- Centre Armand-Frappier Santé Biotechnologie, Institut National de la Recherche Scientifique (INRS), Laval, QC, Canada
Rhamnolipids are surface-active agents of microbial origin used as alternatives to synthetic surfactants. Burkholderia thailandensis is a non-pathogenic rhamnolipid-producing bacterium that could represent an interesting candidate for use in commercial processes. However, current bioprocesses for rhamnolipid production by this bacterium are not efficient enough, mainly due to low yields. Since regulation of rhamnolipid biosynthesis in B. thailandensis remains poorly understood, identifying new regulatory factors could help increase the production of these valuable metabolites. We performed a random transposon mutagenesis screening to identify genes directing rhamnolipid production in B. thailandensis E264. The most efficient rhamnolipid producer we identified harbored an inactivating transposon insertion in the scmR gene, which was recently described to encode as a secondary metabolite regulator in B. thailandensis. We investigated the impact of scmR loss on rhamnolipid biosynthesis and cell growth. Because biosynthesis of rhamnolipids and polyhydroxyalkanoates (PHAs) could share the same pool of lipid precursors, we also investigate the effect of ScmR on PHA production. We found that production of both rhamnolipids and PHAs are modulated by ScmR during the logarithmic growth phase and demonstrate that ScmR downregulates the production of rhamnolipids by affecting the expression of both rhl biosynthetic operons. Furthermore, our results indicate that PHA biosynthesis is reduced in the scmR- mutant, as ScmR promotes the transcription of the phaC and phaZ genes. By studying the relationship between ScmR and quorum sensing (QS) regulation we reveal that QS acts as an activator of scmR transcription. Finally, we pinpoint the QS-3 system as being involved in the regulation of rhamnolipid and PHA biosynthesis. We conclude that ScmR negatively affects rhamnolipid production, whereas it positively impacts PHAs biosynthesis. This could provide an interesting approach for future strain engineering, leading to improved yields of these valuable metabolites.
Introduction
Rhamnolipids are biosurfactants that were first identified in Pseudomonas aeruginosa cultures (Jarvis and Johnson, 1949). The amphiphilic character of rhamnolipids is due to the presence of a hydrophilic polar head, typically containing one or two rhamnose moieties, and a hydrophobic apolar tail, composed of a dimer of two esterified β-hydroxy-fatty acids. This diverse group of compounds comprises more than 60 reported congeners (Abdel-Mawgoud et al., 2010). Variations in the sugar and in the hydrophobic tail lead to structural differences between congeners. Their tensioactive properties, low toxicity and high biodegradability make them suitable for a variety of applications with a low environmental impact (Lang and Wullbrandt, 1999; Banat et al., 2000; Nitschke et al., 2005).
Rhamnolipid biosynthesis has been extensively investigated and the opportunistic pathogen P. aeruginosa remains the principal producing bacterial species. However, we previously showed that Burkholderia thailandensis also naturally synthesizes rhamnolipids and might represent an interesting candidate for industrial scale production of this biosurfactant (Dubeau et al., 2009). Indeed, this bacterium is non-pathogenic and produces one main congener, facilitating downstream processing. B. thailandensis was also recently found to be a producer of polyhydroxyalkanoates (PHAs), another valuable metabolite (Funston et al., 2017; Kourmentza et al., 2018; Martinez and Déziel, 2020) and PHA biosynthesis was suggested to be in metabolic competition with rhamnolipid production (Funston et al., 2017). Production processes for both rhamnolipids and PHAs have not yet been optimized in B. thailandensis likely because little is known on the regulation. Two rhl operons are responsible for rhamnolipid biosynthesis in B. thailandensis (Dubeau et al., 2009). While the global level of transcription of rhl genes was studied for the temperature effect on the rhamnolipid production (Funston et al., 2016), the respective regulation of these two operons has yet to be thoroughly explored.
Quorum sensing (QS) is a global regulatory mechanism of gene expression depending on bacterial density (Fuqua et al., 1994). Briefly, LuxI type synthases are responsible for the production of N-acyl-L-homoserine lactones (AHLs), signaling molecules which accumulate in the environment during bacterial growth until a threshold concentration. Then, the LuxR type transcriptional regulators are activated by the binding of cognate AHLs, allowing the regulation of the expression of QS target genes including the gene encoding the AHL synthase, creating an autoinducing loop. This way, bacteria synchronize their activities and act as multicellular communities in order to perform social functions. In B. thailandensis, the QS systems are referred to as the BtaI1/BtaR1 (QS-1), BtaI2/BtaR2 (QS-2), and BtaI3/BtaR3 (QS-3) systems (Majerczyk et al., 2013), mainly associated with C8-HSL, 3OHC10-HSL, and 3OHC8-HSL, respectively (Chandler et al., 2009; Le Guillouzer et al., 2017). These systems are intricately intertwined and differently regulated depending on the growth phase (Le Guillouzer et al., 2017) and regulate cell auto-aggregation and production of secondary metabolites such as antibiotics (Chandler et al., 2009). In P. aeruginosa and in Burkholderia glumae, rhl biosynthesis genes are under the control of distinct quorum sensing (QS) systems (Soberón-Chávez et al., 2005; Majerczyk et al., 2014a; Nickzad and Déziel, 2016). Although a relationship between QS and the biosynthesis of rhamnolipids has been previously noted in B. thailandensis (Majerczyk et al., 2014b; Irorere et al., 2019), there is very limited information on how QS regulates rhamnolipid production.
The aim of this study was to better understand the regulation of rhamnolipid and PHA production in B. thailandensis using a mutagenesis and functional screening approach. In doing so, we identified ScmR as a modulator of the biosynthesis of these metabolites in B. thailandensis. Since this regulator was also reported to be QS-controlled and to impact the production of AHL signals (Majerczyk et al., 2014a, b; Mao et al., 2017; Le Guillouzer et al., 2020), we further investigated the co-modulation of the biosynthesis of PHA and rhamnolipids by QS and ScmR in B. thailandensis.
Materials and Methods
Strains and Plasmids
Strains used in this study are presented in Table 1.
Growth Conditions
Strains were routinely grown from frozen stocks at 37°C in tryptic soy broth (TSB) (BD Difco) in a TC-7 roller drum at 240 rpm (New Brunswick, Canada), or on TSB agar plates. For rhamnolipid production, nutrient broth (NB) medium (BD Difco) supplemented with 2 or 4% (w/v) glycerol was used (Dubeau et al., 2009). When necessary, antibiotics were used at the following concentrations: 10 μg ml–1 tetracycline (Tc) and 100 μg ml–1 trimethoprim (Tmp) for B. thailandensis and 15 μg ml–1 tetracycline (Tc), 100 μg ml–1 carbenicillin (Cb), and 100 μg ml–1 trimethoprim (Tmp) for E. coli. All experiments were performed in triplicate and conducted at least twice independently. For blue/white screening, 5-bromo-4-chloro-3-indolyl-β-D-galactopyranoside (X-gal) was added in LB plates for final concentration 40 μg ml–1. For experiments with AHL complementation, 2 μM C8-HSL, 3OHC10-HSL, or 3OHC8-HSL (Sigma-Aldrich Co., Oakville, ON, Canada) were added to cultures. AHLs stocks were prepared in HPLC-grade acetonitrile. Acetonitrile only was added to the controls.
Random Mutagenesis
A library of B. thailandensis transconjugants was generated as follows. Plasmid pIT2 carrying the ISlacZ/hah transposon was transferred in B. thailandensis E264 by conjugation with E. coli χ7213 (asd-) strain (Kang et al., 2002; Jacobs et al., 2003). NB agar supplemented with 4% glycerol and tetracycline was used for selection of transposants. The screening for rhamnolipid production was achieved using atomized mineral oil spraying (Burch et al., 2010), with a few modifications. Sudan Red dye (0.5%) was added to mineral oil to provide a better contrast. The presence of a halo surrounding colonies indicates the production of rhamnolipids caused by the amphiphilic properties of surfactants; the diameter of halos around colonies was measured and compared to a WT control. Clones with larger halos were selected as potential candidates for enhanced rhamnolipid production.
Identification of Transposon Insertion Sites by Sequencing (Tn-seq)
Total DNA was extracted from bacterial cultures using a mechanical lysis method, as previously described (Durand et al., 2015). DNA concentrations were estimated using the Quant-iTTM PicoGreen® dsDNA Assay Kit (Invitrogen, Life Technologies, Burlington, ON, Canada) following the instructions of the manufacturer. Total genomic DNA from the selected mutants were pooled together and sent to the McGill University and Génome Québec Innovation Centre for transposon insertion sequencing (MiSeq Illumina). Generated Tn-Seq reads were analyzed as follows: sequences were trimmed in order to remove the 3′ bases from the adaptor used for sequencing; only the 4 last bases from the cassette were conserved: TCAG. All the resulting sequences were alphabetically sorted and clustered. For each cluster, a unique consensus sequence was determined and sequence alignments with B. thailandensis E264 genome were performed on www.burkholderia.com, allowing the identification of the insertion site.
Construction of Plasmids
For pMCG11 construction, the dhfrII gene, encoding for a dihydrofolate reductase conferring resistance to trimethoprim, was amplified by PCR from pGP704N-dfr using primers dhfrFPstI (5′-AAAACTGCAGATATCTG AGCTGTTGACAATTAATCATCC-3′) and dhfrRPstI (5′-A AAACTGCAGCCACCAAACTTAGTTGATGCGTTCAAGCG-3′) and cloned inside the PstI site in the pTZ110 vector. The construct was transformed in E. coli DH5α and trimethoprim was used for selection.
Plasmids containing lacZ reporters to evaluate the transcription of both rhl operons were constructed. First, for pAH1 and pAH2 constructions, the two promoter regions and the first 102 pb of BTH_II1081 (rhlA1) and BTH_II1875 (rhlA2) genes were amplified by PCR using forward primers Thai-UpOp1-F (5′-GGAATTCCCCGAAGGATATCGGTTTTT-3′) for rhlA1 and Thai-UpOp2-F (5′-CCGGAATTCCGCATTC ACCACAATGGA-3′) for rhlA2 respectively, and the reverse primer Thai-UpOp-R (5′-CGGGATCCGTTCACGA GGATGACCGTCT-3′). The PCR products were cloned in the pGEM®-T Easy (Promega) to generate vectors pAH1 and pAH2. DH5α cells were transformed and positive clones were selected on LB plate containing carbenicillin and X-gal. The pAH1 and pAH2 plasmids were digested by BamH1 (NEB) and Ecl136II (Thermo Fisher Scientific) and the 1081 bp and 847 bp fragments respectively were ligated in StuI (NEB) and BamHI (NEB)-digested pMCG11 to generate pAH5 et pAH8. The two reporters were independently transferred either into B. thailandensis wild type (WT) or in the scmR mutant by electroporation (Dennis and Sokol, 1995).
β-Galactosidase Activity Assays
β-galactosidase assays were performed as described (Miller, 1972) with some modifications. Normalization of the activity was calculated using colony-forming units (CFU)/mL instead of OD600.
Rhamnolipid Quantification by Liquid Chromatography/Mass Spectrometry (LC/MS)
Rhamnolipid concentrations in cultures were determined by liquid chromatography coupled to tandem mass spectrometry, as we previously described (Dubeau et al., 2009), with some modifications. After six days of growth, 1 mL culture samples were retrieved and the OD600 was measured (Nanodrop ND-1000, Thermo Fisher Scientific). The samples were centrifuged at 16,800 × g for 10 min to remove the bacteria. A 500 μl sample of supernatant was transferred to an HPLC vial and 500 μl methanol containing 10 mg/L 5,6,7,8-tetradeutero-4-hydroxy-2-heptylquinoline (HHQ-d4) as the internal standard were added. The samples were then analyzed by high-performance liquid chromatography (HPLC; Waters 2795, Mississauga, ON, Canada) equipped with a C8 reverse-phase column (EVO, Phenomenex) using a water/acetonitrile gradient with a constant 2 mmol/L concentration of ammonium acetate (Dubeau et al., 2009). The detector was a tandem quadrupole mass spectrometer (Quattro Premier XE, Waters). Analyses were carried out in the negative electrospray ionization (ESI-) mode.
Quantification of Polyhydroxyalkanoates
Polyhydroxyalkanoates concentrations were estimated as described previously (Martinez and Déziel, 2020). Briefly, culture samples were collected and centrifuged during 3 min at 16,800 × g. Supernatants were discarded, and pellets were suspended in water. Samples were heated at 100°C for 10 min and immediately transferred on ice for 5 min. The samples were then centrifuged for 3 min at 16,800 × g and the pellets were suspended in water and transferred to a 96-well microtiter plate. An equal volume of a 0.02% (w/v) Nile Blue (Sigma Aldrich) solution was added to each well. After 4 min of incubation, the intensity of fluorescence was determined using a Cytation 3 multimode plate reader (Biotek), using excitation and emission wavelengths of 490 and 590 nm, respectively.
Transmission Electron Microscopy (TEM) for Visualization of PHA Granules
Sample preparation and staining were performed as previously described (Martinez and Déziel, 2020). Ultrathin sections (70−100 nm thick) were examined with a Hitachi H-7100 electron microscope with an accelerating voltage of 75 kV.
Quantitative Reverse-Transcription Polymerase Chain Reaction (qRT-PCR)
Extraction of total RNA from cultures of B. thailandensis E264 and btai123, btaI1, btaI2, btaI3 mutants at 24, 72, and 96 h, cDNA synthesis and amplification were done as before (Le Guillouzer et al., 2020). The scmR gene was amplified using primers SLG_qRThmqR_F (5′-CTTCGTATGTGTTGCCGAAC-3′) and SLG_qRThmqR_R (5′-ATGAGACGCGTGTTCAGATG-3′). To evaluate the expression of genes implicated in PHA accumulation, total RNA was extracted from cultures of B. thailandensis E264, scmR mutants at 24 and 48 h and cDNA synthesis and amplification were performed as previously described. The phaC and phaZ genes, which respectively encode for the PHA synthase PhaC and the PHA depolymerase PhaZ were amplified with primers phaC_thai_fwd (5′-GATCTGCTGTACTGGAACG-3′), phaC_thai_rev (5′-AGCTTGTTCTCGAGATAGGT-3′), phaZ_BTH_I0973_fwd (5′-TCTCACTGGGACTTCTATCA-3′), and phaZ_BTH_I0973_rev (5′-TGTATTCGTCGTAG AAGCG-3′).
For all experiments, the reference gene was ndh, which encodes for an NADH dehydrogenase (Subsin et al., 2007). This gene displayed stable expression in the various genetic contexts (Supplementary Figure S1). The primers used were the same as in our previous studies SLG_qRT_ndh_F (5′-ACCAGGGCGAATTGATCTC-3′) and SLG_qRT_ndh_R (5′-GATGACGAGCGTGTCGTATT-3′) (Le Guillouzer et al., 2017, 2018, 2020). Differences in gene expression between B. thailandensis E264 strains were calculated using the 2−ΔΔCT formula (Livak and Schmittgen, 2001).
Results
Random Mutagenesis Reveals a Rhamnolipid Overproducer of B. thailandensis E264
In order to identify factors implicated in the regulation of the production of rhamnolipids in B. thailandensis, we performed a random transposon mutagenesis followed by a functional screening. We tested about 25,000 transposants of strain E264 for rhamnolipid production using an oil spraying method. Among the transposants selected, the best candidate for increased rhamnolipid production was isolate M63. It produced a halo of 22.89 ± 3.85 cm2, significantly bigger (t-test, p = 0.0224) than the area around a WT colony, at 6.3 ± 0.21 cm2 (Figure 1A). In order to validate the results obtained with the oil spraying method, rhamnolipid production for the M63 mutant was quantified in 5 days-old cultures prepared in NB broth with 2% glycerol. Our results confirmed that the M63 isolate produced three times more rhamnolipids than the WT (Figure 1B).
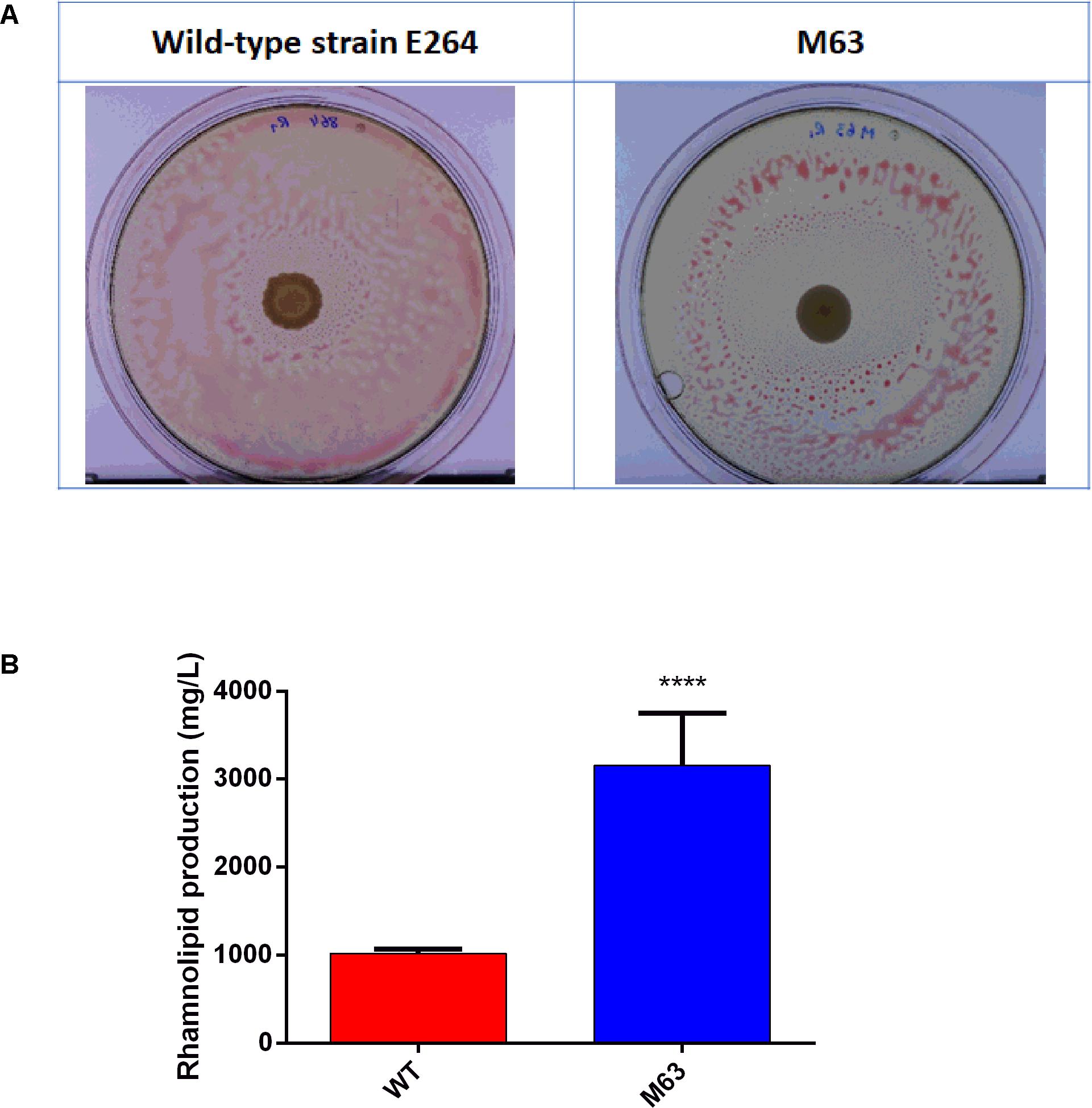
Figure 1. Random mutagenesis identifies a rhamnolipid overproducer of B. thailandensis E264. (A) Phenotypic selection by oil spraying, (B) Rhamnolipid quantification after 5 days of culture in NB + 2% glycerol. The error bars represent standard deviation from the mean (n = 3 independent cultures). Asterisk indicates statistically significant differences (*p < 0.05) in paired Student’s t tests.
A scmR- Mutant Overproduces Rhamnolipids
TnSeq analysis of M63 generated one unique sequence (Supplementary Table S1); this mutant has a transposon inserted in the BTH_I1403 locus, which encodes the LysR-type transcriptional regulator ScmR. Interestingly, this regulator influences the production of several secondary metabolites in B. thailandensis E264 (Mao et al., 2017; Le Guillouzer et al., 2020). To investigate the impact of this mutation further, we performed cultures of a previously characterized scmR- mutant (ED1023, Table 1; Le Guillouzer et al., 2020) in baffled flasks containing NB medium with 2% glycerol and samples were collected daily. Growth was measured by OD600 measurements and live-cell counts (CFU/mL) and rhamnolipid production was quantified by LC-MS analyses. As we observed under different culture conditions (Le Guillouzer et al., 2020), OD600 measurements suggested a growth defect for this mutant (Figure 2A). However, CFU counts (Figure 2B) confirmed that both the WT and the scmR- mutant actually exhibit identical growth profiles. Rhamnolipid production was confirmed to rapidly reach about 10 times the WT levels within 2 days to finally reach a plateau (Figure 2C). A similar pattern was observed for isolate M63 (Supplementary Figure S2). Complementation of the scmR mutant with a plasmid-borne scmR gene restored the production toward WT levels (Figure 2C). Partial complementation is not unusual and can be attributed to several factors. First, an indirect regulation of rhamnolipid production is highly possible, thus complementation would also depend on additional regulatory elements. Second, a constitutive, not the native promoter is driving scmR on the expression plasmid, precluding transcription matching the appropriate level and timing. Third, ScmR is a LysR-type transcriptional regulator, thus requiring a ligand, whose availability might not be optimal, or depend on ScmR itself, since we reported that it is autoregulated (Le Guillouzer et al., 2020).
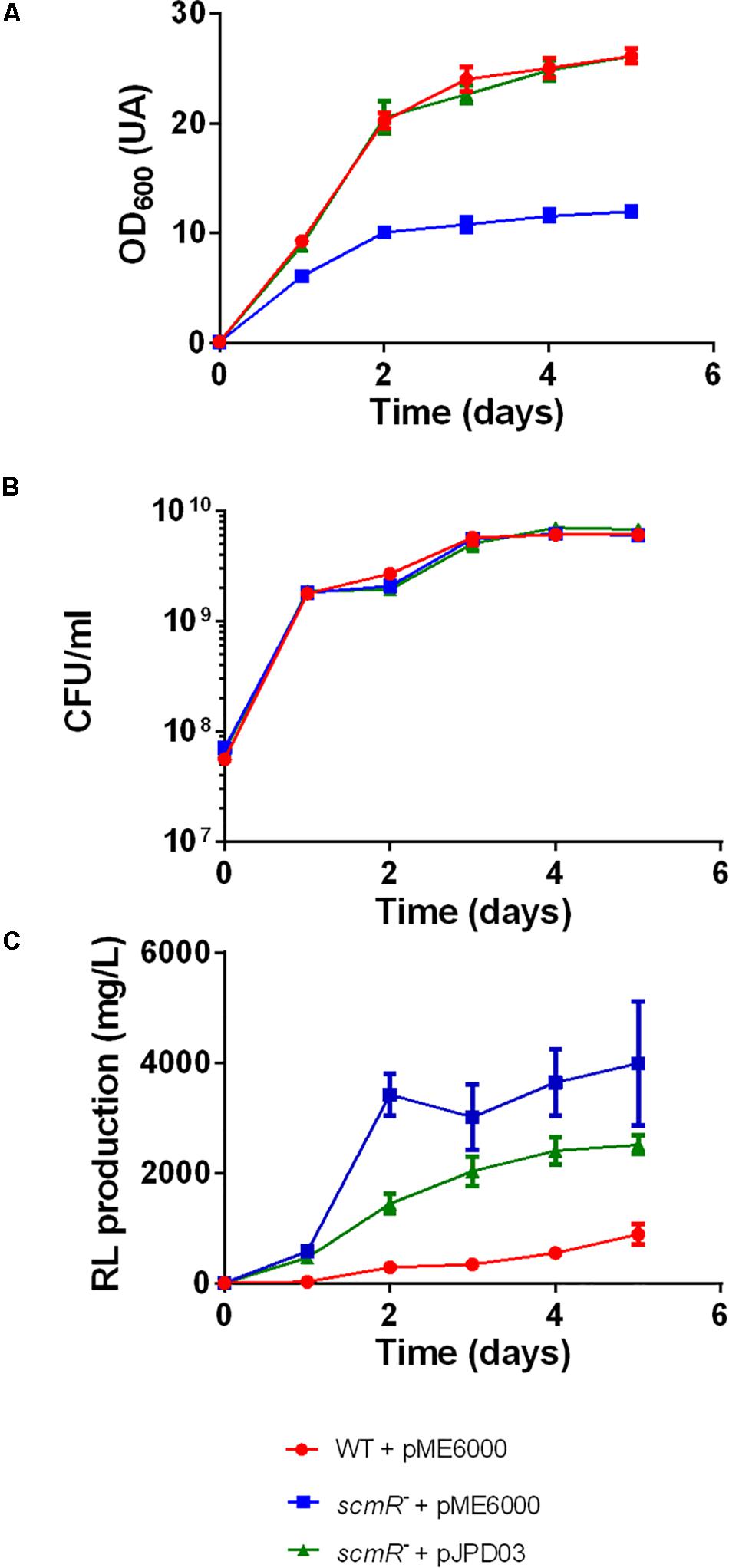
Figure 2. A scmR mutant produces more rhamnolipid than the WT strain. (A) Growth (OD600), (B) Growth (CFU/mL), and (C) rhamnolipid production (mg/L). The values are means ± standard deviation (error bars) for three replicates.
Transcriptions of Both rhl Operons Are Augmented in a scmR- Mutant
In B. thailandensis, two paralogous rhl operons are encoded and functional, both contributing to total rhamnolipid production (Dubeau et al., 2009). We thus verified the expression of each rhl operon in both the WT and the scmR- mutant ED1023 using transcriptional reporters. While the coding regions of the rhl operons are essentially identical, their respective promoter regions are not. Cultures of both the WT and the scmR- mutant ED1023 containing a rhlA1-lacZ or a rhlA2-lacZ reporter were performed in NB medium with 2% (v/v) glycerol. Figure 3 shows that the expression of each operon is higher in the scmR- background compared to the WT, revealing that ScmR acts a repressor of both rhl operons in strain E264. Interestingly, this effect was even more pronounced for the rhl2 operon, for which the transcription was up to 10 times higher than the in WT, while the transcription level was up to 4 times higher for rhl1, after 72 h of cultivation.
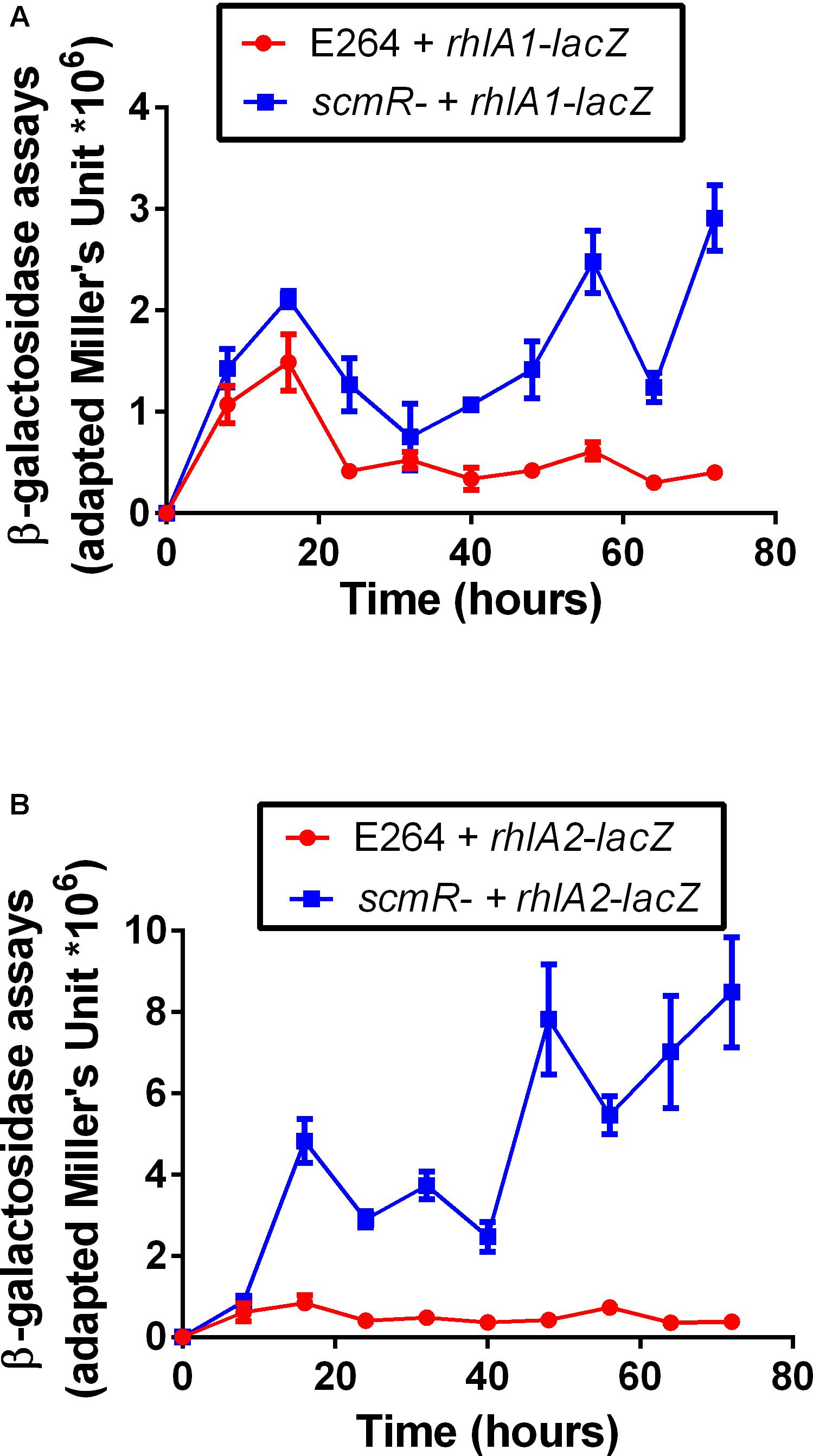
Figure 3. Transcription of both rhl operons is increased in the scmR mutant. β-galactosidase activity was measured using (A) rhlA1-lacZ and (B) rhlA2-lacZ reporters in both the wildtype E264 and the isogenic scmR mutant ED1023 in NB medium supplemented with 2% glycerol. OD420 measurements were normalized by CFU/mL. The values are means ± standard deviation (error bars) for three replicates.
ScmR Positively Activates PHA Biosynthesis
Burkholderia thailandensis is a known PHA producer (Funston et al., 2017; Kourmentza et al., 2018). Indeed, we demonstrated that B. thailandensis synthesizes significant levels of PHAs, leading to presence of granules inside the cells which makes unreliable the use of OD600 to estimate growth of this bacterium under conditions promoting PHA production (Martinez and Déziel, 2020). Judging by the discrepancy observed between OD600 measurements and the CFU counts profiles for both the WT and scmR- mutant strains (Figures 2A,B), and since PHAs are secondary metabolites, we hypothesized that ScmR could also affect the production of this biopolymer.
In order to verify if differences in PHA production are responsible for affecting the OD600 measurements shown in Figure 2A, we measured PHA cellular levels with Nile Blue fluorescent staining. PHA production was indeed lower (by about 50%) in the scmR- mutant vs the WT (Figure 4A). We then confirmed these results using electron microscopy and found that E264 contained an average of nine granules of PHA per cell, whereas the scmR- mutant had much fewer (an average of four granules) (Figures 4B,C). Also, cell shape and length comparisons highlighted that WT cells were 1.4-fold larger than mutant cells. These results revealed that ScmR promotes the production of PHAs in B. thailandensis and that PHA accumulation affects the cellular physiology, explaining the apparent growth defect of the scmR- mutant (Figure 2A). Complementation of the scmR-negative mutant with a plasmid-borne scmR gene corrected the OD600 difference we observed (Supplementary Figure S3) and restored production of PHAs to WT levels (Figures 4A,D).
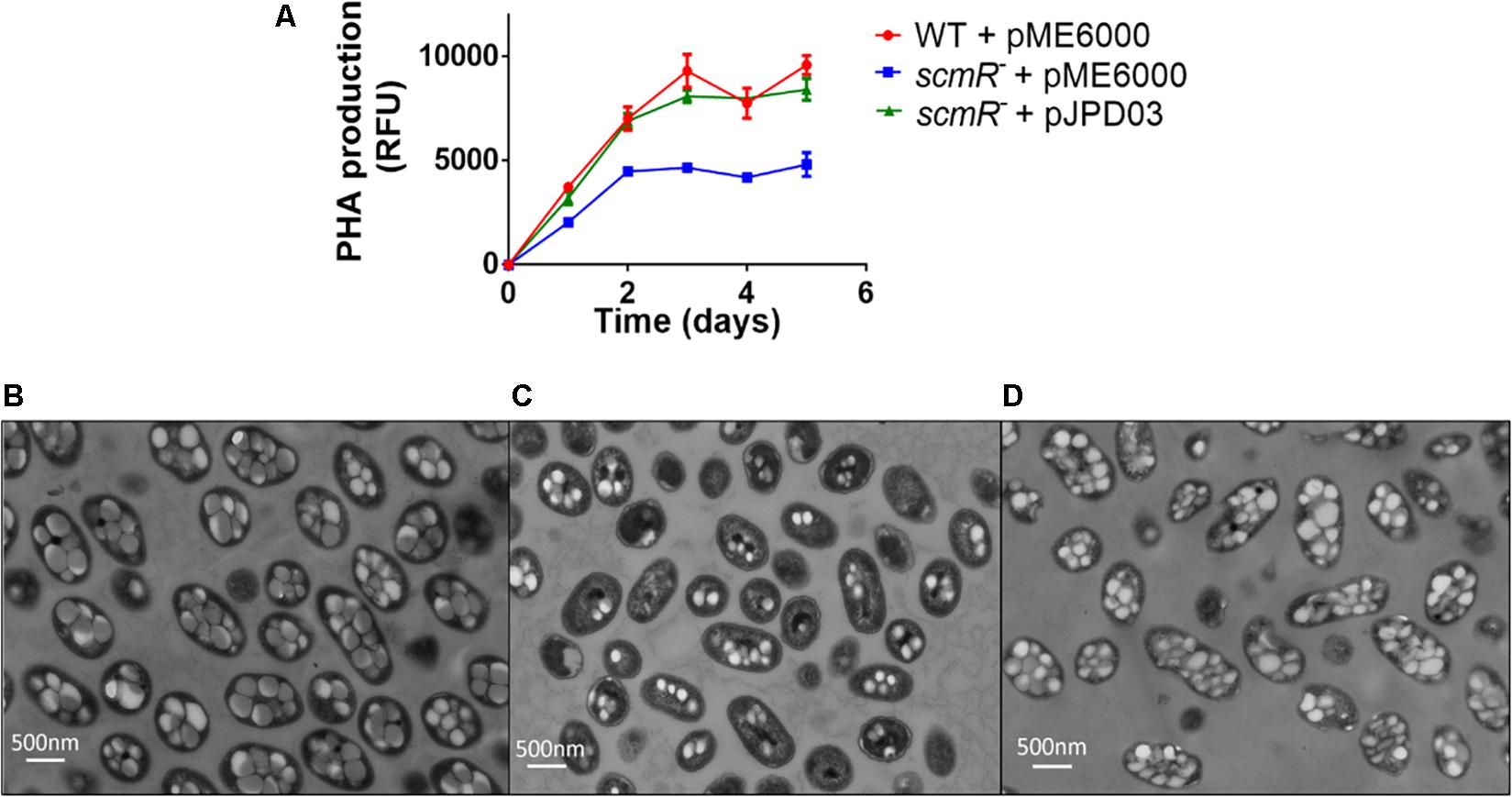
Figure 4. ScmR positively affects PHA biosynthesis. (A) PHA levels were determined by fluorescence following Nile Blue staining. The values are means ± standard deviation (error bars) for three replicates. (B–D) represent TEM images of E264, the scmR- mutant and the complemented scmR- mutant, respectively at a magnification of 10,000X.
Transcription of the Genes Responsible for the PHA Biosynthesis Is Affected in a scmR- Mutant
In order to better understand the effect of ScmR on the production of PHA, we looked at the expression of two genes implicated in PHA production. We previously demonstrated that the phaC gene from B. thailandensis, which encodes the PHA synthase, is homologous to the one found in the Cupriavidus genus (Martinez and Déziel, 2020). Also, in Cupriavidus necator, other genes are playing a role in PHA production, such as phaZ. The transcription levels of phaC and phaZ were quantified by qRT-PCR in the B. thailandensis E264 and in the scmR- mutant grown in NB with 2% glycerol, at 24 and 48 h of incubation. We measured reduced expression of phaC at both time points (Figure 5A), which correlates with the production patterns we observed (Figure 4A). The transcription levels of phaZ were also lower in the scmR mutant after 24 h, compared to the WT strain, while no significant difference was seen at 48 h (Figure 5B). All together, these data indicate that the decrease in PHA production we observed in the scmR mutant was likely caused by a lowered expression of pha metabolic genes.
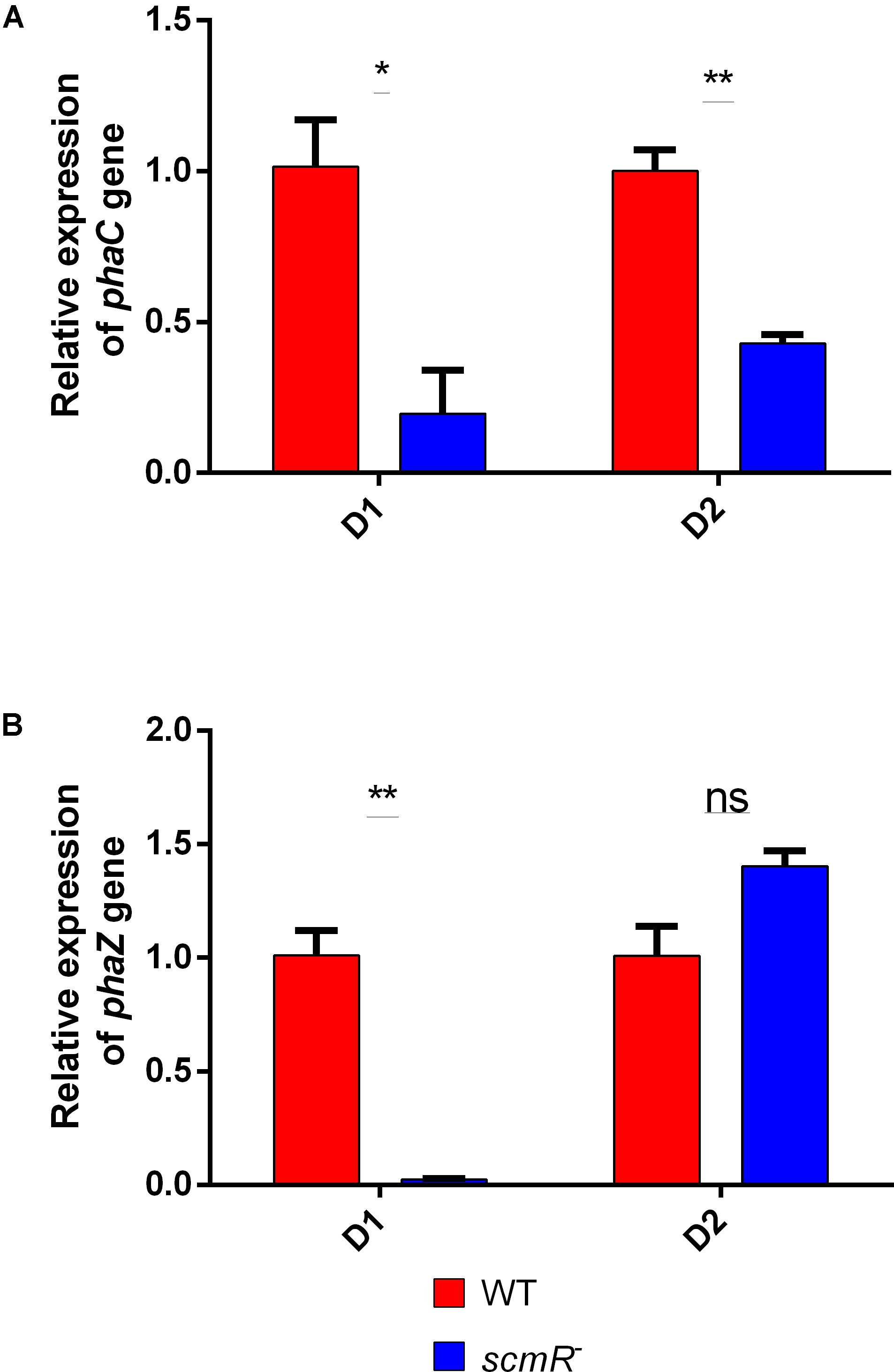
Figure 5. ScmR plays a role in PHA biosynthesis at the transcriptional level. Levels of transcription for the phaC (A) and phaZ (B) genes were determined by RT-qPCR in the wild type and the scmR– mutant strains. D1 and D2 are days of cultivation. The error bars represent standard deviation from the mean (n = 3 independent cultures). Data analyzed using a one-way ANOVA with post hoc. Dunnett’s multiple comparisons tests (∗∗p < 0.01, ∗p < 0.05, and ns = not significant).
The Expression of ScmR Is Regulated by Quorum Sensing
We demonstrated that ScmR is involved in both rhamnolipid and PHA biosynthesis. QS activates the transcription of the scmR gene in cultures of B. thailandensis grown in TSB (Le Guillouzer et al., 2020). Also, concentrations of all three AHLs are reduced in a scmR- mutant compared to the WT (Mao et al., 2017) when B. thailandensis is cultured in LB medium. We thus investigated the interplay between ScmR and QS to verify if they are associated in regulating rhamnolipid and PHA production under our specific conditions.
To further investigate the regulation of scmR by QS in NB with 2% glycerol, the transcription levels of scmR were quantified by qRT-PCR in the B. thailandensis E264 WT strain vs the ΔbtaI1ΔbtaI2ΔbtaI3 mutant. Since B. thailandensis possesses three QS systems (QS-1, QS-2 and QS-3) mainly associated with the production of C8-HSL, 3OHC8-HSL and 3OHC10-HSL respectively, we supplemented (or not) the AHL-defective ΔbtaI1ΔbtaI2ΔbtaI3 mutant with exogenous AHLs during the logarithmic growth phase to distinguish which of the three QS systems modulates the transcription of scmR under these culture conditions. We observed that expression of scmR is reduced in the absence of AHLs (Figure 6A), confirming that scmR transcription is positively modulated by QS. Furthermore, the transcription of scmR was increased in cultures of the ΔbtaI1ΔbtaI2ΔbtaI3 mutant when any of C8-HSL, 3OHC10-HSL, or 3OHC8-HSL were provided (Figure 6A). The response was higher with addition of C8-HSL and 3OHC8-HSL, associated with the QS-1 and QS-3 systems, respectively. The transcription of scmR in the ΔbtaR1, ΔbtaR2, and ΔbtaR3 mutants and the B. thailandensis E264 WT strain was also studied during the logarithmic growth phase in NB with 2% glycerol. No difference in scmR transcription was observed in ΔbtaR2. In contrast, it was decreased in both the ΔbtaR1 and ΔbtaR3 mutants (Figure 6B). Together, these results indicate that both QS-1 and QS-3 upregulate the transcription of scmR, but not QS-2.
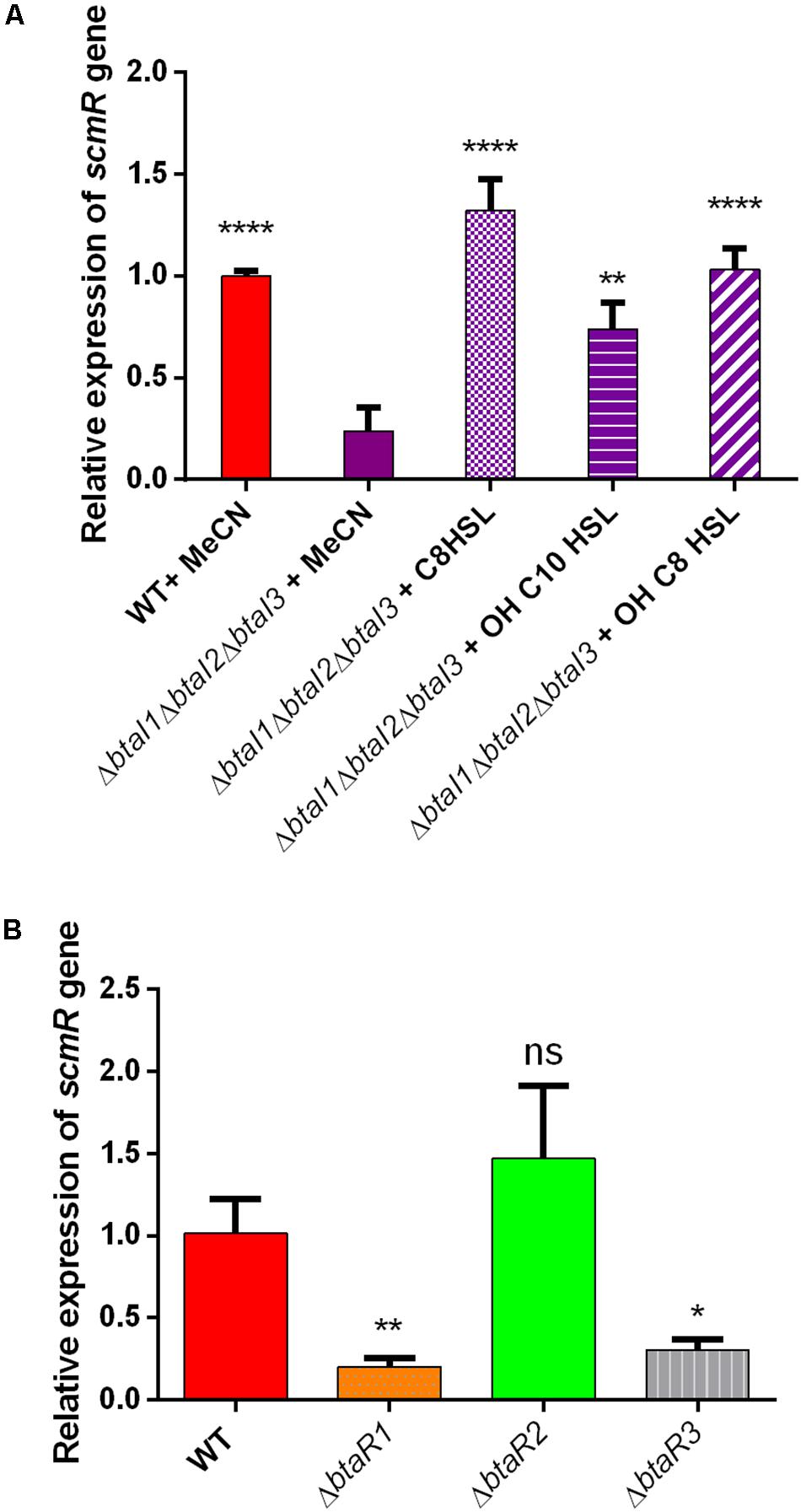
Figure 6. QS-1 and QS-3 activate the transcription of scmR. (A) The relative transcript levels of scmR in the B. thailandensis E264 wild-type and the ΔbtaI1ΔbtaI2ΔbtaI3 mutant strains were determined by qRT-PCR. Cultures were supplemented with 2 μM of C8-HSL, 3OHC10-HSL, or 3OHC8-HSL. Acetonitrile (MeCN) only was added to the controls. The results are presented as relative quantification of transcription of the gene compared to the wild-type strain, which was set at 100%. (B) The relative transcript levels of scmR were assessed by qRT-PCR in cultures of the wild-type and of the ΔbtaR1,ΔbtaR2, and ΔbtaR3 mutants of strain E264. The error bars represent standard deviation from the mean (n = 3 independent cultures), Data analyzed using a one-way ANOVA followed by Dunnett’s multiple comparisons tests (****p < 0.0001, ∗∗p < 0.01, ∗p < 0.05, and ns = not significant).
Quorum Sensing Modulates the Production of Both Rhamnolipids and PHAs
We then investigated the production of rhamnolipids in the AHL-defective mutant ΔbtaI1ΔbtaI2ΔbtaI3 compared to the WT. Because production of rhamnolipids and PHAs are closely related, we also measured PHA levels in the same cultures. Rhamnolipid production was about two times higher in the ΔbtaI1ΔbtaI2ΔbtaI3 mutant vs the WT (Figure 7C). On the other hand, the ΔbtaI1ΔbtaI2ΔbtaI3 mutant produced less than half the PHAs compared to the WT (Figure 7D) and this was confirmed by TEM images of cells sampled at day 4 (Figures 7E,F). Accordingly, we observed a difference in OD600 measurements for the ΔbtaI1ΔbtaI2ΔbtaI3 mutant when compared to WT (Figure 7A), but once again CFU counts confirmed that growth was similar between both strains (Figure 7B). The exact same situation was observed with the scmR- mutant. We thus rationalized that the QS-null strain mostly behaves like the scmR- mutant because QS activates scmR (Figure 7A).
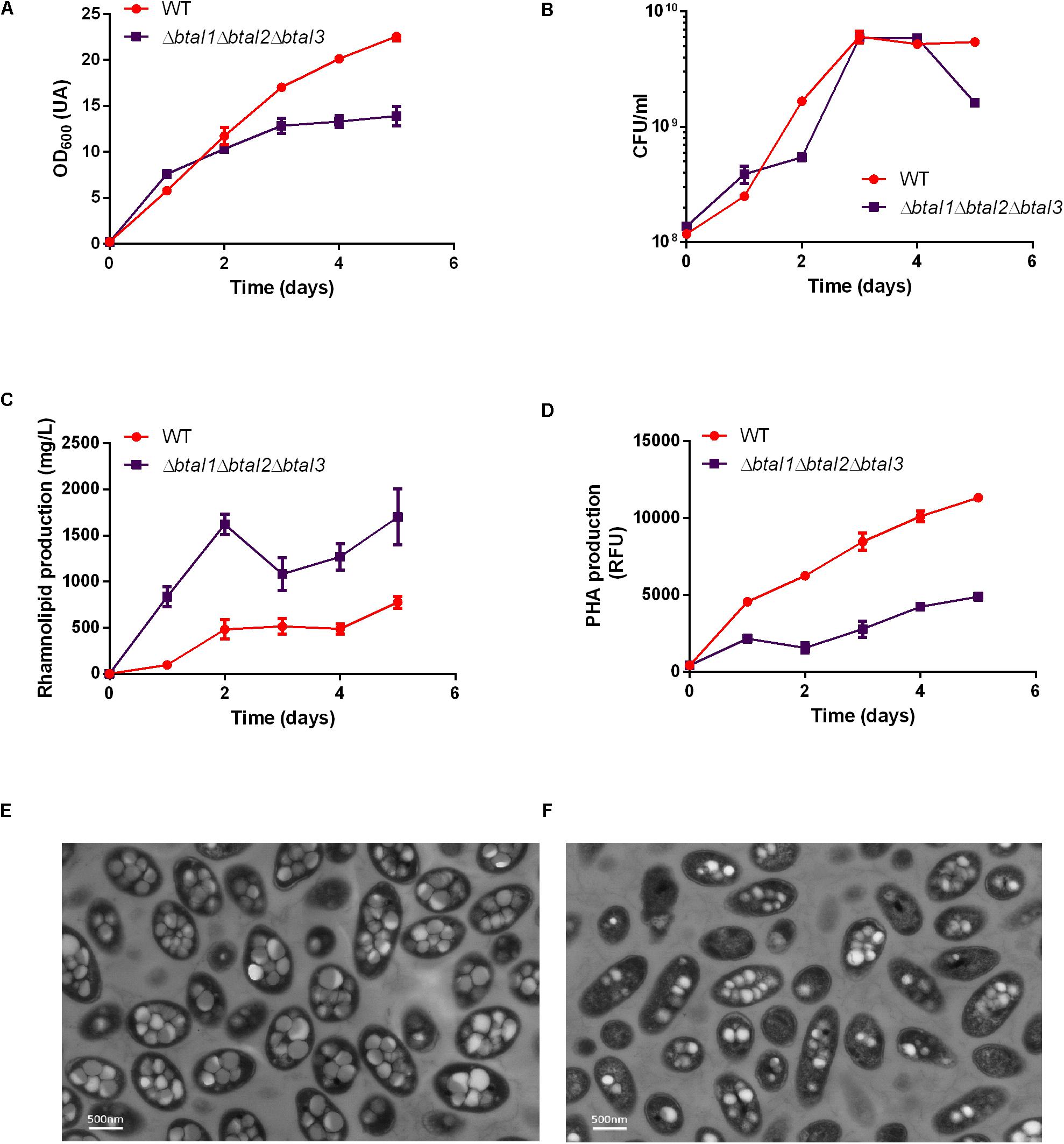
Figure 7. Rhamnolipid and PHA production are quorum sensing-controlled. The wild type strain and the ΔbtaI1ΔbtaI2ΔbtaI3 quorum sensing mutant were cultured for five days at 30°C in NB medium supplemented with 2% glycerol. The (A) OD600, (B) CFU/mL, (C) PHA levels, and (D) rhamnolipid concentration measurements are represented. Values are means ± standard deviations (error bars) for three replicates. TEM images were taken at day 4 for panels (E) the wild type strain and (F) the ΔbtaI1ΔbtaI2ΔbtaI3 mutant.
Finding that loss of scmR increases the production of rhamnolipids and decreases the production of PHAs brought us to identify the complex QS circuitry of B. thailandensis as an apparently contrasting regulator of production of these metabolites. We then sought to separately investigate the effect of the three QS systems in the regulation of rhamnolipid production in E264. We quantified rhamnolipids and PHAs in cultures of individual btaI and btaR mutants after 5 days. Interestingly, none of three individual btaI mutants replicated the OD600 defect of the triple mutant, suggesting no decrease in PHA production, apart from a partial reduction for the btaI3 mutant (Figure 8A). Accordingly, growth was not affected (Figure 8B). No difference was observed in final rhamnolipid levels when we compared the ΔbtaI1, ΔbtaI2 or ΔbtaR1, ΔbtaR2- mutants to the WT strain E264. In contrast, rhamnolipid concentrations were increased when btaI3 or btaR3 were inactivated (Figures 8C,E). Similarly, no significant difference in PHA biosynthesis was observed for QS-1 and QS-2 mutants, while we measured less PHAs in the QS-3 system mutants (Figures 8D,F). Based on these observations, we conclude that the QS-3 system negatively affects rhamnolipid production whereas it positively impacts PHA biosynthesis, similarly to ScmR, but less pronounced.
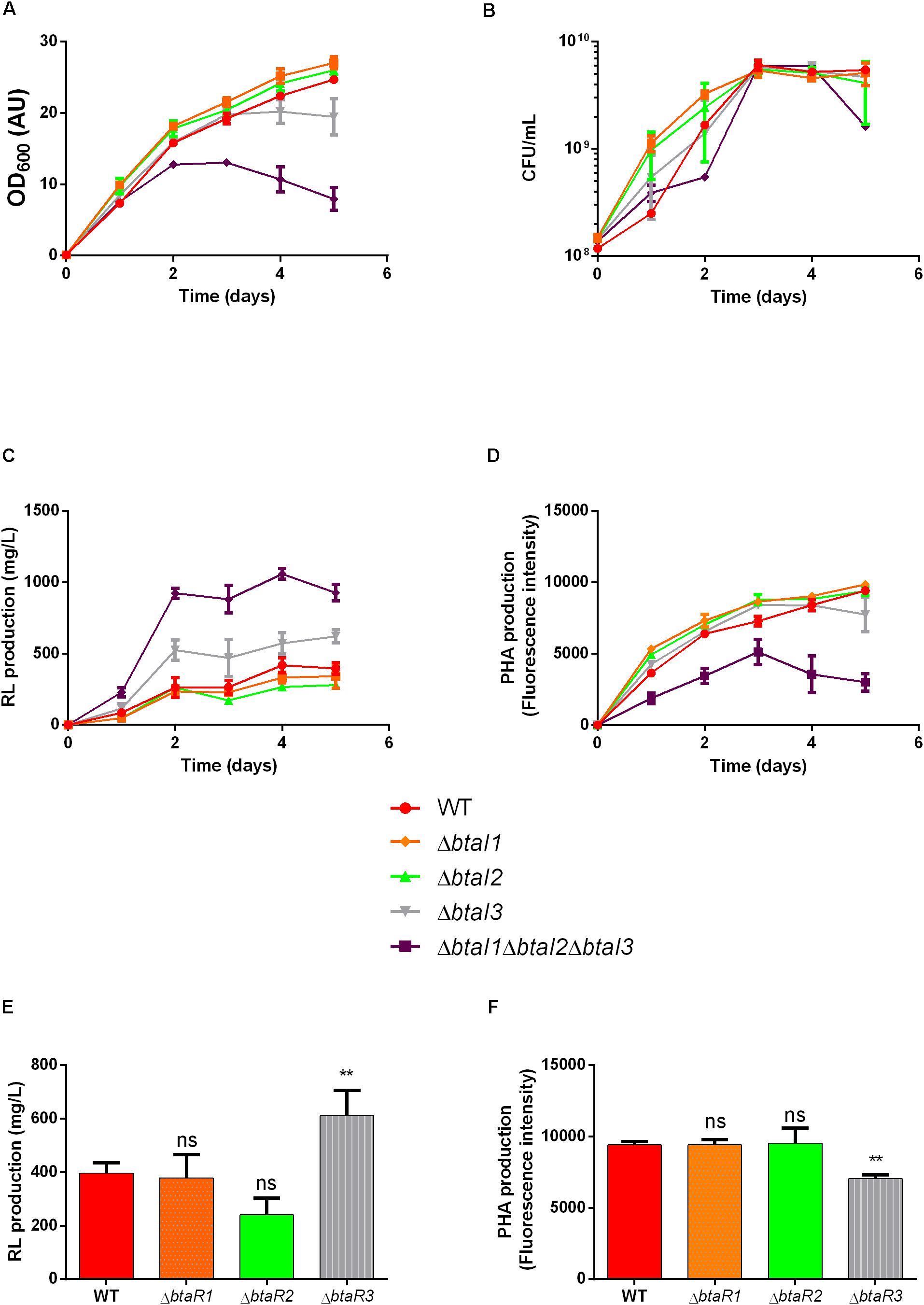
Figure 8. The QS-3 quorum sensing system affects the production of both PHAs and rhamnolipids. Wild type strain E264 and isogenic quorum sensing mutants were cultured during five days at 30°C in NB medium supplemented with 2% glycerol. Growth measurements are described for ΔbtaI mutants in panel (A) OD600 and (B) CFU/mL. (C) Rhamnolipid concentrations and (D) PHA levels are presented for the ΔbtaI mutants. (E) Rhamnolipid concentrations and (F) PHA levels are presented for the ΔbtaR mutants in the QS-1, QS-2 and QS-3 systems. The error bars represent standard deviation from the mean (n = 3 independent cultures), Data analyzed using a one-way ANOVA with post hoc. Dunnett’s multiple comparisons tests (∗∗p < 0.01 and ns = not significant).
Discussion and Conclusion
The non-pathogenic bacterium B. thailandensis is a promising candidate for the production of rhamnolipids (Dubeau et al., 2009; Funston et al., 2016; Irorere et al., 2018; Kourmentza et al., 2018). However, current yields remain low. While the biosynthetic genes have been identified, their regulation is poorly understood. We propose that better knowledge of this aspect is important to improve the production of these metabolites. The primary aim of this work was to identify genes involved in the regulation of rhamnolipid biosynthesis. Our functional screening allowed us to identify ScmR as a negative regulatory element of rhamnolipid production. In B. thailandensis, global transcription of the rhl biosynthetic genes was previously studied (Funston et al., 2016) using qRT-PCR on coding sequences, thus without taking into consideration that two identical rhl operons are present in B. thailandensis (Dubeau et al., 2009). Both operons distinctly contribute to the total rhamnolipid production. Indeed, a mutation in either rhlA1 or rhlA2 causes a decrease but not the abolishment of rhamnolipid biosynthesis. Hence, we previously reported that the rhlA2 operon is responsible for a larger portion of total rhamnolipid production since the rhlA1 mutant produces more rhamnolipids than the rhlA2 mutant (Dubeau et al., 2009). This suggests that both rhl operons are differently regulated. Accordingly, the transcription of both rhl operons was differently increased in the scmR- mutant, at least partially explaining how ScmR represses rhamnolipid biosynthesis. Indeed, the effect on rhlA2 was larger than on rhlA1, showing again that the regulation of both operons is different. Further studies are needed to better understand the distinct regulation of each rhl operon. Whether or not the regulation of ScmR on each of the rhl operons is direct has yet to be confirmed.
Similarly, PHA production is not well documented in B. thailandensis. Only few recent studies reported the production of PHAs in this bacterial species. One of them revealed the potential of PHAs production of strain E264 (Funston et al., 2017). Another one described PHAs produced during growth on used cooking oil-containing medium (Kourmentza et al., 2018), where PHA biosynthesis occurred during the logarithmic phase. In several bacteria, excess carbon availability promotes PHA production when nutritional elements such as N, P or Mg are limiting (Anderson and Dawes, 1990). However, based on our observations, it seems that B. thailandensis belongs to the group of growth-associated PHA producers, meaning that limitation of an essential nutrient is not necessary for the induction of PHA biosynthesis. Other Burkholderia species can produce PHAs, such as B. xenovorans, B. sacchari, and B. cepacia (Urtuvia et al., 2014), so it is consistent that B. thailandensis is also a significant producer. PHA production is regulated by QS in other bacteria such as Pseudomonas chlororaphis (Mohanan et al., 2019), Rhodobacter sphaeroides (Kho et al., 2003) and Vibrio harveyi (Sun et al., 1994). However, QS regulation can be either negative or positive depending on the organism and/or the conditions.
When looking at the transcription of two genes implicated in the biosynthesis of PHAs, we found that the transcription of both phaC and phaZ was affected in the scmR- mutant. When comparing to the sequence of phaZa1 from C. necator H16, there are two predicted PHA or PHB depolymerase genes in the genome of B. thailandensis E264 (BTH_II2312 and BTH_I0973). Sequence comparison analyses show that gene BTH_ I0973 presents the highest identity percentage (79.4%) and we thus consider it as the most likely phaZ candidate (Saegusa et al., 2001; Uchino et al., 2008). Our finding that ScmR activates PHA biosynthesis by modulating the transcription of phaC and phaZ is interesting for future investigations on the potential of B. thailandensis as a PHA producer. Whether the positive effect of ScmR on the transcription of phaC and phaZ is direct or indirect remains to be determined.
The implication of the ScmR regulator in secondary metabolism was recently described in B. thailandensis, where it was reported to act as an important repressor (Mao et al., 2017). Our data showed that ScmR regulates the production of rhamnolipids and PHA while neither rhl nor pha biosynthesis genes were found in transcriptomic studies performed on scmR- mutants (Mao et al., 2017; Le Guillouzer et al., 2020). We noted that the LB medium is not appropriate for rhamnolipid production compared to NB medium complemented with 2% glycerol (Dubeau et al., 2009), at least partially explaining the absence of rhl gene regulation in the Mao et al. (2017) study. While they characterized the ScmR regulon in the stationary phase (Mao et al., 2017), we confirm here that this regulator is already active during the logarithmic phase. Interestingly, we found that in the WT, both metabolites are synthetized during growth but only the concentration of PHAs keeps augmenting when stationary phase is reached. In contrast, when B. thailandensis was cultivated on used cooking oil, rhamnolipid biosynthesis continued after the stationary phase was reached, while PHA production decreased (Kourmentza et al., 2018), suggesting a tight regulation of these two related metabolites.
The slow-down in rhamnolipid production we observed under our conditions at the stationary phase could be because of changes in nutrient availability, in pH, or in dissolved oxygen concentration. For instance, restricted rhamnose availability could explain why rhamnolipid production was reduced while PHAs kept being synthetized using the lipid precursors. In P. aeruginosa, dissolved oxygen concentration has a crucial effect on rhamnolipid production (Zhao et al., 2018; Bazsefidpar et al., 2019). The pH was also reported to impact rhamnolipid production in P. aeruginosa (Chen et al., 2007; Zhu et al., 2012). In B. thailandensis cultures in NB medium with 2% glycerol, the pH increased during the log phase and then decreased at the stationary phase (Supplementary Figure S4A). Oxalate production by Obc1, encoded by the obc1 gene, is involved in the decrease of pH observed in cultures of B. thailandensis (Goo et al., 2012). We know that obc1 transcription is strongly downregulated in the scmR- mutant (Le Guillouzer et al., 2020). Indeed, in cultures of the scmR- mutant, pH remained higher than in cultures of the WT and this difference could be responsible for the slowing in rhamnolipid production. To verify this hypothesis, we compared rhamnolipid production in cultures of an obc1 mutant, unable to produce d-oxalate, vs the WT E264 strain. Rhamnolipid production was not affected in this mutant, suggesting that a pH increase is not involved in the late slow-down in rhamnolipid production (Supplementary Figure S4B).
The QS circuitry of B. thailandensis is very complex, including homeostatic regulatory loops and additional regulators (Le Guillouzer et al., 2017, 2018). Production of rhamnolipids and other biosurfactants are often regulated by QS in various bacterial species. An initial global analysis of QS in B. thailandensis revealed that it seems to affect the transcription of rhlA1, rhlB1 and rhlB2, encoding rhamnolipid biosynthesis (Majerczyk et al., 2014b). However, this study was performed in LB medium, as stated above not ideal to induce rhl genes. Plus, no involvement of QS into the regulation of known PHA synthesis genes was described in this transcriptomic study, again suggesting the use of culture conditions not appropriate to induce biosynthesis. The production of both rhamnolipids and PHAs was specifically studied with the ΔbtaI1ΔbtaI2ΔbtaI3 QS-defective mutant (Irorere et al., 2019) and was affected. Indeed, rhamnolipid production was increased while PHA biosynthesis was decreased (Irorere et al., 2019) which correlates with our observations. Interestingly, in B. thailandensis, QS appears to have an opposite effect on rhamnolipid production compared to in P. aeruginosa or B. glumae. In P. aeruginosa, the disruption of the rhlR QS system leads to a deficiency in rhamnolipid production and a functional RhlR is necessary for biosynthesis (Ochsner et al., 1994; Nakata et al., 1998). Similarly, mutation in the TofI/R QS system in B. glumae downregulates the production of rhamnolipids (Nickzad et al., 2015).
QS and ScmR are closely linked. Hence, the production of all three AHLs is reduced in a scmR- mutant (Mao et al., 2017; Le Guillouzer et al., 2020) which mimics the QS-deficient mutant. The presence of a Lux-box into the scmR promoter suggests that QS could directly activate the transcription of ScmR (Mao et al., 2017). Accordingly, we found lower transcription of scmR in both ΔbtaR1 and ΔbtaR3 mutants (Figure 5; Le Guillouzer et al., 2020). Moreover, the addition of the three AHLs led to increased scmR transcription in a ΔbtaI1ΔbtaI2ΔbtaI3, especially by C8-HSL and the 3OHC8-HSL which are principally produced by the QS-1 and QS-3 systems, respectively. Interestingly, we previously found an interdependence between the QS-1 and QS-3 systems (Le Guillouzer et al., 2017). Indeed, btaI3 transcription is controlled by the BtaR1/C8-HSL complex during the logarithmic growth phase while BtaR3 could be involved in the modulation of the btaI1 gene in conjunction with 3OHC10-HSL and 3OHC8-HSL (Le Guillouzer et al., 2017). Here, we observed a significant augmentation in rhamnolipid production and a decrease in PHA accumulation in the btaI3- and btaR3- mutants, consistent with scmR upregulation by the QS-3 system. In addition, we showed that scmR transcription is activated by BtaR1, presumably through an indirect activation of QS-3 by QS-1. These observations concur with our data obtained when B. thailandensis is grown in TSB medium (Le Guillouzer et al., 2020). Rhamnolipid production was higher in the scmR- mutant compared to the ΔbtaI1ΔbtaI2ΔbtaI3- mutant. Indeed, the ΔbtaI1ΔbtaI2ΔbtaI3 strain produced 1.7 times more rhamnolipid compared to the WT strain while the scmR- mutant produced about 3-4 times more rhamnolipid than the WT. Similarly, PHA production was reduced by 40% in the ΔbtaI1ΔbtaI2ΔbtaI3 and 50% in the scmR- mutant. Collectively, these observations suggest that QS is not the only regulation positively affecting the transcription of the scmR gene. We conclude that inhibition of rhamnolipids and increase in PHAs production by QS probably go through an effect on ScmR.
In conclusion, this study significantly improves our understanding of the regulation of both rhamnolipid and PHA production in B. thailandensis. We demonstrate that QS, and most specifically the QS-3 system, directs this production (Figure 9). We also found that ScmR is a negative regulatory element of rhamnolipid production and positively affects PHA synthesis and confirmed the QS-dependent regulation of the scmR gene. Since there is a difference in PHA and rhamnolipid production between the ΔbtaI1ΔbtaI2ΔbtaI3 and scmR- mutants, we conclude that scmR is not exclusively modulated by QS, suggesting more work is needed to fully uncover the regulation of rhamnolipid and PHA production in B. thailandensis to optimize it to its full potential. ScmR and QS represent promising targets to engineer strains of B. thailandensis producing more rhamnolipids or PHAs.
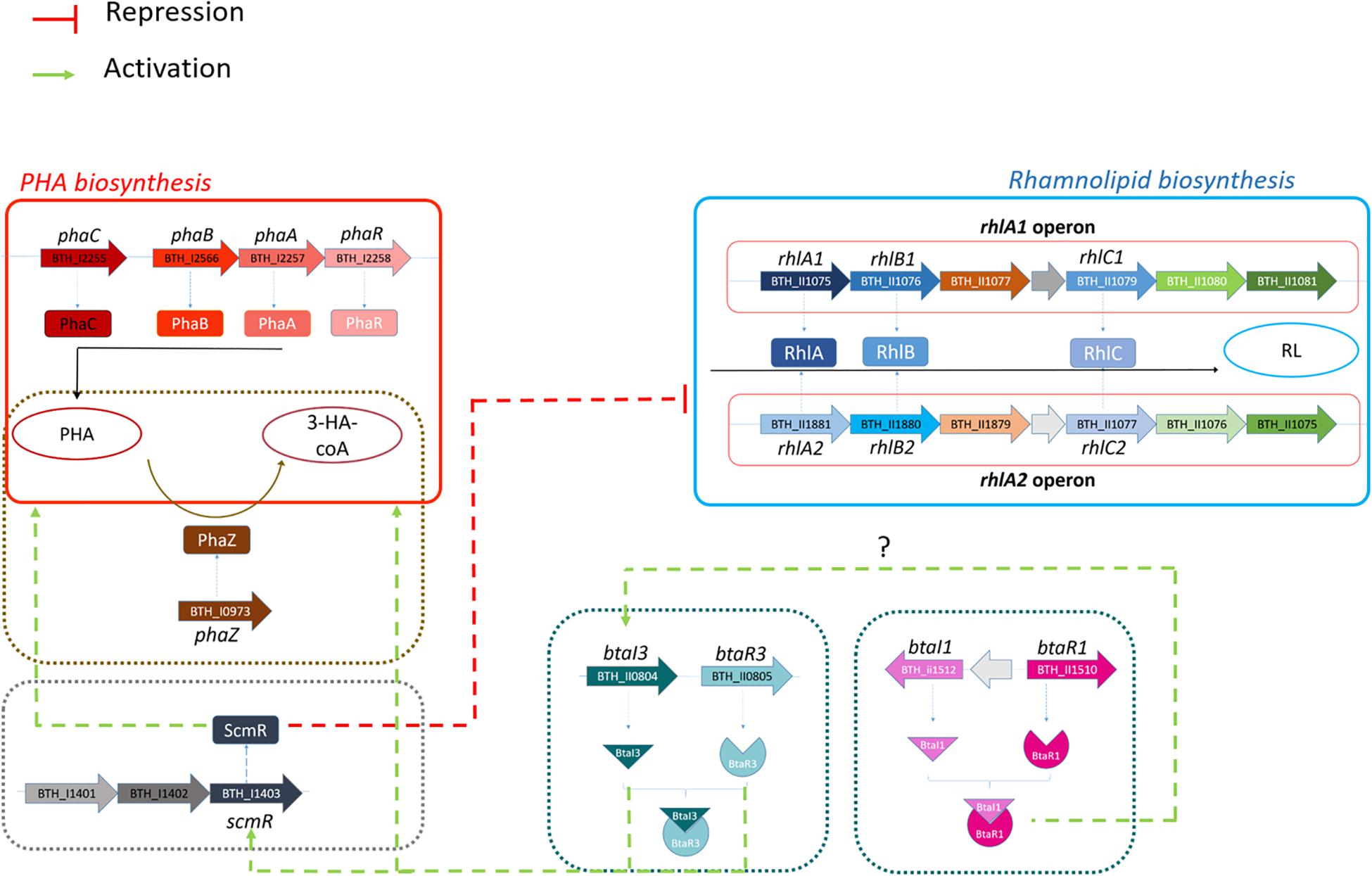
Figure 9. Proposed regulation of rhamnolipid and PHA productions through ScmR and quorum sensing systems in B. thailandensis E264. Rhamnolipid and PHA biosynthesis pathways are presented in red and blue, respectively. The involvement of ScmR, QS-1 and QS-3 on rhamnolipid and PHA production are represented using green dotted arrows for activation or red dotted lines for the repression. Dotted arrow lines indicate that direct regulation has not been confirmed.
Data Availability Statement
All datasets generated for this study are included in the article/Supplementary Material.
Author Contributions
SM, AH, and ED conceived and designed the experiments. SM performed the experiments. SM, AH, M-CG, and ED analyzed the data. AH and M-CG contributed reagents, materials, and analysis tools. SM, M-CG, and ED contributed to writing, editing, and finalizing the manuscript. All authors contributed to the article and approved the submitted version.
Funding
This work was supported by Discovery Grant No. RGPIN-2015-03931 from the Natural Sciences and Engineering Research Council of Canada (NSERC). SM was recipient of a Ph.D. scholarship awarded by the Fondation Armand-Frappier. ED holds the Canada Research Chair in Sociomicrobiology.
Conflict of Interest
The authors declare that the research was conducted in the absence of any commercial or financial relationships that could be construed as a potential conflict of interest.
Acknowledgments
We thank Sylvain Milot and Marianne Piochon for their help with LC/MS analyses and Arnaldo Nakamura for the TEM imaging.
Supplementary Material
The Supplementary Material for this article can be found online at: https://www.frontiersin.org/articles/10.3389/fbioe.2020.01033/full#supplementary-material
References
Abdel-Mawgoud, A. M., Lépine, F., and Déziel, E. (2010). Rhamnolipids: diversity of structures, microbial origins and roles. Appl. Microbiol. Biotechnol. 86, 1323–1336. doi: 10.1007/s00253-010-2498-2
Anderson, A. J., and Dawes, E. A. (1990). Occurrence, metabolism, metabolic role, and industrial uses of bacterial polyhydroxyalkanoates. Microbiol. Rev. 54, 450–472. doi: 10.1128/mmbr.54.4.450-472.1990
Banat, I. M., Makkar, R. S., and Cameotra, S. S. (2000). Potential commercial applications of microbial surfactants. Appl. Microbiol. Biotechnol. 53, 495–508. doi: 10.1007/s002530051648
Bazsefidpar, S., Mokhtarani, B., Panahi, R., and Hajfarajollah, H. (2019). Overproduction of rhamnolipid by fed-batch cultivation of Pseudomonas aeruginosa in a lab-scale fermenter under tight DO control. Biodegradation 30, 59–69. doi: 10.1007/s10532-018-09866-3
Brett, P. J., Deshazer, D., and Woods, D. E. (1998). Burkholderia thailandensis sp. nov., a Burkholderia pseudomallei-like species. Int. J. Syst. Bacteriol. 48, 317–320. doi: 10.1099/00207713-48-1-317
Burch, A. Y., Shimada, B. K., Browne, P. J., and Lindow, S. E. (2010). Novel high-throughput detection method to assess bacterial surfactant production. Appl. Environ. Microbiol. 76, 5363–5372. doi: 10.1128/aem.00592-10
Chandler, J. R., Duerkop, B. A., Hinz, A., West, T. E., Herman, J. P., Churchill, M. E., et al. (2009). Mutational analysis of Burkholderia thailandensis quorum sensing and self-aggregation. J. Bacteriol. 191, 5901–5909. doi: 10.1128/jb.00591-09
Chen, S.-Y., Wei, Y.-H., and Chang, J.-S. (2007). Repeated pH-stat fed-batch fermentation for rhamnolipid production with indigenous Pseudomonas aeruginosa S2. Appl. Microbiol. Biotechnol. 76, 67–74. doi: 10.1007/s00253-007-0980-2
Dennis, J. J., and Sokol, P. A. (1995). “Electrotransformation of,” in Electroporation Protocols for Microorganisms Pseudomonas, ed. J. A. Nickoloff (Berlin: Springer), 125–133.
Dubeau, D., Déziel, E., Woods, D. E., and Lépine, F. (2009). Burkholderia thailandensis harbors two identical rhl gene clusters responsible for the biosynthesis of rhamnolipids. BMC Microbiol. 9:263. doi: 10.1186/1471-2180-9-263
Durand, A.-A., Bergeron, A., Constant, P., Buffet, J.-P., Déziel, E., and Guertin, C. (2015). Surveying the endomicrobiome and ectomicrobiome of bark beetles: the case of Dendroctonus simplex. Sci. Rep. 5:17190.
Funston, S. J., Tsaousi, K., Rudden, M., Smyth, T. J., Stevenson, P. S., Marchant, R., et al. (2016). Characterising rhamnolipid production in Burkholderia thailandensis E264, a non-pathogenic producer. Appl. Microbiol. Biotechnol. 100, 7945–7956. doi: 10.1007/s00253-016-7564-y
Funston, S. J., Tsaousi, K., Smyth, T. J., Twigg, M. S., Marchant, R., and Banat, I. M. (2017). Enhanced rhamnolipid production in Burkholderia thailandensis transposon knockout strains deficient in polyhydroxyalkanoate (PHA) synthesis. Appl. Microbiol. Biotechnol. 101, 8443–8454. doi: 10.1007/s00253-017-8540-x
Fuqua, C., Winans, S. C., and Greenberg, E. P. (1994). Quorum sensing in bacteria: the LuxR-LuxI family of cell density-responsive transcriptional regulators. J.Bacteriol. 176, 269–275. doi: 10.1128/jb.176.2.269-275.1994
Gallagher, L. A., Ramage, E., Patrapuvich, R., Weiss, E., Brittnacher, M., and Manoil, C. (2013). Sequence-defined transposon mutant library of Burkholderia thailandensis. mBio 4:e00604-13.
Goo, E., Majerczyk, C. D., An, J. H., Chandler, J. R., Seo, Y.-S., Ham, H., et al. (2012). Bacterial quorum sensing, cooperativity, and anticipation of stationary-phase stress. Proc. Natl. Acad. Sci. U.S.A. 109, 19775–19780. doi: 10.1073/pnas.1218092109
Irorere, V., Kwiencien, M., Tripathi, L., Cobice, D., Mcclean, S., Marchant, R., et al. (2019). Quorum sensing as a potential target for increased production of rhamnolipid biosurfactant in Burkholderia thailandensis E264. Appl. Microbiol. Biotechnol. 103, 6505–6517. doi: 10.1007/s00253-019-09942-5
Irorere, V. U., Smyth, T. J., Cobice, D., Mcclean, S., Marchant, R., and Banat, I. M. (2018). Fatty acid synthesis pathway provides lipid precursors for rhamnolipid biosynthesis in Burkholderia thailandensis E264. Appl. Microbiol. Biotechnol. 102, 6163–6174. doi: 10.1007/s00253-018-9059-5
Jacobs, M. A., Alwood, A., Thaipisuttikul, I., Spencer, D., Haugen, E., Ernst, S., et al. (2003). Comprehensive transposon mutant library of Pseudomonas aeruginosa. Proc. Natl. Acad. Sci. U.S.A. 100, 14339–14344. doi: 10.1073/pnas.2036282100
Jarvis, F., and Johnson, M. (1949). A glyco-lipide produced by Pseudomonas aeruginosa. J. Am. Chem. Soci. 71, 4124–4126. doi: 10.1021/ja01180a073
Kang, H. Y., Dozois, C. M., Tinge, S. A., Lee, T. H., and Curtiss, R. (2002). Transduction-mediated transfer of unmarked deletion and point mutations through use of counterselectable suicide vectors. J. Bacteriol. 184, 307–312. doi: 10.1128/jb.184.1.307-312.2002
Kho, D. H., Jang, J. H., Kim, H. S., Kim, K. S., and Lee, J. K. (2003). Quorum sensing of Rhodobacter sphaeroides negatively regulates cellular poly-beta-hydroxybutyrate content under aerobic growth conditions. J. Microbiol. Biotechnol. 13, 477–481.
Kourmentza, C., Costa, J., Azevedo, Z., Servin, C., Grandfils, C., De Freitas, V., et al. (2018). Burkholderia thailandensis as a microbial cell factory for the bioconversion of used cooking oil to polyhydroxyalkanoates and rhamnolipids. Bioresour. Technol. 247, 829–837. doi: 10.1016/j.biortech.2017.09.138
Kucera, D., Benesova, P., Ladicky, P., Pekar, M., Sedlacek, P., and Obruca, S. (2017). Production of polyhydroxyalkanoates using hydrolyzates of spruce sawdust: comparison of hydrolyzates detoxification by application of overliming, active carbon, and lignite. Bioengineering 4:53. doi: 10.3390/bioengineering4020053
Lang, S., and Wullbrandt, D. (1999). Rhamnose lipids–biosynthesis, microbial production and application potential. Appl. Microbiol. Biotechnol. 51, 22–32. doi: 10.1007/s002530051358
Le Guillouzer, S., Groleau, M. C., and Déziel, E. (2018). Two rsaM homologues encode central regulatory elements modulating quorum sensing in Burkholderia thailandensis. J. Bacteriol. 200:JB.00727-17.
Le Guillouzer, S., Groleau, M. C., Mauffrey, F., and Déziel, E. (2020). ScmR, a global regulator of gene expression, quorum sensing, pH homeostasis, and virulence in Burkholderia thailandensis. J. Bacteriol. 202:e00776–19. doi: 10.1128/JB.00776-19
Le Guillouzer, S., Groleau, M.-C., and Déziel, E. (2017). The complex quorum sensing circuitry of Burkholderia thailandensis is both hierarchically and homeostatically organized. mBio 8:e01861-17.
Lesic, B., and Carniel, E. (2005). Horizontal transfer of the high-pathogenicity island of Yersinia pseudotuberculosis. J. Bacteriol. 187, 3352–3358. doi: 10.1128/jb.187.10.3352-3358.2005
Livak, K. J., and Schmittgen, T. D. (2001). Analysis of relative gene expression data using real-time quantitative PCR and the 2−ΔΔCT Method. Methods 25, 402–408. doi: 10.1006/meth.2001.1262
Majerczyk, C., Brittnacher, M. J., Jacobs, M. A., Armour, C. D., Radey, M. C., Bunt, R., et al. (2014a). Cross-species comparison of the Burkholderia pseudomallei, Burkholderia thailandensis, and Burkholderia mallei quorum-sensing regulons. J. Bacteriol. 196, 3862–3871. doi: 10.1128/jb.01974-14
Majerczyk, C., Brittnacher, M., Jacobs, M., Armour, C. D., Radey, M., Schneider, E., et al. (2014b). Global Analysis of the Burkholderia thailandensis quorum-sensing-controlled regulon. J. Bacteriol. 196, 1412–1424. doi: 10.1128/jb.01405-13
Majerczyk, C. D., Greenberg, E. P., and Chandler, J. R. (2013). “Quorum sensing in Burkholderia,” in Regulation of Bacterial Virulence, eds M. L. Vasil and A. J. Darwin (Washington, DC: American Society of Microbiology), 40–57. doi: 10.1128/9781555818524.ch3
Mao, D., Bushin, L. B., Moon, K., Wu, Y., and Seyedsayamdost, M. R. (2017). Discovery of scmR as a global regulator of secondary metabolism and virulence in Burkholderia thailandensis E264. Proc. Natl. Acad. Sci. U.S.A. 114, E2920–E2928.
Martinez, S., and Déziel, E. (2020). Changes in polyhydroxyalkanoate granule accumulation make optical density measurement an unreliable method for estimating bacterial growth in Burkholderia thailandensis. Can. J. Microbiol. 66, 256–262. doi: 10.1139/cjm-2019-0342
Maurhofer, M., Reimmann, C., Schmidli-Sacherer, P., Heeb, S., Haas, D., and Défago, G. (1998). Salicylic acid biosynthetic genes expressed in Pseudomonas fluorescens strain P3 improve the induction of systemic resistance in tobacco against tobacco necrosis virus. Phytopathology 88, 678–684. doi: 10.1094/phyto.1998.88.7.678
Miller, J. (1972). “Assay of B-galactosidase,” in Experiments in Molecular Genetics, ed. J. H. Miller (Cold Spring Harbor, NY: Cold Spring Harbor Laboratory Press).
Mohanan, N., Gislason, A., Sharma, P. K., Ghergab, A., Plouffe, J., Levin, D. B., et al. (2019). Quorum sensing and the anaerobic regulator (ANR) control polyhydroxyalkanoate (PHA) production in Pseudomonas chlororaphis PA23. FEMS Microbiol. Lett. 366:fnz223.
Nakata, K., Yoshimoto, A., and Yamada, Y. (1998). Correlation between autoinducers and rhamnolipids production by Pseudomonas aeruginosa IFO 3924. J. Ferment. Bioengine. 86, 608–610. doi: 10.1016/s0922-338x(99)80016-6
Nickzad, A., and Déziel, E. (2016). Adaptive significance of quorum sensing-dependent regulation of rhamnolipids by integration of growth rate in Burkholderia glumae: a trade-off between survival and efficiency. Front. Microbiol. 7:1215. doi: 10.3389/fmicb.2016.01215
Nickzad, A., Lépine, F., and Déziel, E. (2015). Quorum sensing controls swarming motility of Burkholderia glumae through regulation of rhamnolipids. PLoS One 10:e0128509. doi: 10.1371/journal.pone.0128509
Nitschke, M., Costa, S. G., and Contiero, J. (2005). Rhamnolipid surfactants: an update on the general aspects of these remarkable biomolecules. Biotechnol. Prog. 21, 1593–1600. doi: 10.1021/bp050239p
Ochsner, U. A., Koch, A. K., Fiechter, A., and Reiser, J. (1994). Isolation and characterization of a regulatory gene affecting rhamnolipid biosurfactant synthesis in Pseudomonas aeruginosa. J. Bacteriol. 176, 2044–2054. doi: 10.1128/jb.176.7.2044-2054.1994
Saegusa, H., Shiraki, M., Kanai, C., and Saito, T. (2001). Cloning of an intracellular Poly[D(-)-3-Hydroxybutyrate] depolymerase gene from Ralstonia eutropha H16 and characterization of the gene product. J. Bacteriol. 183, 94–100. doi: 10.1128/jb.183.1.94-100.2001
Schweizer, H. P., and Chuanchuen, R. (2001). Small broad-host-range lacZ operon fusion vector with low background activity. Biotechniques 31, 1258–1262. doi: 10.2144/01316bm06
Soberón-Chávez, G., Lépine, F., and Déziel, E. (2005). Production of rhamnolipids by Pseudomonas aeruginosa. Appl. Microbiol. Biotechnol. 68, 718–725.
Subsin, B., Chambers, C. E., Visser, M. B., and Sokol, P. A. (2007). Identification of genes regulated by the cepIR quorum-sensing system in Burkholderia cenocepacia by high-throughput screening of a random promoter library. J. Bacteriol. 189, 968–979. doi: 10.1128/jb.01201-06
Sun, W., Cao, J. G., Teng, K., and Meighen, E. A. (1994). Biosynthesis of poly-3-hydroxybutyrate in the luminescent bacterium, Vibrio harveyi, and regulation by the lux autoinducer, N-(3-hydroxybutanoyl)homoserine lactone. J. Biol. Chem. 269, 20785–20790.
Uchino, K., Saito, T., and Jendrossek, D. (2008). Poly(3-hydroxybutyrate) (PHB) depolymerase PhaZa1 is involved in mobilization of accumulated PHB in Ralstonia eutropha H16. Appl. Environ. Microbiol. 74, 1058–1063. doi: 10.1128/aem.02342-07
Urtuvia, V., Villegas, P., González, M., and Seeger, M. (2014). Bacterial production of the biodegradable plastics polyhydroxyalkanoates. Int. J. Biol. Macromol. 70, 208–213. doi: 10.1016/j.ijbiomac.2014.06.001
Woodcock, D., Crowther, P., Doherty, J., Jefferson, S., Decruz, E., Noyer-Weidner, M., et al. (1989). Quantitative evaluation of Escherichia coli host strains for tolerance to cytosine methylation in plasmid and phage recombinants. Nucleic Acids Res. 17, 3469–3478. doi: 10.1093/nar/17.9.3469
Zhao, F., Shi, R., Ma, F., Han, S., and Zhang, Y. (2018). Oxygen effects on rhamnolipids production by Pseudomonas aeruginosa. Microb. Cell Fact. 17:39.
Keywords: biosurfactants, Burkholderia thailandensis, PHA granules, quorum sensing, gene regulation, metabolites
Citation: Martinez S, Humery A, Groleau M-C and Déziel E (2020) Quorum Sensing Controls Both Rhamnolipid and Polyhydroxyalkanoate Production in Burkholderia thailandensis Through ScmR Regulation. Front. Bioeng. Biotechnol. 8:1033. doi: 10.3389/fbioe.2020.01033
Received: 26 May 2020; Accepted: 10 August 2020;
Published: 04 September 2020.
Edited by:
Manuel Benedetti, University of L’Aquila, ItalyReviewed by:
Daniel Segura, National Autonomous University of Mexico, MexicoIbrahim M. Banat, Ulster University, United Kingdom
Copyright © 2020 Martinez, Humery, Groleau and Déziel. This is an open-access article distributed under the terms of the Creative Commons Attribution License (CC BY). The use, distribution or reproduction in other forums is permitted, provided the original author(s) and the copyright owner(s) are credited and that the original publication in this journal is cited, in accordance with accepted academic practice. No use, distribution or reproduction is permitted which does not comply with these terms.
*Correspondence: Eric Déziel, ZXJpYy5kZXppZWxAaWFmLmlucnMuY2E=