- Institute of Chemical, Environmental and Bioscience Engineering, Research Area Biochemical Engineering, Technische Universität Wien, Vienna, Austria
The magnetization of non-magnetic cells has great potential to aid various processes in medicine, but also in bioprocess engineering. Current approaches to magnetize cells with magnetic nanoparticles (MNPs) require cellular uptake or adsorption through in vitro manipulation of cells. A relatively new field of research is “magnetogenetics” which focuses on in vivo production and accumulation of magnetic material. Natural intrinsically magnetic cells (IMCs) produce intracellular, MNPs, and are called magnetotactic bacteria (MTB). In recent years, researchers have unraveled function and structure of numerous proteins from MTB. Furthermore, protein engineering studies on such MTB proteins and other potentially magnetic proteins, like ferritins, highlight that in vivo magnetization of non-magnetic hosts is a thriving field of research. This review summarizes current knowledge on recombinant IMC generation and highlights future steps that can be taken to succeed in transforming non-magnetic cells to IMCs.
Introduction
Magnetic nanoparticles (MNPs) have been of importance since the successful use of magnetically labeled antibodies for cell separation (Whitesides et al., 1983; Kemsheadl and Ugelstad, 1985). Since then, magnet-assisted cell separation (MACS) has been frequently used for detection and isolation of cells from complex mixtures [e.g., (McCloskey et al., 2003; Kuhara et al., 2004; Zborowski and Chalmers, 2011)]. The use of MNPs is also of great interest as magnetic resonance imaging (MRI; Li et al., 2016) and magnetic particle imaging (MPI) contrast agent (Kraupner et al., 2017), for targeted drug delivery (Huang et al., 2016; Price et al., 2018), stem cell-based regenerative medicine (Van de Walle et al., 2020), targeted cell delivery (Wu L. et al., 2018), hyperthermia treatment (Kobayashi, 2011; Liu et al., 2020), and magneto-mechanical cell fate regulation (Wu C. et al., 2018). In these approaches, cells take up or adhere MNPs (Figure 1A). Recent research showed that internalized MNPs can undergo degradation (Van de Walle et al., 2019; Curcio et al., 2020). Interestingly, cells showed re-magnetization after MNP degradation, which demonstrated that some cells might contain quiescent abilities to magnetize in vivo. Furthermore, there exist prokaryotic organisms that naturally magnetize themselves through intracellular MNP formation (Blakemore, 1975). This existence of natural, cellular magnetization initiated research to uncover important prerequisites for cell magnetization. “Magnetogenetics” focuses on intracellular production and accumulation of magnetic material in non-magnetic cells in vivo (Meister, 2016; Nimpf and Keays, 2017; Figure 1B). Several reviews describe the accumulation (Li et al., 2011; Singh et al., 2016; Gorobets et al., 2017; Gahlawat and Choudhury, 2019) and current applications of MNPs (Plouffe et al., 2015; Li et al., 2016; Mohammed et al., 2017; Vargas et al., 2018; Wu et al., 2019; Van de Walle et al., 2020). However, no overview on the generation and application of whole magnetic cells is available. This review specifically discusses intrinsically magnetic cells (IMCs) and recombinant approaches to generate them.
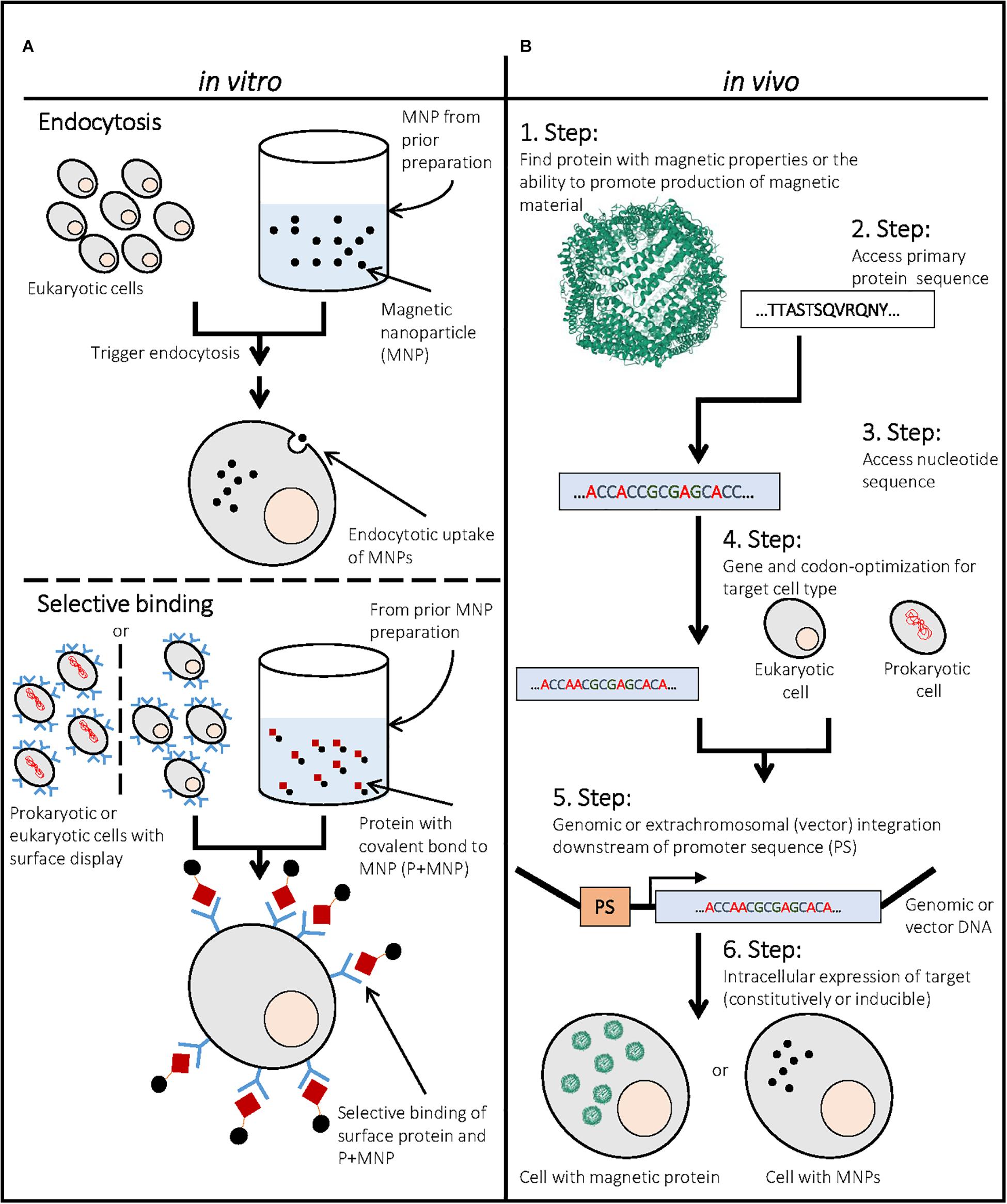
Figure 1. Generation of magnetic cells by in vitro and in vivo approaches. (A) Upper part: Example for the generation of magnetic cells by endocytosis of magnetic nanoparticles (MNPs) with eukaryotic cells. MNPs have to be prepared in a prior step to promote endocytosis. (A) Lower part: Example for the generation of magnetic cells through the selective binding of a protein with covalently bound MNP to another cell surface protein (e.g., surface display of antibody that binds MNP-bound antigen). This approach can be performed for eukaryotic and prokaryotic cells by expressing and targeting a respective protein to the cell surface. The MNPs have to be chemically modified to covalently bind a respective antigen, preferably through a covalent bond. (B) Example for the generation of intrinsically magnetic cells through expression of a respective protein in the cell. In this case, the iron oxide containing protein ferritin [PDB ID: 1FHA (Lawson et al., 1991)] is seen as an example. First, a respective protein that confers a magnetic moment or is able to generate magnetic precipitates in the cell has to be identified. Further its primary sequence and nucleotide sequence have to be assessed and optimized. Finally, the respective protein can be integrated in a vector or genomic DNA of the cell and expressed to yield intrinsically magnetic cells that contain high amounts of the respective magnetic protein or a magnetic precipitate.
Magnetism
Intrinsically magnetic cells are magnetic cells, therefore a brief overview on magnetism and important characteristics in the nano- and micrometer range is given. Due to availability, biocompatibility and magnetic properties, we focus on the element iron. There are different types of magnetism: depending on the magnetic moments that arise from electrons and their two spin states, a material can show ferromagnetism, antiferromagnetism and ferrimagnetism through unpaired electrons (unpaired spins; Figure 2A) or diamagnetism through paired electrons (paired opposite spins). Diamagnetic molecules, like water, are commonly classified as non-magnetic materials and are repelled by strong magnetic fields. In paramagnetic materials, randomly oriented spins are found (Figure 2A; O’Handley, 2000).
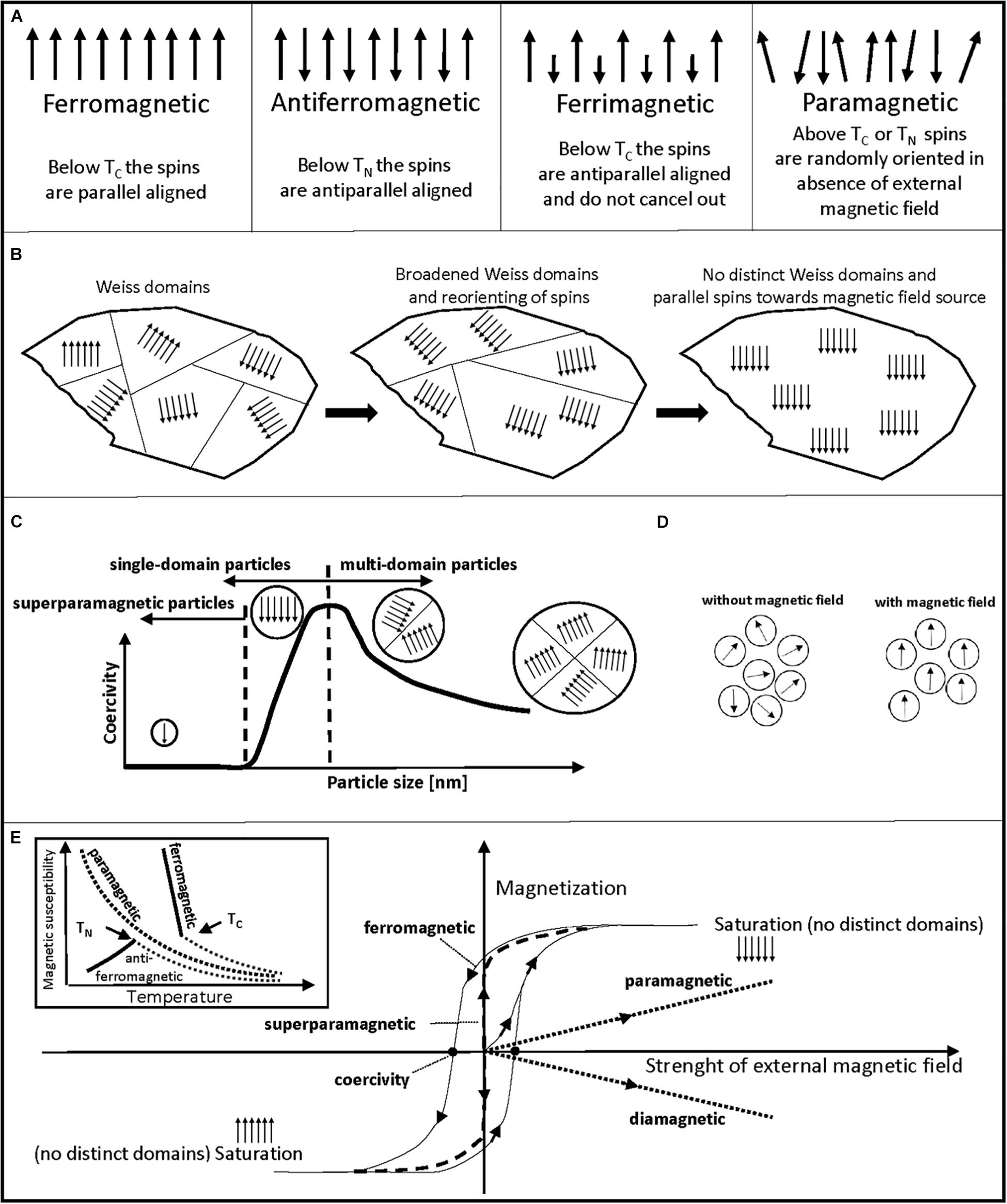
Figure 2. Schematic description of different magnetic properties. (A) Schematic orientation of magnetic moments (spins) of ferromagnetic, antiferromagnetic, ferrimagnetic, and paramagnetic materials are shown. (B) Broadening and dissipation of Weiss domains of a ferromagnetic material through an increasing, external magnetic field is shown from left to right. (C) Schematic graph of coercivity versus particle size. Superparamagnetic particles show no coercivity, coercivity increases for single-domain particles with increasing size until a maximum, when a switch to the energetically more favorable multi-domain state occurs. (D) Schematic sample of superparamagnetic particles is shown. Without an external magnetic field, particles show random orientation that leads to no net magnetization of the sample. With an external magnetic field, magnetic moments orient parallel toward the source of the magnetic field. (E) Schematic depiction of magnetization curves and their extractable information on magnetic properties of a sample. Temperature (inlet) and magnetic field strength dependency in SQUID magnetometry measurements is shown. Ferro- and ferrimagnetic materials usually show similar curves, only ferromagnetism is shown. Inlet: Magnetic susceptibility versus temperature graph is shown. Based on the changing magnetic susceptibility at rising temperature, one can differentiate antiferromagnetic, ferromagnetic, or paramagnetic properties of a sample. Magnetic susceptibility decreases exponentially with increasing temperature for paramagnetic samples. Until TN, antiferromagnetic samples show an increase in magnetic susceptibility with temperature. Near TC, ferro- and ferrimagnetic samples show a steep decrease in magnetic susceptibility with temperature. Then, above TN and TC paramagnetic behavior is visible for ferro-, ferri-, and antiferromagnetic samples. Main graph: Sample magnetization versus strength of external magnetic field. Non-magnetized samples start at zero magnetization without an external magnetic field. Measurement allows to find coercivity points as marked for hysteresis curve of ferro- and ferrimagnetic samples that arises from moving/dissipating Weiss domains with magnetic field strength. No coercivity and hysteresis is usually visible for superparamagnetic particles. A linear course of sample magnetization is common for paramagnetic and diamagnetic samples. Plateau of sample magnetization marks saturation magnetization of sample, where no distinct Weiss domains exist in multi-domain state particles and is used as a mean to compare magnetic potency of a sample.
Above a certain temperature, called Curie-temperature (TC) and Néel-temperature (TN), the magnetic behavior of materials can change to paramagnetism. Metallic iron is ferromagnetic at room temperature and shows equal and aligned magnetic moments below TC. In antiferromagnetic materials, like hematite (α-Fe2O3), the spins show opposing directions, but equal magnetic moments. In ferrimagnetic materials, like magnetite (Fe3O4), maghemite (γ-Fe2O3) or greigite (Fe3S4), unequal magnetic moments and opposing directions are present (Figure 2A; O’Handley, 2000). Greigite has a similar structure to magnetite, but with sulfur instead of oxygen ions (Roberts et al., 2011). Reported saturation magnetization values at room temperature are 218 Am2 kg–1 for ferromagnetic iron (Wohlfarth, 1986), 92–100 Am2 kg–1 for magnetite, 60–80 Am2 kg–1 for maghemite, 59 Am2 kg–1 for greigite and 0.3 Am2 kg–1 for hematite (Cornell and Schwertmann, 2003; Chang et al., 2008).
The magnetic behavior is not only affected by temperature, but also by particle size. Above a critical particle size, an energetically favorable division of ferro-, ferri-, and antiferromagnetic materials into microscopic domains occurs, called Weiss domains (Figure 2B). These domains show different spin orientations, which result in a low net magnetic field of a macroscopic sample (O’Handley, 2000). When an external magnetic field is applied, these Weiss domains broaden and the spins align, which leads to magnetic attraction and dissipation of the domains (Figure 2B). Additionally, below a certain particle size, the Weiss domains also dissipate to form one single domain (single-domain state). This size-dependency is also visible through coercivity (demagnetization point) measurements in sensitive magnetometry experiments. In the single-domain state not only Weiss domains dissipate, but the external magnetic field required for demagnetization also decreases (Figure 2C). The characteristic size for a certain magnetic material to form a single-domain state differs. For example, a switch to single-domain state was reported below 73 nm for synthetic magnetite crystals (Li et al., 2017). Additionally, some materials exhibit superparamagnetism below 20 nm and above a certain temperature (blocking temperature). Exemplary sizes for superparamagnetic particles are 10 nm for cube-like magnetite (Li et al., 2017), 15–18 nm for maghemite (Ali K. et al., 2015), 16 nm for hematite (Bødker et al., 2000), and 9–14 nm for greigite (Lyubutin et al., 2013). Superparamagnetic particles show a fixed magnetic moment that varies only in direction, have no coercivity and a sample of such particles will have an overall zero net magnetization without an external magnetic field, due to random orientation of particles (Figure 2D). Since superparamagnetic particles also show high initial magnetic susceptibility, only low fields are needed for magnetization, making these particles valuable in medical and biotechnological approaches (Huber, 2005).
Several techniques are currently employed to investigate magnetic materials (Nisticò et al., 2020): superconducting quantum interference device (SQUID) magnetometry (Jenks et al., 1997; Sawicki et al., 2011) and vibrating sample mode (VSM) magnetometry (Foner, 1959) to gain magnetization curves, X-ray diffraction (XRD) to identify the atomic and molecular structure of crystals (Warren, 1990; Mos et al., 2018), and Mössbauer spectroscopy to gain valence state of iron, type of coordination polyhedron occupied by iron atoms and identification of iron oxide phases (Gütlich and Schröder, 2012; Keune, 2012; Kamnev and Tugarova, 2017). SQUID magnetometry is often the method of choice to determine if a sample is ferro-, ferri-, antiferro-, para-, or diamagnetic through measurement at different temperatures and external magnetic field strengths (Jenks et al., 1997; Sawicki et al., 2011). A schematic depiction of SQUID graphs and their extractable magnetic information is given in Figure 2E. For example, variation in temperature allows to distinguish between ferro-/ ferri-, antiferro-, and paramagnetic samples (inlet; Figure 2E). A variation in external magnetic field strength allows one to distinguish between ferro-/ ferri-, para-, dia-, and superparamagnetic samples (Figure 2E). This magnetization to field strength analysis can be also used to determine coercivity points and the saturation magnetization of ferro- and ferrimagnetic samples (Sorensen, 2001).
General Considerations for in vivo Magnetization of Cells
We define an IMC as a cell that (i) exhibits a measurable magnetic moment, (ii) changes its flow direction in a magnetic field, and (iii) has not acquired these properties through uptake of – or surface modification with – MNPs. Living cells mainly consist of water, thus they are usually classified as diamagnetic. However, diamagnetism can be overcome (O’Handley, 2000). One might ask (1) the type and (2) the amount of magnetic material to overcome diamagnetism.
In respect of type, iron is the element of choice, due to its availability, biocompatibility, and magnetic properties. Furthermore, prokaryotic and eukaryotic cells have integrated iron homeostasis (Crichton et al., 2002; Konhauser et al., 2011). In a cell, iron is never present in its metallic form, but in its cationic forms [ferrous (Fe2+), ferric (Fe3+)] with critical functions in cellular processes. Under physiological conditions only 10–18 M soluble Fe3+, but 10–1 M soluble Fe2+ can be accumulated (Bou-Abdallah, 2010). Unpaired electrons are needed for magnetic attraction and a brief look on the electron configuration of Fe2+ ([Ar]3d6) with 4 unpaired electrons and Fe3+ ([Ar]3d5) with 5 unpaired electrons might indicate that generation of IMCs is already feasible by intracellular accumulation of soluble iron cations. However, most intracellular iron is complexed by proteins, since accumulation of soluble iron is cytotoxic (Imlay, 2008; Yamada et al., 2012). Since iron cofactor proteins present limited options for cell magnetization, MNPs are a better strategy to magnetize cells – through uptake [e.g., (Van de Walle et al., 2020)] or adsorption [e.g., (McCloskey et al., 2003)]. However, IMCs need to produce MNPs in vivo through precipitation and crystallization of magnetic iron oxides (e.g., magnetite) or sulfides (e.g., greigite). Due to its strong magnetization, magnetite has been the material of choice for that purpose.
In respect to the required amount of magnetic material to overcome diamagnetism, it is difficult to state generally valid minimum quantities. The magnetic force and three additional forces are directly proportional to the radius of the respective cell (McCloskey et al., 2003; Plouffe et al., 2015; Zahn et al., 2017). Opposite to the direction of the magnetic force, a viscous drag force slows the cell down. Additionally, the gravitational force and the buoyancy force act on the cell. Calculations for MNP uptake (Plouffe et al., 2015) and adsorption (McCloskey et al., 2003) showed that increasing cell size requires more magnetic material to gain comparable magnetic properties. Furthermore, concerning the size of the intracellular magnetic material the characteristics of superparamagnetic and single-domain state magnetic behavior have to be considered. Recent calculations showed that MNP arrangements influence the magnetic state (Muxworthy and Williams, 2009). The authors showed that chain-like arrangement increases the range of the single-domain state to 12–197 nm for touching cubic-like magnetite particles.
Many molecules and ions that are of utmost importance for the cellular machinery, have small molecular magnetic moments, due to their nuclear spins. Despite the small value of these magnetic moments, a high gradient magnetic field might act on them (Zablotskii et al., 2016a). Thus, magnetic forces might compete and interfere with electrical forces (Zablotskii et al., 2016a). Several studies were performed on a variety of cells to analyze the impact of magnetism on cells. For example, it was long hoped to use high magnetic fields to reduce microbial growth for sanitization. However, neither static nor pulsed magnetic fields showed a significant impact on microbial growth (Harte et al., 2001), viability (László and Kutasi, 2010), or endospore germination (Wu et al., 2017). It is still noteworthy that transposition and heat shock protein activity was induced in Escherichia coli cells when exposed to magnetic fields (Chow and Tung, 2000; Potenza et al., 2004; Del Re et al., 2006). Low-level static magnetic fields significantly increased superoxide dismutase and peroxidase activity in suspension-cultured tobacco plant cells (Abdolmaleki et al., 2007; Sahebjamei et al., 2007). A variety of effects were found for mammalian cells. An in vitro study on human lymphocytes and macrophages showed morphological changes in an inhomogeneous static magnetic field (Vergallo et al., 2014). In an in vivo study with human endothelial cells, proliferation was inhibited and angiogenesis impaired by magnetic field gradients around 2 T m–1 (Wang et al., 2009). However, a static homogenous magnetic field (370 mT) showed no impact on human lung fibroblast cells (Romeo et al., 2016). Recent reviews (Zablotskii et al., 2016a,b) highlight that cellular parameters are rather dependent on the value of the magnetic field gradient, but not on the strength of the magnetic field.
Currently Known Natural IMCs
Magnetotactic Bacteria
Magnetotactic bacteria (MTB) form intracellular liposomes, called magnetosomes, filled with crystalline MNPs [mostly magnetite (Blakemore, 1975) or greigite (Mann et al., 1990; Lefèvre et al., 2011)]. MTB also show a chain-like arrangement of the magnetosomes, often positioned mid-cell (Figures 3A,B; Matsunaga et al., 1991; Williams et al., 2012; Bazylinski et al., 2013; Silva et al., 2013), which helps them to passively align their swimming to magnetic field lines (i.e., magnetotaxis; Blakemore, 1975; Bazylinski and Frankel, 2004; Müller et al., 2020). Magnetosomes make MTB susceptible to magnetic fields as low as the earth’s magnetic field (∼0.5 Gauss = 0.05 mT; Blakemore, 1975). It has been also shown that single MTB cells have magnetic moments around 2 × 10–16 A m2 in magnetic fields below 23 mT (Reufer et al., 2014; Zahn et al., 2017) and can be efficiently sorted and enriched with high-throughput microfluidic methods (Tay et al., 2018). Most magnetosome crystal sizes are between 35 and 120 nm (Bazylinski and Frankel, 2004; Araujo et al., 2015). This results in single-domain states with measureable coercivity rather than superparamagnetism. Furthermore, the linear arrangement and distance of magnetosomes increases the possible range of single-domain particles (Muxworthy and Williams, 2009) and increases cellular motility within external magnetic fields (Pfeiffer and Schüler, 2020).
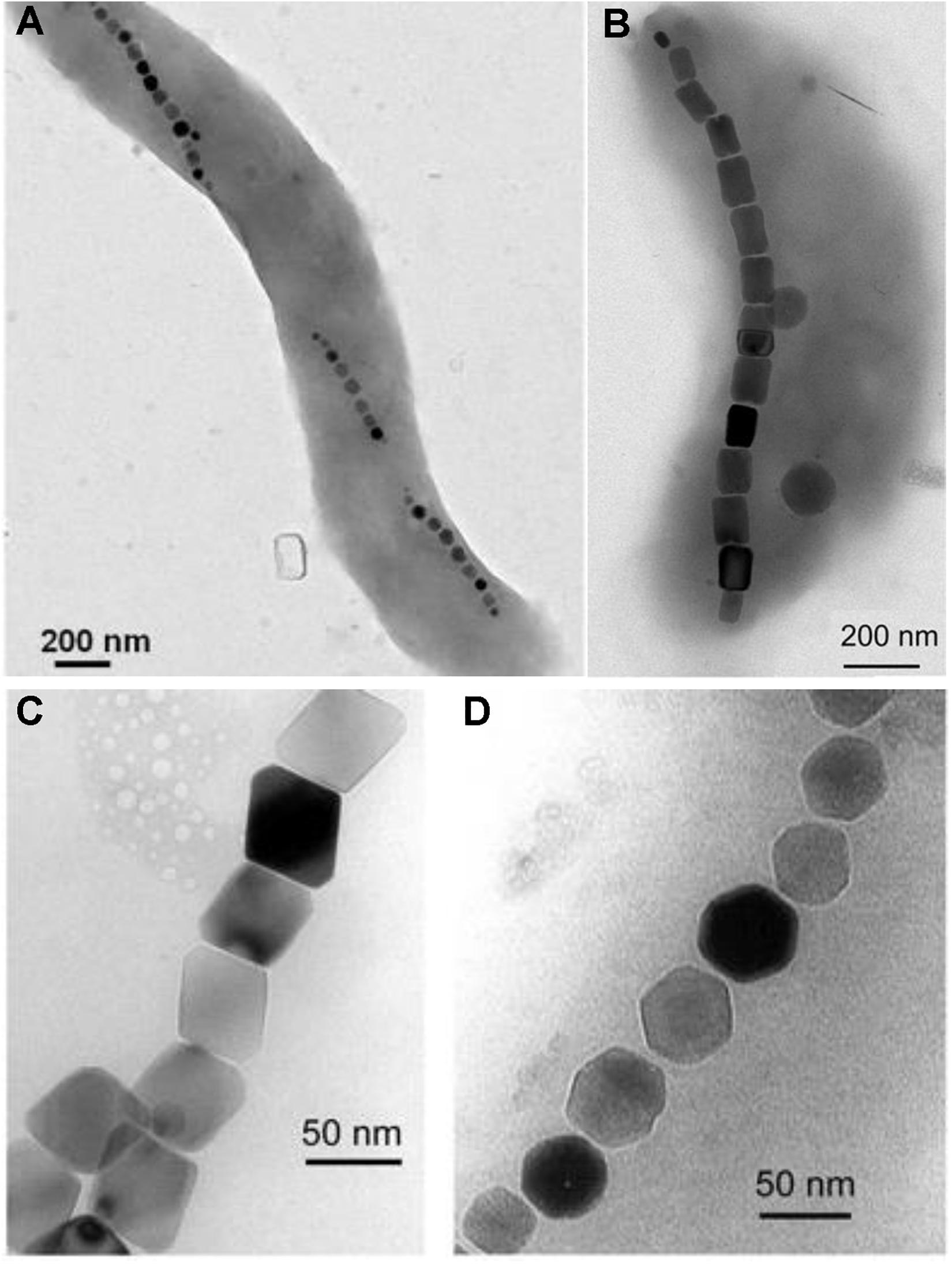
Figure 3. Exemplary transmission electron microscope images of magnetotactic bacteria with magnetosome chains and different magnetosome shapes. (A) Example of a magnetotactic Magnetospirillum species with chain-like magnetosome arrangement. (B) Example of a magnetotactic Magntovibrio species with chain-like magnetosome arrangement. (C) Magnetite magnetosomes with octahedral and (D) cuboctahedral morphologies. Image in part (A) was adapted from Alphandéry (2014). Images in part (B–D) were adapted from Pósfai et al. (2013).
Most MTB are found in micro-oxic and anoxic environments with an abundance of soluble iron (6–60 μM Fe2+; Flies et al., 2005) and show species-specific shape and size properties of MNPs (Lefèvre et al., 2013; Araujo et al., 2015; Uebe and Schüler, 2016; Figures 3C,D). Frequently described species of MTB are Magnetospirillum (Blakemore et al., 1979; Matsunaga et al., 1991), Magnetovibrio (Silva et al., 2013), Magnetospira (Zhu et al., 2010; Williams et al., 2012), Magnetofaba (Morillo et al., 2014), and Magnetococcus (Lefèvre et al., 2009; Bazylinski et al., 2013). Magnetospirillum gryphiswaldense and Magnetospirillum magneticum AMB-1 are frequently used to study magnetosome formation and magnetotaxis (Müller et al., 2020).
Magnetotactic bacteria accumulate magnetite up to 2–4% of their dry cell weight (DCW; Blakemore, 1982). It is thought that crystal nucleation proceeds through co-precipitation of soluble Fe2+ and Fe3+ with subsequent transformation to magnetite (Faivre and Godec, 2015). The thought precursor mineral is ferrihydrite (Faivre and Godec, 2015) that naturally forms nanocrystals below 10 nm (Michel et al., 2007, 2010a), can transform to ordered ferrimagnetic ferrihydrite (Michel et al., 2010a) and is found in the ubiquitous iron-storage protein ferritin (Chasteen and Harrison, 1999; Lewin et al., 2005; Michel et al., 2010b). MTB have a reservoir of special proteins that are located in or close to the magnetosome membrane with up to 120 copies per magnetosome particle (Raschdorf et al., 2018). These proteins are necessary for iron transport (Nies, 2011; Uebe and Schüler, 2016; Uebe et al., 2018; Keren-Khadmy et al., 2020), crystal growth and shape (Arakaki et al., 2010, 2014, 2016; Lopez-Moreno et al., 2017; Nudelman et al., 2018), and intracellular magnetosome arrangement (Scheffel et al., 2006; Abreu et al., 2014; Toro-Nahuelpan et al., 2019). Additionally, some exhibit peroxidase-like activity (Guo et al., 2012; Li et al., 2015), which help to reduce reactive oxygen species from Fenton reactions (Hongping et al., 2015), or prevent accumulation of free radicals (Amor et al., 2020). MTB have magnetosome gene clusters, often with mam (magnetosome membrane) and mms (magnetic-particle-membrane specific) genes that regulate magnetosome formation (Schübbe et al., 2003; Fukuda et al., 2006). In magnetotactic alphaproteobacteria, magnetosome-related genes are often abbreviated with mam or mms, in other recently characterized MTB of deltaproteobacteria, nitrospirae and omnitrophica, magnetosome-related genes can be denoted as mad or man genes (Barber-Zucker and Zarivach, 2017). The magnetosome genes are organized in operons and cluster in a single region, called magnetosome island (Uebe and Schüler, 2016; Barber-Zucker and Zarivach, 2017; McCausland and Komeili, 2020).
In general, magnetosome production requires large amounts of adenosine triphosphate (Yang et al., 2013) and leads to a strong increase of intracellular NADH/NAD+ ratio (∼15 after 40 h; Yang et al., 2013). MNP formation is a sensitive process that requires numerous protein-protein interactions and specific environmental conditions. MTB cultivation requires precise media and culture conditions (Ali et al., 2017). A major milestone was the first detailed analysis of magnetosome production in the controlled, microaerobic environment of a bioreactor (Heyen and Schüler, 2003). A maximum magnetite yield of 6.3 mg L–1 day–1 was found during batch cultivation of M. gryphiswaldense with a medium supplemented with 40 μM of iron. However, a cell density of only 0.40 g DCW L–1 was achieved. Later, researchers were able to produce the current maximum of 9.16 g DCW L–1 and 178.26 mg magnetite L–1 day–1 with M. gryphiswaldense (Zhang et al., 2011). It is also noteworthy to mention that defined growth media were recently developed that are devoid of uncharacterized and toxic products and yield magnetosome cores with higher iron content (99.8%) compared to common growth media [93.8% (Zhang et al., 2011)] for MTB (Berny et al., 2020).
Magnetic Non-magnetotactic Cells
Erythrocytes
Erythrocytes (red blood cells) are crucial for delivery of O2 in the body. They achieve this through their high intracellular hemoglobin content [≤96% of DCW (Weed et al., 1963) or 5.5 mM (Spees et al., 2001)]. Hemoglobin is made of four monomers, each with a covalently bound, Fe2+-containing prosthetic heme group (Khoshouei et al., 2017). Three hemoglobin forms are characterized by the state of the iron cation: Deoxyhemoglobin has no bound O2 and is paramagnetic due to unpaired electrons of Fe2+ (Kurokawa et al., 2018), Oxyhemoglobin has a diamagnetic character due to its bond of Fe2+ and O2 (Kurokawa et al., 2018), and Methemoglobin contains oxidized Fe3+, has paramagnetic behavior and is not able to bind O2. Erythrocytes rich in Deoxyhemoglobin or Methemoglobin can be characterized as natural IMCs, because they can be separated from a complex matrix through high magnetic fields (Melville et al., 1975). In fact, erythrocytes are usually separated by intense magnetic field gradients (Toner and Irimia, 2005). It is also worth mentioning that malaria-infected patients show an astonishingly high content in magnetic erythrocytes, which is also used to diagnose the infection with the malaria parasite Plasmodium falciparum [e.g., (Zimmerman et al., 2006)]. In its blood stage, P. falciparum resides in erythrocytes and digests up to 80% of the present hemoglobin. During the degradation, heme is released into the digestive vacuole, Fe2+ is oxidized and after further modifications, cyclic ferriprotoporphyrin IX [Fe(III)PPIX] dimers are produced. The dimers are linked to each other to form a brown, insoluble, crystalline and paramagnetic malaria pigment, called hemozoin (Egan, 2008).
Proteins and Approaches for the Generation of IMCs
Iron Importers
Uncontrolled import of iron without a specific intracellular recipient will likely result in disturbances in iron homeostasis (Crichton et al., 2002; Konhauser et al., 2011) and an increase in damaging Fenton reactions (Imlay, 2008; Yamada et al., 2012). However, we want to mention a recent study that showed successful magnetization of algae through overexpression of iron importers (Buck et al., 2015). Although separation of magnetic algae was demonstrated, the researchers highlighted this approach as economically unfeasible, due to low magnetization of algae.
MTB Proteins
In a hallmark study, researchers generated a recombinant IMC through genomic engineering of the non-magnetic, photosynthetic alphaproteobacterium Rhodospirillum rubrum with genes from the magnetotactic alphaproteobacterium M. gryphiswaldense (Kolinko et al., 2014). Their final strain harbored 29 relevant magnetosome genes of M. gryphiswaldense and was attracted to permanent magnets. The respective intracellular magnetic particles were crystalline magnetite with an average diameter of 24 nm and strongly resembled the magnetosomes of the donor strain. Based on this work, researchers recently aimed to (re)magnetize three non-magnetic Magnetospirillum species through a single-step transfer of a vector with > 30 major magnetosome genes from M. gryphiswaldense (Dziuba et al., 2020). However, only one strain genomically integrated the vector and produced small, unaligned magnetosomes that hindered efficient magnetotaxis under conditions similar to the geomagnetic field. It is evident that genomic integration of 29 genes and more requires tedious work and single MTB specific vectors have limited applicability for other hosts. In this respect, researchers have identified a possible pool of magnetite interacting MTB proteins. The proteins Mms6 of the mms6 operon, MamD (Mms7), MamC (Mms13), and MamG (Mms5) of the mamGFDC operon strongly bind to magnetite crystals through a charged, hydrophilic C-terminal region, rich in amino acids Glu, Asp, Tyr, Ser, and Thr (Arakaki et al., 2010). Furthermore, this C-terminal region can initiate crystal nucleation and shape of magnetite crystals (Arakaki et al., 2003; Ubago-Rodríguez et al., 2019). The proteins MamG and MamC contain a loop, rich in charged residues that interact with magnetite crystals, which was also found in MmsF and its homologue MamF of the mamGFDC operon (Nudelman and Zarivach, 2014; Rawlings et al., 2014; Valverde-Tercedor et al., 2015). Additionally, all contain a hydrophobic glycine-leucine repeat motif, which is common for self-assembling proteins, like silk fibroin (Zhou et al., 2001; Nudelman and Zarivach, 2014).
MamD
MamD is a 30.2 kDa magnetosome membrane protein that controls crystal size (Scheffel et al., 2008) and has its Leu-Gly repeat containing N-terminus inside the magnetosome lumen (Arakaki et al., 2003; Nudelman and Zarivach, 2014). However, studies on MamD are scarce and its highly hydrophobic character makes it a hard-to-express protein (Nudelman and Zarivach, 2014).
MamG
Similarly to MamD, studies on MamG are scarce and the 8 kDa MamG protein mostly contains hydrophobic transmembrane helices and charged amino acids residues at its ends (Lang and Schüler, 2008; Nudelman and Zarivach, 2014), which makes it also hard to express.
MamF/MmsF
The 12.3 kDa MamF protein has a 61% identity with the MmsF protein (Lohße et al., 2011). Both have an integral membrane character with three predicted hydrophobic helices, with the first connecting loop in the magnetosome lumen that contains charged residues for magnetite interaction (Nudelman and Zarivach, 2014). Surprisingly, it was possible to produce both proteins recombinantly in E. coli (Rawlings et al., 2014). Even more interesting, the authors found that almost all protein was found in the soluble fraction with an apparent 2-fold mass of 26 kDa under denaturing conditions. They hypothesized extremely stable dimers to be the reason. Cultivation of transfected mesenchymal stem cells that expressed the codon-optimized mmsF gene in 35 mM ferric quinate supplemented medium successfully yielded cells with intracellular MNPs (Elfick et al., 2017).
MamC
The MamC protein is found in magnetosome membranes and regulates the size of magnetite crystals (Scheffel et al., 2008; Valverde-Tercedor et al., 2015; Peigneux et al., 2016). Due to the hydrophobic character, whole MamC has only been produced as inclusion bodies in E. coli (Valverde-Tercedor et al., 2015; Lopez-Moreno et al., 2017). Although the 12.4 kDa protein has two transmembrane helices with a helical connecting loop, rich in charged residues (Nudelman and Zarivach, 2014; Nudelman et al., 2016), it could be a proper candidate for in vivo IMC generation. The two acidic residues (Glu66 and Asp70) of the loop match the distance between iron cations in magnetite (Nudelman et al., 2016) and in vitro studies on MamC and protein constructs that contained the helical MamC loop resulted in better magnetite size control (Nudelman et al., 2016; Ubago-Rodríguez et al., 2019). This highlighted that only parts of MTB proteins can be used to induce magnetite formation through fusion proteins.
Mms6
Mms6 represents an astonishing and heavily studied magnetosome-associated protein (Staniland and Rawlings, 2016). In M. magneticum AMB-1, the native Mms6 protein occurs in two distinct sizes 14.5 kDa and 6 kDa (Nguyen et al., 2016). The smaller 6 kDa version is tightly associated to magnetite crystals in magnetosomes and contains one transmembrane helix between N- and C-terminus (Arakaki et al., 2003; Grünberg et al., 2004; Nudelman and Zarivach, 2014). In addition to magnetite binding, the 14.5 kDa Mms6 version was found to be a binding partner of the important MamA (Nguyen et al., 2016). MamA surrounds magnetosomes and facilitates important protein-protein interactions (Komeili et al., 2004; Yamamoto et al., 2010; Zeytuni et al., 2011). Mms6 is also able to form micelles in vitro (200–400 kDa) with a diameter of approximately 10 nm in aqueous solution and the C-terminal, hydrophilic regions exposed (Wang et al., 2012). Astonishingly, addition of iron caused the micelles to form higher order structures (Zhang et al., 2015). Purified Mms6 improved MNP homogeneity during in vitro magnetite precipitation (Arakaki et al., 2003; Amemiya et al., 2007; Galloway et al., 2011; Staniland and Rawlings, 2016). Furthermore, experiments with Mms6 and MamC showed that magnetite formation was increased compared to the single protein approaches (Peigneux et al., 2019). Nevertheless, recombinant production of Mms6 in E. coli results in inclusion body formation (Amemiya et al., 2007; Prozorov et al., 2007; Bird et al., 2015). However, expression of codon-optimized mms6 is possible in eukaryotic cells (Zhang et al., 2014; Elfick et al., 2017). Cultivation of mms6 transfected mouse gliosarcoma 9L cells (Zhang et al., 2014) in 200 μM ferric citrate supplemented medium resulted in formation of intracellular, dark particles as seen by transmission electron microscopy (TEM) images (Zhang et al., 2014). The authors stated an increased iron content of transfected cells together with an increased MRI contrast, but without a decrease in viability. Furthermore, after inoculation of cells into mice, the resulting tumors showed an increased MRI contrast. However, further magnetic studies or iron oxide identification analyses were not performed. A more detailed analysis of mms6 transfected human mesenchymal stem cells also revealed dark aggregates between 10 and 500 nm when cultivated in 34 mM ferric quinate supplemented medium (Elfick et al., 2017; Figures 4A,B). SQUID magnetometry measurements of whole cells highlighted a superparamagnetic character with a saturation magnetization of 23.5 Am2 kg–1 at 310.15 K (37°C). Based on their measurements, the authors hypothesized the particles to be superparamagnetic magnetite with an average diameter of 12 nm after 2 weeks and approximately 6 × 106 particles per gram biomass after 3 weeks. Thus, Mms6 seems to be a proper candidate for eukaryotic IMC generation. Interestingly, a recent review mentioned unpublished data of this study on the co-transfection of codon-optimized mms6 and mmsF that resulted in less magnetism compared to mms6 transfection alone (Kerans et al., 2018).
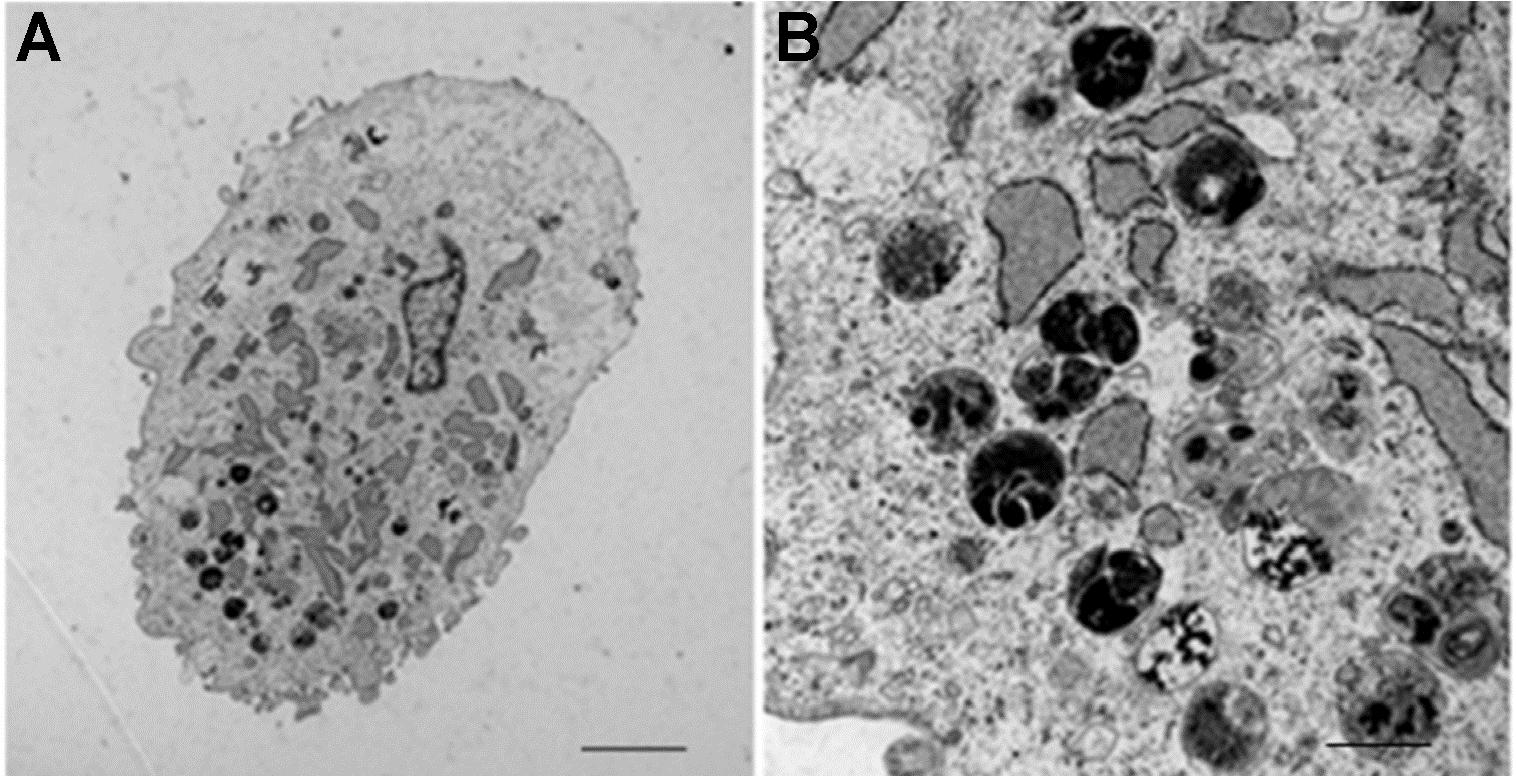
Figure 4. Transmission electron microscope images of mms6-transfected mesenchymal stem cells. Pictures were taken after 15 days of cultivation in ferric quinate supplemented medium and show accumulation of membrane-bound, intracytoplasmic electron-dense nanoparticles. (A) scale bar = 10 μm. (B) scale bar = 100 nm. Images in part (A,B) are adapted from Kerans et al. (2018).
MagA
The magA gene encodes a putative 46.8 kDa transmembrane iron transporter (Nakamura et al., 1995). Studies with recombinant MagA have focused on increasing MRI contrast of mammalian cells (Zurkiya et al., 2008; Goldhawk et al., 2009; Cho et al., 2014; Rohani et al., 2014; Sengupta et al., 2014). All studies stated that iron supplementation was crucial to achieve MRI contrast and all but one (Cho et al., 2014) reported no adverse effects on cell proliferation and/or cytotoxicity. Interestingly, it was reported that MagA is definitely not involved in magnetosome formation in M. magneticum AMB-1 and M. gryphiswaldense (Uebe et al., 2012). However, a detailed study with human embryonic kidney (HEK) 293FT-derived clonal cells, that expressed the magA gene in 200 μM ferric citrate supplemented medium, described 3–5 nm intracellular particles (Zurkiya et al., 2008). The particles were visualized by TEM and a further extraction and analysis by XRD highlighted similarities to magnetite. An increase in MRI contrast was stated, but faded back to normal values, when induction of the magA expression was stopped. The authors hypothesized that the particles were diluted through cell division. However, formation of magnetite through MagA was questioned (Uebe et al., 2012). Magnetite formation could result randomly from high intracellular iron concentrations, due to the ability of MagA to transport iron (Uebe et al., 2012). Recent studies also questioned the general use of MagA to increase MRI contrast. One study reported strong toxic effects of MagA production in murine mesenchymal/stromal cells and kidney-derived stem cells (Pereira et al., 2016). This study also showed that magA transfected HEK293TN cells showed comparable accumulation of 5 nm particles, increased intracellular iron concentration and MRI contrast when cultivated in 200 μM ferric citrate supplemented medium. Another study reported an increased intracellular iron content, but no increased MRI contrast of magA transfected undifferentiated embryonic mouse teratocarcinoma, multipotent P19 cells when cultured in 250 μM ferric nitrate supplemented medium (Liu et al., 2019). Summarizing, MagA seems to be usable to increase MRI contrast in some mammalian cells, but definite generation of IMCs has not been achieved yet.
Magnetite Interacting Scaffolds
All of the mentioned MTB proteins (MagA, MamC, MamD, MamF, MmsF, MamG, and Mms6) contain transmembrane regions that limit their usability and overall expression levels. As recently reviewed (Rawlings, 2016), the problems that come with transmembrane regions could be bypassed through in-silico methods and protein fusions. This was recently shown for MTB peptides in Adhirons (Rawlings et al., 2015), which represent non-antibody scaffold proteins with high thermal stability (melting temperature ∼101°C) and high soluble expression in E. coli (Tiede et al., 2014). The authors used phage display to display Adhirons with two variable binding loops and screened their interaction with cubic magnetite nanoparticles (Rawlings et al., 2015). Through several selection rounds, they identified lysine and histidine residues as key amino acids for magnetite interaction. Furthermore, they showed that only one loop with the sequence QKFVPKSTN was crucial for magnetite interaction and modestly improved magnetite particle appearance in magnetite precipitation reactions. Another study reported the generation of a new non-antibody scaffold protein that resembled an antiparallel, coiled-coil hairpin (Rawlings et al., 2019). The authors further identified magnetite interacting regions of the MTB proteins MamC and MmsF and engineered the scaffold protein to display these regions. Unfortunately, the protein was produced as inclusion body in E. coli and needed on-column refolding. In in vitro magnetite precipitation experiments, the MmsF construct, but not the MamC construct, produced magnetite particles in size and shape comparable to the native MmsF protein. More studies on such magnetite interacting scaffolds are definitely needed.
Hemoglobin and Myoglobin
The paramagnetic forms of hemoglobin, namely deoxyhemoglobin, methemoglobin and the crystalline hemozoin, are naturally occurring in erythrocytes, although hemozoin only during malaria infection (Egan, 2008). The paramagnetic behavior can be explained by unpaired electrons of the respective iron cations. When occurring in high amounts, hemoglobins can render the whole cell magnetic (Melville et al., 1975; Egan, 2008). Similarly, myoglobin can become paramagnetic metmyoglobin under oxidizing conditions (Sofla et al., 2013). Myoglobin and each of the four hemoglobin subunits contain a porphyrin ring with an iron at its center (Khoshouei et al., 2017). The rather small size and simple folding pattern of myoglobin and the single hemoglobin subunits make them suitable for recombinant expression. Cytosolic expression of active myoglobin from sperm whale to approximately 10% of total protein was successful in E. coli (Springer and Sligar, 1987). Cytosolic production of recombinant hemoglobin above 5% and 7% of total protein content in E. coli and Saccharomyces cerevisiae, respectively, was also reported (Hoffman et al., 1990; Martínez et al., 2015). However, given the high natural hemoglobin content of erythrocytes, even higher expression levels are needed. Astonishingly, a total intracellular, soluble protein yield of 65% was reported for recombinant overexpression of leghemoglobin (LegH) from soy bean in a proprietary methylotrophic Komagataella phaffii MXY0291 strain (Fraser et al., 2018). LegH shows structural similarities to myoglobin (Singh and Varma, 2017). Aside from the legH gene, the respective strain also overexpressed all 8 genes of the heme biosynthesis pathway and the transcriptional activator Mxr1 (Fraser et al., 2018) that has been shown to be crucial for the yeast’s methanol pathway and peroxisomal enzymes (Lin-Cereghino et al., 2006). Unfortunately, data on the strain’s physiology, cultivation and magnetic behavior is not available. However, this proofs that active folding and high titers of heme-containing globins are not limited to mammalian cells as long as the necessary genetic prerequisites are given. Nevertheless, high intracellular heme-protein content for cell magnetization might be rather limited to erythrocytes. Furthermore, based on magnetic properties, heme proteins will never result in cell magnetization comparable to in vivo magnetite production. However, it must be mentioned that extracellular triggers can be used to increase magnetic susceptibility of heme-protein-rich cells, as shown for myoglobin-rich cardiomyocytes (Sofla et al., 2013). Naturally, the cytoplasmic protein fraction of these cells consists only of 5–10% of the heme-protein myoglobin (Berridge et al., 2013). The use of the highly oxidizing agent NaNO2 transformed all myoglobin to paramagnetic metmyoglobin to generate magnetic cardiomyocytes (Sofla et al., 2013).
Ferritin
Ferritins are a family of highly conserved protein nanostructures that hold and sequester iron atoms. Theoretically, ferritins store up to 4,500 iron atoms as iron oxides in their inner cavity, but typically an average of 2,000 iron atoms are present (Jutz et al., 2015). All ferritins have an α-helix structure with strong helix-helix interactions that make the proteins very stable (Theil, 1987; Martsev et al., 1998). Ferritins usually consist of 24 subunits and have a size around 450 kDa in the apo-ferritin state (Theil, 1987). Ferritin cavities can have diameters between 5 and 8 nm (Theil, 2012). Mammalian and plant ferritins consist of a certain ratio of H- and L-subunits and are soluble in the cytoplasm and plastids. The H-subunit has an iron center, where fast conversion of Fe2+ to Fe3+ by O2 or hydrogen peroxide occurs (Lawson et al., 1989). The L-subunit contributes to the nucleation of the iron core and protein stability (Santambrogio et al., 1992). Bacteria have two types of ferritins, the archetypal ferritins with 24 identical H-type subunits and the heme containing bacterioferritins, also consisting of 24 H-subunits. Bacterioferritins (∼450 kDa) and bacterial non-heme ferritins (∼470 kDa) from E. coli are essential for growth and store around 2,500–2,700 iron atoms (Andrews et al., 2003). The iron oxide core structure and crystallinity can vary from amorphous to nanocrystalline and often contains a material similar to ferrihydrite (Lewin et al., 2005; Michel et al., 2010b) that naturally forms nanocrystals below 10 nm (Michel et al., 2007).
Due to their iron oxide storage capacity, ferritins have been studied as intrinsic MRI contrast agents (Naumova and Vande Velde, 2018). Researchers have been also eager to use ferritin for in vitro production of MNPs. Early in vitro studies mainly produced maghemite MNPs with horse spleen ferritin (Meldrum et al., 1992; Wong et al., 1998) and human H-chain ferritin (hHf; Uchida et al., 2006). Later, studies with hHf succeeded in magnetite MNP production (Cao et al., 2010; Walls et al., 2013). The authors found a surprisingly high number of iron atoms (8,400) in the hHf cavity (Walls et al., 2013). Convincingly, they argued that magnetite is denser than other iron oxides and that the 7.5 nm wide cavity of hHf would fit nearly 9,000 Fe atoms (Walls et al., 2013). Based on these successful in vitro results, in vivo formation of magnetic ferritins to increase intrinsic cellular MRI contrast and to generate IMCs seems possible. This was tried with mammalian HEK293T cells (Kim et al., 2012). First, the authors found that cultivation in 3 mM ferrous ammonium sulfate supplemented medium and recombinant expression of hHf resulted only in minor intracellular iron accumulation and no cell magnetization. However, additional co-expression of the divalent metal transporter 1 (DMT1) increased intracellular iron content significantly. Only then, the cells were attracted to permanent magnets by 30 μm s–1. The engineered cells were also retained to 25% on a MACS column, but unfortunately no further analyses were performed to characterize the source of cellular magnetism. Another study reported an almost 3-fold increase in magnetic binding of S. cerevisiae cells through expression of a mutated form of the thermostable, homo-multimeric ferritin of Pyrococcus furiosus (Matsumoto et al., 2015). The authors assumed that higher ferritin iron content would increase ferritin magnetization, as recently shown in vitro for hHf (Walls et al., 2013) and therefore selected ferritin mutants with increased iron binding. Their most promising mutant ferritin candidate, L55P, induced an almost 2-fold greater iron accumulation than the wild-type (Matsumoto et al., 2015). Although their mutant ferritin showed paramagnetic behavior at 5 K in SQUID magnetometry measurements and around 60% of mutant-expressing yeast cells were retained on a magnetic column, MRI analysis of the respective yeast cells showed no significant difference of the signals when normalized to the intracellular iron content. This implied no formation of a distinct magnetic iron oxide form, but rather an increase of magnetization through intracellular iron. Similar results were found for an E. coli knockout strain without the native ferritin-clan genes (ftnA, bfr, and dps) and iron exporters (rcnA, fieF, and zntA) that overexpressed a ferritin (FtnA) double-mutant (H34L, T64I) together with iron importers (Liu et al., 2016). SQUID magnetometry measurements of the mutant cells indicated a paramagnetic character and around 70% of cells were retained on a MACS column. However, no distinct formation of magnetic iron oxides in the ferritin cores was stated.
Together, these results highlight that overexpression of ferritins and mutant ferritins with higher iron binding capacity is a promising starting point, but more sophisticated methods to induce in vivo magnetite formation with ferritins are needed. One possible approach was recently shown with a ferritin-M6A chimera (Radoul et al., 2016). The authors fused the charged C-terminal region (M6A) of the MTB Mms6 protein that strongly binds magnetite and is crucial for magnetite biomineralization (Arakaki et al., 2003; Ubago-Rodríguez et al., 2019) to the mouse H-ferritin subunit (Radoul et al., 2016). Ferritin-M6A formed ∼440 kDa structures that were found to be ∼12 nm protein particles with 24 (∼25.8 kDa) monomers able to bind iron. Expression of ferritin-M6A in rat glioma C6 cells resulted in increased iron content, and in vivo analysis of tumor xenografts in mice that expressed ferritin-M6A showed also increased MRI contrast. Summarizing, engineered ferritins have the potential to magnetize cells, their easy expression in various hosts increases their applicability, but definite magnetite formation for strong magnetization of cells requires further research.
Encapsulins
Prokaryotic, proteinaceous proteins, called encapsulins, were structurally described to form large icosahedral shells by intracellular self-assembly of monomers that function as a minimal compartment (Sutter et al., 2008). These nanocompartments are suggested to play an important role in many microbial organisms and form capsids around 25–35 nm in diameter (Nichols et al., 2017). Similar to ferritin, they are pH resistant and temperature stable. However, they have a native set of cargo molecules that are packaged into the shells via a specific short terminal peptide tag that can be used to target other proteins to the shell (Cassidy-Amstutz et al., 2016). Encapsulins were not only successfully produced in prokaryotes (McHugh et al., 2014; Lagoutte et al., 2018), but also in eukaryotes (Lau et al., 2018; Sigmund et al., 2018), which makes them versatile tools for localized reactions. An interesting candidate for the generation of IMCs could be the encapsulin of the gram-negative bacterium Myxococcus xanthus. The M. xanthus encapsulin consists of a shell protein (EncA; 32.5 kDa) and contains three internal proteins (EncB; 17 kDa; EncC; 13 kDa; EncD; 11 kDa; McHugh et al., 2014). Built from 180 EncA monomers, the final encapsulin has an average diameter of 32 nm with an internal diameter of 26 nm. Furthermore, the authors showed that EncB and EncC contain ferritin-like domains attached to the inner encapsulin surface. They further found ∼5 nm electron-dense granules inside the encapsulin and calculated that it could potentially hold up to 30,000 iron atoms, which marks an approximate 10-fold increase compared to ferritin. However, the authors gave no indication on the iron phase inside the encapsulin (McHugh et al., 2014). In a recent study, the authors expressed the EncA protein of M. xanthus together with EncB or EncC in mammalian HEK293T cells (Sigmund et al., 2018). They reported efficient iron encapsulation for MRI contrast and MACS. Cell viability was not negatively affected. Unfortunately, the authors performed no further magnetometry investigations on the cells. Interestingly, they tried to use the beneficial magnetite interacting ability of Mms6 and MamD (Mms7) through fusion of the C-terminal regions to EncA, B, or C. However, they reported no beneficial impact on iron loading compared to the native encapsulin proteins. Nevertheless, they demonstrated that encapsulins are potential candidates for recombinant IMC generation.
Iron-Sulfur Cluster Proteins
Iron-sulfur cluster proteins have a crucial role in various physiological processes. In the oxidized state, [2Fe-2S] cluster proteins have Fe3+ atoms, leading to 5 unpaired electrons at each iron. It has been shown that the two spin-bearing iron centers are coupled to each other through an exchange-driven antiferromagnetic coupling mechanism (Ali M.E. et al., 2015). Some iron-sulfur cluster proteins can be expressed to high titers of 30% total soluble protein (Ta and Vickery, 1992; Gubernator et al., 2003). However to our knowledge, magnetic separation techniques have not been performed with iron-sulfur cluster proteins until recently. Migratory animals are thought to have special, magnetically sensitive receptors that help in navigation on their journeys. Recently, a potential protein candidate was found (Qin et al., 2016). The authors demonstrated that the protein MagR (magnetic receptor), a homologue of the Iron-Sulfur Cluster Assembly 1 (Isca1) protein, when in complex with another protein, cryptochrome (Cry), formed a multimeric protein complex that responded to magnetic fields in vitro. Based on a structural analysis of MagR multimers and the MagR/Cry complex, the authors argued that the magnetic behavior could result from alignment of MagR monomers (Qin et al., 2016). Additionally, they were able to enrich the MagR protein and MagR/Cry complex, respectively, from a complex matrix with a magnet and non-magnetized iron beads (Qin et al., 2016). Later, this method was also shown to be effective to capture MagR fusion proteins from a complex matrix (Jiang et al., 2017; Wang L. et al., 2019). However, the physical capabilities of this protein complex were recently questioned (Meister, 2016; Winklhofer and Mouritsen, 2016) and the putative magnetic properties have been still under review. When MagR-membrane channel constructs were subjected to magnetic stimuli in HEK cells, they were not able to induce significant membrane channel activity in a magnetic field (Pang et al., 2017; Wang G. et al., 2019). Therefore, usability of MagR for IMC generation might be farfetched at this point and research on MagR and similar iron-sulfur cluster proteins is needed.
Envisioned Applications of IMCs
Recombinant Production of Magnetosomes and MNPs
Magnetic nanoparticles have a wide application range in medicine (Li et al., 2016), but their production has to be cost-efficient. Although research on the mass production of MTB has brought fruitful results, common industrially relevant cellular organisms, like E. coli or S. cerevisiae, are easier to cultivate to high cell densities. Therefore, transformation of non-magnetic cells to magnetosome-producing cells is a quest (Kolinko et al., 2014; Uebe and Schüler, 2016). Recently, a patent has been granted (US9913918B2) that protects the heterologous expression of gene cassettes that comprise several MTB operons (Kolinko et al., 2014). Another set of patents describing the introduction of MTB into eukaryotic cells as artificial endosymbionts was recently granted (e.g., US8828681B2). Briefly, it is stated that MTB are genetically modified to survive conditions in the eukaryotic cytosol that further provides sufficient nutrients and micro-oxic conditions for magnetosome formation. Technically, the resulting eukaryotic cell has to be regarded as an IMC. Although not stated specifically, successful generation of MTB-endosymbiotic yeasts could be one way to facilitate mass production. Given the production of superparamagnetic MNPs with an estimated size of 12 nm in mms6 transfected human mesenchymal stem cells (Elfick et al., 2017), production of MNPs, as side products in costly mammalian cell cultures, could be also envisioned, especially in pharmaceutically-relevant mammalian antibody production cell lines.
MRI Contrast
In recent years concerns have arisen surrounding the long-term safety of current Gadolinium(III)-based MRI contrast compounds (Runge, 2017), and this has spurred research into alternatives. It was shown that MTB can produce positive MRI contrast (Benoit et al., 2009). Additionally, although application in humans will be a future challenge, a number of studies focused on the improvement of cellular MRI contrast through genetic engineering of cells through MTB proteins (Zurkiya et al., 2008; Goldhawk et al., 2009; Cho et al., 2014; Rohani et al., 2014; Sengupta et al., 2014; Elfick et al., 2017) and ferritins (Cohen et al., 2005; Matsumoto et al., 2015; Liu et al., 2016; Radoul et al., 2016).
Magnetic Drug and Cell Delivery
Targeted drug delivery refers to predominant drug accumulation at a target zone (Torchilin, 2000). A small number of publications dealt with the use of MTB for magnetic drug and cell delivery in cancer treatment (Martel et al., 2009; Felfoul et al., 2016). In tissue engineering, intrinsically magnetic mesenchymal stem cells could be used to repair tissue damage by tracking, targeting and local long-term retention of cells at the specific site of damage (Kerans et al., 2018).
Magnetic Hyperthermia in Cancer Treatment
Magnetic hyperthermia is based on the concept that MNPs generate heat when exposed to an external, alternating magnetic field (Carrey et al., 2011; Beik et al., 2016). In hyperthermia therapy, heat is increased within or near a tumor to induce cellular changes including protein denaturation, damage to the cytoskeleton and disruption of DNA repair that lead to cell death (Jha et al., 2016). Studies with M. gryphiswaldense and M. magneticum AMB-1 showed that IMCs present proper tools for magnetic hyperthermia (Alphandéry et al., 2011; Gandia et al., 2019). Additionally, a recent review mentioned the potential use of intrinsically magnetic mesenchymal stem cells for localized magnetic hyperthermia treatment, but also highlighted that future studies have to generate a sufficient amount of intracellular MNPs (Kerans et al., 2018).
IMCs in Bioprocess Engineering
Intrinsically magnetic cells are interesting for immobilized, whole cell catalysis that often uses magnetic beads for cell immobilization (Al-Qodah et al., 2018). With IMCs, immobilization of cells can be solely done through magnetic fields, which eliminates chemical coupling to bead surfaces.
Some cells, especially mammalian cells, are very sensitive to shear forces, which could be minimized through magnetic harvesting. Once mammalian cells exhibit magnetic behavior, they can be simply harvested through a magnetic field that attracts cells to the walls or bottom of the vessel, rather than using stressful centrifugation and filtration. The same approach might be applied for cell retention in continuous bioprocessing.
Outlook
Simple overexpression of iron importers to increase intracellular iron concentrations is not the method of choice to magnetize cells. Free iron cations induce Fenton reactions and disturb iron homeostasis and show only little magnetic properties (Buck et al., 2015). Also the use of heme-proteins to magnetize cells seems to be limited. Researchers showed that heme-proteins can be expressed to high intracellular titers, but their magnetic character is only sufficiently present under oxygen-free or highly oxidizing conditions (Sofla et al., 2013). Moreover, the example of erythrocytes shows that extensive amounts of heme-proteins must be present for cell magnetization, which clearly limits recombinant approaches. Similarly, MagR might not be usable for IMC generation, since current in vivo studies failed. Better prospects for IMC generation are clearly envisioned for MTB proteins, ferritins and encapsulins.
Magnetotactic bacteria accumulate magnetic minerals to around 3% of their DCW, their magnetosomes show single-domain magnetic behavior, have sizes between 35 and 120 nm and are chain-like arranged. MTB present the golden standard of an IMC. Genetic engineering of non-magnetic hosts like R. rubrum with 29 magnetosome genes from M. gryphiswaldense yielded a magnetic cell (Kolinko et al., 2014). This study also yielded the largest recombinantly produced MNPs (∼24 nm) in an initially non-magnetic cell. However, transferring a large pool of MTB proteins to non-magnetic hosts does not necessarily yield IMCs, as shown for non-magnetic Magnetospirillum species (Dziuba et al., 2020). We also argue that expression of MTB proteins might be tricky in common industrial hosts, like the bacterium E. coli. The work of Juodeikis in 2016 presents a good overview on soluble and insoluble production of various MTB proteins in E. coli (Juodeikis, 2016). Although recombinant overexpression of MTB proteins (e.g., MagA, MmsF, or Mms6) showed better results in eukaryotic hosts, probably due to the better equipped protein folding machinery and posttranslational protein modifications (Barber and Rinehart, 2018), we argue that increasing the soluble protein expression must be a priority to successfully generate IMCs with MTB proteins. The amino acid sequence QKFVPKSTN, crucial for magnetite interaction and improvement of magnetite particle appearance, seems to be especially promising for these approaches (Rawlings et al., 2015). Furthermore, we hypothesize that the Mms6 protein marks a proper starting point for IMC generation (Elfick et al., 2017).
Compared to MTB proteins, ferritins are limited in their possible MNP size (∼5–8 nm). Therefore, ferritins could be the proteins of choice for in vivo production of superparamagnetic MNPs. Their ubiquitous occurrence and soluble character makes them perfect for recombinant expression. Native ferritins naturally contain no magnetite, but rather amorphous or crystalline ferrihydrite that forms crystals below 10 nm. However, it was already proven that ferritins can form relatively pure magnetite in vitro (Walls et al., 2013). Weak cell magnetization with ferritins was already achieved in bacteria (Liu et al., 2016), yeasts (Matsumoto et al., 2015), and mammalian cells (Kim et al., 2012), but unfortunately no study reported magnetite in vivo. Radoul et al. (2016) presented a promising ferritin-M6A fusion to increase in vivo magnetite formation. It would be interesting to investigate how this ferritin-M6A fusion can be improved in iron loading through mutagenesis.
Similar to ferritins, encapsulins provide a protecting shell for iron oxide formation, but research on encapsulins has only started. Through their ability to form >20 nm nanocompartments, good expression levels in prokaryotes and eukaryotes and protein targeting inside these nanocompartments, their usability for localized magnetite forming reactions seems promising. Native M. xanthus encapsulin weakly magnetized mammalian cells in vivo only through the amount of iron stored in its inner core (Sigmund et al., 2018). The authors also tried to improve the encapsulin’s iron storage abilities through fusions of MTB protein peptides, but this resulted in no improvement. However, we argue that further studies should be performed with encapsulins. It might be interesting to investigate how hypoxic conditions affect iron oxide content of encapsulins and if engineered encapsulin proteins could be used to produce rather big, single-domain iron oxide particles.
Author Contributions
AP read the literature and drafted the manuscript. OS supervised and corrected the manuscript draft. All authors contributed to the article and approved the submitted version.
Funding
The authors acknowledge the TU Wien Bibliothek for financial support through its Open Access Funding Program.
Conflict of Interest
The authors declare that the research was conducted in the absence of any commercial or financial relationships that could be construed as a potential conflict of interest.
Abbreviations
DCW, dry cell weight; hHf, human H-chain ferritin; IMCs, intrinsically magnetic cells; M6A, charged C-terminal region of the MTB Mms6 protein; MACS, magnet-assisted cell separation; mam, magnetosome membrane; mms, magnetic-particle-membrane specific; MNPs, magnetic nanoparticles; MPI, magnetic particle imaging; MRI, magnetic resonance imaging; MTB, magnetotactic bacteria; P + MNP, protein coupled to magnetic nanoparticles; SQUID, superconducting quantum interference device; TC, Curie-temperature; TEM, transmission electron microscopy; TN, Néel-temperature; VSM, vibrating sample mode; XRD, X-ray diffraction.
References
Abdolmaleki, P., Ghanati, F., Sahebjamei, H., and Sarvestani, A. S. (2007). Peroxidase activity, lignification and promotion of cell death in tobacco cells exposed to static magnetic field. Environmentalist 27, 435–440. doi: 10.1007/s10669-007-9080-1
Abreu, N., Mannoubi, S., Ozyamak, E., Pignol, D., Ginet, N., and Komeili, A. (2014). Interplay between two bacterial actin homologs, MamK and MamK-like, is required for the alignment of magnetosome organelles in Magnetospirillum magneticum AMB-1. J. Bacteriol. 196, 3111–3121. doi: 10.1128/jb.01674-14
Ali, I., Peng, C., Khan, Z. M., and Naz, I. (2017). Yield cultivation of magnetotactic bacteria and magnetosomes: a review. J. Basic Microbiol. 57, 643–652. doi: 10.1002/jobm.201700052
Ali, K., Sarfraz, A. K., Mirza, I. M., Bahadur, A., Iqbal, S., and ul Haq, A. (2015). Preparation of superparamagnetic maghemite (γ-Fe2O3) nanoparticles by wet chemical route and investigation of their magnetic and dielectric properties. Curr. Appl. Phys. 15, 925–929. doi: 10.1016/j.cap.2015.04.030
Ali, M. E., Staemmler, V., and Marx, D. (2015). Magnetostructural dynamics of rieske versus ferredoxin iron–sulfur cofactors. Phys. Chem. Chem. Phys. 17, 6289–6296. doi: 10.1039/C4CP05465B
Alphandéry, E. (2014). Applications of magnetosomes synthesized by magnetotactic bacteria in medicine. Front. Bioeng. Biotechnol. 2:5. doi: 10.3389/fbioe.2014.00005
Alphandéry, E., Faure, S., Raison, L., Duguet, E., Howse, P. A., and Bazylinski, D. A. (2011). Heat production by bacterial magnetosomes exposed to an oscillating magnetic field. J. Phys. Chem. C 115, 18–22. doi: 10.1021/jp104580t
Al-Qodah, Z., Al-Shannag, M., Al-Bosoul, M., Penchev, I., Al-Ahmadi, H., and Al-Qodah, K. (2018). On the performance of immobilized cell bioreactors utilizing a magnetic field. Rev. Chem. Eng. 34:385. doi: 10.1515/revce-2016-0059
Amemiya, Y., Arakaki, A., Staniland, S. S., Tanaka, T., and Matsunaga, T. (2007). Controlled formation of magnetite crystal by partial oxidation of ferrous hydroxide in the presence of recombinant magnetotactic bacterial protein Mms6. Biomaterials 28, 5381–5389. doi: 10.1016/j.biomaterials.2007.07.051
Amor, M., Mathon, F. P., Monteil, C. L., Busigny, V., and Lefevre, C. T. (2020). Iron-biomineralizing organelle in magnetotactic bacteria: function, synthesis and preservation in ancient rock samples. Environ. Microbiol. doi: 10.1111/1462-2920.15098 [Epub ahead of print].
Andrews, S. C., Robinson, A. K., and Rodríguez-Quiñones, F. (2003). Bacterial iron homeostasis. FEMS Microbiol. Rev. 27, 215–237. doi: 10.1016/s0168-6445(03)00055-x
Arakaki, A., Kikuchi, D., Tanaka, M., Yamagishi, A., Yoda, T., and Matsunaga, T. (2016). Comparative subcellular localization analysis of magnetosome proteins reveals a unique localization behavior of Mms6 protein onto magnetite crystals. J. Bacteriol. 198, 2794–2802. doi: 10.1128/jb.00280-16
Arakaki, A., Masuda, F., Amemiya, Y., Tanaka, T., and Matsunaga, T. (2010). Control of the morphology and size of magnetite particles with peptides mimicking the Mms6 protein from magnetotactic bacteria. J. Colloid Interface Sci. 343, 65–70. doi: 10.1016/j.jcis.2009.11.043
Arakaki, A., Webb, J., and Matsunaga, T. (2003). A novel protein tightly bound to bacterial magnetic particles in Magnetospirillum magneticum strain AMB-1. J. Biol. Chem. 278, 8745–8750. doi: 10.1074/jbc.M211729200
Arakaki, A., Yamagishi, A., Fukuyo, A., Tanaka, M., and Matsunaga, T. (2014). Co-ordinated functions of Mms proteins define the surface structure of cubo-octahedral magnetite crystals in magnetotactic bacteria. Mol. Microbiol. 93, 554–567. doi: 10.1111/mmi.12683
Araujo, A. C., Abreu, F., Silva, K. T., Bazylinski, D. A., and Lins, U. (2015). Magnetotactic bacteria as potential sources of bioproducts. Mar. Drugs 13, 389–430. doi: 10.3390/md13010389
Barber, K. W., and Rinehart, J. (2018). The ABCs of PTMs. Nat. Chem. Biol. 14, 188–192. doi: 10.1038/nchembio.2572
Barber-Zucker, S., and Zarivach, R. (2017). A look into the biochemistry of magnetosome biosynthesis in magnetotactic bacteria. ACS Chem. Biol. 12, 13–22. doi: 10.1021/acschembio.6b01000
Bazylinski, D. A., and Frankel, R. B. (2004). Magnetosome formation in prokaryotes. Nat. Rev. Microbiol. 2, 217–230. doi: 10.1038/nrmicro842
Bazylinski, D. A., Williams, T. J., Lefèvre, C. T., Berg, R. J., Zhang, C. L., Bowser, S. S., et al. (2013). Magnetococcus marinus gen. nov., sp. nov., a marine, magnetotactic bacterium that represents a novel lineage (Magnetococcaceae fam. nov., Magnetococcales ord. nov.) at the base of the Alphaproteobacteria. Int. J. Syst. Evol. Microbiol. 63, 801–808. doi: 10.1099/ijs.0.038927-0
Beik, J., Abed, Z., Ghoreishi, F. S., Hosseini-Nami, S., Mehrzadi, S., Shakeri-Zadeh, A., et al. (2016). Nanotechnology in hyperthermia cancer therapy: from fundamental principles to advanced applications. J. Control. Release 235, 205–221. doi: 10.1016/j.jconrel.2016.05.062
Benoit, M. R., Mayer, D., Barak, Y., Chen, I. Y., Hu, W., Cheng, Z., et al. (2009). Visualizing implanted tumors in mice with magnetic resonance imaging using magnetotactic bacteria. Clin. Cancer Res. 15, 5170–5177. doi: 10.1158/1078-0432.ccr-08-3206
Berny, C., Le Fèvre, R., Guyot, F., Blondeau, K., Guizonne, C., Rousseau, E., et al. (2020). A method for producing highly pure magnetosomes in large quantity for medical applications using Magnetospirillum gryphiswaldense MSR-1 magnetotactic bacteria amplified in minimal growth media. Front. Bioeng. Biotechnol. 8:16. doi: 10.3389/fbioe.2020.00016
Berridge, B. R., Van Vleet, J. F., and Herman, E. (2013). “Chapter 46 - cardiac, vascular, and skeletal muscle systems,” in Haschek and Rousseaux’s Handbook of Toxicologic Pathology, 3rd Edn, eds W. M. Haschek, C. G. Rousseaux, and M. A. Wallig (Boston, MA: Academic Press), 1567–1665. doi: 10.1016/b978-0-12-415759-0.00046-7
Bird, S. M., Galloway, J. M., Rawlings, A. E., Bramble, J. P., and Staniland, S. S. (2015). Taking a hard line with biotemplating: cobalt-doped magnetite magnetic nanoparticle arrays. Nanoscale 7, 7340–7351. doi: 10.1039/c5nr00651a
Blakemore, R. P. (1982). Magnetotactic bacteria. Annu. Rev. Microbiol. 36, 217–238. doi: 10.1146/annurev.mi.36.100182.001245
Blakemore, R. P., Maratea, D., and Wolfe, R. S. (1979). Isolation and pure culture of a freshwater magnetic spirillum in chemically defined medium. J. Bacteriol. 140, 720–729. doi: 10.1128/jb.140.2.720-729.1979
Bødker, F., Hansen, M. F., Koch, C. B., Lefmann, K., and Mørup, S. (2000). Magnetic properties of hematite nanoparticles. Phys. Rev. B 61, 6826–6838. doi: 10.1103/PhysRevB.61.6826
Bou-Abdallah, F. (2010). The iron redox and hydrolysis chemistry of the ferritins. Biochim. Biophys. Acta 1800, 719–731. doi: 10.1016/j.bbagen.2010.03.021
Buck, A., Moore, L. R., Lane, C. D., Kumar, A., Stroff, C., White, N., et al. (2015). Magnetic separation of algae genetically modified for increased intracellular iron uptake. J. Magn. Magn. Mater. 380, 201–204. doi: 10.1016/j.jmmm.2014.09.008
Cao, C., Tian, L., Liu, Q., Liu, W., Chen, G., and Pan, Y. (2010). Magnetic characterization of noninteracting, randomly oriented, nanometer-scale ferrimagnetic particles. J. Geophys. Res. Solid Earth 115:B07103. doi: 10.1029/2009jb006855
Carrey, J., Mehdaoui, B., and Respaud, M. (2011). Simple models for dynamic hysteresis loop calculations of magnetic single-domain nanoparticles: application to magnetic hyperthermia optimization. J. Appl. Phys. 109:083921. doi: 10.1063/1.3551582
Cassidy-Amstutz, C., Oltrogge, L., Going, C. C., Lee, A., Teng, P., Quintanilla, D., et al. (2016). Identification of a minimal peptide tag for in vivo and in vitro loading of encapsulin. Biochemistry 55, 3461–3468. doi: 10.1021/acs.biochem.6b00294
Chang, L., Roberts, A. P., Tang, Y., Rainford, B. D., Muxworthy, A. R., and Chen, Q. (2008). Fundamental magnetic parameters from pure synthetic greigite (Fe3S4). J. Geophys. Res. Solid Earth 113:B06104. doi: 10.1029/2007jb005502
Chasteen, N. D., and Harrison, P. M. (1999). Mineralization in ferritin: an efficient means of iron storage. J. Struct. Biol. 126, 182–194. doi: 10.1006/jsbi.1999.4118
Cho, I. K., Moran, S. P., Paudyal, R., Piotrowska-Nitsche, K., Cheng, P.-H., Zhang, X., et al. (2014). Longitudinal monitoring of stem cell grafts in vivo using magnetic resonance imaging with inducible magA as a genetic reporter. Theranostics 4, 972–989. doi: 10.7150/thno.9436
Chow, K.-C., and Tung, W. L. (2000). Magnetic field exposure stimulates transposition through the induction of DnaK/J synthesis. Biochem. Biophys. Res. Commun. 270, 745–748. doi: 10.1006/bbrc.2000.2496
Cohen, B., Dafni, H., Meir, G., Harmelin, A., and Neeman, M. (2005). Ferritin as an endogenous MRI reporter for noninvasive imaging of gene expression in C6 glioma tumors. Neoplasia 7, 109–117. doi: 10.1593/neo.04436
Cornell, R. M., and Schwertmann, U. (2003). The Iron Oxides: Structure, Properties, Reactions, Occurrences and Uses. Hoboken, NJ: John Wiley & Sons.
Crichton, R. R., Wilmet, S., Legssyer, R., and Ward, R. J. (2002). Molecular and cellular mechanisms of iron homeostasis and toxicity in mammalian cells. J. Inorg. Biochem. 91, 9–18. doi: 10.1016/s0162-0134(02)00461-0
Curcio, A., Van de Walle, A., Serrano, A., Preveral, S., Péchoux, C., Pignol, D., et al. (2020). Transformation cycle of magnetosomes in human stem cells: from degradation to biosynthesis of magnetic nanoparticles anew. ACS Nano 14, 1406–1417. doi: 10.1021/acsnano.9b08061
Del Re, B., Bersani, F., Mesirca, P., and Giorgi, G. (2006). Synthesis of DnaK and GroEL in Escherichia coli cells exposed to different magnetic field signals. Bioelectrochemistry 69, 99–103. doi: 10.1016/j.bioelechem.2005.11.006
Dziuba, M. V., Zwiener, T., Uebe, R., and Schüler, D. (2020). Single-step transfer of biosynthetic operons endows a non-magnetotactic Magnetospirillum strain from wetland with magnetosome biosynthesis. Environ. Microbiol. 22, 1603–1618. doi: 10.1111/1462-2920.14950
Egan, T. J. (2008). Haemozoin formation. Mol. Biochem. Parasitol. 157, 127–136. doi: 10.1016/j.molbiopara.2007.11.005
Elfick, A., Rischitor, G., Mouras, R., Azfer, A., Lungaro, L., Uhlarz, M., et al. (2017). Biosynthesis of magnetic nanoparticles by human mesenchymal stem cells following transfection with the magnetotactic bacterial gene mms6. Sci. Rep. 7:39755. doi: 10.1038/srep39755
Faivre, D., and Godec, T. U. (2015). From bacteria to mollusks: the principles underlying the biomineralization of iron oxide materials. Angew. Chem. Int. Ed. Engl. 54, 4728–4747. doi: 10.1002/anie.201408900
Felfoul, O., Mohammadi, M., Taherkhani, S., de Lanauze, D., Zhong Xu, Y., Loghin, D., et al. (2016). Magneto-aerotactic bacteria deliver drug-containing nanoliposomes to tumour hypoxic regions. Nat. Nanotechnol. 11, 941–947. doi: 10.1038/nnano.2016.137
Flies, C. B., Jonkers, H. M., de Beer, D., Bosselmann, K., Böttcher, M. E., and Schüler, D. (2005). Diversity and vertical distribution of magnetotactic bacteria along chemical gradients in freshwater microcosms. FEMS Microbiol. Ecol. 52, 185–195. doi: 10.1016/j.femsec.2004.11.006
Foner, S. (1959). Versatile and sensitive vibrating-sample magnetometer. Rev. Sci. Instrum. 30, 548–557. doi: 10.1063/1.1716679
Fraser, R. Z., Shitut, M., Agrawal, P., Mendes, O., and Klapholz, S. (2018). Safety evaluation of soy leghemoglobin protein preparation derived from Pichia pastoris, intended for use as a flavor catalyst in plant-based meat. Int. J. Toxicol. 37, 241–262. doi: 10.1177/1091581818766318
Fukuda, Y., Okamura, Y., Takeyama, H., and Matsunaga, T. (2006). Dynamic analysis of a genomic island in Magnetospirillum sp. strain AMB-1 reveals how magnetosome synthesis developed. FEBS Lett. 580, 801–812. doi: 10.1016/j.febslet.2006.01.003
Gahlawat, G., and Choudhury, A. R. (2019). A review on the biosynthesis of metal and metal salt nanoparticles by microbes. RSC Adv. 9, 12944–12967. doi: 10.1039/C8RA10483B
Galloway, J. M., Arakaki, A., Masuda, F., Tanaka, T., Matsunaga, T., and Staniland, S. S. (2011). Magnetic bacterial protein Mms6 controls morphology, crystallinity and magnetism of cobalt-doped magnetite nanoparticles in vitro. J. Mater. Chem. 21, 15244–15254. doi: 10.1039/C1JM12003D
Gandia, D., Gandarias, L., Rodrigo, I., Robles-García, J., Das, R., Garaio, E., et al. (2019). Unlocking the potential of magnetotactic bacteria as magnetic hyperthermia agents. Small 15:1902626. doi: 10.1002/smll.201902626
Goldhawk, D. E., Lemaire, C., McCreary, C. R., McGirr, R., Dhanvantari, S., Thompson, R. T., et al. (2009). Magnetic resonance imaging of cells overexpressing MagA, an endogenous contrast agent for live cell imaging. Mol. Imaging 8, 129–139. doi: 10.2310/7290.2009.00006
Gorobets, O., Gorobets, S., and Koralewski, M. (2017). Physiological origin of biogenic magnetic nanoparticles in health and disease: from bacteria to humans. Int. J. Nanomed. 12, 4371–4395. doi: 10.2147/IJN.S130565
Grünberg, K., Müller, E.-C., Otto, A., Reszka, R., Linder, D., Kube, M., et al. (2004). Biochemical and proteomic analysis of the magnetosome membrane in Magnetospirillum gryphiswaldense. Appl. Environ. Microbiol. 70, 1040–1050. doi: 10.1128/aem.70.2.1040-1050.2004
Gubernator, B., Seidler, A., Rögner, M., and Szczepaniak, A. (2003). Overexpression and reconstitution of a Rieske iron–sulfur protein from the higher plant. Protein Expr. Purif. 29, 8–14. doi: 10.1016/s1046-5928(03)00016-0
Guo, F. F., Yang, W., Jiang, W., Geng, S., Peng, T., and Li, J. L. (2012). Magnetosomes eliminate intracellular reactive oxygen species in Magnetospirillum gryphiswaldense MSR-1. Environ. Microbiol. 14, 1722–1729. doi: 10.1111/j.1462-2920.2012.02707.x
Gütlich, P., and Schröder, C. (2012). “Mössbauer spectroscopy,” in Methods in Physical Chemistry, eds R. Schäfer and P. C. Schmidt (Weinheim: Wiley-VCH), 351–389.
Harte, F., Martin, M. F. S., Lacerda, A. H., Lelieveld, H. L. M., Swanson, B. G., and Barbosa-Cánovas, G. V. (2001). Potential use of 18 tesla static and pulsed magnetic fields on Escherichia coli and Saccharomyces cerevisiae. J. Food Process. Preserv. 25, 223–235. doi: 10.1111/j.1745-4549.2001.tb00456.x
Heyen, U., and Schüler, D. (2003). Growth and magnetosome formation by microaerophilic Magnetospirillum strains in an oxygen-controlled fermentor. Appl. Microbiol. Biotechnol. 61, 536–544. doi: 10.1007/s00253-002-1219-x
Hoffman, S. J., Looker, D. L., Roehrich, J. M., Cozart, P. E., Durfee, S. L., Tedesco, J. L., et al. (1990). Expression of fully functional tetrameric human hemoglobin in Escherichia coli. Proc. Natl. Acad. Sci. U.S.A. 87, 8521–8525. doi: 10.1073/pnas.87.21.8521
Hongping, H., Zhong, Y., Liang, X., Wei, T., Jianxi, Z., and Wang, C. Y. (2015). Natural magnetite: an efficient catalyst for the degradation of organic contaminant. Sci. Rep. 5:10139.
Huang, J., Li, Y., Orza, A., Lu, Q., Guo, P., Wang, L., et al. (2016). Magnetic nanoparticle facilitated drug delivery for cancer therapy with targeted and image-guided approaches. Adv. Funct. Mater. 26, 3818–3836. doi: 10.1002/adfm.201504185
Huber, D. L. (2005). Synthesis, properties, and applications of iron nanoparticles. Small 1, 482–501. doi: 10.1002/smll.200500006
Imlay, J. A. (2008). Cellular defenses against superoxide and hydrogen peroxide. Annu. Rev. Biochem. 77, 755–776. doi: 10.1146/annurev.biochem.77.061606.161055
Jenks, W., Sadeghi, S., and Wikswo, J. P. Jr. (1997). SQUIDs for nondestructive evaluation. J. Phys. D Appl. Phys. 30, 293–323.
Jha, S., Sharma, P. K., and Malviya, R. (2016). Hyperthermia: role and risk factor for cancer treatment. Achiev. Life Sci. 10, 161–167. doi: 10.1016/j.als.2016.11.004
Jiang, M., Zhang, L., Wang, F., Zhang, J., Liu, G., Gao, B., et al. (2017). Novel application of magnetic protein: convenient one-step purification and immobilization of proteins. Sci. Rep. 7:13329. doi: 10.1038/s41598-017-13648-x
Juodeikis, R. (2016). Engineering Membranes in Escherichia coli: The Magnetosome, LemA Protein Family and Outer Membrane Vesicles. Canterbury: University of Kent.
Jutz, G., van Rijn, P., Santos Miranda, B., and Böker, A. (2015). Ferritin: a versatile building block for bionanotechnology. Chem. Rev. 115, 1653–1701. doi: 10.1021/cr400011b
Kamnev, A. A., and Tugarova, A. V. (2017). Sample treatment in Mössbauer spectroscopy for protein-related analyses: nondestructive possibilities to look inside metal-containing biosystems. Talanta 174, 819–837. doi: 10.1016/j.talanta.2017.06.057
Kemsheadl, J. T., and Ugelstad, J. (1985). Magnetic separation techniques: their application to medicine. Mol. Cell. Biochem. 67, 11–18. doi: 10.1007/BF00220980
Kerans, F. F. A., Lungaro, L., Azfer, A., and Salter, D. M. (2018). The potential of intrinsically magnetic mesenchymal stem cells for tissue engineering. Int. J. Mol. Sci. 19:3159. doi: 10.3390/ijms19103159
Keren-Khadmy, N., Zeytuni, N., Kutnowski, N., Perriere, G., Monteil, C., and Zarivach, R. (2020). From conservation to structure, studies of magnetosome associated cation diffusion facilitators (CDF) proteins in Proteobacteria. PLoS One 15:e0231839. doi: 10.1371/journal.pone.0231839
Keune, W. (2012). Application of Mössbauer spectroscopy in magnetism. Hyperfine Interact. 204, 13–45. doi: 10.1007/978-94-007-4762-3_5
Khoshouei, M., Danev, R., Plitzko, J. M., and Baumeister, W. (2017). Revisiting the structure of hemoglobin and myoglobin with cryo-electron microscopy. J. Mol. Biol. 429, 2611–2618. doi: 10.1016/j.jmb.2017.07.004
Kim, T., Moore, D., and Fussenegger, M. (2012). Genetically programmed superparamagnetic behavior of mammalian cells. J. Biotechnol. 162, 237–245. doi: 10.1016/j.jbiotec.2012.09.019
Kobayashi, T. (2011). Cancer hyperthermia using magnetic nanoparticles. Biotechnol. J. 6, 1342–1347. doi: 10.1002/biot.201100045
Kolinko, I., Lohße, A., Borg, S., Raschdorf, O., Jogler, C., Tu, Q., et al. (2014). Biosynthesis of magnetic nanostructures in a foreign organism by transfer of bacterial magnetosome gene clusters. Nat. Nanotechnol. 9, 193–197. doi: 10.1038/nnano.2014.13
Komeili, A., Vali, H., Beveridge, T. J., and Newman, D. K. (2004). Magnetosome vesicles are present before magnetite formation, and MamA is required for their activation. Proc. Natl. Acad. Sci. U.S.A. 101, 3839–3844. doi: 10.1073/pnas.0400391101
Konhauser, K. O., Kappler, A., and Roden, E. E. (2011). Iron in microbial metabolisms. Elements 7, 89–93. doi: 10.2113/gselements.7.2.89
Kraupner, A., Eberbeck, D., Heinke, D., Uebe, R., Schüler, D., and Briel, A. (2017). Bacterial magnetosomes – nature’s powerful contribution to MPI tracer research. Nanoscale 9, 5788–5793. doi: 10.1039/C7NR01530E
Kuhara, M., Takeyama, H., Tanaka, T., and Matsunaga, T. (2004). Magnetic cell separation using antibody binding with protein a expressed on bacterial magnetic particles. Anal. Chem. 76, 6207–6213. doi: 10.1021/ac0493727
Kurokawa, D., Gueriba, J. S., and Diño, W. A. (2018). Spin-dependent O2 binding to hemoglobin. ACS Omega 3, 9241–9245. doi: 10.1021/acsomega.8b00879
Lagoutte, P., Mignon, C., Stadthagen, G., Potisopon, S., Donnat, S., Mast, J., et al. (2018). Simultaneous surface display and cargo loading of encapsulin nanocompartments and their use for rational vaccine design. Vaccine 36, 3622–3628. doi: 10.1016/j.vaccine.2018.05.034
Lang, C., and Schüler, D. (2008). Expression of green fluorescent protein fused to magnetosome proteins in microaerophilic magnetotactic bacteria. Appl. Environ. Microbiol. 74, 4944–4953. doi: 10.1128/aem.00231-08
László, J., and Kutasi, J. (2010). Static magnetic field exposure fails to affect the viability of different bacteria strains. Bioelectromagnetics 31, 220–225. doi: 10.1002/bem.20551
Lau, Y. H., Giessen, T. W., Altenburg, W. J., and Silver, P. A. (2018). Prokaryotic nanocompartments form synthetic organelles in a eukaryote. Nat. Commun. 9:1311. doi: 10.1038/s41467-018-03768-x
Lawson, D. M., Artymiuk, P. J., Yewdall, S. J., Smith, J. M. A., Livingstone, J. C., Treffry, A., et al. (1991). Solving the structure of human H ferritin by genetically engineering intermolecular crystal contacts. Nature 349, 541–544. doi: 10.1038/349541a0
Lawson, D. M., Treffry, A., Artymiuk, P. J., Harrison, P. M., Yewdall, S. J., Luzzago, A., et al. (1989). Identification of the ferroxidase centre in ferritin. FEBS Lett. 254, 207–210. doi: 10.1016/0014-5793(89)81040-3
Lefèvre, C. T., Bernadac, A., Yu-Zhang, K., Pradel, N., and Wu, L.-F. (2009). Isolation and characterization of a magnetotactic bacterial culture from the Mediterranean Sea. Environ. Microbiol. 11, 1646–1657. doi: 10.1111/j.1462-2920.2009.01887.x
Lefèvre, C. T., Menguy, N., Abreu, F., Lins, U., Pósfai, M., Prozorov, T., et al. (2011). A cultured greigite-producing magnetotactic bacterium in a novel group of sulfate-reducing bacteria. Science 334, 1720–1723. doi: 10.1126/science.1212596
Lefèvre, C. T., Trubitsyn, D., Abreu, F., Kolinko, S., Jogler, C., de Almeida, L. G. P., et al. (2013). Comparative genomic analysis of magnetotactic bacteria from the Deltaproteobacteria provides new insights into magnetite and greigite magnetosome genes required for magnetotaxis. Environ. Microbiol. 15, 2712–2735. doi: 10.1111/1462-2920.12128
Lewin, A., Moore, G. R., and Le Brun, N. E. (2005). Formation of protein-coated iron minerals. Dalton Trans. 22, 3597–3610. doi: 10.1039/B506071K
Li, K., Chen, C., Chen, C., Wang, Y., Wei, Z., Pan, W., et al. (2015). Magnetosomes extracted from Magnetospirillum magneticum strain AMB-1 showed enhanced peroxidase-like activity under visible-light irradiation. Enzyme Microb. Technol. 72, 72–78. doi: 10.1016/j.enzmictec.2015.02.009
Li, Q., Kartikowati, C. W., Horie, S., Ogi, T., Iwaki, T., and Okuyama, K. (2017). Correlation between particle size/domain structure and magnetic properties of highly crystalline Fe3O4 nanoparticles. Sci. Rep. 7:9894.
Li, X., Wei, J., Aifantis, K. E., Fan, Y., Feng, Q., Cui, F.-Z., et al. (2016). Current investigations into magnetic nanoparticles for biomedical applications. J. Biomed. Mater. Res. Part A 104, 1285–1296. doi: 10.1002/jbm.a.35654
Li, X., Xu, H., Chen, Z.-S., and Chen, G. (2011). Biosynthesis of nanoparticles by microorganisms and their applications. J. Nanomater. 2011:270974. doi: 10.1155/2011/270974
Lin-Cereghino, G. P., Godfrey, L., de la Cruz, B. J., Johnson, S., Khuongsathiene, S., Tolstorukov, I., et al. (2006). Mxr1p, a key regulator of the methanol utilization pathway and peroxisomal genes in Pichia pastoris. Mol. Cell. Biol. 26, 883–897. doi: 10.1128/MCB.26.3.883-897.2006
Liu, D., Hong, Y., Li, Y., Hu, C., Yip, T.-C., Yu, W.-K., et al. (2020). Targeted destruction of cancer stem cells using multifunctional magnetic nanoparticles that enable combined hyperthermia and chemotherapy. Theranostics 10, 1181–1196. doi: 10.7150/thno.38989
Liu, L., Alizadeh, K., Donnelly, S. C., Dassanayake, P., Hou, T. T., McGirr, R., et al. (2019). MagA expression attenuates iron export activity in undifferentiated multipotent P19 cells. PLoS One 14:e0217842. doi: 10.1371/journal.pone.0217842
Liu, X., Lopez, P. A., Giessen, T. W., Giles, M., Way, J. C., and Silver, P. A. (2016). Engineering genetically-encoded mineralization and magnetism via directed evolution. Sci. Rep. 6:38019. doi: 10.1038/srep38019
Lohße, A., Ullrich, S., Katzmann, E., Borg, S., Wanner, G., Richter, M., et al. (2011). Functional analysis of the magnetosome island in Magnetospirillum gryphiswaldense: the mamAB operon is sufficient for magnetite biomineralization. PLoS One 6:e25561. doi: 10.1371/journal.pone.0025561
Lopez-Moreno, R., Fernández-Vivas, A., Valverde-Tercedor, C., Azuaga Fortes, A. I., Casares Atienza, S., Rodriguez-Navarro, A. B., et al. (2017). Magnetite nanoparticles biomineralization in the presence of the magnetosome membrane protein MamC: effect of protein aggregation and protein structure on magnetite formation. Cryst. Growth Des. 17, 1620–1629. doi: 10.1021/acs.cgd.6b01643
Lyubutin, I. S., Starchikov, S. S., Lin, C.-R., Lu, S.-Z., Shaikh, M. O., Funtov, K. O., et al. (2013). Magnetic, structural, and electronic properties of iron sulfide Fe3S4 nanoparticles synthesized by the polyol mediated process. J. Nanopart. Res. 15:1397.
Mann, S., Sparks, N. H., Frankel, R. B., Bazylinski, D. A., and Jannasch, H. W. (1990). Biomineralization of ferrimagnetic greigite (Fe 3 S 4) and iron pyrite (FeS 2) in a magnetotactic bacterium. Nature 343, 258–261. doi: 10.1038/343258a0
Martel, S., Mohammadi, M., Felfoul, O., Lu, Z., and Pouponneau, P. (2009). Flagellated magnetotactic bacteria as controlled MRI-trackable propulsion and steering systems for medical nanorobots operating in the human microvasculature. Int. J. Robot. Res. 28, 571–582. doi: 10.1177/0278364908100924
Martínez, J. L., Liu, L., Petranovic, D., and Nielsen, J. (2015). Engineering the oxygen sensing regulation results in an enhanced recombinant human hemoglobin production by Saccharomyces cerevisiae. Biotechnol. Bioeng. 112, 181–188. doi: 10.1002/bit.25347
Martsev, S. P., Vlasov, A. P., and Arosio, P. (1998). Distinct stability of recombinant L and H subunits of human ferritin: calorimetric and ANS binding studies. Protein Eng. Des. Sel. 11, 377–381. doi: 10.1093/protein/11.5.377
Matsumoto, Y., Chen, R., Anikeeva, P., and Jasanoff, A. (2015). Engineering intracellular biomineralization and biosensing by a magnetic protein. Nat. Commun. 6:8721. doi: 10.1038/ncomms9721
Matsunaga, T., Sakaguchi, T., and Tadakoro, F. (1991). Magnetite formation by a magnetic bacterium capable of growing aerobically. Appl. Microbiol. Biotechnol. 35, 651–655. doi: 10.1007/BF00169632
McCausland, H. C., and Komeili, A. (2020). Magnetic genes: studying the genetics of biomineralization in magnetotactic bacteria. PLoS Genet. 16:e1008499. doi: 10.1371/journal.pgen.1008499
McCloskey, K. E., Chalmers, J. J., and Zborowski, M. (2003). Magnetic cell separation: characterization of magnetophoretic mobility. Anal. Chem. 75, 6868–6874. doi: 10.1021/ac034315j
McHugh, C. A., Fontana, J., Nemecek, D., Cheng, N., Aksyuk, A. A., Heymann, J. B., et al. (2014). A virus capsid-like nanocompartment that stores iron and protects bacteria from oxidative stress. EMBO J. 33, 1896–1911. doi: 10.15252/embj.201488566
Meldrum, F., Heywood, B., and Mann, S. (1992). Magnetoferritin: in vitro synthesis of a novel magnetic protein. Science 257, 522–523. doi: 10.1126/science.1636086
Melville, D., Paul, F., and Roath, S. (1975). Direct magnetic separation of red cells from whole blood. Nature 255:706. doi: 10.1038/255706a0
Michel, F. M., Barrón, V., Torrent, J., Morales, M. P., Serna, C. J., Boily, J.-F., et al. (2010a). Ordered ferrimagnetic form of ferrihydrite reveals links among structure, composition, and magnetism. Proc. Natl. Acad. Sci. U.S.A. 107, 2787–2792. doi: 10.1073/pnas.0910170107
Michel, F. M., Hosein, H.-A., Hausner, D. B., Debnath, S., Parise, J. B., and Strongin, D. R. (2010b). Reactivity of ferritin and the structure of ferritin-derived ferrihydrite. Biochim. Biophys. Acta 1800, 871–885. doi: 10.1016/j.bbagen.2010.05.007
Michel, F. M., Ehm, L., Antao, S. M., Lee, P. L., Chupas, P. J., Liu, G., et al. (2007). The structure of ferrihydrite, a nanocrystalline material. Science 316, 1726–1729. doi: 10.1126/science.1142525
Mohammed, L., Gomaa, H. G., Ragab, D., and Zhu, J. (2017). Magnetic nanoparticles for environmental and biomedical applications: a review. Particuology 30, 1–14. doi: 10.1016/j.partic.2016.06.001
Morillo, V., Abreu, F., Araujo, A. C., de Almeida, L. G. P., Enrich-Prast, A., Farina, M., et al. (2014). Isolation, cultivation and genomic analysis of magnetosome biomineralization genes of a new genus of South-seeking magnetotactic cocci within the Alphaproteobacteria. Front. Microbiol. 5:72. doi: 10.3389/fmicb.2014.00072
Mos, Y. M., Vermeulen, A. C., Buisman, C. J. N., and Weijma, J. (2018). X-ray diffraction of iron containing samples: the importance of a suitable configuration. Geomicrobiol. J. 35, 511–517. doi: 10.1080/01490451.2017.1401183
Müller, F. D., Schüler, D., and Pfeiffer, D. (2020). A compass to boost navigation: cell biology of bacterial magnetotaxis. J. Bacteriol. doi: 10.1128/JB.00398-20
Muxworthy, A. R., and Williams, W. (2009). Critical superparamagnetic/single-domain grain sizes in interacting magnetite particles: implications for magnetosome crystals. J. R. Soc. Interface 6, 1207–1212. doi: 10.1098/rsif.2008.0462
Nakamura, C., Burgess, J. G., Sode, K., and Matsunaga, T. (1995). An iron-regulated gene, magA, encoding an iron transport protein of Magnetospirillum sp. strain AMB-1. J. Biol. Chem. 270, 28392–28396. doi: 10.1074/jbc.270.47.28392
Naumova, A. V., and Vande Velde, G. (2018). Genetically encoded iron-associated proteins as MRI reporters for molecular and cellular imaging. Wiley Interdiscip. Rev. Nanomed. Nanobiotechnol. 10:e1482. doi: 10.1002/wnan.1482
Nguyen, H. V., Suzuki, E., Oestreicher, Z., Minamide, H., Endoh, H., Fukumori, Y., et al. (2016). A protein-protein interaction in magnetosomes: TPR protein MamA interacts with an Mms6 protein. Biochem. Biophys. Rep. 7, 39–44. doi: 10.1016/j.bbrep.2016.05.010
Nichols, R. J., Cassidy-Amstutz, C., Chaijarasphong, T., and Savage, D. F. (2017). Encapsulins: molecular biology of the shell. Crit. Rev. Biochem. Mol. Biol. 52, 583–594. doi: 10.1080/10409238.2017.1337709
Nies, D. H. (2011). How iron is transported into magnetosomes. Mol. Microbiol. 82, 792–796. doi: 10.1111/j.1365-2958.2011.07864.x
Nimpf, S., and Keays, D. A. (2017). Is magnetogenetics the new optogenetics? EMBO J. 36, 1643–1646. doi: 10.15252/embj.201797177
Nisticò, R., Cesano, F., and Garello, F. (2020). Magnetic materials and systems: domain structure visualization and other characterization techniques for the application in the materials science and biomedicine. Inorganics 8:6. doi: 10.3390/inorganics8010006
Nudelman, H., Perez Gonzalez, T., Kolushiva, S., Widdrat, M., Reichel, V., Peigneux, A., et al. (2018). The importance of the helical structure of a MamC-derived magnetite-interacting peptide for its function in magnetite formation. Acta Crystallogr. D Struct. Biol. 74, 10–20. doi: 10.1107/S2059798317017491
Nudelman, H., Valverde-Tercedor, C., Kolusheva, S., Perez Gonzalez, T., Widdrat, M., Grimberg, N., et al. (2016). Structure-function studies of the magnetite-biomineralizing magnetosome-associated protein MamC. J. Struct. Biol. 194, 244–252. doi: 10.1016/j.jsb.2016.03.001
Nudelman, H., and Zarivach, R. (2014). Structure prediction of magnetosome-associated proteins. Front. Microbiol. 5:9. doi: 10.3389/fmicb.2014.00009
O’Handley, R. C. (2000). Modern Magnetic Materials: Principles and Applications. New York, NY: John Wiley.
Pang, K., You, H., Chen, Y., Chu, P., Hu, M., Shen, J., et al. (2017). MagR alone is insufficient to confer cellular calcium responses to magnetic stimulation. Front. Neural Circuits 11:11. doi: 10.3389/fncir.2017.00011
Peigneux, A., Jabalera, Y., Vivas, M. A. F., Casares, S., Azuaga, A. I., and Jimenez-Lopez, C. (2019). Tuning properties of biomimetic magnetic nanoparticles by combining magnetosome associated proteins. Sci. Rep. 9:8804.
Peigneux, A., Valverde-Tercedor, C., López-Moreno, R., Pérez-González, T., Fernández-Vivas, M. A., and Jiménez-López, C. (2016). Learning from magnetotactic bacteria: a review on the synthesis of biomimetic nanoparticles mediated by magnetosome-associated proteins. J. Struct. Biol. 196, 75–84. doi: 10.1016/j.jsb.2016.06.026
Pereira, S. M., Williams, S. R., Murray, P., and Taylor, A. (2016). MS-1 magA: revisiting its efficacy as a reporter gene for MRI. Mol. Imaging 15:1536012116641533. doi: 10.1177/1536012116641533
Pfeiffer, D., and Schüler, D. (2020). Quantifying the benefit of a dedicated “Magnetoskeleton” in bacterial magnetotaxis by live-cell motility tracking and soft agar swimming assay. Appl. Environ. Microbiol. 86:e01976-19.
Plouffe, B. D., Murthy, S. K., and Lewis, L. H. (2015). Fundamentals and application of magnetic particles in cell isolation and enrichment: a review. Rep. Prog. Phys. 78:016601. doi: 10.1088/0034-4885/78/1/016601
Pósfai, M., Lefèvre, C., Trubitsyn, D., Bazylinski, D., and Frankel, R. (2013). Phylogenetic significance of composition and crystal morphology of magnetosome minerals. Front. Microbiol. 4:344. doi: 10.3389/fmicb.2013.00344
Potenza, L., Ubaldi, L., De Sanctis, R., De Bellis, R., Cucchiarini, L., and Dachà, M. (2004). Effects of a static magnetic field on cell growth and gene expression in Escherichia coli. Mutat. Res. Genet. Toxicol. Environ. Mutagen. 561, 53–62. doi: 10.1016/j.mrgentox.2004.03.009
Price, P. M., Mahmoud, W. E., Al-Ghamdi, A. A., and Bronstein, L. M. (2018). Magnetic drug delivery: where the field is going. Front. Chem. 6:619. doi: 10.3389/fchem.2018.00619
Prozorov, T., Mallapragada, S. K., Narasimhan, B., Wang, L., Palo, P., Nilsen-Hamilton, M., et al. (2007). Protein-mediated synthesis of uniform superparamagnetic magnetite nanocrystals. Adv. Funct. Mater. 17, 951–957. doi: 10.1002/adfm.200600448
Qin, S., Yin, H., Yang, C., Dou, Y., Liu, Z., Zhang, P., et al. (2016). A magnetic protein biocompass. Nat. Mater. 15, 217–226. doi: 10.1038/nmat4484
Radoul, M., Lewin, L., Cohen, B., Oren, R., Popov, S., Davidov, G., et al. (2016). Genetic manipulation of iron biomineralization enhances MR relaxivity in a ferritin-M6A chimeric complex. Sci. Rep. 6:26550. doi: 10.1038/srep26550
Raschdorf, O., Bonn, F., Zeytuni, N., Zarivach, R., Becher, D., and Schüler, D. (2018). A quantitative assessment of the membrane-integral sub-proteome of a bacterial magnetic organelle. J. Proteomics 172, 89–99. doi: 10.1016/j.jprot.2017.10.007
Rawlings, A. E. (2016). Membrane proteins: always an insoluble problem? Biochem. Soc. Trans. 44, 790–795. doi: 10.1042/BST20160025
Rawlings, A. E., Bramble, J. P., Tang, A. A. S., Somner, L. A., Monnington, A. E., Cooke, D. J., et al. (2015). Phage display selected magnetite interacting Adhirons for shape controlled nanoparticle synthesis. Chem. Sci. 6, 5586–5594. doi: 10.1039/C5SC01472G
Rawlings, A. E., Bramble, J. P., Walker, R., Bain, J., Galloway, J. M., and Staniland, S. S. (2014). Self-assembled MmsF proteinosomes control magnetite nanoparticle formation in vitro. Proc. Natl. Acad. Sci. U.S.A. 111, 16094–16099. doi: 10.1073/pnas.1409256111
Rawlings, A. E., Somner, L. A., Fitzpatrick-Milton, M., Roebuck, T. P., Gwyn, C., Liravi, P., et al. (2019). Artificial coiled coil biomineralisation protein for the synthesis of magnetic nanoparticles. Nat. Commun. 10:2873.
Reufer, M., Besseling, R., Schwarz-Linek, J., Martinez, V. A., Morozov, A. N., Arlt, J., et al. (2014). Switching of swimming modes in Magnetospirillium gryphiswaldense. Biophys. J. 106, 37–46. doi: 10.1016/j.bpj.2013.10.038
Roberts, A. P., Chang, L., Rowan, C. J., Horng, C.-S., and Florindo, F. (2011). Magnetic properties of sedimentary greigite (Fe3S4): an update. Rev. Geophys. 49:RG1002. doi: 10.1029/2010rg000336
Rohani, R., Figueredo, R., Bureau, Y., Koropatnick, J., Foster, P., Thompson, R. T., et al. (2014). Imaging tumor growth non-invasively using expression of MagA or modified ferritin subunits to augment intracellular contrast for repetitive MRI. Mol. Imaging Biol. 16, 63–73. doi: 10.1007/s11307-013-0661-8
Romeo, S., Sannino, A., Scarfì, M. R., Massa, R., d’Angelo, R., and Zeni, O. (2016). Lack of effects on key cellular parameters of MRC-5 human lung fibroblasts exposed to 370 mT static magnetic field. Sci. Rep. 6:19398. doi: 10.1038/srep19398
Runge, V. M. (2017). Critical questions regarding gadolinium deposition in the brain and body after injections of the gadolinium-based contrast agents, safety, and clinical recommendations in consideration of the EMA’s pharmacovigilance and risk assessment committee recommendation for suspension of the marketing authorizations for 4 linear agents. Invest. Radiol. 52, 317–323. doi: 10.1097/rli.0000000000000374
Sahebjamei, H., Abdolmaleki, P., and Ghanati, F. (2007). Effects of magnetic field on the antioxidant enzyme activities of suspension-cultured tobacco cells. Bioelectromagnetics 28, 42–47. doi: 10.1002/bem.20262
Santambrogio, P., Levi, S., Arosio, P., Palagi, L., Vecchio, G., Lawson, D. M., et al. (1992). Evidence that a salt bridge in the light chain contributes to the physical stability difference between heavy and light human ferritins. J. Biol. Chem. 267, 14077–14083.
Sawicki, M., Stefanowicz, W., and Ney, A. (2011). Sensitive SQUID magnetometry for studying nanomagnetism. Semicond. Sci. Technol. 26:064006. doi: 10.1088/0268-1242/26/6/064006
Scheffel, A., Gärdes, A., Grünberg, K., Wanner, G., and Schüler, D. (2008). The major magnetosome proteins MamGFDC are not essential for magnetite biomineralization in Magnetospirillum gryphiswaldense but regulate the size of magnetosome crystals. J. Bacteriol. 190, 377–386. doi: 10.1128/jb.01371-07
Scheffel, A., Gruska, M., Faivre, D., Linaroudis, A., Plitzko, J. M., and Schüler, D. (2006). An acidic protein aligns magnetosomes along a filamentous structure in magnetotactic bacteria. Nature 440, 110–114. doi: 10.1038/nature04382
Schübbe, S., Kube, M., Scheffel, A., Wawer, C., Heyen, U., Meyerdierks, A., et al. (2003). Characterization of a spontaneous nonmagnetic mutant of Magnetospirillum gryphiswaldense reveals a large deletion comprising a putative magnetosome island. J. Bacteriol. 185, 5779–5790. doi: 10.1128/jb.185.19.5779-5790.2003
Sengupta, A., Quiaoit, K., Thompson, R. T., Prato, F. S., Gelman, N., and Goldhawk, D. E. (2014). Biophysical features of MagA expression in mammalian cells: implications for MRI contrast. Front. Microbiol. 5:29. doi: 10.3389/fmicb.2014.00029
Sigmund, F., Massner, C., Erdmann, P., Stelzl, A., Rolbieski, H., Desai, M., et al. (2018). Bacterial encapsulins as orthogonal compartments for mammalian cell engineering. Nat. Commun. 9:1990.
Silva, K. T., Leao, P. E., Abreu, F., Lopez, J. A., Gutarra, M. L., Farina, M., et al. (2013). Optimization of magnetosome production and growth by the magnetotactic vibrio Magnetovibrio blakemorei strain MV-1 through a statistics-based experimental design. Appl. Environ. Microbiol. 79, 2823–2827. doi: 10.1128/aem.03740-12
Singh, P., Kim, Y.-J., Zhang, D., and Yang, D.-C. (2016). Biological synthesis of nanoparticles from plants and microorganisms. Trends Biotechnol. 34, 588–599. doi: 10.1016/j.tibtech.2016.02.006
Singh, S., and Varma, A. (2017). “Structure, function, and estimation of leghemoglobin,” in Rhizobium Biology and Biotechnology, eds A. P. Hansen, D. K. Choudhary, P. K. Agrawal, and A. Varma (Cham: Springer International Publishing), 309–330. doi: 10.1007/978-3-319-64982-5_15
Sofla, A., Cirkovic, B., Hsieh, A., Miklas, J. W., Filipovic, N., and Radisic, M. (2013). Enrichment of live unlabelled cardiomyocytes from heterogeneous cell populations using manipulation of cell settling velocity by magnetic field. Biomicrofluidics 7:14110. doi: 10.1063/1.4791649
Sorensen, C. M. (2001). “Magnetism,” in Nanoscale Materials in Chemistry, ed. K. J. Klabunde (Hoboken, NJ: John Wiley & Sons, Inc).
Spees, W. M., Yablonskiy, D. A., Oswood, M. C., and Ackerman, J. J. H. (2001). Water proton MR properties of human blood at 1.5 Tesla: magnetic susceptibility, T1, T2, T, and non-Lorentzian signal behavior. Magn. Reson. Med. 45, 533–542. doi: 10.1002/mrm.1072
Springer, B. A., and Sligar, S. G. (1987). High-level expression of sperm whale myoglobin in Escherichia coli. Proc. Natl. Acad. Sci. U.S.A. 84, 8961–8965. doi: 10.1073/pnas.84.24.8961
Staniland, S. S., and Rawlings, A. E. (2016). Crystallizing the function of the magnetosome membrane mineralization protein Mms6. Biochem. Soc. Trans. 44, 883–890. doi: 10.1042/BST20160057
Sutter, M., Boehringer, D., Gutmann, S., Günther, S., Prangishvili, D., Loessner, M. J., et al. (2008). Structural basis of enzyme encapsulation into a bacterial nanocompartment. Nat. Struct. Mol. Biol. 15, 939–947. doi: 10.1038/nsmb.1473
Ta, D. T., and Vickery, L. E. (1992). Cloning, sequencing, and overexpression of a [2Fe-2S] ferredoxin gene from Escherichia coli. J. Biol. Chem. 267, 11120–11125.
Tay, A., Pfeiffer, D., Rowe, K., Tannenbaum, A., Popp, F., Strangeway, R., et al. (2018). High-throughput microfluidic sorting of live magnetotactic bacteria. Appl. Environ. Microbiol. 84:e01308-18.
Theil, E. C. (1987). Ferritin: structure, gene regulation, and cellular function in animals, plants, and microorganisms. Annu. Rev. Biochem. 56, 289–315. doi: 10.1146/annurev.bi.56.070187.001445
Theil, E. C. (2012). Ferritin protein nanocages-the story. Nanotechnol. Percept. 8, 7–16. doi: 10.4024/n03th12a.ntp.08.01
Tiede, C., Tang, A. A., Deacon, S. E., Mandal, U., Nettleship, J. E., Owen, R. L., et al. (2014). Adhiron: a stable and versatile peptide display scaffold for molecular recognition applications. Protein Eng. Des. Sel. 27, 145–155. doi: 10.1093/protein/gzu007
Toner, M., and Irimia, D. (2005). Blood-on-a-chip. Annu. Rev. Biomed. Eng. 7, 77–103. doi: 10.1146/annurev.bioeng.7.011205.135108
Toro-Nahuelpan, M., Giacomelli, G., Raschdorf, O., Borg, S., Plitzko, J. M., Bramkamp, M., et al. (2019). MamY is a membrane-bound protein that aligns magnetosomes and the motility axis of helical magnetotactic bacteria. Nat. Microbiol. 4, 1978–1989. doi: 10.1038/s41564-019-0512-8
Ubago-Rodríguez, A., Casares Atienza, S., Fernández-Vivas, A., Peigneux, A., Jabalera, Y., de la Cuesta-Rivero, M., et al. (2019). Structure–function of MamC loop and its effect on the in vitro precipitation of biomimetic magnetite nanoparticles. Cryst. Growth Des. 19, 2927–2935. doi: 10.1021/acs.cgd.9b00150
Uchida, M., Flenniken, M. L., Allen, M., Willits, D. A., Crowley, B. E., Brumfield, S., et al. (2006). Targeting of cancer cells with ferrimagnetic ferritin cage nanoparticles. J. Am. Chem. Soc. 128, 16626–16633. doi: 10.1021/ja0655690
Uebe, R., Henn, V., and Schüler, D. (2012). The MagA protein of magnetospirilla is not involved in bacterial magnetite biomineralization. J. Bacteriol. 194, 1018–1023. doi: 10.1128/jb.06356-11
Uebe, R., Keren-Khadmy, N., Zeytuni, N., Katzmann, E., Navon, Y., Davidov, G., et al. (2018). The dual role of MamB in magnetosome membrane assembly and magnetite biomineralization. Mol. Microbiol. 107, 542–557. doi: 10.1111/mmi.13899
Uebe, R., and Schüler, D. (2016). Magnetosome biogenesis in magnetotactic bacteria. Nat. Rev. Microbiol. 14, 621–637. doi: 10.1038/nrmicro.2016.99
Valverde-Tercedor, C., Montalbán-López, M., Perez-Gonzalez, T., Sanchez-Quesada, M. S., Prozorov, T., Pineda-Molina, E., et al. (2015). Size control of in vitro synthesized magnetite crystals by the MamC protein of Magnetococcus marinus strain MC-1. Appl. Microbiol. Biotechnol. 99, 5109–5121. doi: 10.1007/s00253-014-6326-y
Van de Walle, A., Perez, J. E., Abou-Hassan, A., Hémadi, M., Luciani, N., and Wilhelm, C. (2020). Magnetic nanoparticles in regenerative medicine: what of their fate and impact in stem cells? Mater. Today Nano 11:100084. doi: 10.1016/j.mtnano.2020.100084
Van de Walle, A., Plan Sangnier, A., Abou-Hassan, A., Curcio, A., Hémadi, M., Menguy, N., et al. (2019). Biosynthesis of magnetic nanoparticles from nano-degradation products revealed in human stem cells. Proc. Natl. Acad. Sci. U.S.A. 116, 4044–4053. doi: 10.1073/pnas.1816792116
Vargas, G., Cypriano, J., Correa, T., Leão, P., Bazylinski, D. A., and Abreu, F. (2018). Applications of magnetotactic bacteria, magnetosomes and magnetosome crystals in biotechnology and nanotechnology: mini-review. Molecules 23:2438. doi: 10.3390/molecules23102438
Vergallo, C., Ahmadi, M., Mobasheri, H., and Dini, L. (2014). Impact of inhomogeneous static magnetic field (31.7–232.0 mT) exposure on human neuroblastoma SH-SY5Y cells during cisplatin administration. PLoS One 9:e113530. doi: 10.1371/journal.pone.0113530
Walls, M. G., Cao, C., Yu-Zhang, K., Li, J., Che, R., and Pan, Y. (2013). Identification of ferrous-ferric Fe3O4 nanoparticles in recombinant human ferritin cages. Microsc. Microanal. 19, 835–841. doi: 10.1017/S1431927613001724
Wang, G., Zhang, P., Mendu, S. K., Wang, Y., Zhang, Y., Kang, X., et al. (2019). Revaluation of magnetic properties of Magneto. Nat. Neurosci. 23, 1047–1050. doi: 10.1038/s41593-019-0473-5
Wang, L., Prozorov, T., Palo, P. E., Liu, X., Vaknin, D., Prozorov, R., et al. (2012). Self-assembly and biphasic iron-binding characteristics of Mms6, a bacterial protein that promotes the formation of superparamagnetic magnetite nanoparticles of uniform size and shape. Biomacromolecules 13, 98–105. doi: 10.1021/bm201278u
Wang, L., Xu, H., Liu, Z., Sun, T., Yuan, C., Yang, Y., et al. (2019). Magnetic immobilization of a quorum sensing signal hydrolase, AiiA. Microbiologyopen 8:e00797. doi: 10.1002/mbo3.797
Wang, Z., Yang, P., Xu, H., Qian, A., Hu, L., and Shang, P. (2009). Inhibitory effects of a gradient static magnetic field on normal angiogenesis. Bioelectromagnetics 30, 446–453. doi: 10.1002/bem.20501
Weed, R. I., Reed, C. F., and Berg, G. (1963). Is hemoglobin an essential structural component of human erythrocyte membranes? J. Clin. Invest. 42, 581–588. doi: 10.1172/JCI104747
Whitesides, G. M., Kazlauskas, R. J., and Josephson, L. (1983). Magnetic separations in biotechnology. Trends Biotechnol. 1, 144–148. doi: 10.1016/0167-7799(83)90005-7
Williams, T. J., Lefèvre, C. T., Zhao, W., Beveridge, T. J., and Bazylinski, D. A. (2012). Magnetospira thiophila gen. nov., sp. nov., a marine magnetotactic bacterium that represents a novel lineage within the Rhodospirillaceae (Alphaproteobacteria). Int. J. Syst. Evol. Microbiol. 62, 2443–2450. doi: 10.1099/ijs.0.037697-0
Winklhofer, M., and Mouritsen, H. (2016). A room-temperature ferrimagnet made of metallo-proteins? bioRxiv [Preprint]. doi: 10.1101/094607
Wong, K. K., Douglas, T., Gider, S., Awschalom, D. D., and Mann, S. (1998). Biomimetic synthesis and characterization of magnetic proteins (magnetoferritin). Chem. Mater. 10, 279–285. doi: 10.1021/cm970421o
Wu, C., Shen, Y., Chen, M., Wang, K., Li, Y., and Cheng, Y. (2018). Recent advances in magnetic-nanomaterial-based mechanotransduction for cell fate regulation. Adv. Mater. 30:e1705673. doi: 10.1002/adma.201705673
Wu, K., Su, D., Liu, J., Saha, R., and Wang, J.-P. (2019). Magnetic nanoparticles in nanomedicine: a review of recent advances. Nanotechnology 30:502003. doi: 10.1088/1361-6528/ab4241
Wu, L., Zhang, F., Wei, Z., Li, X., Zhao, H., Lv, H., et al. (2018). Magnetic delivery of Fe3O4@polydopamine nanoparticle-loaded natural killer cells suggest a promising anticancer treatment. Biomater. Sci. 6, 2714–2725. doi: 10.1039/C8BM00588E
Wu, W. J., Liu, S. L., and Yung, P. T. (2017). Effect of static magnetic field on endospore germination. Bioelectromagnetics 38, 121–127. doi: 10.1002/bem.22017
Yamada, M., Shimizu, M., Katafuchi, A., Grúz, P., Fujii, S., Usui, Y., et al. (2012). Escherichia coli DNA polymerase III is responsible for the high level of spontaneous mutations in mutT strains. Mol. Microbiol. 86, 1364–1375. doi: 10.1111/mmi.12061
Yamamoto, D., Taoka, A., Uchihashi, T., Sasaki, H., Watanabe, H., Ando, T., et al. (2010). Visualization and structural analysis of the bacterial magnetic organelle magnetosome using atomic force microscopy. Proc. Natl. Acad. Sci. U.S.A. 107, 9382–9387. doi: 10.1073/pnas.1001870107
Yang, J., Li, S., Huang, X., Tang, T., Jiang, W., Zhang, T., et al. (2013). A key time point for cell growth and magnetosome synthesis of Magnetospirillum gryphiswaldense based on real-time analysis of physiological factors. Front. Microbiol. 4:210. doi: 10.3389/fmicb.2013.00210
Zablotskii, V., Lunov, O., Kubinova, S., Polyakova, T., Sykova, E., and Dejneka, A. (2016a). Effects of high-gradient magnetic fields on living cell machinery. J. Phys. D Appl. Phys. 49:493003. doi: 10.1088/0022-3727/49/49/493003
Zablotskii, V., Polyakova, T., Lunov, O., and Dejneka, A. (2016b). How a high-gradient magnetic field could affect cell life. Sci. Rep. 6:37407. doi: 10.1038/srep37407
Zahn, C., Keller, S., Toro-Nahuelpan, M., Dorscht, P., Gross, W., Laumann, M., et al. (2017). Measurement of the magnetic moment of single Magnetospirillum gryphiswaldense cells by magnetic tweezers. Sci. Rep. 7:3558. doi: 10.1038/s41598-017-03756-z
Zborowski, M., and Chalmers, J. J. (2011). Rare cell separation and analysis by magnetic sorting. Anal. Chem. 83, 8050–8056. doi: 10.1021/ac200550d
Zeytuni, N., Ozyamak, E., Ben-Harush, K., Davidov, G., Levin, M., Gat, Y., et al. (2011). Self-recognition mechanism of MamA, a magnetosome-associated TPR-containing protein, promotes complex assembly. Proc. Natl. Acad. Sci. U.S.A. 108, E480–E487. doi: 10.1073/pnas.1103367108
Zhang, H., Liu, X., Feng, S., Wang, W., Schmidt-Rohr, K., Akinc, M., et al. (2015). Morphological transformations in the magnetite biomineralizing protein Mms6 in iron solutions: a small-angle X-ray scattering study. Langmuir 31, 2818–2825. doi: 10.1021/la5044377
Zhang, X. Y., Robledo, B. N., Harris, S. S., and Hu, X. P. (2014). A bacterial gene, mms6, as a new reporter gene for magnetic resonance imaging of mammalian cells. Mol. Imaging 13, 1–12. doi: 10.2310/7290.2014.00046
Zhang, Y., Zhang, X., Jiang, W., Li, Y., and Li, J. (2011). Semicontinuous culture of Magnetospirillum gryphiswaldense MSR-1 cells in an autofermentor by nutrient-balanced and isosmotic feeding strategies. Appl. Environ. Microbiol. 77, 5851–5856. doi: 10.1128/aem.05962-11
Zhou, C. Z., Confalonieri, F., Jacquet, M., Perasso, R., Li, Z. G., and Janin, J. (2001). Silk fibroin: structural implications of a remarkable amino acid sequence. Proteins 44, 119–122. doi: 10.1002/prot.1078
Zhu, K., Pan, H., Li, J., Yu-Zhang, K., Zhang, S.-D., Zhang, W.-Y., et al. (2010). Isolation and characterization of a marine magnetotactic spirillum axenic culture QH-2 from an intertidal zone of the China Sea. Res. Microbiol. 161, 276–283. doi: 10.1016/j.resmic.2010.02.003
Zimmerman, P. A., Thomson, J. M., Fujioka, H., Collins, W. E., and Zborowski, M. (2006). Diagnosis of malaria by magnetic deposition microscopy. Am. J. Trop. Med. Hyg. 74, 568–572. doi: 10.4269/ajtmh.2006.74.568
Keywords: intrinsic magnetism, magnetic cells, magnetotactic bacteria, magnetic protein, ferritin, encpasulin, continuous cultivation
Citation: Pekarsky A and Spadiut O (2020) Intrinsically Magnetic Cells: A Review on Their Natural Occurrence and Synthetic Generation. Front. Bioeng. Biotechnol. 8:573183. doi: 10.3389/fbioe.2020.573183
Received: 16 June 2020; Accepted: 29 September 2020;
Published: 19 October 2020.
Edited by:
Peter Neubauer, Technical University of Berlin, GermanyReviewed by:
Robert Kourist, Graz University of Technology, AustriaClaire Wilhelm, UMR 7057 Laboratoire Matière et Systèmes Complexes (MSC), France
Copyright © 2020 Pekarsky and Spadiut. This is an open-access article distributed under the terms of the Creative Commons Attribution License (CC BY). The use, distribution or reproduction in other forums is permitted, provided the original author(s) and the copyright owner(s) are credited and that the original publication in this journal is cited, in accordance with accepted academic practice. No use, distribution or reproduction is permitted which does not comply with these terms.
*Correspondence: Oliver Spadiut, b2xpdmVyLnNwYWRpdXRAdHV3aWVuLmFjLmF0