown Si-O-Si bonds, leading to the formation of Si-OH bonds on the surface of the BG. The Si-OH bonds condense and form a SiO2 layer on the BG surface. The calcium and phosphate ions then move to the top of the newly formed SiO2 layer to form a new calcium-phosphate-rich layer (Piattelli et al., 2000). Lastly, hydroxyl carbonate apatite crystals form in the calcium-phosphate-rich layer, which is stoichiometrically equivalent to human bone (Johnson et al., 1997; Piattelli et al., 2000).
BG scaffolds exhibit good biocompatibility (Yang et al., 2014a) as osteoblast and multinucleated cells were shown to proliferate around BG particles implanted in a rabbit bone defect for 4 weeks, and mature bone was formed around the BG particles, and no gaps were seen at the bone-BG interface (Piattelli et al., 2000). It was reported that chitosan/BG 3D porous scaffolds exhibited good biocompatibility with high cell proliferation rates, and potential for BTE (Yang et al., 2014a). It has been shown that BG exhibited increased hemostasis, excellent handling properties, and tissue response with no adverse cellular reactions (Johnson et al., 1997). Furthermore, BG is osteoconductive, forming a stable bond to living bone, as well as osteoinductive, stimulating a path of regeneration and self-repair (Kargozar et al., 2018). Thus, BG has been used to augment bony defects at sites of dental implants (Johnson et al., 1997) and functions as both a filler and a coating material for implants in bone cavities (Turunen et al., 1997).
Polymeric materials are easier to fabricate and mold into the desired shape and size (Hosseinpour et al., 2017). Natural polymers are intrinsically biocompatible and biodegradable and hence readily suited for tissue engineering applications, and in fact many kinds of polymeric materials are widely used in BTE (Müllner, 2019). Natural polymers commonly used in BTE include chitosan, collagen, gelatin, silk, alginate, and hyaluronic acid (Table 2) (Murphy et al., 2013; Ramesh et al., 2018). Synthetic polymers have increased longevity compared to natural polymers in terms of shelf life. Furthermore, they can be processed and fabricated in order to meet specific mechanical requirements. A number of synthetic polymers have been employed for BTE, including poly (lactic acid) (PLA), poly (glycolic acid) (PGA), poly (lactic-coglycolic acid) (PLGA), poly-ε-caprolactone (PCL), and poly(polyethylene glycol citrate-coN-isopropylacrylamide) (PPCN) (Table 3) (Murphy et al., 2013; Ramesh et al., 2018).
Chitosan is mainly extracted from crustaceans (shrimps, crabs, lobsters, etc.), representing the most abundant natural amino polysaccharide and a versatile natural biopolymer formed by partial deacetylation of chitin under alkaline conditions (Younes and Rinaudo, 2015). Chitosan has the same structure as glycosaminoglycans, one of the components of the extracellular matrix critical for cell-cell adhesion. Thanks to its favorable properties including biocompatibility, biodegradability and anti-bacterial effects, chitosan-based scaffolds are used extensively in biomedical applications (LogithKumar et al., 2016; Balagangadharan et al., 2017; Preethi Soundarya et al., 2018). However, the mechanical strength of chitosan is low; therefore, other natural or synthetic polymers, metals and ceramics are added to chitosan to enhance its mechanical strength (Azzopardi et al., 2010). Nonetheless, chitosan can promote cell adhesion, proliferation, and differentiation (Jin et al., 2012; Dinescu et al., 2014). It was reported that, when adipose-derived MSCs were cultured on chitosan/silk nanofiber scaffolds, cell viability was enhanced (Chen et al., 2018), and that chitosan-based injectable and thermosensitive hydrogels improved the viability and proliferative activity of cultured mouse BMSCs at 7 days (Yan et al., 2010).
Chitosan is degraded into non-toxic absorbable small molecular amino acids and polysaccharides by chitosanolytic enzymes (Cheng et al., 2019). Chitosan modifications (e.g., covalent crosslinking and thiolation) can alter its degradation profile (Rottensteiner-Brandl et al., 2018). Chitosan scaffolds exhibit high cellular affinity for stem cells and promote stem cell osteogenesis as it was reported that chitosan-based nanofibrous scaffolds could mimic the extracellular matrix and recruit stem cells gathering to the scaffolds to facilitate seed cell adhesion, proliferation, and osteogenic differentiation in vitro (Cheng et al., 2014), and induced expression of osteogenic genes, ALP activity, and calcium deposition of stem cells (He et al., 2017). Chitosan not only promotes osteoblastogenesis but also reduces osteoclastogenesis as chitosan was shown to improve healing of fractures with greater trabecular bone formation in mice (Tan et al., 2014). We recently demonstrated that a pH-triggered, self-assembled, and bioprintable hybrid hydrogel scaffold composited with carboxymethyl chitosan and amorphous calcium phosphate exhibited excellent biocompatibility and promoted bone formation in vivo (Zhao et al., 2019). Chitosan has also been found to affect the activation of osteogenesis-related signaling pathways and to promote mineralization to facilitate bone regeneration via stimulation of trabecular bone production through the upregulation of the Runx2/osteocalcin/ALP signaling pathway in C57LB/6 mice (Ho et al., 2015).
Collagen is the most abundant and widely distributed protein, constituting more than one-third of body protein tissue by weight (Preethi Soundarya et al., 2018). Approximately 29 types of collagen have been identified thus far. Among these, types 1, 2, 3, 5, and 9 collagen are known to form fibers and type 1 collagen is the most prevalent type in the ECM, especially in bone (Ferreira et al., 2012). Collagen protein has a complex hierarchical conformation, which is divided into four structures and is composed of 25 different alpha chains with each alpha chain composed of repeating units of Gly-X-Y. The X and Y residues are proline or hydroxyproline. Hydroxyproline is unique to collagen as it constitutes more than 50% of the total amino acid content. The fibrils are the final quaternary structure of the collagen protein (Ferreira et al., 2012). Collagen has been among the most widely used biomaterials in BTE thanks to its abundance and excellent properties, including biocompatibility, low antigenicity, absorbability, and easy processing (Nocera et al., 2018; Busra and Lokanathan, 2019).
Collagen can be processed into different physical forms, including injectable hydrogels, membranes and films, sponges and scaffolds, as well as micro-and nanospheres using a wide range of fabrication technologies to aid in targeted delivery, biocompatibility, stem cell differentiation and osteogenesis (Hu et al., 2008; Mencía Castaño et al., 2019). The chitosan–collagen materials promoted cell spread and proliferation (Wang and Stegemann, 2010). Collagen has also been utilized in guided bone regeneration procedures due to their biodegradability, osteoconduction, and osteoinduction (Ferreira et al., 2012). Collagen scaffolds provide an ideal environment for bone formation (Wang et al., 2016). They also serve as the best carrier for targeted delivery of small molecules such as antagonists of miR-16, which increases the relative levels of Runx2 and osteocalcin, thereby promoting mineral calcium deposition (Mencía Castaño et al., 2019). 3D nanofiber collagen scaffolds were shown to promote the proliferation of MC3T3-E1 cells, the expression of osteogenesis genes, ALP activity, and calcified matrix formation (Lee and Kim, 2018). However, the poor mechanical properties and low stiffness of collagen limit its solo applications in BTE (Ferreira et al., 2012) despite its favorable biocompatibility, biodegradation, and osteogenic properties (Elango et al., 2016).
Gelatin is a product of collagen degradation and has the RGD (arginine, glycine, and aspartate) binding sequence. It is one of the most versatile naturally occurring biopolymers and widely used both individually and as a mixture in BTE owing to its ability to promote cell adhesion, differentiation, and proliferation (Mahmoudi Saber, 2019). As a natural origin polymer, gelatin holds several advantages over its precursor, collagen, such as low water solubility, one of the main limitations of collagen as a biomaterial (Kolesky et al., 2016; Echave et al., 2019). Gelatin can be enzymatically degraded into its respective amino acids and cause no harm to the body or cells (Mahmoudi Saber, 2019). In addition, gelatin can create poly-ionic complexes with different therapeutic factors such as proteins, growth factors, nucleotides, and polysaccharides (Echave et al., 2019).
The composite scaffold of HA/gelatin is potentially useful for hard-tissue regeneration (Kuttappan et al., 2016). A gelatin-siloxane scaffold showed ideal cytocompatibility, while also stimulating osteoblast proliferation and differentiation in vitro (Ren et al., 2002), while when siloxane-calcium silicate was modified by gelatin, it showed good biocompatibility and osteoconductivity, as well as robust bone formation capability as tested by implanting the scaffold into rat calvarial defects (Kuttappan et al., 2016). It was also reported that multi-sized porous β-TCP scaffolds coated with 3% gelatin under a vacuum displayed enhanced compressive strength as well as increased cell differentiation in comparison to their non-coated counterparts (Kim et al., 2012). Furthermore, gelatin-calcium phosphate nanofibers, composed of β-TCP nanoparticles incorporated into electropunk gelatin nanofibers, improved proliferation and osteogenic differentiation of rat MSCs compared to pure calcium phosphate nanofibers (Kuttappan et al., 2016). Lastly, gelatin is also an ideal carrier for proteins and various growth factors (Krishnan et al., 2015; Anjana et al., 2016). For example, Gelatin/HA fibrous scaffold with platelet-rich plasma gel was able to induce the differentiation of MSCs into an osteogenic cell line (Anjana et al., 2016).
Silk is a naturally occurring protein biopolymer produced by silkworms, bees, spiders, mites, and scorpions although the silk from each species is tailored to their specific evolutionary needs (Bhattacharjee et al., 2017). Regardless of source, silks are primarily composed of two types of proteins namely silk fibroin (fibrous) and sericin (globular). Silk fibroin makes up the fibrous part of the filament and is structurally composed of heavy chains and light chains, whereas sericin is a water-soluble, glue-like protein that coats the two chains of fibroin and constitutes about 25–30% of the weight of silk fibroin (Nguyen et al., 2019). Silk protein is a promising biomaterial in BTE owing to its excellent mechanical properties and biocompatibility (Midha et al., 2016). Silk can also be molded easily into various forms of scaffolds (Murab et al., 2013), hydrogels (Murab et al., 2015), and composites (Sun et al., 2012).
The biodegradability of silk biomaterial can be controlled by modulating its structures including chain length and secondary conformations (Bhattacharjee et al., 2017). Silk fiber processing solvents can affect the secondary structure and biodegradability of silk. Organic solvent-based silk takes a year or more to get completely absorbed in vivo (Zhang et al., 2009), while aqueous solvent-based silk only requires a maximum of 6 months to get completely absorbed in vivo (Li S. et al., 2018). This property is helpful in repairing critical bone defects as silk scaffolds can provide sufficient structural support until native bone is completely regenerated (Mandal and Kundu, 2009).
The products of silk degradation are glycine and alanine, which can be reused to synthesize new proteins in vivo (Bhattacharjee et al., 2017). Hence, silk biomaterials have excellent biocompatibility and a low risk of triggering immunogenic reactions (Bhattacharjee et al., 2017; Du et al., 2019; Sartika et al., 2020). A pure 3D silk scaffold that was made using a lyophilization method demonstrated superior biocompatibility in vitro (Sartika et al., 2020). Although the low osteogenic potential of silk limits its applications in BTE, in combination with other biomaterials, silk can enhance bone regeneration (Lee et al., 2017). When MSCs were cultured on a silk/HA scaffold, such scaffold showed an increase in ALP activity and mineralization after 12 days (Farokhi et al., 2018).
Alginate is the most abundant marine anionic biopolymer, which is produced industrially from brown seaweeds such as Laminaria hyperborea, Laminaria digitata, Laminaria japonica, Ascophyllum nodosum, and Macrocystis pyrifera. The primary source of alginate is from the cell walls and intracellular spaces of brown seaweed (Venkatesan et al., 2014). It is composed of guluronic acid (G) and mannuronic acid (M). The composition (G/M ratio), sequence, and molecular weight are critical factors affecting the physical properties of alginate (George and Abraham, 2006). The alginate molecules are necessary for plant growth in the sea because they provide the plant with both flexibility, and strength. The attractive properties of Alginate include biocompatibility, non-toxicity, non-immunity, and biodegradability in addition to its high abundance with a low cost. Chemical and physical modifications of alginate can fine tune its properties and functions, such as biodegradability, mechanical strength, gelation property, and cell affinity, toward respective applications. Furthermore, alginate can be easily formed into a variety of hydrogels, microspheres, microcapsules, sponges, foams, and fibers (Sharmila et al., 2020). Therefore, alginate and its composites can be prepared through various crosslinking methods and are widely used as a biomaterial in BTE, next to chitosan among the natural polysaccharides (Sharmila et al., 2020).
The biocompatibility of alginate scaffolds has been measured with various types of cells in vitro (Pravdyuk et al., 2013). When human MSCs were encapsulated in alginate microspheres and then cryopreserved, the viability of MSCs and their metabolic rates were maintained (Pravdyuk et al., 2013). Alginate-based scaffolds also showed favorable biocompatibility (Florczyk et al., 2011; Sharmila et al., 2020). For example, a porous chitosan-alginate scaffold was shown to enhanced cell proliferation (Florczyk et al., 2011).
Alginate scaffolds possess bone regeneration properties (Hwang et al., 2009; Kim et al., 2020), as embryonic stem cells encapsulated in alginate hydrogels cultured in a microgravity bioreactor, showed enhanced differentiation and osteogenesis (Hwang et al., 2009). HA mixed with alginate increased its mechanical strength, and the resulting scaffold possessed excellent osteogenic effects (Venkatesan et al., 2015). Injection of a thermosensitive alginate/β-TCP/aspirin scaffold with an interconnected porous microstructure into the periosteum of rat cranial bone promoted new bone formation (Fang et al., 2018). Furthermore, alginate was also used in combination with other biomaterials, such as collagen, gelatin, and bioglass, to increase its mechanical strength, cell adhesion, and osteogenic differentiation ability (Venkatesan et al., 2015).
Hyaluronic acid is a hydrophilic linear unbranched glycosaminoglycan made up of alternating residues of d-glucuronic acid and N-acetyl glucosamine with highly variable length and molecular weight (up to 106 Da) (Zhao et al., 2016). It can be isolated from the vitreous humor of cows, although it is found in almost all living organisms, mainly in the connective tissues and load bearing joints, etc. (Ramesh et al., 2018). Hyaluronic acid is the primary component of the extracellular matrix of connective tissues, and presented in high concentrations in the synovial joint fluids, vitreous of the eyes, hyaline cartilage, intervertebral disc nucleus, and umbilical cord (Hemshekhar et al., 2016). Hyaluronic acid plays important physiological roles, such as lubrication of arthritic joints, control of the viscoelastic properties of soft tissues and the motility, adhesion and organization of cells (Hemshekhar et al., 2016; Gokila et al., 2018). Hyaluronic acid is also involved in key signaling pathways and regulates cell proliferation, survival, motility, and differentiation (Toole, 2004). Furthermore, it has been shown that hyaluronic acid regulates inflammatory chemokines, metalloproteinases, and tissue inhibitors, which help form better scaffolds. Owing to its elasticity, biocompatibility, non-immunogenicity, water solubility, and osteoinductivity, hyaluronic acid is an excellent polymer of choice for BTE (Collins and Birkinshaw, 2013).
Hyaluronic acid scaffolds were shown to support the attachment and proliferation of MC3T3-E1 cells and could be degraded at acidic pH values or by hyaluronidase and reducing substances (Cui et al., 2015). The rapid enzymatic degradation of hyaluronic acid must be taken into account in vivo, potentially decreasing the mechanical properties of hyaluronic acid -based scaffolds (Drury and Mooney, 2003). Hyaluronic acid and gelatin (Gel) hydrogel loaded into a BCP ceramic created a new scaffold with highly interconnected porosity, with an average compressive strength of 2.8 ± 0.15 MPa, ideal as a cancellous bone substitute. This scaffold increased cell proliferation and ALP activity, and promoted new bone formation and mineralization, with delayed degradation within 3 months after implantation (Nguyen and Lee, 2014). Thus, hyaluronic acid has great potential as a scaffolding material in BTE.
Poly (lactic acid) (PLA) is a product of polyesterification reaction of lactic acid, characterized by several features fundamental for bone regeneration, such as non-toxicity, biocompatibility, thermal stability, and biodegradability (Gregor et al., 2017). PLA has been approved by the FDA as a hydrophobic aliphatic polyester in different biomedical and clinical applications as it degrades to the physiological product lactic acid (Tyler et al., 2016). The thermal stability and biodegradability of PLA are dependent on the choice and distribution of stereoisomers within the polymer chains as well as molecular weights (Gremare et al., 2018). Therefore, there are four different forms of PLA, including poly (L-lactic acid) (PLLA), poly (D-lactic acid) (PDLA), Poly (D,L-lactic acid) (PDLLA), and meso-poly(lactic acid), which result in PLA with a broad range of physiochemical properties (Ramot et al., 2016).
PLA was successfully printed in scaffolds with different pore sizes, sufficient mechanical integrity, and biodegradability, and BMSCs cultured on the scaffold, were not affected in terms of metabolic activity and cell viability (Gremare et al., 2018). Osteosarcoma cells were also tested and indicated the PLA scaffold was non-cytotoxic and promoted cell growth, cell viability, and osteogenic gene expression (Velioglu et al., 2019). PLA-based composite materials with other polymers or ceramics were shown osteoconductive and osteoinductive (Ramesh et al., 2018). PLA/HA biocomposites showed good biocompatibility and osteo-differentiation toward osteoblast precursors with the increase in cell numbers, cell adhesion, and promotion of ALP activity (Kim et al., 2006). When BMSCs were seeded on a bent nanofibrous mesh scaffold containing PLA and gelatin (in a 1:1 in weight ratio), the mesh promoted osteogenic differentiation of BMSC sheets compared to the control, and the blended biomaterial increased bone formation within rat cranial defects (Ren et al., 2017).
PGA is a semicrystalline polymer with a high tensile modulus and insoluble in most organic solvents. Owing to its high porosity, PGA allows diffusion of nutrients upon implantation and subsequent neovascularization (Ramesh et al., 2018). Moreover, PGA is easily handled and can be fabricated into different shapes (Asti and Gioglio, 2014). PGA was the first synthetic biodegradable polymer that was approved by the FDA for use in the production of absorbable sutures (Ramesh et al., 2018). However, PGA is absorbed rapidly (within 6 to 8 weeks), the degradation products, such as glycolic acid and other acidic products can evoke a strong inflammatory response, which limits its biomedical applications (Ulery et al., 2011). For this reason, PGA is not used alone but in the combination form of PLGA (Ceccarelli et al., 2017). Another disadvantage of PGA is its low mechanical strength. To overcome these drawbacks, PGA is usually used in a composite form with other biomaterials, such as HA (Dunne et al., 2010; Cojocaru et al., 2020). For example, Dunne et al. found that PGA/HA composite scaffolds had good biodegradability and the proper porosity required for cellular infiltration and attachment (Dunne et al., 2010). PGA-hyaluronan with high porosity was used in the three-dimensional culturing of meniscus cells, where the scaffold displayed good biocompatibility (Cojocaru et al., 2020).
PLGA is a linear copolymer that combines PLA and PGA, and is a widely used synthetic polymer material in BTE. It has favorable properties as a biomaterial in BTE, including biodegradability, biocompatibility, controllable mechanical properties, and easy processing (Boukari et al., 2017). PLGA has also been approved by the FDA for use in clinical applications as it mitigates the disadvantages of both PLA and PGA (Lü et al., 2009). One appealing property of PLGA is that its degradation rate is tunable, ranging from weeks to months, via a variation of the ratio of the two monomers (Donnaloja et al., 2020). For example, PLGA containing 75% PGA is amorphous and hydrolytically unstable, thus degrading faster. PLGA is easily broken down in vivo by hydrolysis into lactic acid and glycolic acid, both of which are non-toxic monomers physiologically metabolized by the tricarboxylic acid cycle for final excretion in the lungs (Essa et al., 2020). In fact, PLGA is one of the most widely used synthetic polymers for drug delivery systems and therapeutic biomolecules due to its favorable biological properties (Ortega-Oller et al., 2015). Nonetheless, its suboptimal mechanical properties, poor osteoinductivity, and poor cell adhesion limit its usage in BTE.
In order to overcome the above shortcomings in BTE, PLGA often combines with other biomaterials, such as ceramics like bioglass, HA, TCP and natural polymers (Boukari et al., 2017; Zhao et al., 2017; Bharadwaz and Jayasuriya, 2020; Probst et al., 2020). For example, PLGA/HA composite fibrous scaffolds were fabricated by the melt-spinning method, in which BMP-2 was incorporated with the help of polydopamine (Zhao et al., 2017). The enhanced roughness of PLGA/HA fibrous scaffolds due to the polydopamine coat caused increased hydrophilic property, cell adhesion and proliferation of cells. Moreover, the composite scaffold increased bone mineral deposition and ALP activity (Zhao et al., 2017). PLGA/chitosan scaffolds were shown to have better mechanical properties and a much steadier drug release (Boukari et al., 2017). When human MSCs were cultured on this composite scaffold, cell proliferation increased over time and the extent of mineralization increased after 21 days (Boukari et al., 2017).
PCL is one of the most commonly used synthetic polymers in BTE. PCL has easily manipulable mechanical properties, a high degree of crystallinity, and suitable properties such as non-toxicity, biocompatibility, and biodegradability, leading to its use as an implantable polymer material approved by the FDA (Atyabi et al., 2016; Sharifi et al., 2018). Though biodegradable, PCL, especially in its high molecular weight form, has a slow degradation rate, restricting its usage in BTE. In fact, it degrades at the slowest rate among all polyesters and may require 3 years to completely eliminate itself from the host body, either by microorganisms or by hydrolysis of its aliphatic ester linkage (Shive and Anderson, 1997), although a PCL-based composite scaffold showed a faster degradation rate, such as bioactive glass microspheres reinforced to PCL (Lei et al., 2012).
The poor hydrophilic nature of PCL affects the cell attachment, proliferation and differentiation, posting the most important challenge to the initial step of cell culturing (Sharifi et al., 2018). To increase the hydrophilicity of PCL, its surface can be modified using several methods, such as coating the scaffold with composites of silk, gelatin, growth factors, and proteoglycans (Dwivedi et al., 2020). For example, a porous nanofibrous scaffold composed of HA, silk, and PCL was blended via a one-step emulsion electrospinning, and the resulting product exhibited hydrophilicity while promoting human primary skin fibroblast proliferation and filopodia protrusion (Li et al., 2012). The PCL/Cobalt/HA composite membrane increased osteoblast cell proliferation and calcium deposition production, compared with PCL only (Lin W. C. et al., 2019).
PCL scaffolds were shown to promote bone regeneration (Faia-Torres et al., 2015; Kim and Kim, 2015; Dwivedi et al., 2020). It was shown that PCL scaffolds with a surface roughness of 0.9–2.1 μm promoted the osteogenic ability of the hMSCs seeded on it when cultured in a dexamethasone-deprived osteogenic induction medium (Faia-Torres et al., 2015). Highly roughened PCL surfaces on an apatite layer using oxygen plasma-etching led to improved cell viability and osteogenic ability of MC3T3-E1 cells when soaked in simulated body fluid (Kim and Kim, 2015).
Poly(N-isopropylacrylamide) (PNIPAAm) is a thermoresponsive hydrogel used to copolymerize and graft synthetic polymers in the biomedical field (Lanzalaco and Armelin, 2017). PNIPAAm is well-known for its water solubility and its lower critical solution temperature that falls close to body temperature (around 36.5–37.5°C) (Lanzalaco and Armelin, 2017). Furthermore, PNIPAAm is highly biocompatible with animal cells (Ohya et al., 2004; Lanzalaco and Armelin, 2017). It was reported that the PNIPAAm/gelatin scaffold in the subcutaneous tissue of rat promoted fibroblast proliferation (Ohya et al., 2004). However, the poor biodegradability of PNIPAAm hydrogel has limited its applications. Several strategies for the preparation of biodegradable PNIPAAm-based hydrogels are being explored via mixing with poly-amino acids, polysaccharides, and poly-esters (Lanzalaco and Armelin, 2017). Another limitation for PNIPAAm-based materials is it may induce oxidative stress in tissue, which can contribute to chronic inflammation, leading to impaired wound healing and lowered the efficacy of cell-based therapies and medical devices.
To address the above challenges, a thermoresponsive macromolecule poly(polyethylene glycol citrateco-N-isopropylacrylamide) (PPCN), based on PNIPAAm, was developed. PPCN is a novel antioxidant which is both degradable and biocompatible (Yang et al., 2014b). The process of designing PPCN involves first synthesizing a water-soluble acrylated polydiolcitrate using citric acid, polyethylene glycol, and glycerol 1,3 diglycerolate diacrylate. Then, the water-soluble polydiolcitrate can be further functionalized with PNIPAAm to form a PPCN polymer (Yang et al., 2014b). PPCN has been proven to be an excellent biomaterial for bone formation as demonstrated in our laboratory (Dumanian et al., 2017; Morochnik et al., 2018; Zhao et al., 2018; Lee et al., 2019). We showed that when graphene oxide was incorporated into PPCN to fabricate the resultant hybrid materials referred to as GO-P, the composite scaffold maintained the thermoresponsive behavior of PPCN, effectively supporting BMSC survival and proliferation (Zhao et al., 2018). Furthermore, the GO-P scaffolds promoted BMP9-transduced BMSC mineralization and vascularized trabecular bone (Zhao et al., 2018). Mesenchymal progenitor immortalized murine adipocyte cells (iMADs) transduced with adenovirus expressing BMP-9 were cultured on a PPCN/gelatin scaffold to cure critical-sized cranial defects in mice. The scaffold promoted osteogenesis and exhibited significantly greater osteogenesis in vivo (Lee et al., 2019). Another study of murine-derived calvarial mesenchymal progenitor cells (iCALs) transduced by BMP-9 cultured on a PPCN/gelatin scaffold, to repair critical sized cranial defects, also promoted osseointegration and mature bone formation (Dumanian et al., 2017).
Even though biomaterials may possess favorable properties such as biocompatibility, biodegradability, osteoconductivity, and osteoinductivity, those materials are unable to replicate the complex microenvironment of native bone tissue (Benders et al., 2013). While allografts and xenografts are ideal methods for reconstructing bone defects, they carry the risk of transmission of infectious agents. To improve the safe use of allo- and xenografts, decellularization techniques are used to produce acellular scaffolds or decellularized extracellular matrix (dECM) (Gentili and Cancedda, 2009; Pati et al., 2015). The dECM functions as the non-cellular component of tissue that provides the structural and functional molecules to determine the cell's fate and serve as potential therapeutic materials (Frantz et al., 2010).
The major components of ECM are collagen and proteoglycans that are secreted by resident cells and assembled in a manner specific to individual tissue types, leading to a specialized ECM for each tissue type. The ECM is a dynamic entity that consistently responds to external influences including biomechanics and hormones (Nelson and Bissell, 2006). Given the high complexity, inherent compositional specificity, and the ability to support cell growth and differentiation, the engineered scaffolds based on tissue-specific natural ECM are likely more suitable than those built from artificial compounds for bone tissue regeneration (Benders et al., 2013).
Decellularization is the first step in processing ECM for use in regenerative therapy preserving the complex biomolecular and physical cues of the ECM (Crapo et al., 2011). The foremost challenge of the decellularization process is maximal removal of cellular components while limiting damage to the ECM (Crapo et al., 2011). The specific methods of decellularization depend on the tissue types and usually involve a combination of physical, enzymatic, and chemical processes. Physical decellularization is the least disruptive method, and includes freezing-thawing cycles, osmotic pressure, hydrostatic pressure, sonication, and electroporation (Oliveira et al., 2013; Santoso et al., 2014). Chemical decellularization can be divided into two subcategories. The first is to treat tissues with acids or bases to promote cell degradation and remove cellular components such as nucleic acids (Petersen et al., 2012). The second is to use non-ionic, ionic, or zwitterionic detergents such as Triton X-100, sodium dodecyl sulfate (SDS), and 3-((3-cholamidopropyl) dimethylammonio)-1-propanesulfonate, respectively, to denature proteins (Calle et al., 2016). Enzymatic decellularization is the use of nucleases, like deoxyribonuclease, ribonuclease, proteases, and trypsin, to facilitate cell degradation and the removal of residual nuclear materials from the tissue after chemical decellularization (Crapo et al., 2011). Since each decellularization method alone cannot completely remove cellular debris from the tissue, a combination of three methods is usually employed (Lu et al., 2012).
The scaffold forms of dECM used in BTE demonstrated fine biocompatibility and osteogenic capacity maintaining original geometry, cell-laid matrix, hydrogel, and particles (Benders et al., 2013). Smith et al. used a biocompatible biological scaffold from ECM, prepared via a novel washing technique to remove marrow components, which increased biocompatibility, conserved biomechanical stability, and induced osteogenic differentiation of MSCs (Smith et al., 2015). The cell-laid matrix refers to a layer of dECM coating on scaffolds, initially secreted by cells seeded onto the scaffolds, then decellularized, and recellularized for use as a regenerative therapy (Kumar et al., 2016). Kumar et al. seeded osteoblasts to deposit mineralized ECM on PCL/HA scaffolds, which displayed higher cell adhesion, growth, motility, and osteogenic differentiation compared to bare PCL/HA scaffolds (Kumar et al., 2016). Thibault et al. seeded rat MSCs on electrospun PCL and then cultured the sample in a complete osteogenic medium to generate mineralized ECM. The cell-laid matrix scaffold triggered higher osteogenic differentiation of MSCs and calcium deposition compared to bare PCL scaffolds (Thibault et al., 2010).
Decellularized small intestinal submucosa (dSIS) for ECM ornamentation has also been investigated (Li M. et al., 2018). Li et al. seeded osteoblasts on a dSIS scaffold to generate an osteogenic and mineralized ECM construct (Os/M-ECM-dSIS), which had similar morphology and inorganic components compared to natural bone and a higher mechanical strength than ECM-SIS (Li M. et al., 2018). Furthermore, these scaffolds also enhanced cell adhesion, proliferation, and osteogenic differentiation. In a calvarial defect model, new bone formation was enhanced in defects implanted with the Os/M-ECM-SIS scaffolds compared with ECM-SIS scaffolds via the Bmp/Smad-signaling pathway (Li M. et al., 2018). The dECM hydrogel was also created from solubilized dECM digested with pepsin. Since the dECM is digested into a homogenized and solubilized biomaterial, it does not preserve the architecture of the natural ECM (Sawkins et al., 2013). In terms of bone dECM, the production of a homogenized and solubilized biomaterial requires demineralization without digestion. A thermally responsive hydrogel made from both demineralized and decellularized bovine bone ECM was shown to promote the growth of primary calvarial cells in mice (Sawkins et al., 2013). Another novel bone dECM hydrogel scaffold combined with alginate incorporating human BMSCs and BMP2 was injected between segmental defects of embryonic chicken femurs and cultured ex vivo for 10 days, showing that the scaffold augmented skeletal tissue formation (Smith et al., 2014).
The bone dECM can be milled into particles that contain bone ECM components, which can be further combined with other biomaterials for use in BTE (Townsend et al., 2017; Beachley et al., 2018). Townsend et al. combined HA, decellularized cartilage, and hyaluronic acid to form colloidal gels that promoted bone regeneration when used in the treatment of bone defects in vivo (Townsend et al., 2017). Beachley et al. found a dECM particle-based composite hydrogel could be formulated by using modified GAGs (chondroitin sulfate, hyaluronic acid) that covalently bind to tissue particles; and bone dECM particles. The dECM particle-based hydrogel demonstrated enhanced bone formation compared to hydrogels that did not contain dECM particles (Beachley et al., 2018). Hung et al. created novel 3D printed hybrid dECM/PCL scaffolds by combining the bone dECM particles with PCL for bone regeneration. The scaffolds displayed robust mechanical properties, enhanced cell adhesion, upregulated osteogenic differentiation, generated greater bone volume in vivo, and increased calcification, compared with PCL alone (Hung et al., 2016).
Overall, dECM is a tissue-derived biomaterial that can be used as a bioactive component for tissue engineering applications. The addition of bone dECM frequently exhibited enhanced bone regenerative capabilities and guided the osteogenic differentiation of seeded stem cells even without the addition of exogenous growth factors. However, many improvements have to be made for the use of dECM in standard clinical treatments, such as methods of dECM tissue processing, tissue sources, and storage conditions. Therefore, the utilization of dECM in tissue engineering applications is still in development and requires more research, especially in exploring the best decellularization methods.
BTE is regarded as the most promising solution for critical-sized bone defects. The basic subunit in BTE is the scaffold, which provides a site for cell attachment, proliferation, and differentiation, as well as providing mechanical strength. Biomaterial selection and scaffold fabrication techniques are the two most important aspects to achieve these goals. Although the biomaterials discussed in this review have many advantages as scaffold materials in BTE, there are also shortcomings that limit their applications when used on their own. Therefore, discovery of new materials and manufacturing of composite scaffolds using these existing biomaterials will still constitute the center of future research efforts in the field of BTE. When combined with the developing array of bioactive materials, growth factors, functionalization techniques, and biomimetic scaffold designs, together with 3D bioprinting technology, the potential to create complex BTE scaffolds tailored to patient-specific applications in the future is vast.
All authors contributed significantly to the work and have read and concurred with the content of the manuscript.
The reported work was supported in part by research grants from the National Institutes of Health (CA226303 to T-CH) and the Scoliosis Research Society (T-CH and ML). WW was supported by the Medical Scientist Training Program of the National Institutes of Health (T32 GM007281). This project was also supported in part by The University of Chicago Cancer Center Support Grant (P30CA014599) and the National Center for Advancing Translational Sciences (NCATS) of the National Institutes of Health (NIH) through Grant Number 5UL1TR002389-02 that funds the Institute for Translational Medicine (ITM) although the contents are solely the responsibility of the authors and do not necessarily represent the official views of the NIH. T-CH was supported by the Mabel Green Myers Research Endowment Fund and The University of Chicago Orthopaedics Alumni Fund. Funding sources were not involved in the study design; in the collection, analysis, and interpretation of data; in the writing of the report; and in the decision to submit the paper for publication.
The authors declare that the research was conducted in the absence of any commercial or financial relationships that could be construed as a potential conflict of interest.
Ambard, A. J., and Mueninghoff, L. (2006). Calcium phosphate cement: review of mechanical and biological properties. J. Prosthodont. 15, 321–328. doi: 10.1111/j.1532-849X.2006.00129.x
Amini, A. R., Laurencin, C. T., and Nukavarapu, S. P. (2012). Bone tissue engineering: recent advances and challenges. Crit. Rev. Biomed. Eng. 40, 363–408. doi: 10.1615/CritRevBiomedEng.v40.i5.10
Anada, T., Kumagai, T., Honda, Y., Masuda, T., Kamijo, R., Kamakura, S., et al. (2008). Dose-dependent osteogenic effect of octacalcium phosphate on mouse bone marrow stromal cells. Tissue Eng. Part A 14, 965–978. doi: 10.1089/ten.tea.2007.0339
Anjana, J., Kuttappan, S., Keyan, K. S., and Nair, M. B. (2016). Evaluation of osteoinductive and endothelial differentiation potential of platelet-rich plasma incorporated gelatin-nanohydroxyapatite fibrous matrix. J. Biomed. Mater. Res. Part B Appl. Biomater. 104, 771–781. doi: 10.1002/jbm.b.33605
Asti, A., and Gioglio, L. (2014). Natural and synthetic biodegradable polymers: different scaffolds for cell expansion and tissue formation. Int. J. Artif. Organs 37, 187–205. doi: 10.530/ijao.5000307
Atyabi, S. M., Sharifi, F., Irani, S., Zandi, M., Mivehchi, H., and Nagheh, Z. (2016). Cell attachment and viability study of PCL nano-fiber modified by cold atmospheric plasma. Cell Biochem. Biophys. 74, 181–190. doi: 10.1007/s12013-015-0718-1
Azzopardi, P. V., O'Young, J., Lajoie, G., Karttunen, M., Goldberg, H. A., and Hunter, G. K. (2010). Roles of electrostatics and conformation in protein-crystal interactions. PLoS ONE 5:e9330. doi: 10.1371/journal.pone.0009330
Babilotte, J., Guduric, V., Le Nihouannen, D., Naveau, A., Fricain, J. C., and Catros, S. (2019). 3D printed polymer-mineral composite biomaterials for bone tissue engineering: fabrication and characterization. J. Biomed. Mater. Res. Part B Appl. Biomater. 107, 2579–2595. doi: 10.1002/jbm.b.34348
Baino, F., Hamzehlou, S., and Kargozar, S. (2018). Bioactive glasses: where are we and where are we going? J. Funct. Biomater 9:25. doi: 10.3390/jfb9010025
Balagangadharan, K., Dhivya, S., and Selvamurugan, N. (2017). Chitosan based nanofibers in bone tissue engineering. Int. J. Biol. Macromol. 104, 1372–1382. doi: 10.1016/j.ijbiomac.2016.12.046
Beachley, V., Ma, G., Papadimitriou, C., Gibson, M., Corvelli, M., and Elisseeff, J. (2018). Extracellular matrix particle-glycosaminoglycan composite hydrogels for regenerative medicine applications. J. Biomed. Mater. Res. A 106, 147–159. doi: 10.1002/jbm.a.36218
Benders, K. E., van Weeren, P. R., Badylak, S. F., Saris, D. B., Dhert, W. J., and Malda, J. (2013). Extracellular matrix scaffolds for cartilage and bone regeneration. Trends Biotechnol. 31, 169–176. doi: 10.1016/j.tibtech.2012.12.004
Bharadwaz, A., and Jayasuriya, A. C. (2020). Recent trends in the application of widely used natural and synthetic polymer nanocomposites in bone tissue regeneration. Mater. Sci. Eng. C Mater. Biol. Appl. 110:110698. doi: 10.1016/j.msec.2020.110698
Bhattacharjee, P., Kundu, B., Naskar, D., Kim, H. W., Maiti, T. K., Bhattacharya, D., et al. (2017). Silk scaffolds in bone tissue engineering: an overview. Acta Biomater. 63, 1–17. doi: 10.1016/j.actbio.2017.09.027
Bigi, A., Bracci, B., Cuisinier, F., Elkaim, R., Fini, M., Mayer, I., et al. (2005). Human osteoblast response to pulsed laser deposited calcium phosphate coatings. Biomaterials 26, 2381–2389. doi: 10.1016/j.biomaterials.2004.07.057
Bishop, E. S., Mostafa, S., Pakvasa, M., Luu, H. H., Lee, M. J., Wolf, J. M., et al. (2017). 3-D bioprinting technologies in tissue engineering and regenerative medicine: current and future trends. Genes Dis. 4, 185–195. doi: 10.1016/j.gendis.2017.10.002
Boukari, Y., Qutachi, O., Scurr, D. J., Morris, A. P., Doughty, S. W., and Billa, N. (2017). A dual-application poly (dl-lactic-co-glycolic) acid (PLGA)-chitosan composite scaffold for potential use in bone tissue engineering. J. Biomater. Sci. Polym. Ed. 28, 1966–1983. doi: 10.1080/09205063.2017.1364100
Bouler, J. M., Pilet, P., Gauthier, O., and Verron, E. (2017). Biphasic calcium phosphate ceramics for bone reconstruction: a review of biological response. Acta Biomater. 53, 1–12. doi: 10.1016/j.actbio.2017.01.076
Budsamongkol, T., Intarak, N., Theerapanon, T., Yodsanga, S., Porntaveetus, T., and Shotelersuk, V. (2019). A novel mutation in COL1A2 leads to osteogenesis imperfecta/Ehlers-Danlos overlap syndrome with brachydactyly. Genes Dis. 6, 138–146. doi: 10.1016/j.gendis.2019.03.001
Busra, M. F. M., and Lokanathan, Y. (2019). Recent development in the fabrication of collagen scaffolds for tissue engineering applications: a review. Curr. Pharm. Biotechnol. 20, 992–1003. doi: 10.2174/1389201020666190731121016
Calle, E. A., Hill, R. C., Leiby, K. L., Le, A. V., Gard, A. L., Madri, J. A., et al. (2016). Targeted proteomics effectively quantifies differences between native lung and detergent-decellularized lung extracellular matrices. Acta Biomater. 46, 91–100. doi: 10.1016/j.actbio.2016.09.043
Campana, V., Milano, G., Pagano, E., Barba, M., Cicione, C., Salonna, G., et al. (2014). Bone substitutes in orthopaedic surgery: from basic science to clinical practice. J. Mater. Sci. Mater. Med. 25, 2445–2461. doi: 10.1007/s10856-014-5240-2
Ceccarelli, G., Presta, R., Benedetti, L., Cusella De Angelis, M. G., Lupi, S. M., and Rodriguez, Y. B. R. (2017). Emerging perspectives in scaffold for tissue engineering in oral surgery. Stem Cells Int. 2017:4585401. doi: 10.1155/2017/4585401
Chen, J., Zhan, Y., Wang, Y., Han, D., Tao, B., Luo, Z., et al. (2018). Chitosan/silk fibroin modified nanofibrous patches with mesenchymal stem cells prevent heart remodeling post-myocardial infarction in rats. Acta Biomater. 80, 154–168. doi: 10.1016/j.actbio.2018.09.013
Cheng, F., Wu, Y., Li, H., Yan, T., Wei, X., Wu, G., et al. (2019). Biodegradable N, O-carboxymethyl chitosan/oxidized regenerated cellulose composite gauze as a barrier for preventing postoperative adhesion. Carbohydr. Polym. 207, 180–190. doi: 10.1016/j.carbpol.2018.10.077
Cheng, H., Chabok, R., Guan, X., Chawla, A., Li, Y., Khademhosseini, A., et al. (2018). Synergistic interplay between the two major bone minerals, hydroxyapatite and whitlockite nanoparticles, for osteogenic differentiation of mesenchymal stem cells. Acta Biomater. 69, 342–351. doi: 10.1016/j.actbio.2018.01.016
Cheng, Y., Ramos, D., Lee, P., Liang, D., Yu, X., and Kumbar, S. G. (2014). Collagen functionalized bioactive nanofiber matrices for osteogenic differentiation of mesenchymal stem cells: bone tissue engineering. J. Biomed. Nanotechnol. 10, 287–298. doi: 10.1166/jbn.2014.1753
Coalson, E., Bishop, E., Liu, W., Feng, Y., Spezia, M., Liu, B., et al. (2019). Stem cell therapy for chronic skin wounds in the era of personalized medicine: from bench to bedside. Genes Dis. 6, 342–358. doi: 10.1016/j.gendis.2019.09.008
Cojocaru, D. G., Hondke, S., Krüger, J. P., Bosch, C., Croicu, C., Florescu, S., et al. (2020). Meniscus-shaped cell-free polyglycolic acid scaffold for meniscal repair in a sheep model. J. Biomed. Mater. Res. Part B Appl. Biomater. 108, 809–818. doi: 10.1002/jbm.b.34435
Collins, M. N., and Birkinshaw, C. (2013). Hyaluronic acid based scaffolds for tissue engineering–a review. Carbohydr. Polym. 92, 1262–1279. doi: 10.1016/j.carbpol.2012.10.028
Cordonnier, T., Layrolle, P., Gaillard, J., Langonné, A., Sensebé, L., Rosset, P., et al. (2010). 3D environment on human mesenchymal stem cells differentiation for bone tissue engineering. J. Mater. Sci. Mater. Med. 21, 981–987. doi: 10.1007/s10856-009-3916-9
Crapo, P. M., Gilbert, T. W., and Badylak, S. F. (2011). An overview of tissue and whole organ decellularization processes. Biomaterials 32, 3233–3243. doi: 10.1016/j.biomaterials.2011.01.057
Cui, N., Qian, J., Liu, T., Zhao, N., and Wang, H. (2015). Hyaluronic acid hydrogel scaffolds with a triple degradation behavior for bone tissue engineering. Carbohydr. Polym. 126, 192–198. doi: 10.1016/j.carbpol.2015.03.013
Darimont, G. L., Cloots, R., Heinen, E., Seidel, L., and Legrand, R. (2002). In vivo behaviour of hydroxyapatite coatings on titanium implants: a quantitative study in the rabbit. Biomaterials 23, 2569–2575. doi: 10.1016/S0142-9612(01)00392-1
Dhivya, S., Saravanan, S., Sastry, T. P., and Selvamurugan, N. (2015). Nanohydroxyapatite-reinforced chitosan composite hydrogel for bone tissue repair in vitro and in vivo. J. Nanobiotechnol. 13:40. doi: 10.1186/s12951-015-0099-z
Dimitriou, R., Jones, E., McGonagle, D., and Giannoudis, P. V. (2011). Bone regeneration: current concepts and future directions. BMC Med. 9:66. doi: 10.1186/1741-7015-9-66
Dinescu, S., Ionita, M., Pandele, A. M., Galateanu, B., Iovu, H., Ardelean, A., et al. (2014). In vitro cytocompatibility evaluation of chitosan/graphene oxide 3D scaffold composites designed for bone tissue engineering. Biomed. Mater. Eng. 24, 2249–2256. doi: 10.3233/BME-141037
Donnaloja, F., Jacchetti, E., Soncini, M., and Raimondi, M. T. (2020). Natural and synthetic polymers for bone scaffolds optimization. Polymers 12:905. doi: 10.3390/polym12040905
Dorozhkin, S. V. (2012). Biphasic, triphasic and multiphasic calcium orthophosphates. Acta Biomater. 8, 963–977. doi: 10.1016/j.actbio.2011.09.003
Douglas, T., Pamula, E., Hauk, D., Wiltfang, J., Sivananthan, S., Sherry, E., et al. (2009). Porous polymer/hydroxyapatite scaffolds: characterization and biocompatibility investigations. J. Mater. Sci. Mater. Med. 20, 1909–1915. doi: 10.1007/s10856-009-3756-7
Drury, J. L., and Mooney, D. J. (2003). Hydrogels for tissue engineering: scaffold design variables and applications. Biomaterials 24, 4337–4351. doi: 10.1016/S0142-9612(03)00340-5
Du, X., Wei, D., Huang, L., Zhu, M., Zhang, Y., and Zhu, Y. (2019). 3D printing of mesoporous bioactive glass/silk fibroin composite scaffolds for bone tissue engineering. Mater. Sci. Eng. C Mater. Biol. Appl. 103:109731. doi: 10.1016/j.msec.2019.05.016
Dumanian, Z. P., Tollemar, V., Ye, J., Lu, M., Zhu, Y., Liao, J., et al. (2017). Repair of critical sized cranial defects with BMP9-transduced calvarial cells delivered in a thermoresponsive scaffold. PLoS ONE 12:e0172327. doi: 10.1371/journal.pone.0172327
Dunne, N., Jack, V., O'Hara, R., Farrar, D., and Buchanan, F. (2010). Performance of calcium deficient hydroxyapatite-polyglycolic acid composites: an in vitro study. J. Mater. Sci. Mater. Med. 21, 2263–2270. doi: 10.1007/s10856-010-4021-9
Dvorak, M. M., Siddiqua, A., Ward, D. T., Carter, D. H., Dallas, S. L., Nemeth, E. F., et al. (2004). Physiological changes in extracellular calcium concentration directly control osteoblast function in the absence of calciotropic hormones. Proc. Natl. Acad. Sci. U.S.A. 101, 5140–5145. doi: 10.1073/pnas.0306141101
Dwivedi, R., Kumar, S., Pandey, R., Mahajan, A., Nandana, D., Katti, D. S., et al. (2020). Polycaprolactone as biomaterial for bone scaffolds: review of literature. J. Oral Biol. Craniofac. Res. 10, 381–388. doi: 10.1016/j.jobcr.2019.10.003
Ebrahimi, M., Botelho, M. G., and Dorozhkin, S. V. (2017). Biphasic calcium phosphates bioceramics (HA/TCP): concept, physicochemical properties and the impact of standardization of study protocols in biomaterials research. Mater. Sci. Eng. C Mater. Biol. Appl. 71, 1293–1312. doi: 10.1016/j.msec.2016.11.039
Echave, M. C., Hernaez-Moya, R., Iturriaga, L., Pedraz, J. L., Lakshminarayanan, R., Dolatshahi-Pirouz, A., et al. (2019). Recent advances in gelatin-based therapeutics. Expert Opin. Biol. Ther. 19, 773–779. doi: 10.1080/14712598.2019.1610383
Elango, J., Zhang, J., Bao, B., Palaniyandi, K., Wang, S., Wenhui, W., et al. (2016). Rheological, biocompatibility and osteogenesis assessment of fish collagen scaffold for bone tissue engineering. Int. J. Biol. Macromol. 91, 51–59. doi: 10.1016/j.ijbiomac.2016.05.067
Ercal, P., and Pekozer, G. G. (2020). A current overview of scaffold-based bone regeneration strategies with dental stem cells. Adv. Exp. Med. Biol. 1288:61–85. doi: 10.1007/5584_2020_505
Essa, D., Kondiah, P. P. D., Choonara, Y. E., and Pillay, V. (2020). The design of poly(lactide-co-glycolide) nanocarriers for medical applications. Front. Bioeng. Biotechnol. 8:48. doi: 10.3389/fbioe.2020.00048
Faia-Torres, A. B., Charnley, M., Goren, T., Guimond-Lischer, S., Rottmar, M., Maniura-Weber, K., et al. (2015). Osteogenic differentiation of human mesenchymal stem cells in the absence of osteogenic supplements: a surface-roughness gradient study. Acta Biomater. 28, 64–75. doi: 10.1016/j.actbio.2015.09.028
Fang, X., Lei, L., Jiang, T., Chen, Y., and Kang, Y. (2018). Injectable thermosensitive alginate/β-tricalcium phosphate/aspirin hydrogels for bone augmentation. J. Biomed. Mater. Res. Part B Appl. Biomater. 106, 1739–1751. doi: 10.1002/jbm.b.33982
Farokhi, M., Mottaghitalab, F., Samani, S., Shokrgozar, M. A., Kundu, S. C., Reis, R. L., et al. (2018). Silk fibroin/hydroxyapatite composites for bone tissue engineering. Biotechnol. Adv. 36, 68–91. doi: 10.1016/j.biotechadv.2017.10.001
Fellah, B. H., Weiss, P., Gauthier, O., Rouillon, T., Pilet, P., Daculsi, G., et al. (2006). Bone repair using a new injectable self-crosslinkable bone substitute. J. Orthop. Res. 24, 628–635. doi: 10.1002/jor.20125
Ferreira, A. M., Gentile, P., Chiono, V., and Ciardelli, G. (2012). Collagen for bone tissue regeneration. Acta Biomater. 8, 3191–3200. doi: 10.1016/j.actbio.2012.06.014
Florczyk, S. J., Kim, D. J., Wood, D. L., and Zhang, M. (2011). Influence of processing parameters on pore structure of 3D porous chitosan-alginate polyelectrolyte complex scaffolds. J. Biomed. Mater. Res. A 98, 614–620. doi: 10.1002/jbm.a.33153
Frantz, C., Stewart, K. M., and Weaver, V. M. (2010). The extracellular matrix at a glance. J. Cell Sci. 123, 4195–4200. doi: 10.1242/jcs.023820
Gentili, C., and Cancedda, R. (2009). Cartilage and bone extracellular matrix. Curr. Pharm. Des 15, 1334–1348. doi: 10.2174/138161209787846739
George, M., and Abraham, T. E. (2006). Polyionic hydrocolloids for the intestinal delivery of protein drugs: alginate and chitosan–a review. J. Control. Release 114, 1–14. doi: 10.1016/j.jconrel.2006.04.017
Gokila, S., Gomathi, T., Vijayalakshmi, K., Faleh, A., Sukumaran, A., and Sudha, P. (2018). Development of 3D scaffolds using nanochitosan/silk-fibroin/hyaluronic acid biomaterials for tissue engineering applications. Int. J. Biol. Macromol. 120, 876–885. doi: 10.1016/j.ijbiomac.2018.08.149
Gregor, A., Filova, E., Novak, M., Kronek, J., Chlup, H., Buzgo, M., et al. (2017). Designing of PLA scaffolds for bone tissue replacement fabricated by ordinary commercial 3D printer. J. Biol. Eng. 11:31. doi: 10.1186/s13036-017-0074-3
Gremare, A., Guduric, V., Bareille, R., Heroguez, V., Latour, S., L'Heureux, N., et al. (2018). Characterization of printed PLA scaffolds for bone tissue engineering. J. Biomed. Mater. Res. A 106, 887–894. doi: 10.1002/jbm.a.36289
Gustavsson, J., Ginebra, M. P., Planell, J., and Engel, E. (2012). Osteoblast-like cellular response to dynamic changes in the ionic extracellular environment produced by calcium-deficient hydroxyapatite. J. Mater. Sci. Mater. Med. 23, 2509–2520. doi: 10.1007/s10856-012-4705-4
He, Y., Wang, W., Tang, X., and Liu, X. (2017). Osteogenic induction of bone marrow mesenchymal cells on electrospun polycaprolactone/chitosan nanofibrous membrane. Dent. Mater. J. 36, 325–332. doi: 10.4012/dmj.2016-203
Hemshekhar, M., Thushara, R. M., Chandranayaka, S., Sherman, L. S., Kemparaju, K., and Girish, K. S. (2016). Emerging roles of hyaluronic acid bioscaffolds in tissue engineering and regenerative medicine. Int. J. Biol. Macromol. 86, 917–928. doi: 10.1016/j.ijbiomac.2016.02.032
Herford, A. S., Stoffella, E., and Tandon, R. (2011). Reconstruction of mandibular defects using bone morphogenic protein: can growth factors replace the need for autologous bone grafts? A systematic review of the literature. Plast Surg. Int. 2011:165824. doi: 10.1155/2011/165824
Ho, M. H., Yao, C. J., Liao, M. H., Lin, P. I., Liu, S. H., and Chen, R. M. (2015). Chitosan nanofiber scaffold improves bone healing via stimulating trabecular bone production due to upregulation of the Runx2/osteocalcin/alkaline phosphatase signaling pathway. Int. J. Nanomed. 10, 5941–5954. doi: 10.2147/IJN.S90669
Honda, Y., Anada, T., Kamakura, S., Morimoto, S., Kuriyagawa, T., and Suzuki, O. (2009). The effect of microstructure of octacalcium phosphate on the bone regenerative property. Tissue Eng. Part A 15, 1965–1973. doi: 10.1089/ten.tea.2008.0300
Horch, H. H., Sader, R., Pautke, C., Neff, A., Deppe, H., and Kolk, A. (2006). Synthetic, pure-phase beta-tricalcium phosphate ceramic granules (Cerasorb) for bone regeneration in the reconstructive surgery of the jaws. Int. J. Oral Maxillofac. Surg. 35, 708–713. doi: 10.1016/j.ijom.2006.03.017
Hosseinpour, S., Ghazizadeh Ahsaie, M., Rezai Rad, M., Baghani, M. T., Motamedian, S. R., and Khojasteh, A. (2017). Application of selected scaffolds for bone tissue engineering: a systematic review. Oral Maxillofac. Surg. 21, 109–129. doi: 10.1007/s10006-017-0608-3
Hu, K., Cui, F., Lv, Q., Ma, J., Feng, Q., Xu, L., et al. (2008). Preparation of fibroin/recombinant human-like collagen scaffold to promote fibroblasts compatibility. J. Biomed. Mater. Res. A 84, 483–490. doi: 10.1002/jbm.a.31440
Hung, B. P., Naved, B. A., Nyberg, E. L., Dias, M., Holmes, C. A., Elisseeff, J. H., et al. (2016). Three-dimensional printing of bone extracellular matrix for craniofacial regeneration. ACS Biomater. Sci. Eng. 2, 1806–1816. doi: 10.1021/acsbiomaterials.6b00101
Hwang, Y. S., Cho, J., Tay, F., Heng, J. Y., Ho, R., Kazarian, S. G., et al. (2009). The use of murine embryonic stem cells, alginate encapsulation, and rotary microgravity bioreactor in bone tissue engineering. Biomaterials 30, 499–507. doi: 10.1016/j.biomaterials.2008.07.028
Iaquinta, M. R., Mazzoni, E., Bononi, I., Rotondo, J. C., Mazziotta, C., Montesi, M., et al. (2019). Adult stem cells for bone regeneration and repair. Front. Cell Dev. Biol. 7:268. doi: 10.3389/fcell.2019.00268
Jang, H. L., Lee, H. K., Jin, K., Ahn, H. Y., Lee, H. E., and Nam, K. T. (2015). Phase transformation from hydroxyapatite to the secondary bone mineral, whitlockite. J. Mater. Chem. B 3, 1342–1349. doi: 10.1039/C4TB01793E
Jang, H. L., Zheng, G. B., Park, J., Kim, H. D., Baek, H. R., Lee, H. K., et al. (2016). In vitro and in vivo evaluation of whitlockite biocompatibility: comparative study with hydroxyapatite and beta-tricalcium phosphate. Adv. Healthc. Mater 5, 128–136. doi: 10.1002/adhm.201400824
Jeong, J., Kim, J. H., Shim, J. H., Hwang, N. S., and Heo, C. Y. (2019). Bioactive calcium phosphate materials and applications in bone regeneration. Biomater Res 23:4. doi: 10.1186/s40824-018-0149-3
Jin, H. H., Kim, D. H., Kim, T. W., Shin, K. K., Jung, J. S., Park, H. C., et al. (2012). In vivo evaluation of porous hydroxyapatite/chitosan-alginate composite scaffolds for bone tissue engineering. Int. J. Biol. Macromol. 51, 1079–1085. doi: 10.1016/j.ijbiomac.2012.08.027
Johnson, M. W., Sullivan, S. M., Rohrer, M., and Collier, M. (1997). Regeneration of peri-implant infrabony defects using PerioGlas: a pilot study in rabbits. Int. J. Oral Maxillofac. Implants 12, 835–839.
Kamakura, S., Sasano, Y., Homma, H., Suzuki, O., Kagayama, M., and Motegi, K. (1999). Implantation of octacalcium phosphate (OCP) in rat skull defects enhances bone repair. J. Dent. Res. 78, 1682–1687. doi: 10.1177/00220345990780110401
Kamitakahara, M., Ohtsuki, C., and Miyazaki, T. (2008). Review paper: behavior of ceramic biomaterials derived from tricalcium phosphate in physiological condition. J. Biomater. Appl. 23, 197–212. doi: 10.1177/0885328208096798
Karakuzu-Ikizler, B., Terzioglu, P., Basaran-Elalmis, Y., Tekerek, B. S., and Yücel, S. (2020). Role of magnesium and aluminum substitution on the structural properties and bioactivity of bioglasses synthesized from biogenic silica. Bioact. Mater. 5, 66–73. doi: 10.1016/j.bioactmat.2019.12.007
Kargozar, S., Baino, F., Hamzehlou, S., Hill, R. G., and Mozafari, M. (2018). Bioactive glasses entering the mainstream. Drug Discov. Today 23, 1700–1704. doi: 10.1016/j.drudis.2018.05.027
Kaur, G., Kumar, V., Baino, F., Mauro, J. C., Pickrell, G., Evans, I., et al. (2019). Mechanical properties of bioactive glasses, ceramics, glass-ceramics and composites: state-of-the-art review and future challenges. Mater. Sci. Eng. C Mater. Biol. Appl. 104:109895. doi: 10.1016/j.msec.2019.109895
Kikawa, T., Kashimoto, O., Imaizumi, H., Kokubun, S., and Suzuki, O. (2009). Intramembranous bone tissue response to biodegradable octacalcium phosphate implant. Acta Biomater. 5, 1756–1766. doi: 10.1016/j.actbio.2008.12.008
Kim, D. Y., Han, Y. H., Lee, J. H., Kang, I. K., Jang, B. K., and Kim, S. (2014). Characterization of multiwalled carbon nanotube-reinforced hydroxyapatite composites consolidated by spark plasma sintering. Biomed Res. Int. 2014:768254. doi: 10.1155/2014/768254
Kim, H. D., Jang, H. L., Ahn, H. Y., Lee, H. K., Park, J., Lee, E. S., et al. (2017b). Biomimetic whitlockite inorganic nanoparticles-mediated in situ remodeling and rapid bone regeneration. Biomaterials 112, 31–43. doi: 10.1016/j.biomaterials.2016.10.009
Kim, H. W., Lee, H. H., and Knowles, J. C. (2006). Electrospinning biomedical nanocomposite fibers of hydroxyapatite/poly(lactic acid) for bone regeneration. J. Biomed. Mater. Res. A 79, 643–649. doi: 10.1002/jbm.a.30866
Kim, S. H., Thambi, T., Giang Phan, V. H., and Lee, D. S. (2020). Modularly engineered alginate bioconjugate hydrogel as biocompatible injectable scaffold for in situ biomineralization. Carbohydr. Polym. 233:115832. doi: 10.1016/j.carbpol.2020.115832
Kim, S. M., Yi, S. A., Choi, S. H., Kim, K. M., and Lee, Y. K. (2012). Gelatin-layered and multi-sized porous β-tricalcium phosphate for tissue engineering scaffold. Nanoscale Res. Lett. 7:78. doi: 10.1186/1556-276X-7-78
Kim, Y., and Kim, G. (2015). Highly roughened polycaprolactone surfaces using oxygen plasma-etching and in vitro mineralization for bone tissue regeneration: fabrication, characterization, and cellular activities. Colloids Surf. B Biointerfaces 125, 181–189. doi: 10.1016/j.colsurfb.2014.11.033
Kim, Y. S., Majid, M., Melchiorri, A. J., and Mikos, A. G. (2019). Applications of decellularized extracellular matrix in bone and cartilage tissue engineering. Bioeng Transl. Med. 4, 83–95. doi: 10.1002/btm2.10110
Kim, H. D., Amirthalingam, S., Kim, S. L., Lee, S. S., Rangasamy, J., and Hwang, N. S. (2017a). Biomimetic materials and fabrication approaches for bone tissue engineering. Adv. Healthc. Mater. 6. doi: 10.1002/adhm.201700612
Kolesky, D. B., Homan, K. A., Skylar-Scott, M. A., and Lewis, J. A. (2016). Three-dimensional bioprinting of thick vascularized tissues. Proc. Natl. Acad. Sci. U.S.A. 113, 3179–3184. doi: 10.1073/pnas.1521342113
Krishnan, A. G., Jayaram, L., Biswas, R., and Nair, M. (2015). Evaluation of antibacterial activity and cytocompatibility of ciprofloxacin loaded gelatin-hydroxyapatite scaffolds as a local drug delivery system for osteomyelitis treatment. Tissue Eng. Part A 21, 1422–1431. doi: 10.1089/ten.tea.2014.0605
Kumar, A., Nune, K. C., and Misra, R. D. (2016). Biological functionality of extracellular matrix-ornamented three-dimensional printed hydroxyapatite scaffolds. J. Biomed. Mater. Res. A 104, 1343–1351. doi: 10.1002/jbm.a.35664
Kuttappan, S., Mathew, D., and Nair, M. B. (2016). Biomimetic composite scaffolds containing bioceramics and collagen/gelatin for bone tissue engineering - a mini review. Int. J. Biol. Macromol. 93, 1390–1401. doi: 10.1016/j.ijbiomac.2016.06.043
Lanzalaco, S., and Armelin, E. (2017). Poly(N-isopropylacrylamide) and copolymers: a review on recent progresses in biomedical applications. Gels 3:36. doi: 10.3390/gels3040036
Lee, C. S., Bishop, E. S., Dumanian, Z., Zhao, C., Song, D., Zhang, F., et al. (2019). Bone morphogenetic protein-9-stimulated adipocyte-derived mesenchymal progenitors entrapped in a thermoresponsive nanocomposite scaffold facilitate cranial defect repair. J. Craniofac. Surg. 30, 1915–1919. doi: 10.1097/SCS.0000000000005465
Lee, D. H., Tripathy, N., Shin, J. H., Song, J. E., Cha, J. G., Min, K. D., et al. (2017). Enhanced osteogenesis of β-tricalcium phosphate reinforced silk fibroin scaffold for bone tissue biofabrication. Int. J. Biol. Macromol. 95, 14–23. doi: 10.1016/j.ijbiomac.2016.11.002
Lee, J., and Kim, G. (2018). Three-dimensional hierarchical nanofibrous collagen scaffold fabricated using fibrillated collagen and pluronic f-127 for regenerating bone tissue. ACS Appl. Mater. Interfaces 10, 35801–35811. doi: 10.1021/acsami.8b14088
Lei, B., Shin, K. H., Noh, D. Y., Koh, Y. H., Choi, W. Y., and Kim, H. E. (2012). Bioactive glass microspheres as reinforcement for improving the mechanical properties and biological performance of poly(ε-caprolactone) polymer for bone tissue regeneration. J. Biomed. Mater. Res. Part B Appl. Biomater. 100, 967–975. doi: 10.1002/jbm.b.32659
Li, L., Qian, Y., Jiang, C., Lv, Y., Liu, W., Zhong, L., et al. (2012). The use of hyaluronan to regulate protein adsorption and cell infiltration in nanofibrous scaffolds. Biomaterials 33, 3428–3445. doi: 10.1016/j.biomaterials.2012.01.038
Li, M., Zhang, C., Mao, Y., Zhong, Y., and Zhao, J. (2018). A Cell-Engineered Small Intestinal Submucosa-Based Bone Mimetic Construct for Bone Regeneration. Tissue Eng. Part A 24, 1099–1111. doi: 10.1089/ten.tea.2017.0407
Li, S., Yu, D., Ji, H., Zhao, B., Ji, L., and Leng, X. (2018). In vivo degradation and neovascularization of silk fibroin implants monitored by multiple modes ultrasound for surgical applications. Biomed. Eng. Online 17:87. doi: 10.1186/s12938-018-0478-4
Li, X., Song, T., Chen, X., Wang, M., Yang, X., Xiao, Y., et al. (2019). Osteoinductivity of Porous Biphasic Calcium Phosphate Ceramic Spheres with Nanocrystalline and Their Efficacy in Guiding Bone Regeneration. ACS Appl. Mater. Interfaces 11, 3722–3736. doi: 10.1021/acsami.8b18525
Lin, W. C., Yao, C., Huang, T. Y., Cheng, S. J., and Tang, C. M. (2019). Long-term in vitro degradation behavior and biocompatibility of polycaprolactone/cobalt-substituted hydroxyapatite composite for bone tissue engineering. Dent. Mater. 35, 751–762. doi: 10.1016/j.dental.2019.02.023
Lin, Y., Huang, S., Zou, R., Gao, X., Ruan, J., Weir, M. D., et al. (2019). Calcium phosphate cement scaffold with stem cell co-culture and prevascularization for dental and craniofacial bone tissue engineering. Dent. Mater. 35, 1031–1041. doi: 10.1016/j.dental.2019.04.009
LogithKumar, R., KeshavNarayan, A., Dhivya, S., Chawla, A., Saravanan, S., and Selvamurugan, N. (2016). A review of chitosan and its derivatives in bone tissue engineering. Carbohydr. Polym. 151, 172–188. doi: 10.1016/j.carbpol.2016.05.049
Lu, H., Hoshiba, T., Kawazoe, N., and Chen, G. (2012). Comparison of decellularization techniques for preparation of extracellular matrix scaffolds derived from three-dimensional cell culture. J. Biomed. Mater. Res. A 100, 2507–2516. doi: 10.1002/jbm.a.34150
Lü, J. M., Wang, X., Marin-Muller, C., Wang, H., Lin, P. H., Yao, Q., et al. (2009). Current advances in research and clinical applications of PLGA-based nanotechnology. Expert Rev. Mol. Diagn. 9, 325–341. doi: 10.1586/erm.09.15
Lu, Y., Li, M., Long, Z., Di, Y., Guo, S., Li, J., et al. (2019). Collagen/β-TCP composite as a bone-graft substitute for posterior spinal fusion in rabbit model: a comparison study. Biomed. Mater. 14:045009. doi: 10.1088/1748-605X/ab1caf
Luginbuehl, V., Ruffieux, K., Hess, C., Reichardt, D., von Rechenberg, B., and Nuss, K. (2010). Controlled release of tetracycline from biodegradable beta-tricalcium phosphate composites. J. Biomed. Mater. Res. Part B Appl. Biomater. 92, 341–352. doi: 10.1002/jbm.b.31520
Mahmoudi Saber, M. (2019). Strategies for surface modification of gelatin-based nanoparticles. Colloids Surf. B Biointerfaces 183:110407. doi: 10.1016/j.colsurfb.2019.110407
Mandal, B. B., and Kundu, S. C. (2009). Osteogenic and adipogenic differentiation of rat bone marrow cells on non-mulberry and mulberry silk gland fibroin 3D scaffolds. Biomaterials 30, 5019–5030. doi: 10.1016/j.biomaterials.2009.05.064
Mencía Castaño, I., Curtin, C. M., Duffy, G. P., and O'Brien, F. J. (2019). Harnessing an inhibitory role of miR-16 in osteogenesis by human mesenchymal stem cells for advanced scaffold-based bone tissue engineering. Tissue Eng. Part A 25, 24–33. doi: 10.1089/ten.tea.2017.0460
Midha, S., Murab, S., and Ghosh, S. (2016). Osteogenic signaling on silk-based matrices. Biomaterials 97, 133–153. doi: 10.1016/j.biomaterials.2016.04.020
Morochnik, S., Zhu, Y., Duan, C., Cai, M., Reid, R. R., He, T. C., et al. (2018). A thermoresponsive, citrate-based macromolecule for bone regenerative engineering. J. Biomed. Mater. Res. A 106, 1743–1752. doi: 10.1002/jbm.a.36358
Mostafa, S., Pakvasa, M., Coalson, E., Zhu, A., Alverdy, A., Castillo, H., et al. (2019). The wonders of BMP9: from mesenchymal stem cell differentiation, angiogenesis, neurogenesis, tumorigenesis, and metabolism to regenerative medicine. Genes Dis. 6, 201–223. doi: 10.1016/j.gendis.2019.07.003
Müllner, M. (2019). Functional Natural and Synthetic Polymers. Macromol. Rapid Commun. 40:e1900151. doi: 10.1002/marc.201900151
Murab, S., Chameettachal, S., Bhattacharjee, M., Das, S., Kaplan, D. L., and Ghosh, S. (2013). Matrix-embedded cytokines to simulate osteoarthritis-like cartilage microenvironments. Tissue Eng. Part A 19, 1733–1753. doi: 10.1089/ten.tea.2012.0385
Murab, S., Samal, J., Shrivastava, A., Ray, A. R., Pandit, A., and Ghosh, S. (2015). Glucosamine loaded injectable silk-in-silk integrated system modulate mechanical properties in bovine ex-vivo degenerated intervertebral disc model. Biomaterials 55, 64–83. doi: 10.1016/j.biomaterials.2015.03.032
Murphy, C. M., O'Brien, F. J., Little, D. G., and Schindeler, A. (2013). Cell-scaffold interactions in the bone tissue engineering triad. Eur. Cell. Mater. 26, 120–132. doi: 10.22203/eCM.v026a09
Nakamura, M., Hentunen, T., Salonen, J., Nagai, A., and Yamashita, K. (2013). Characterization of bone mineral-resembling biomaterials for optimizing human osteoclast differentiation and resorption. J. Biomed. Mater. Res. A 101, 3141–3151. doi: 10.1002/jbm.a.34621
Neamat, A., Gawish, A., and Gamal-Eldeen, A. M. (2009). beta-Tricalcium phosphate promotes cell proliferation, osteogenesis and bone regeneration in intrabony defects in dogs. Arch. Oral Biol. 54, 1083–1090. doi: 10.1016/j.archoralbio.2009.09.003
Nelson, C. M., and Bissell, M. J. (2006). Of extracellular matrix, scaffolds, and signaling: tissue architecture regulates development, homeostasis, and cancer. Annu. Rev. Cell Dev. Biol. 22, 287–309. doi: 10.1146/annurev.cellbio.22.010305.104315
Neo, M., Nakamura, T., Ohtsuki, C., Kokubo, T., and Yamamuro, T. (1993). Apatite formation on three kinds of bioactive material at an early stage in vivo: a comparative study by transmission electron microscopy. J. Biomed. Mater. Res. 27, 999–1006. doi: 10.1002/jbm.820270805
Nguyen, T. B., and Lee, B. T. (2014). A combination of biphasic calcium phosphate scaffold with hyaluronic acid-gelatin hydrogel as a new tool for bone regeneration. Tissue Eng. Part A 20, 1993–2004. doi: 10.1089/ten.tea.2013.0352
Nguyen, T. P., Nguyen, Q. V., Nguyen, V. H., Le, T. H., Huynh, V. Q. N., Vo, D. N., et al. (2019). Silk fibroin-based biomaterials for biomedical applications: a review. Polymers 11:1933. doi: 10.3390/polym11121933
Nocera, A. D., Comín, R., Salvatierra, N. A., and Cid, M. P. (2018). Development of 3D printed fibrillar collagen scaffold for tissue engineering. Biomed. Microdevices 20:26. doi: 10.1007/s10544-018-0270-z
Ogose, A., Hotta, T., Hatano, H., Kawashima, H., Tokunaga, K., Endo, N., et al. (2002). Histological examination of beta-tricalcium phosphate graft in human femur. J. Biomed. Mater. Res. 63, 601–604. doi: 10.1002/jbm.10380
Ohya, S., Nakayama, Y., and Matsuda, T. (2004). In vivo evaluation of poly(N-isopropylacrylamide) (PNIPAM)-grafted gelatin as an in situ-formable scaffold. J. Artif. Organs 7, 181–186. doi: 10.1007/s10047-004-0265-9
Oliveira, A. C., Garzón, I., Ionescu, A. M., Carriel, V., Cardona Jde, L., González-Andrades, M., et al. (2013). Evaluation of small intestine grafts decellularization methods for corneal tissue engineering. PLoS ONE 8:e66538. doi: 10.1371/journal.pone.0066538
Ortega-Oller, I., Padial-Molina, M., Galindo-Moreno, P., O'Valle, F., Jódar-Reyes, A. B., and Peula-García, J. M. (2015). Bone regeneration from PLGA micro-nanoparticles. Biomed Res. Int. 2015:415289. doi: 10.1155/2015/415289
Pakvasa, M., Haravu, P., Boachie-Mensah, M., Jones, A., Coalson, E., Liao, J., et al. (2021). Notch signaling: its essential roles in bone and craniofacial development. Genes Dis. doi: 10.1016/j.gendis.2020.04.006
Patel, N., Best, S. M., Bonfield, W., Gibson, I. R., Hing, K. A., Damien, E., et al. (2002). A comparative study on the in vivo behavior of hydroxyapatite and silicon substituted hydroxyapatite granules. J. Mater. Sci. Mater. Med. 13, 1199–1206. doi: 10.1023/A:1021114710076
Pati, F., Song, T. H., Rijal, G., Jang, J., Kim, S. W., and Cho, D. W. (2015). Ornamenting 3D printed scaffolds with cell-laid extracellular matrix for bone tissue regeneration. Biomaterials 37, 230–241. doi: 10.1016/j.biomaterials.2014.10.012
Perez, J. R., Kouroupis, D., Li, D. J., Best, T. M., Kaplan, L., and Correa, D. (2018). Tissue Engineering and cell-based therapies for fractures and bone defects. Front. Bioeng. Biotechnol. 6:105. doi: 10.3389/fbioe.2018.00105
Petersen, T. H., Calle, E. A., Colehour, M. B., and Niklason, L. E. (2012). Matrix composition and mechanics of decellularized lung scaffolds. Cells Tissues Organs 195, 222–231. doi: 10.1159/000324896
Piattelli, A., Scarano, A., Piattelli, M., Coraggio, F., and Matarasso, S. (2000). Bone regeneration using Bioglass: an experimental study in rabbit tibia. J. Oral Implantol. 26, 257–261. doi: 10.1563/1548-1336(2000)026<0257:BRUBAE>2.3.CO;2
Pravdyuk, A. I., Petrenko, Y. A., Fuller, B. J., and Petrenko, A. Y. (2013). Cryopreservation of alginate encapsulated mesenchymal stromal cells. Cryobiology 66, 215–222. doi: 10.1016/j.cryobiol.2013.02.002
Preethi Soundarya, S., Haritha Menon, A., Viji Chandran, S., and Selvamurugan, N. (2018). Bone tissue engineering: scaffold preparation using chitosan and other biomaterials with different design and fabrication techniques. Int. J. Biol. Macromol. 119, 1228–1239. doi: 10.1016/j.ijbiomac.2018.08.056
Probst, F. A., Fliefel, R., Burian, E., Probst, M., Eddicks, M., Cornelsen, M., et al. (2020). Bone regeneration of minipig mandibular defect by adipose derived mesenchymal stem cells seeded tri-calcium phosphate- poly(D,L-lactide-co-glycolide) scaffolds. Sci. Rep. 10:2062. doi: 10.1038/s41598-020-59038-8
Qasim, M., Chae, D. S., and Lee, N. Y. (2019). Advancements and frontiers in nano-based 3D and 4D scaffolds for bone and cartilage tissue engineering. Int. J. Nanomed. 14, 4333–4351. doi: 10.2147/IJN.S209431
Ramesh, N., Moratti, S. C., and Dias, G. J. (2018). Hydroxyapatite-polymer biocomposites for bone regeneration: a review of current trends. J. Biomed. Mater. Res. Part B Appl. Biomater 106, 2046–2057. doi: 10.1002/jbm.b.33950
Ramot, Y., Haim-Zada, M., Domb, A. J., and Nyska, A. (2016). Biocompatibility and safety of PLA and its copolymers. Adv. Drug Deliv. Rev. 107, 153–162. doi: 10.1016/j.addr.2016.03.012
Ren, L., Tsuru, K., Hayakawa, S., and Osaka, A. (2002). Novel approach to fabricate porous gelatin-siloxane hybrids for bone tissue engineering. Biomaterials 23, 4765–4773. doi: 10.1016/S0142-9612(02)00226-0
Ren, Z., Ma, S., Jin, L., Liu, Z., Liu, D., Zhang, X., et al. (2017). Repairing a bone defect with a three-dimensional cellular construct composed of a multi-layered cell sheet on electrospun mesh. Biofabrication 9:025036. doi: 10.1088/1758-5090/aa747f
Rothrauff, B. B., and Tuan, R. S. (2020). Decellularized bone extracellular matrix in skeletal tissue engineering. Biochem. Soc. Trans. 48, 755–764. doi: 10.1042/BST20190079
Rottensteiner-Brandl, U., Detsch, R., Sarker, B., Lingens, L., Kohn, K., Kneser, U., et al. (2018). Encapsulation of rat bone marrow derived mesenchymal stem cells in alginate dialdehyde/gelatin microbeads with and without nanoscaled bioactive glass for in vivo bone tissue engineering. Materials 11:1880. doi: 10.3390/ma11101880
Rustom, L. E., Poellmann, M. J., and Wagoner Johnson, A. J. (2019). Mineralization in micropores of calcium phosphate scaffolds. Acta Biomater 83, 435–455. doi: 10.1016/j.actbio.2018.11.003
Santoso, E. G., Yoshida, K., Hirota, Y., Aizawa, M., Yoshino, O., Kishida, A., et al. (2014). Application of detergents or high hydrostatic pressure as decellularization processes in uterine tissues and their subsequent effects on in vivo uterine regeneration in murine models. PLoS ONE 9:e103201. doi: 10.1371/journal.pone.0103201
Sartika, D., Wang, C. H., Wang, D. H., Cherng, J. H., Chang, S. J., Fan, G. Y., et al. (2020). Human adipose-derived mesenchymal stem cells-incorporated silk fibroin as a potential bio-scaffold in guiding bone regeneration. Polymers 12:853. doi: 10.3390/polym12040853
Sawkins, M. J., Bowen, W., Dhadda, P., Markides, H., Sidney, L. E., Taylor, A. J., et al. (2013). Hydrogels derived from demineralized and decellularized bone extracellular matrix. Acta Biomater. 9, 7865–7873. doi: 10.1016/j.actbio.2013.04.029
Sharifi, F., Atyabi, S. M., Norouzian, D., Zandi, M., Irani, S., and Bakhshi, H. (2018). Polycaprolactone/carboxymethyl chitosan nanofibrous scaffolds for bone tissue engineering application. Int. J. Biol. Macromol. 115, 243–248. doi: 10.1016/j.ijbiomac.2018.04.045
Sharmila, G., Muthukumaran, C., Kirthika, S., Keerthana, S., Kumar, N. M., and Jeyanthi, J. (2020). Fabrication and characterization of Spinacia oleracea extract incorporated alginate/carboxymethyl cellulose microporous scaffold for bone tissue engineering. Int. J. Biol. Macromol. 156, 430–437. doi: 10.1016/j.ijbiomac.2020.04.059
Shelton, R. M., Liu, Y., Cooper, P. R., Gbureck, U., German, M. J., and Barralet, J. E. (2006). Bone marrow cell gene expression and tissue construct assembly using octacalcium phosphate microscaffolds. Biomaterials 27, 2874–2881. doi: 10.1016/j.biomaterials.2005.12.031
Shive, M. S., and Anderson, J. M. (1997). Biodegradation and biocompatibility of PLA and PLGA microspheres. Adv. Drug Deliv. Rev. 28, 5–24. doi: 10.1016/S0169-409X(97)00048-3
Shue, L., Yufeng, Z., and Mony, U. (2012). Biomaterials for periodontal regeneration: a review of ceramics and polymers. Biomatterials 2, 271–277. doi: 10.4161/biom.22948
Shui, W., Zhang, W., Yin, L., Nan, G., Liao, Z., Zhang, H., et al. (2014). Characterization of scaffold carriers for BMP9-transduced osteoblastic progenitor cells in bone regeneration. J. Biomed. Mater. Res. A 102, 3429–3438. doi: 10.1002/jbm.a.35006
Siddiqui, H. A., Pickering, K. L., and Mucalo, M. R. (2018). A review on the use of hydroxyapatite-carbonaceous structure composites in bone replacement materials for strengthening purposes. Materials 11:1813. doi: 10.3390/ma11101813
Singh, R. K., Awasthi, S., Dhayalan, A., Ferreira, J. M., and Kannan, S. (2016). Deposition, structure, physical and in vitro characteristics of Ag-doped β-Ca3(PO4)2/chitosan hybrid composite coatings on titanium metal. Mater. Sci. Eng. C Mater. Biol. Appl. 62, 692–701. doi: 10.1016/j.msec.2016.02.013
Smith, C. A., Richardson, S. M., Eagle, M. J., Rooney, P., Board, T., and Hoyland, J. A. (2015). The use of a novel bone allograft wash process to generate a biocompatible, mechanically stable and osteoinductive biological scaffold for use in bone tissue engineering. J. Tissue Eng. Regen. Med. 9, 595–604. doi: 10.1002/term.1934
Smith, E. L., Kanczler, J. M., Gothard, D., Roberts, C. A., Wells, J. A., White, L. J., et al. (2014). Evaluation of skeletal tissue repair, part 2: enhancement of skeletal tissue repair through dual-growth-factor-releasing hydrogels within an ex vivo chick femur defect model. Acta Biomater. 10, 4197–4205. doi: 10.1016/j.actbio.2014.05.025
Sun, L., Parker, S. T., Syoji, D., Wang, X., Lewis, J. A., and Kaplan, D. L. (2012). Direct-write assembly of 3D silk/hydroxyapatite scaffolds for bone co-cultures. Adv. Healthc. Mater. 1, 729–735. doi: 10.1002/adhm.201200057
Suzuki, O. (2010). Octacalcium phosphate: osteoconductivity and crystal chemistry. Acta Biomater. 6, 3379–3387. doi: 10.1016/j.actbio.2010.04.002
Suzuki, O., Shiwaku, Y., and Hamai, R. (2020). Octacalcium phosphate bone substitute materials: comparison between properties of biomaterials and other calcium phosphate materials. Dent. Mater. J. 39, 187–199. doi: 10.4012/dmj.2020-001
Tan, M. L., Shao, P., Friedhuber, A. M., van Moorst, M., Elahy, M., Indumathy, S., et al. (2014). The potential role of free chitosan in bone trauma and bone cancer management. Biomaterials 35, 7828–7838. doi: 10.1016/j.biomaterials.2014.05.087
Thibault, R. A., Scott Baggett, L., Mikos, A. G., and Kasper, F. K. (2010). Osteogenic differentiation of mesenchymal stem cells on pregenerated extracellular matrix scaffolds in the absence of osteogenic cell culture supplements. Tissue Eng. Part A 16, 431–440. doi: 10.1089/ten.tea.2009.0583
Toole, B. P. (2004). Hyaluronan: from extracellular glue to pericellular cue. Nat. Rev. Cancer 4, 528–539. doi: 10.1038/nrc1391
Tortelli, F., and Cancedda, R. (2009). Three-dimensional cultures of osteogenic and chondrogenic cells: a tissue engineering approach to mimic bone and cartilage in vitro. Eur. Cell. Mater. 17, 1–14. doi: 10.22203/eCM.v017a01
Townsend, J. M., Dennis, S. C., Whitlow, J., Feng, Y., Wang, J., Andrews, B., et al. (2017). Colloidal gels with extracellular matrix particles and growth factors for bone regeneration in critical size rat calvarial defects. AAPS J. 19, 703–711. doi: 10.1208/s12248-017-0045-0
Turunen, T., Peltola, J., Helenius, H., Yli-Urpo, A., and Happonen, R. P. (1997). Bioactive glass and calcium carbonate granules as filler material around titanium and bioactive glass implants in the medullar space of the rabbit tibia. Clin. Oral Implants Res. 8, 96–102. doi: 10.1034/j.1600-0501.1997.080204.x
Tuukkanen, J., and Nakamura, M. (2017). Hydroxyapatite as a nanomaterial for advanced tissue engineering and drug therapy. Curr. Pharm. Des. 23, 3786–3793. doi: 10.2174/1381612823666170615105454
Tyler, B., Gullotti, D., Mangraviti, A., Utsuki, T., and Brem, H. (2016). Polylactic acid (PLA) controlled delivery carriers for biomedical applications. Adv. Drug Deliv. Rev. 107, 163–175. doi: 10.1016/j.addr.2016.06.018
Udomluck, N., Koh, W. G., Lim, D. J., and Park, H. (2019). Recent developments in nanofiber fabrication and modification for bone tissue engineering. Int. J. Mol. Sci 21:99. doi: 10.3390/ijms21010099
Ulery, B. D., Nair, L. S., and Laurencin, C. T. (2011). Biomedical applications of biodegradable polymers. J. Polym. Sci. B Polym. Phys. 49, 832–864. doi: 10.1002/polb.22259
Urist, M. R., Nilsson, O., Rasmussen, J., Hirota, W., Lovell, T., Schmalzreid, T., et al. (1987). Bone regeneration under the influence of a bone morphogenetic protein (BMP) beta tricalcium phosphate (TCP) composite in skull trephine defects in dogs. Clin. Orthop. Relat. Res. 214, 295–304. doi: 10.1097/00003086-198701000-00041
Velioglu, Z. B., Pulat, D., Demirbakan, B., Ozcan, B., Bayrak, E., and Erisken, C. (2019). 3D-printed poly(lactic acid) scaffolds for trabecular bone repair and regeneration: scaffold and native bone characterization. Connect. Tissue Res. 60, 274–282. doi: 10.1080/03008207.2018.1499732
Venkatesan, J., Bhatnagar, I., Manivasagan, P., Kang, K. H., and Kim, S. K. (2015). Alginate composites for bone tissue engineering: a review. Int. J. Biol. Macromol. 72, 269–281. doi: 10.1016/j.ijbiomac.2014.07.008
Venkatesan, J., Nithya, R., Sudha, P. N., and Kim, S. K. (2014). Role of alginate in bone tissue engineering. Adv. Food Nutr. Res. 73, 45–57. doi: 10.1016/B978-0-12-800268-1.00004-4
Wang, C., Huang, W., Zhou, Y., He, L., He, Z., Chen, Z., et al. (2020a). 3D printing of bone tissue engineering scaffolds. Bioact. Mater. 5, 82–91. doi: 10.1016/j.bioactmat.2020.01.004
Wang, C., Jeong, K. J., Park, H. J., Lee, M., Ryu, S. C., Hwang, D. Y., et al. (2020b). Synthesis and formation mechanism of bone mineral, whitlockite nanocrystals in tri-solvent system. J. Colloid Interface Sci. 569, 1–11. doi: 10.1016/j.jcis.2020.02.072
Wang, H., Li, Y., Zuo, Y., Li, J., Ma, S., and Cheng, L. (2007). Biocompatibility and osteogenesis of biomimetic nano-hydroxyapatite/polyamide composite scaffolds for bone tissue engineering. Biomaterials 28, 3338–3348. doi: 10.1016/j.biomaterials.2007.04.014
Wang, L., and Stegemann, J. P. (2010). Thermogelling chitosan and collagen composite hydrogels initiated with beta-glycerophosphate for bone tissue engineering. Biomaterials 31, 3976–3985. doi: 10.1016/j.biomaterials.2010.01.131
Wang, Q., Wang, M. H., Wang, K. F., Liu, Y., Zhang, H. P., Lu, X., et al. (2015). Computer simulation of biomolecule-biomaterial interactions at surfaces and interfaces. Biomed. Mater. 10:032001. doi: 10.1088/1748-6041/10/3/032001
Wang, Y., Van Manh, N., Wang, H., Zhong, X., Zhang, X., and Li, C. (2016). Synergistic intrafibrillar/extrafibrillar mineralization of collagen scaffolds based on a biomimetic strategy to promote the regeneration of bone defects. Int. J. Nanomed. 11, 2053–2067. doi: 10.2147/IJN.S102844
Wilson, J., Pigott, G. H., Schoen, F. J., and Hench, L. L. (1981). Toxicology and biocompatibility of bioglasses. J. Biomed. Mater. Res. 15, 805–817. doi: 10.1002/jbm.820150605
Xiao, D., Zhang, J., Zhang, C., Barbieri, D., Yuan, H., Moroni, L., et al. (2020). The role of calcium phosphate surface structure in osteogenesis and the mechanisms involved. Acta Biomater. 106, 22–33. doi: 10.1016/j.actbio.2019.12.034
Xynos, I. D., Hukkanen, M. V., Batten, J. J., Buttery, L. D., Hench, L. L., and Polak, J. M. (2000). Bioglass 45S5 stimulates osteoblast turnover and enhances bone formation In vitro: implications and applications for bone tissue engineering. Calcif. Tissue Int. 67, 321–329. doi: 10.1007/s002230001134
Yan, J., Yang, L., Wang, G., Xiao, Y., Zhang, B., and Qi, N. (2010). Biocompatibility evaluation of chitosan-based injectable hydrogels for the culturing mice mesenchymal stem cells in vitro. J. Biomater. Appl. 24, 625–637. doi: 10.1177/0885328208100536
Yan, S., Zhang, R., Wu, K., Cui, J., Huang, S., Ji, X., et al. (2018). Characterization of the essential role of bone morphogenetic protein 9 (BMP9) in osteogenic differentiation of mesenchymal stem cells (MSCs) through RNA interference. Genes Dis. 5, 172–184. doi: 10.1016/j.gendis.2018.04.006
Yanagisawa, T., Yasuda, A., Makkonen, R. I., and Kamakura, S. (2020). Influence of pre-freezing conditions of octacalcium phosphate and collagen composite for reproducible appositional bone formation. J. Biomed. Mater. Res. Part B Appl. Biomater. 108, 2827–2834. doi: 10.1002/jbm.b.34613
Yang, J., Long, T., He, N. F., Guo, Y. P., Zhu, Z. A., and Ke, Q. F. (2014a). Fabrication of a chitosan/bioglass three-dimensional porous scaffold for bone tissue engineering applications. J. Mater. Chem. B 2, 6611–6618. doi: 10.1039/C4TB00940A
Yang, J., van Lith, R., Baler, K., Hoshi, R. A., and Ameer, G. A. (2014b). A thermoresponsive biodegradable polymer with intrinsic antioxidant properties. Biomacromolecules 15, 3942–3952. doi: 10.1021/bm5010004
Yao, C. H., Liu, B. S., Hsu, S. H., Chen, Y. S., and Tsai, C. C. (2004). Biocompatibility and biodegradation of a bone composite containing tricalcium phosphate and genipin crosslinked gelatin. J. Biomed. Mater. Res. A 69, 709–717. doi: 10.1002/jbm.a.30045
Younes, I., and Rinaudo, M. (2015). Chitin and chitosan preparation from marine sources. Structure, properties and applications. Mar Drugs 13, 1133–1174. doi: 10.3390/md13031133
Yuan, H., De Bruijn, J. D., Li, Y., Feng, J., Yang, Z., De Groot, K., et al. (2001). Bone formation induced by calcium phosphate ceramics in soft tissue of dogs: a comparative study between porous alpha-TCP and beta-TCP. J. Mater. Sci. Mater. Med. 12, 7–13. doi: 10.1023/A:1026792615665
Zhang, L., Luo, Q., Shu, Y., Zeng, Z., Huang, B., Feng, Y., et al. (2019). Transcriptomic landscape regulated by the 14 types of bone morphogenetic proteins (BMPs) in lineage commitment and differentiation of mesenchymal stem cells (MSCs). Genes Dis. 6, 258–275. doi: 10.1016/j.gendis.2019.03.008
Zhang, X., Reagan, M. R., and Kaplan, D. L. (2009). Electrospun silk biomaterial scaffolds for regenerative medicine. Adv. Drug Deliv. Rev. 61, 988–1006. doi: 10.1016/j.addr.2009.07.005
Zhao, C., Qazvini, N. T., Sadati, M., Zeng, Z., Huang, S., De La Lastra, A. L., et al. (2019). A pH-triggered, self-assembled, and bioprintable hybrid hydrogel scaffold for mesenchymal stem cell based bone tissue engineering. ACS Appl. Mater. Interfaces 11, 8749–8762. doi: 10.1021/acsami.8b19094
Zhao, C., Zeng, Z., Qazvini, N. T., Yu, X., Zhang, R., Yan, S., et al. (2018). Thermoresponsive citrate-based graphene oxide scaffold enhances bone regeneration from bmp9-stimulated adipose-derived mesenchymal stem cells. ACS Biomater. Sci. Eng. 4, 2943–2955. doi: 10.1021/acsbiomaterials.8b00179
Zhao, N., Wang, X., Qin, L., Zhai, M., Yuan, J., Chen, J., et al. (2016). Effect of hyaluronic acid in bone formation and its applications in dentistry. J. Biomed. Mater. Res. A 104, 1560–1569. doi: 10.1002/jbm.a.35681
Keywords: bone tissue engineering, biomaterials—cells, decellularizate ECM, polymer, stem cells—cartilage—bone marrow stem cells clinical application, mesenchymal stem cell
Citation: Zhang Y, Wu D, Zhao X, Pakvasa M, Tucker AB, Luo H, Qin KH, Hu DA, Wang EJ, Li AJ, Zhang M, Mao Y, Sabharwal M, He F, Niu C, Wang H, Huang L, Shi D, Liu Q, Ni N, Fu K, Chen C, Wagstaff W, Reid RR, Athiviraham A, Ho S, Lee MJ, Hynes K, Strelzow J, He T-C and El Dafrawy M (2020) Stem Cell-Friendly Scaffold Biomaterials: Applications for Bone Tissue Engineering and Regenerative Medicine. Front. Bioeng. Biotechnol. 8:598607. doi: 10.3389/fbioe.2020.598607
Received: 25 August 2020; Accepted: 27 November 2020;
Published: 14 December 2020.
Edited by:
Bin Li, Soochow University, ChinaReviewed by:
Joaquim Miguel Oliveira, University of Minho, PortugalCopyright © 2020 Zhang, Wu, Zhao, Pakvasa, Tucker, Luo, Qin, Hu, Wang, Li, Zhang, Mao, Sabharwal, He, Niu, Wang, Huang, Shi, Liu, Ni, Fu, Chen, Wagstaff, Reid, Athiviraham, Ho, Lee, Hynes, Strelzow, He and El Dafrawy. This is an open-access article distributed under the terms of the Creative Commons Attribution License (CC BY). The use, distribution or reproduction in other forums is permitted, provided the original author(s) and the copyright owner(s) are credited and that the original publication in this journal is cited, in accordance with accepted academic practice. No use, distribution or reproduction is permitted which does not comply with these terms.
*Correspondence: Tong-Chuan He, dGNoZUB1Y2hpY2Fnby5lZHU=; Mostafa El Dafrawy, bWVsZGFmcmF3eUBic2QudWNoaWNhZ28uZWR1
Disclaimer: All claims expressed in this article are solely those of the authors and do not necessarily represent those of their affiliated organizations, or those of the publisher, the editors and the reviewers. Any product that may be evaluated in this article or claim that may be made by its manufacturer is not guaranteed or endorsed by the publisher.
Research integrity at Frontiers
Learn more about the work of our research integrity team to safeguard the quality of each article we publish.