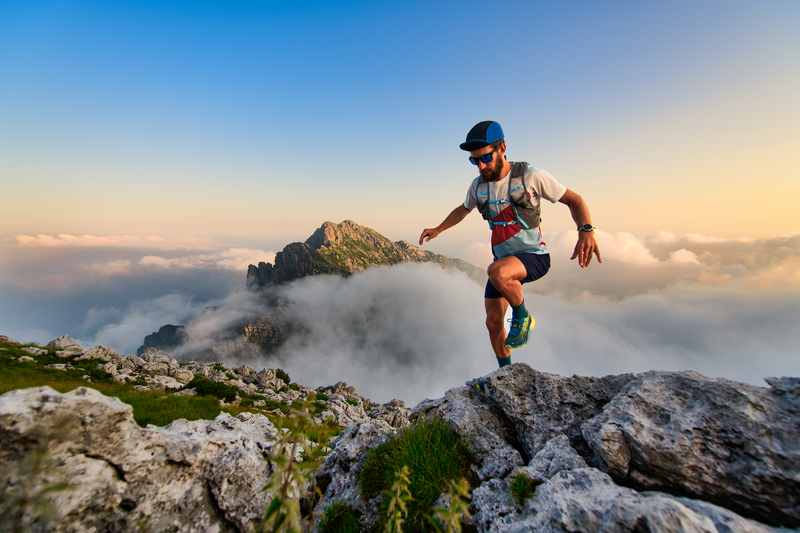
95% of researchers rate our articles as excellent or good
Learn more about the work of our research integrity team to safeguard the quality of each article we publish.
Find out more
MINI REVIEW article
Front. Bioeng. Biotechnol. , 07 October 2020
Sec. Biomaterials
Volume 8 - 2020 | https://doi.org/10.3389/fbioe.2020.591467
This article is part of the Research Topic Biomaterials for Engineering Cellular Environments in Tissue Engineering View all 15 articles
Bioceramics have experienced great development over the past 50 years. Modern bioceramics are designed to integrate bioactive ions within ceramic granules to trigger living tissue regeneration. Preclinical and clinical studies have shown that strontium is a safe and effective divalent metal ion for preventing osteoporosis, which has led to its incorporation in calcium phosphate-based ceramics. The local release of strontium ions during degradation results in moderate concentrations that trigger osteogenesis with few systemic side effects. Moreover, strontium has been proven to generate a favorable immune environment and promote early angiogenesis at the implantation site. Herein, the important aspects of strontium-enriched calcium phosphate bioceramics (Sr-CaPs), and how Sr-CaPs affect the osteogenic microenvironment, are described.
Bone is a metabolically active specialized connective tissue with the capacity for continuous resorption and reformation, but the reconstruction process requires extra support in large bone defect cases, for example, injury, tumor excision, and age-related diseases (Loi et al., 2016; Ginebra et al., 2018). Clinically, autografts still remain the most common strategy to support and stimulate bone growth. While biologically desirable, the supply of autografts from a patient’s own body cannot meet the amount required for large-sized bone defects (Wang and Yeung, 2017). Grafts from bone banks or other animal species can provide a vast range of grafting forms by virtue of their relatively easy availability (Wang and Yeung, 2017). Nevertheless, they are still subject to attenuated osteointegration and disease transmission.
Synthetic bioactive ceramics can overcome these limitations. They are obtained by chemical reagent deposition through a controlled process that allows their properties to be adapted to the specific requirements of different clinical situations. Calcium phosphate ceramics (CaPs) are basic bone repair materials with excellent osteoinductive and osteoconductive features; examples include hydroxyapatite (HAP), β-tricalcium phosphate (β-TCP), calcium polyphosphate (CPP), and biphasic calcium phosphate (BCP). They have been widely used in clinical applications in the form of implant surface coatings, the composition of cements, and scaffolds (Jeong et al., 2019). Moreover, CaPs encapsulated with pharmacologics and biologics in scaffolds have shown multiple abilities, such as enhanced osteointegration, angiogenesis, and antibacterial properties (Bose and Tarafder, 2012; Marques et al., 2016). However, these biologically active molecules are only surface-loaded and show explosive release effects with little long-term benefit (Wu et al., 2014). This raises some concerns about their safety. For example, recombinant human bone morphogenetic protein-2 (rhBMP-2) is the first commercial synthetic osteoinductive molecule, and the combined use of rhBMP-2 and calcium phosphate ceramics has achieved many clinical successes. But it is worth noting that serious side effects related to ectopic or unwanted bone formation in certain situations have made the FDA increasingly resistant to approving the use of such materials (Vavken et al., 2016).
An alternative long-lasting and potentially safer strategy is the incorporation of trace metallic elements into CaPs. The mineral composition of our bone itself is not a homogenous and bioinert calcium phosphate-based material (Von Euw et al., 2019). Bone incorporates and releases various trace elements (such as Mg2+, Zn2+, and Sr2+) into the microenvironment during bone metabolism (Bose et al., 2013). Previous studies have confirmed that CaPs modified with these bivalent trace metallic ions can lead to controlled degradation, increase the mechanical strength of the materials, and enhance bioactive properties. But among these bioactive ions, strontium (Sr) has gained great attention since strontium ranelate (SrRan) has been approved as an anti-osteoporotic drug for post-menopausal osteoporosis since it increases bone strength (Neves et al., 2017; Marx et al., 2020). Recent findings suggest that oral administration of SrRan may have no anabolic action on bone formation in humans, and even inhibits osteogenic differentiation under the in vitro experiment conditions (Wornham et al., 2014; Marx et al., 2020). However, the comprehensively described SrCaPs do exert positively influence on new bone formation and accelerate the healing process (Neves et al., 2017). These discrepancies may be partially due to the implant microenvironments where biomaterials interact with various cells. The local delivery of Sr ions from SrCaPs implanted in bone changes local microenvironment, which is involved in several biological processes such as osteogenesis, angiogenesis, osteoimmunomodulation.
Consequently, in this review, we present recent developments in strontium-substituted calcium phosphate ceramics (SrCaPs) and offer insights into how Sr ions released from implants influence the immune response, angiogenesis, and osteoblastic differentiation of bone marrow stromal cells (bMSCs).
In the 1960s, surgeons valued the inertness of surgical materials in the human tissue environment, and first-generation bioceramics (alumina, zirconia, diverse forms of carbon) were developed as bone substitutes, mainly for femoral head fabrication (Smith, 1963). However, they elicited a foreign body reaction, forming fibrous capsules that isolated them from the body. In the 1980s, Larry Hench invented a bioactive glass with the ability to bond to living tissue, and Heughebaert reported that CaPs could induce bone formation (Hench and Wilson, 1984; Heughebaert et al., 1988). Since then, osteoinductive and tissue-inducing materials have become the mainstream of biomaterial science and engineering (Hench and Polak, 2002). CaPs are the most studied and implanted bioactive ceramics in the clinic because of their cost-effective preparation from chemical regents or natural resources. Various calcium phosphate compounds are in different crystalline phases and often classified on the basis of the Ca/P ratio. Of great interest is the subgroup of apatite compounds, which exhibit similarities to vertebrate hard tissues. Indeed, both bone and teeth are well-crystallized apatites (hydroxyapatite), and this structure can easily accommodate many cations and anions for substitution, thereby achieving adaptive biophysical functions (Drouet et al., 2017).
Strontium (Sr) is naturally deposited in the mineral phase of bones via consuming a normal diet, and it replaces approximately 0.035% of Ca content (International Programme on Chemical and Inter-Organization Programme for the Sound Management, 2010). The majority of in vitro experiments support a dual-acting mechanism in which Sr stimulates bone formation and hinders bone resorption (Saidak and Marie, 2012). The exact mechanism of the role of Sr in bone remains unclear, but it has been proposed that Sr acts on similar cellular targets as Ca2+ by activating the calcium sensing receptor (CaSR), thus interacting with Ca-driven signaling pathways related to bone metabolism regulation (Saidak and Marie, 2012). Furthermore, small animal trials and clinical trials have produced overwhelming evidence that Sr benefits bone remodeling and increases bone-mineral density, especially in the treatment of osteoporosis (Meunier et al., 2004; Reginster et al., 2012; Marx et al., 2020). Taking these beneficial effects into consideration, it is likely that local Sr ion delivery will enhance osteoinduction and osseointegration at the bone-implant interface, contributing to a faster healing microenvironment.
Recently, Sr has been widely incorporated into calcium phosphate-based materials for biomedical applications. Krishnan reported using Sr doped hydroxyapatite (SrHAP) particles to repair dental enamel with the potential to treat white spot lesions and incipient carious lesions (Krishnan et al., 2016). It is also an effective additive for toothpaste in the prevention of cariogenesis (Surdacka et al., 2007). These particles cannot only be used as a raw material, but also associated with other biomaterial compounds to facilitate new functions. Sr-CaPs are fabricated as coatings on the surfaces of metallic implants in order to accelerate bone healing at early implantation times (Li et al., 2010; Arcos and Vallet-Regí, 2020). Titanium and titanium-based alloys are commonly used for load-bearing applications (such as total joint replacement and fracture fixation elements), but carry a risk of loosening, especially when implanted in osteoporotic bones. Tao et al. (2016a) conducted an in vivo study on rats with ovariectomy-induced osteoporosis and reported that a strontium-containing HAP coating on shaped titanium implants was better than an Sr-free HAP coating in terms of new bone formation and push-out force. Furthermore, they conducted an comparative study that found that under the same the molar ratio [Zn, Mg, Sr/(Zn, Mg, Sr+Ca) = 10%], the SrHAP coating exhibited better osseointegration than zinc- and magnesium-substituted HAP coatings (Tao et al., 2016b).
SrCaP cements (SrCPC) consist of a combination of a precursor powder and liquid phase, allowing it to fill and heal bone defects during minimally invasive procedures. Kuang et al. (2015) prepared pre-set SrCPC for a metaphyseal defect in Sprague-Dawley rats and found early endochondral ossification at 2 weeks post-operation. Thormann et al. (2013) further demonstrated that Sr was deposited at newly formed tissues around the implanted SrCPC as visualized by Time-of-flight secondary ion mass spectrometry (ToF-SIMS) imaging and found a significant increase of mineralized extracellular matrix in the SrCPC group compared to the Sr-free calcium phosphate cement (CPC) and empty defect groups. Sr-CaP scaffolds can be further modified with drugs, especially antibiotics and growth factors. The chemical composition of SrHAP provides strong surface adsorption capacity and increases surface area, allowing drug release for a longer period of time (Xu et al., 2018). Lin et al. (2013) reported that SrHAP microspheres with 3D hierarchically mesoporous structures not only promoted an osteogenic response, but exhibited sustained vancomycin release. Yan et al. (2018) developed an Sr-nHAP/SF-Hep-BMP-2 scaffold system with controlled BMP-2 release that supported critical size calvarial defect healing in Sprague-Dawley rats. Tao et al. (2018) revealed synergistic bioactive properties of BMP-2 and Sr released from CPC composites, which achieved rapid bone healing in osseous defects in an ovariectomized rat model. These new applications for strontium-containing calcium phosphate extends their biomedical potential.
Sr is chemically and physically close to calcium but has a larger ionic radius (112 vs. 99 pm). The partial substitution of Ca by Sr results in higher solubility when compared with Sr-free CaPs owning to the enlargement of the unit cell (Zhu et al., 2018). The surfaces of SrCaPs are reactive and biodegradable, and bone-like apatite forms on them through continuous dissolution and precipitation. The newly formed apatite creates a strong bond between the living tissues and implants. However, it degrades over time when in contact with bodily fluids and it elevates the local concentrations of Sr2+, Ca2+, and PO43– ions. These released ions affect important components of the bone microenvironment, including the mineral deposited layer phase, the cellular phase (osteoblasts, macrophages, and vascular endothelial cells), and the soluble factor phase (growth factors and/or cytokines) and provide an extracellular osteogenic signaling network. The incorporation of an appropriate proportion of Sr into CaPs allows them to build an osteogenic microenvironment via modulating the inflammatory response, stimulating osteogenic differentiation of bone marrow mesenchymal stem cells, and promoting early angiogenesis (Figure 1).
Figure 1. Schematic demonstrating the microenvironment of the host bone-implant interface. The degradation-precipitation reactions of bioactive Sr-CaPs modulate local ion concentrations and influence peripheral physiological processes, including ① hMSC osteogenic differentiation, ② immune responses such as macrophage polarization to M2, and ③ revascularization processes.
SrCaPs is a promising biomaterial to help bone reconstruction. Mounting evidence shows that Sr replacement of Ca in CaPs leads to increased solubility due to the expansion of the crystal structure, and the high local Sr2+, Ca2+, and PO43– ionic concentrations are believed to be of great importance for osteoinduction and osteoconduction (Bose et al., 2013; Neves et al., 2017). Current research shows that both Sr and Ca mediate key cellular functions in osteogenesis-related cells via Ca-sensing receptor (CaSR)-dependent mechanisms (Marx et al., 2020). In the extracellular microenvironment, a high Ca concentration is a potent chemical signal for bMSCs osteogenic differentiation (Dvorak and Riccardi, 2004; Barradas et al., 2012). A lack of calcium significantly retards cell growth and differentiation (Dvorak et al., 2004; Barradas et al., 2012). Interestingly, CaPs with Sr modification tend to have decreased Ca concentrations around the material due to induced apatite precipitation, though lower concentrations of Ca do not impair the proliferation or osteogenic differentiation of bMSCs (Schumacher et al., 2013; Kruppke et al., 2019b). In an in vitro study, Kruppke et al. (2019a) confirmed that Sr may compensate for the adverse effects of lowered Ca ion concentrations to a certain degree and potentially stimulate bone regeneration. They investigated the effect of varied Ca and Sr ion concentrations, respectively, on proliferation and differentiation of bMSCs and concluded that under low calcium concentrations culture conditions (0.3–0.4 mM due to the addition of fetal calf serum), Sr at 0.4–0.9 mM promoted differentiation of bMSCs while 0.9–1.8 mM stimulated the highest proliferation. And in a second study exploring the relationship between Sr and Ca on bone formation, they conducted in vitro and in vivo experiments to evaluate the osteogenesis effects of Sr together with two concentration gradients of Ca (normal calcium of 1.8 mM or high calcium of 9 mM) and concluded that Sr (1 mM) enhanced mineralization and ALP expression of MC3T3-E1 pre-osteoblast cells under a high dose of calcium (9 mM) (Xie et al., 2018). This may explain the results by Wornham et al. (2014) in which concentrations of Sr ranging from 10 μM to 1 mM inhibit the mineralization ability of primary rat osteoblasts under 1.8 mM Ca present in the culture system. These experiments illustrate the importance of the implant microenvironment because bone has higher Ca levels than plasma. It also suggests that both Sr and Ca from ion-leaching SrCaPs may interact to promote bone regeneration at the bone-implant interface. In addition, the phosphate ion is a basic component in the human body and accumulates in bone minerals in the form of calcium phosphates along with calcium ions (Khoshniat et al., 2011). When phosphate ions are not present in high quantities, the generation of new bone will be blocked due to insufficient formation of hydroxyapatite (Penido and Alon, 2012).
Sr-rich CaPs consistently outperform Sr-free CaPs in in vitro and in vivo studies (Neves et al., 2017; Marx et al., 2020). In the absence of doping with other osteogenic ions, the local release of Sr is sufficient to promote bone formation, but it is difficult to ignore the effects that surface morphology, porosity, hydrophilicity, dissolution process, and local pH changes may have on osteogenesis regulation at the implantation site.
Increased angiogenesis can relieve the symptoms of ischemia and facilitate the transport nutrients, which is essential for bone repair/regeneration. Sr-CaPs have been found to increase neovascularization in many convincing experiments (Liu et al., 2011; Gu et al., 2013; Wang et al., 2014). Endothelial cells are the major seed cells that drive angiogenesis. Chen et al. (2008) observed that degradation products of Sr doped calcium polyphosphate (SCPPs) promote the proliferation, migration, and tube-like structure formation of endothelial cells (ECV304). It has been reported that in situ production of angiogenic growth factors, such as vascular endothelial growth factor (VEGF) and basic fibroblast growth factor (bFGF), can promote more cell infiltration, extracellular matrix synthesis, and faster angiogenesis (Saberianpour et al., 2018). Liu et al. (2011) directly seeded osteoblasts (ROS17/2.8) on SCPP scaffolds with various amounts of Sr and found increased secretion of VEGF and bFGF in the culture medium. Wang et al. (2014) observed the stimulating effects of SCPP on protein secretion and mRNA expression of VEGF, bFGF, and MMP-2 from endothelial cells in vitro. Gu et al. (2014) co-cultured umbilical vein endothelial cells and osteoblasts on SCPP scaffolds in vitro and demonstrated higher VEGF and bFGF protein production compared with CPP and HAP. Furthermore, Ye et al. (2019) fabricated Sr-doped calcium phosphate/polycaprolactone/chitosan (Sr-CaP/PCL/CS) nanohybrid fibrous membranes and demonstrated high VEGF secretion from bMSCs. In the future, more experiments are needed to test the ability of SrCaPs to promote blood vessel formation in vivo, and more attention should be paid to the impact of the immune response.
Every implantable material must be biocompatible, meaning that only a limited inflammatory response occurs in the host body. Huang et al. (2020) reported the incorporation of fish-derived nano CaPs (Ca/P molar ratio was 2.35) into gelatin methacrylate generated a suitable immune microenvironment by inducing the secretion of cytokines and promoting macrophage phenotype conversion. The controllable inflammation may promote the osteogenic differentiation and angiogenesis (Zhao et al., 2018; Huang et al., 2020). The local release of Sr from implants to the adjacent host bone can reduce unfavorable inflammatory responses (Renaudin et al., 2008), and Sr has been proven to be an anti-inflammatory agent (Wang et al., 2020). Buache et al. (2012) first verified that Sr-BCP can decrease the production of pro-inflammatory cytokines (TNF-α and IL-6) and the chemokine interleukin 8 in human monocytes. Braux et al. (2016) demonstrated the downregulating effect of Sr-BCP on inflammatory mediator production (MCP-1 and Gro-α) by human primary osteoblasts. Sr has also been shown to suppress the expression of proinflammatory cytokines (IL-6 and TNF-α) in periodontal ligament cells and macrophages (Römer et al., 2012; Gu et al., 2014). Among various immune-related cells, macrophages play a significant role in regulating bone healing after trauma or the implantation of biomaterials (Alexander et al., 2011; Batoon et al., 2019). At the early time of bone repair, resident or infiltrating monocyte-derived macrophages mostly present the pro-inflammatory M1 phenotype, and a timely switch from M1 to M2 (anti-inflammatory phenotype) leads to osteogenic cytokine release, including that of IL-10, TGFβ, and VEGF. Interestingly, prolonged M1 polarization leads to an increased release of fiber-enhancing cytokines from M2 macrophages, which leads to the formation of fibrous capsules (Chen et al., 2016). Previous evidence suggests that Sr-integrated implants elicit more pro-regenerative M2 macrophages; examples include Sr-doped calcium polyphosphate particles, Sr-substituted bioactive glass, and Ca- and Sr-modified titanium implant surfaces (Gu et al., 2014; Lee et al., 2016; Zhao et al., 2018). The incorporation of Sr into CaPs endows traditional CaPs with osteoimmunomodulation properties and positively regulates cytokine production and immune cell functions for better bone remodeling.
Local Sr release is a critical property of SrCaPs, but the optimal concentration remains to be determined. Evidence from in vitro studies shows that Sr promotes osteogenic differentiation in a dose-dependent manner, and different cell lineages respond differently to Sr at similar concentrations (Table 1). Peng et al. (2009) showed that 1 mM can promote osteogenic differentiation of C3H10T1/2 or mice primary bMSCs, while Aimaiti et al. (2017) reported that 1 mM induces apoptosis and inhibits osteogenic differentiation in human primary adipose-derived stem cells. Moreover, inhibition at this concentration also occurs for primary rat osteoblastic cells and MC3T3-E1 cells (Wornham et al., 2014; Xie et al., 2018). However, the maximum circulating concentrations of Sr in the serum of patients treated with strontium ranelate (2 g per day for 3 years) is about 0.1 mM (Meunier et al., 2004). Although several in vitro studies have shown that Sr concentration above 1 mM can stimulate osteogenesis, it is preferable to take clinical trial results into consideration, and the effective range (below 500 mM) is recommended here (Bonnelye et al., 2008; Peng et al., 2009; Guo et al., 2016). To the best of our knowledge, no in vivo study on the local administration of Sr with the use of modified CaPs has reported adverse effects on bone formation and osseointegration. The reasons may be that, first, the implantation microenvironment in the body is dynamically changing, and a high dose of Sr does not last for a long time. Second, the degradation product is not only Sr, but also Ca, which matters because of the equilibrium effect of Ca to Sr (Xie et al., 2018). Third, Sr positively modulates the microenvironment via regulating immune cells and endothelial cells in the process of bone formation.
In summary, integrating Sr ions into CaPs offers an alternative to biologics in the design of bioactive materials. It is of low cost, has a longer shelf life, and presents low systemic risk compared to growth factors. These added benefits make Sr as therapeutic agent attractive in tissue engineering and regenerative medicine applications (Jiménez et al., 2019; Prabha et al., 2019; Mao et al., 2020). However, the mechanism of osteoinduction of SrCaPs remains complicated because inflammation and angiogenesis accompany the entire process of bone healing. In light of the previous studies, we propose a hypothesis for the osteoinduction mechanism of SrCaPs: SrCaPs build the osteoinductive microenvironment of the host bone-implant interface (Figure 2). Functional ion release and apatite layer formation on the surfaces of SrCaP ceramics allow them to interact with cells and extracellular matrices in the host system, providing bioactive bonding to bone. Specifically, a higher local concentration of Sr stimulates the osteoblastic differentiation of MSCs (1 in Figure 1), induces macrophage polarization toward a pro-regenerative M2 phenotype (2 in Figure 1), and contributes to angiogenesis (3 in Figure 1). Collectively, understanding the biological microenvironment of implant-to-tissue interactions at the bone site is important for the development of high-performance bioceramics.
Figure 2. Hypothesis for osteogenic microenvironments of SrCaPs. The local ionic environment not only stimulates the osteoblastic differentiation of bMSCs but interacts with the inflammatory cells and vascular endothelial cells.
BW was the first author and drafted the manuscript. DC and RW designed the work. YS, JG, JC, and HW summarized related publications. All authors approved the final version to be published.
This work was financially supported by the National Natural Science Foundation of China (51973021 and 51932002), the Beijing Natural Science Foundation (7192027), and the Beijing Municipal Health Commission (BMHC-2019-9, BMHC-2018-4, and PXM2020_026275_000002).
The authors declare that the research was conducted in the absence of any commercial or financial relationships that could be construed as a potential conflict of interest.
Aimaiti, A., Maimaitiyiming, A., Boyong, X., Aji, K., Li, C., and Cui, L. (2017). Low-dose strontium stimulates osteogenesis but high-dose doses cause apoptosis in human adipose-derived stem cells via regulation of the ERK1/2 signaling pathway. Stem Cell Res. Ther. 8:282.
Alexander, K. A., Chang, M. K., Maylin, E. R., Kohler, T., Müller, R., Wu, A. C., et al. (2011). Osteal macrophages promote in vivo intramembranous bone healing in a mouse tibial injury model. J. Bone Miner. Res. 26, 1517–1532. doi: 10.1002/jbmr.354
Arcos, D., and Vallet-Regí, M. (2020). Substituted hydroxyapatite coatings of bone implants. J. Mater. Chem. B 8, 1781–1800. doi: 10.1039/c9tb02710f
Barradas, A. M. C., Fernandes, H. A. M., Groen, N., Chai, Y. C., Schrooten, J., Van De Peppel, J., et al. (2012). A calcium-induced signaling cascade leading to osteogenic differentiation of human bone marrow-derived mesenchymal stromal cells. Biomaterials 33, 3205–3215. doi: 10.1016/j.biomaterials.2012.01.020
Batoon, L., Millard, S. M., Wullschleger, M. E., Preda, C., Wu, A. C.-K., Kaur, S., et al. (2019). CD169+ macrophages are critical for osteoblast maintenance and promote intramembranous and endochondral ossification during bone repair. Biomaterials 196, 51–66. doi: 10.1016/j.biomaterials.2017.10.033
Bonnelye, E., Chabadel, A., Saltel, F., and Jurdic, P. (2008). Dual effect of strontium ranelate: stimulation of osteoblast differentiation and inhibition of osteoclast formation and resorption in vitro. Bone 42, 129–138. doi: 10.1016/j.bone.2007.08.043
Bose, S., Fielding, G., Tarafder, S., and Bandyopadhyay, A. (2013). Understanding of dopant-induced osteogenesis and angiogenesis in calcium phosphate ceramics. Trends Biotechnol. 31, 594–605. doi: 10.1016/j.tibtech.2013.06.005
Bose, S., and Tarafder, S. (2012). Calcium phosphate ceramic systems in growth factor and drug delivery for bone tissue engineering: a review. Acta Biomater. 8, 1401–1421. doi: 10.1016/j.actbio.2011.11.017
Braux, J., Velard, F., Guillaume, C., Jourdain, M.-L., Gangloff, S. C., Jallot, E., et al. (2016). Strontium-substituted bioceramics particles: a new way to modulate MCP-1 and Gro-α production by human primary osteoblastic cells. Materials 9:985. doi: 10.3390/ma9120985
Buache, E., Velard, F., Bauden, E., Guillaume, C., Jallot, E., Nedelec, J. M., et al. (2012). Effect of strontium-substituted biphasic calcium phosphate on inflammatory mediators production by human monocytes. Acta Biomater. 8, 3113–3119. doi: 10.1016/j.actbio.2012.04.045
Chen, Y. W., Feng, T., Shi, G. Q., Ding, Y. L., Yu, X. X., Zhang, X. H., et al. (2008). Interaction of endothelial cells with biodegradable strontium-doped calcium polyphosphate for bone tissue engineering. Appl. Surface Sci. 255, 331–335. doi: 10.1016/j.apsusc.2008.06.154
Chen, Z., Klein, T., Murray, R. Z., Crawford, R., Chang, J., Wu, C., et al. (2016). Osteoimmunomodulation for the development of advanced bone biomaterials. Mater. Today 19, 304–321. doi: 10.1016/j.mattod.2015.11.004
Drouet, C., Leriche, A., Hampshire, S., Kashani, M., Stamboulis, A., Iafisco, M., et al. (2017). “2 - types of ceramics: material class,” in Advances in Ceramic Biomaterials, eds P. Palmero, F. Cambier, and E. De Barra (Cambridge, MA: Woodhead Publishing), 21–82.
Dvorak, M. M., and Riccardi, D. (2004). Ca2+ as an extracellular signal in bone. Cell Calcium 35, 249–255. doi: 10.1016/j.ceca.2003.10.014
Dvorak, M. M., Siddiqua, A., Ward, D. T., Carter, D. H., Dallas, S. L., Nemeth, E. F., et al. (2004). Physiological changes in extracellular calcium concentration directly control osteoblast function in the absence of calciotropic hormones. Proc. Natl. Acad. Sci. U.S.A. 101, 5140–5145. doi: 10.1073/pnas.0306141101
Ginebra, M.-P., Espanol, M., Maazouz, Y., Bergez, V., and Pastorino, D. (2018). Bioceramics and bone healing. EFORT Open Rev. 3, 173–183. doi: 10.1302/2058-5241.3.170056
Gu, Z., Xie, H., Huang, C., Peng, H., Tan, H., Li, L., et al. (2014). Effects of strontium-doped calcium polyphosphate on angiogenic growth factors expression of co-culturing system in vitro and of host cell in vivo. RSC Adv. 4, 2783–2792. doi: 10.1039/c3ra44323j
Gu, Z., Zhang, X., Li, L., Wang, Q., Yu, X., and Feng, T. (2013). Acceleration of segmental bone regeneration in a rabbit model by strontium-doped calcium polyphosphate scaffold through stimulating VEGF and bFGF secretion from osteoblasts. Mater. Sci. Eng. C 33, 274–281. doi: 10.1016/j.msec.2012.08.040
Guo, X., Wei, S., Lu, M., Shao, Z., Lu, J., Xia, L., et al. (2016). Dose-dependent effects of strontium ranelate on ovariectomy rat bone marrow mesenchymal stem cells and human umbilical vein endothelial cells. Int. J. Biol. Sci. 12, 1511–1522. doi: 10.7150/ijbs.16499
Hench, L. L., and Polak, J. M. (2002). Third-generation biomedical materials. Science 295, 1014–1017. doi: 10.1126/science.1067404
Hench, L. L., and Wilson, J. (1984). Surface-active biomaterials. Science 226, 630–636. doi: 10.1126/science.6093253
Heughebaert, M., Legeros, R. Z., Gineste, M., Guilhem, A., and Bonel, G. (1988). Physicochemical characterization of deposits associated with HA ceramics implanted in nonosseous sites. J. Biomed. Mater. Res. 22, 257–268. doi: 10.1002/jbm.820221406
Huang, L., Zhang, J., Hu, J., Zhao, T., and Gu, Z. (2020). Biomimetic gelatin methacrylate/nano fish bone hybrid hydrogel for bone regeneration via osteoimmunomodulation. ACS Biomater. Sci. Eng. 6, 3270–3274. doi: 10.1021/acsbiomaterials.0c00443
International Programme on Chemical Inter-Organization Programme for the Sound Management (2010). Strontium and Strontium Compounds. Geneva: World Health Organization.
Jeong, J., Kim, J. H., Shim, J. H., Hwang, N. S., and Heo, C. Y. (2019). Bioactive calcium phosphate materials and applications in bone regeneration. Biomater. Res. 23:4.
Jiménez, M., Abradelo, C., San Román, J., and Rojo, L. (2019). Bibliographic review on the state of the art of strontium and zinc based regenerative therapies. Recent developments and clinical applications. J. Mater. Chem. B 7, 1974–1985. doi: 10.1039/c8tb02738b
Khoshniat, S., Bourgine, A., Julien, M., Weiss, P., Guicheux, J., and Beck, L. (2011). The emergence of phosphate as a specific signaling molecule in bone and other cell types in mammals. Cell. Mol. Life Sci. 68, 205–218. doi: 10.1007/s00018-010-0527-z
Krishnan, V., Bhatia, A., and Varma, H. (2016). Development, characterization and comparison of two strontium doped nano hydroxyapatite molecules for enamel repair/regeneration. Dental Mater. 32, 646–659. doi: 10.1016/j.dental.2016.02.002
Kruppke, B., Heinemann, C., Wagner, A.-S., Farack, J., Wenisch, S., Wiesmann, H.-P., et al. (2019a). Strontium ions promote in vitro human bone marrow stromal cell proliferation and differentiation in calcium-lacking media. Dev., Growth Diff. 61, 166–175. doi: 10.1111/dgd.12588
Kruppke, B., Wagner, A.-S., Rohnke, M., Heinemann, C., Kreschel, C., Gebert, A., et al. (2019b). Biomaterial based treatment of osteoclastic/osteoblastic cell imbalance – Gelatin-modified calcium/strontium phosphates. Mater. Sci. Eng. C 104:109933. doi: 10.1016/j.msec.2019.109933
Kuang, G.-M., Yau, W. P., Wu, J., Yeung, K. W. K., Pan, H., Lam, W. M., et al. (2015). Strontium exerts dual effects on calcium phosphate cement: accelerating the degradation and enhancing the osteoconductivity both in vitro and in vivo. J. Biomed. Mater. Res. A 103, 1613–1621. doi: 10.1002/jbm.a.35298
Lee, C.-H., Kim, Y.-J., Jang, J.-H., and Park, J.-W. (2016). Modulating macrophage polarization with divalent cations in nanostructured titanium implant surfaces. Nanotechnology 27:085101. doi: 10.1088/0957-4484/27/8/085101
Li, Y., Li, J., Zhu, S., Luo, E., Feng, G., Chen, Q., et al. (2012). Effects of strontium on proliferation and differentiation of rat bone marrow mesenchymal stem cells. Biochem. Biophys. Res. Commun. 418, 725–730. doi: 10.1016/j.bbrc.2012.01.088
Li, Y., Li, Q., Zhu, S., Luo, E., Li, J., Feng, G., et al. (2010). The effect of strontium-substituted hydroxyapatite coating on implant fixation in ovariectomized rats. Biomaterials 31, 9006–9014. doi: 10.1016/j.biomaterials.2010.07.112
Lin, K., Liu, P., Wei, L., Zou, Z., Zhang, W., Qian, Y., et al. (2013). Strontium substituted hydroxyapatite porous microspheres: surfactant-free hydrothermal synthesis, enhanced biological response and sustained drug release. Chem. Eng. J. 222, 49–59. doi: 10.1016/j.cej.2013.02.037
Liu, F., Zhang, X., Yu, X., Xu, Y., Feng, T., and Ren, D. (2011). In vitro study in stimulating the secretion of angiogenic growth factors of strontium-doped calcium polyphosphate for bone tissue engineering. J. Mater. Sci. Mater. Med. 22, 683–692. doi: 10.1007/s10856-011-4247-1
Loi, F., Córdova, L. A., Pajarinen, J., Lin, T.-H., Yao, Z., and Goodman, S. B. (2016). Inflammation, fracture and bone repair. Bone 86, 119–130. doi: 10.1016/j.bone.2016.02.020
Mao, Y., Pan, M., Yang, H., Lin, X., and Yang, L. (2020). Injectable hydrogel wound dressing based on strontium ion cross-linked starch. Front. Mater. Sci. 14, 232–241. doi: 10.1007/s11706-020-0508-6
Marques, C. F., Lemos, A., Vieira, S. I., Da Cruz, E., Silva, O. A. B., Bettencourt, A., et al. (2016). Antibiotic-loaded Sr-doped porous calcium phosphate granules as multifunctional bone grafts. Ceram. Int. 42, 2706–2716. doi: 10.1016/j.ceramint.2015.11.001
Marx, D., Rahimnejad Yazdi, A., Papini, M., and Towler, M. (2020). A review of the latest insights into the mechanism of action of strontium in bone. Bone Rep. 12:100273. doi: 10.1016/j.bonr.2020.100273
Meunier, P. J., Roux, C., Seeman, E., Ortolani, S., Badurski, J. E., Spector, T. D., et al. (2004). The effects of strontium ranelate on the risk of vertebral fracture in women with postmenopausal osteoporosis. New Engl. J. Med. 350, 459–468.
Neves, N., Linhares, D., Costa, G., Ribeiro, C. C., and Barbosa, M. A. (2017). In vivo and clinical application of strontium-enriched biomaterials for bone regeneration. Bone Joint Res. 6, 366–375. doi: 10.1302/2046-3758.66.bjr-2016-0311.r1
Peng, S., Zhou, G., Luk, K. D. K., Cheung, K. M. C., Li, Z., Lam, W. M., et al. (2009). Strontium promotes osteogenic differentiation of mesenchymal stem cells through the Ras/MAPK signaling pathway. Cell. Physiol. Biochem. 23, 165–174. doi: 10.1159/000204105
Penido, M. G. M. G., and Alon, U. S. (2012). Phosphate homeostasis and its role in bone health. Pediatr. Nephrol. 27, 2039–2048. doi: 10.1007/s00467-012-2175-z
Prabha, R. D., Nair, B. P., Ditzel, N., Kjems, J., Nair, P. D., and Kassem, M. (2019). Strontium functionalized scaffold for bone tissue engineering. Mater. Sci. Eng. C 94, 509–515. doi: 10.1016/j.msec.2018.09.054
Reginster, J., Kaufman, J., Goemaere, S., Devogelaer, J., Benhamou, C., Felsenberg, D., et al. (2012). Maintenance of antifracture efficacy over 10 years with strontium ranelate in postmenopausal osteoporosis. Osteoporosis Int. 23, 1115–1122. doi: 10.1007/s00198-011-1847-z
Renaudin, G., Laquerrière, P., Filinchuk, Y., Jallot, E., and Nedelec, J. M. (2008). Structural characterization of sol–gel derived Sr-substituted calcium phosphates with anti-osteoporotic and anti-inflammatory properties. J. Mater. Chem. 18, 3593–3600. doi: 10.1039/b804140g
Römer, P., Desaga, B., Proff, P., Faltermeier, A., and Reicheneder, C. (2012). Strontium promotes cell proliferation and suppresses IL-6 expression in human PDL cells. Ann. Anat. Anatomischer Anzeiger 194, 208–211. doi: 10.1016/j.aanat.2011.09.008
Saberianpour, S., Heidarzadeh, M., Geranmayeh, M. H., Hosseinkhani, H., Rahbarghazi, R., and Nouri, M. (2018). Tissue engineering strategies for the induction of angiogenesis using biomaterials. J. Biol. Eng. 12:36.
Saidak, Z., and Marie, P. J. (2012). Strontium signaling: molecular mechanisms and therapeutic implications in osteoporosis. Pharmacol. Ther. 136, 216–226. doi: 10.1016/j.pharmthera.2012.07.009
Schumacher, M., Lode, A., Helth, A., and Gelinsky, M. (2013). A novel strontium(II)-modified calcium phosphate bone cement stimulates human-bone-marrow-derived mesenchymal stem cell proliferation and osteogenic differentiation in vitro. Acta Biomater. 9, 9547–9557. doi: 10.1016/j.actbio.2013.07.027
Sila-Asna, M., Bunyaratvej, A., Maeda, S., Kitaguchi, H., and Bunyaratavej, N. (2007). Osteoblast differentiation and bone formation gene expression in strontium-inducing bone marrow mesenchymal stem cell. Kobe J. Med. Sci. 53, 25–35.
Smith, L. (1963). Ceramic-plastic material as a bone substitute. Arch. Surg. 87, 653–661. doi: 10.1001/archsurg.1963.01310160115023
Surdacka, A., Stopa, J., and Torlinski, L. (2007). In Situ effect of strontium toothpaste on artificially decalcified human enamel. Biol. Trace Elem. Res. 116, 147–153. doi: 10.1007/s12011-007-9024-0
Tao, Z., Zhou, W., Jiang, Y., Wu, X., Xu, Z., Yang, M., et al. (2018). Effects of strontium-modified calcium phosphate cement combined with bone morphogenetic protein-2 on osteoporotic bone defects healing in rats. J. Biomater. Appl. 33, 3–10. doi: 10.1177/0885328218765847
Tao, Z.-S., Bai, B.-L., He, X.-W., Liu, W., Li, H., Zhou, Q., et al. (2016a). A comparative study of strontium-substituted hydroxyapatite coating on implant’s osseointegration for osteopenic rats. Med. Biol. Eng. Comput. 54, 1959–1968. doi: 10.1007/s11517-016-1494-9
Tao, Z.-S., Zhou, W.-S., He, X.-W., Liu, W., Bai, B.-L., Zhou, Q., et al. (2016b). A comparative study of zinc, magnesium, strontium-incorporated hydroxyapatite-coated titanium implants for osseointegration of osteopenic rats. Mater. Sci. Eng. C 62, 226–232. doi: 10.1016/j.msec.2016.01.034
Thormann, U., Ray, S., Sommer, U., Elkhassawna, T., Rehling, T., Hundgeburth, M., et al. (2013). Bone formation induced by strontium modified calcium phosphate cement in critical-size metaphyseal fracture defects in ovariectomized rats. Biomaterials 34, 8589–8598. doi: 10.1016/j.biomaterials.2013.07.036
Vavken, J., Mameghani, A., Vavken, P., and Schaeren, S. (2016). Complications and cancer rates in spine fusion with recombinant human bone morphogenetic protein-2 (rhBMP-2). Eur. Spine J. 25, 3979–3989. doi: 10.1007/s00586-015-3870-9
Von Euw, S., Wang, Y., Laurent, G., Drouet, C., Babonneau, F., Nassif, N., et al. (2019). Bone mineral: new insights into its chemical composition. Sci. Rep. 9:8456.
Wang, R., Wang, R., Chen, D., Qin, G., and Zhang, E. (2020). Novel CoCrWNi alloys with Cu addition: microstructure, mechanical properties, corrosion properties and biocompatibility. J. Alloys Compounds 824:153924. doi: 10.1016/j.jallcom.2020.153924
Wang, W., and Yeung, K. W. K. (2017). Bone grafts and biomaterials substitutes for bone defect repair: a review. Bioactive Mater. 2, 224–247. doi: 10.1016/j.bioactmat.2017.05.007
Wang, X., Wang, Y., Li, L., Gu, Z., Xie, H., and Yu, X. (2014). Stimulations of strontium-doped calcium polyphosphate for bone tissue engineering to protein secretion and mRNA expression of the angiogenic growth factors from endothelial cells in vitro. Ceram. Int. 40, 6999–7005. doi: 10.1016/j.ceramint.2013.12.027
Wornham, D. P., Hajjawi, M. O., Orriss, I. R., and Arnett, T. R. (2014). Strontium potently inhibits mineralisation in bone-forming primary rat osteoblast cultures and reduces numbers of osteoclasts in mouse marrow cultures. Osteoporosis Int. 25, 2477–2484. doi: 10.1007/s00198-014-2791-5
Wu, Y., Hou, J., Yin, M., Wang, J., and Liu, C. (2014). Enhanced healing of rabbit segmental radius defects with surface-coated calcium phosphate cement/bone morphogenetic protein-2 scaffolds. Mater. Sci. Eng. C 44, 326–335. doi: 10.1016/j.msec.2014.08.020
Xie, H., Gu, Z., He, Y., Xu, J., Xu, C., Li, L., et al. (2018). Microenvironment construction of strontium–calcium-based biomaterials for bone tissue regeneration: the equilibrium effect of calcium to strontium. J. Mater. Chem. B 6, 2332–2339. doi: 10.1039/c8tb00306h
Xu, Y., An, L., Chen, L., Xu, H., Zeng, D., and Wang, G. (2018). Controlled hydrothermal synthesis of strontium-substituted hydroxyapatite nanorods and their application as a drug carrier for proteins. Adv. Powder Technol. 29, 1042–1048. doi: 10.1016/j.apt.2018.01.008
Yan, S., Feng, L., Zhu, Q., Yang, W., Lan, Y., Li, D., et al. (2018). Controlled release of BMP-2 from a heparin-conjugated strontium-substituted nanohydroxyapatite/silk fibroin scaffold for bone regeneration. ACS Biomat. Sci. Eng. 4, 3291–3303. doi: 10.1021/acsbiomaterials.8b00459
Ye, H., Zhu, J., Deng, D., Jin, S., Li, J., and Man, Y. (2019). Enhanced osteogenesis and angiogenesis by PCL/chitosan/Sr-doped calcium phosphate electrospun nanocomposite membrane for guided bone regeneration. J. Biomater. Sci. Polym. Ed. 30, 1505–1522. doi: 10.1080/09205063.2019.1646628
Zhao, F., Lei, B., Li, X., Mo, Y., Wang, R., Chen, D., et al. (2018). Promoting in vivo early angiogenesis with sub-micrometer strontium-contained bioactive microspheres through modulating macrophage phenotypes. Biomaterials 178, 36–47. doi: 10.1016/j.biomaterials.2018.06.004
Keywords: calcium phosphate ceramics, strontium substitution, microenvironment, bone regeneration, biomaterials
Citation: Wan B, Wang R, Sun Y, Cao J, Wang H, Guo J and Chen D (2020) Building Osteogenic Microenvironments With Strontium-Substituted Calcium Phosphate Ceramics. Front. Bioeng. Biotechnol. 8:591467. doi: 10.3389/fbioe.2020.591467
Received: 04 August 2020; Accepted: 16 September 2020;
Published: 07 October 2020.
Edited by:
Xin Zhao, Hong Kong Polytechnic University, Hong KongCopyright © 2020 Wan, Wang, Sun, Cao, Wang, Guo and Chen. This is an open-access article distributed under the terms of the Creative Commons Attribution License (CC BY). The use, distribution or reproduction in other forums is permitted, provided the original author(s) and the copyright owner(s) are credited and that the original publication in this journal is cited, in accordance with accepted academic practice. No use, distribution or reproduction is permitted which does not comply with these terms.
*Correspondence: Dafu Chen, Y2hlbmRhZnVqc3RAMTI2LmNvbQ==; Renxian Wang, d3J4cHVtY0AxMjYuY29t
Disclaimer: All claims expressed in this article are solely those of the authors and do not necessarily represent those of their affiliated organizations, or those of the publisher, the editors and the reviewers. Any product that may be evaluated in this article or claim that may be made by its manufacturer is not guaranteed or endorsed by the publisher.
Research integrity at Frontiers
Learn more about the work of our research integrity team to safeguard the quality of each article we publish.