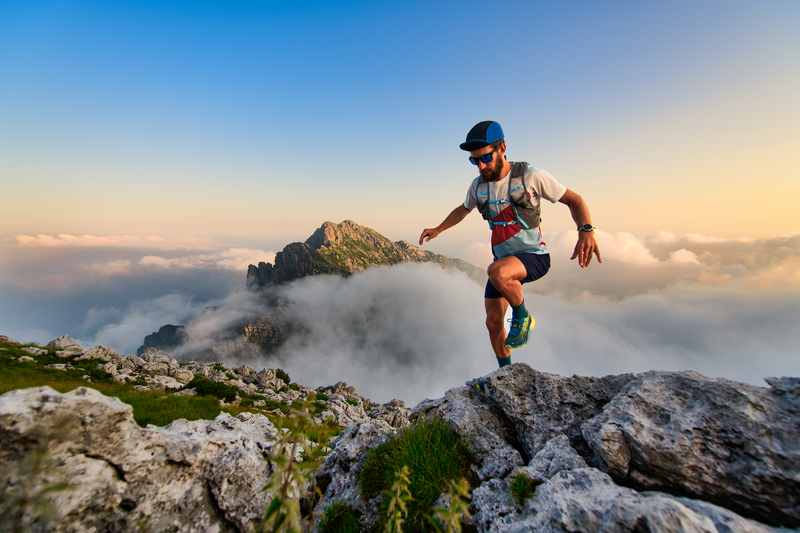
95% of researchers rate our articles as excellent or good
Learn more about the work of our research integrity team to safeguard the quality of each article we publish.
Find out more
REVIEW article
Front. Bioeng. Biotechnol. , 28 July 2020
Sec. Cell and Gene Therapy
Volume 8 - 2020 | https://doi.org/10.3389/fbioe.2020.00860
This article is part of the Research Topic Cell-Based Approaches for Modulating Cartilage and Bone Phenotype View all 11 articles
The infrapatellar fat pad (IFP) has until recently been viewed as a densely vascular and innervated intracapsular/extrasynovial tissue with biomechanical roles in the anterior compartment of the knee. Over the last decade, secondary to the proposition that the IFP and synovium function as a single unit, its recognized tight molecular crosstalk with emerging roles in the pathophysiology of joint disease, and the characterization of immune-related resident cells with varying phenotypes (e.g., pro and anti-inflammatory macrophages), this structural complex has gained increasing attention as a potential therapeutic target in patients with various knee pathologies including osteoarthritis (KOA). Furthermore, the description of the presence of mesenchymal stem/stromal cells (MSC) as perivascular cells within the IFP (IFP-MSC), exhibiting immunomodulatory, anti-fibrotic and neutralizing activities over key local mediators, has promoted the IFP as an alternative source of MSC for cell-based therapy protocols. These complementary concepts have supported the growing notion of immune and inflammatory events participating in the pathogenesis of KOA, with the IFP/synovium complex engaging not only in amplifying local pathological responses, but also as a reservoir of potential therapeutic cell-based products. Consequently, the aim of this review is to outline the latest discoveries related with the IFP/synovium complex as both an active participant during KOA initiation and progression thus emerging as a potential target, and a source of therapeutic IFP-MSCs. Finally, we discuss how these notions may help the design of novel treatments for KOA through modulation of local cellular and molecular cascades that ultimately lead to joint destruction.
The infrapatellar fat pad (IFP), also known as Hoffa’s fat pad, is a cylinder-like piece of adipose tissue that sits posterior to the patella and fills the anterior knee compartment (Dragoo et al., 2012). Though the function of the IFP has not yet been fully defined, studies have shown that the IFP plays an important biomechanical role within the knee (Bohnsack et al., 2004; Gallagher et al., 2005). In addition, recent evidence has shown that the IFP in concert with the synovium participates in the pathogenesis and progression of various pathologies within the knee joint such as osteoarthritis (KOA) (Benito et al., 2005; Scanzello and Goldring, 2012; Sokolove and Lepus, 2013; Lieberthal et al., 2015; Felson et al., 2016; Favero et al., 2017; Mathiessen and Conaghan, 2017), given that these structures serve as sites of immune cell infiltration and origin of pro-inflammatory (e.g., IFNγ, TNFα and IL1β) and articular cartilage degradative (e.g., MMPs) molecules (Bondeson et al., 2010; Kalaitzoglou et al., 2017; Li et al., 2017). On the other hand, they may be related with repair attempts after injury, due to the presence of mesenchymal stem/stromal cells (MSCs) within both the IFP (IFP-MSC) (Garcia et al., 2016a; Tangchitphisut et al., 2016; Kouroupis et al., 2019a) and the synovium (sMSC) (Mizuno et al., 2018; To et al., 2019) exhibiting disease-modifying capacities (Caplan and Correa, 2011; Stagg and Galipeau, 2013; Uccelli and de Rosbo, 2015; Galipeau et al., 2016). Consequently, the IFP and synovium engage not only in amplifying local pathological responses, but also act as a reservoir of disease-modifying cellular products, promoting them as potential novel targets in joint disease (Attur et al., 2010).
IFP-MSCs have generated increased interest in recent literature due to their easy accessibility compared to other stem cell sources such as bone marrow and adipose tissue (AT), while displaying similar multipotency, growth potential, and immunomodulatory abilities (Sun et al., 2018). Their relative ease of isolation compared to bone marrow aspiration (thus removing the potential surgical complications seen with aspiration) have made them a popular resource for experimentation and regenerative medicine (Vilalta et al., 2008; Mizuno et al., 2012; Siciliano et al., 2016). However, because of its relatively newfound MSC population, current literature has re-focused on updating the knowledge of IFP anatomy, function, and most importantly its cellular composition beyond MSC. This has not only led to extensive investment in the IFP’s potential for regenerative medicine in Orthopedics, but also the role the IFP may play in certain pathological processes including KOA. For example, more established theories believe that the IFP communicates with the joint via the synovium and may play a role in cartilage and/or bone regeneration via the secretion of adipose tissue derived growth factors (Jiang et al., 2019). However, a shift in our understanding of the IFP anatomy and pathophysiology demonstrates not only that the IFP and the synovium constitute one structural and functional unit (Macchi et al., 2018), but that IFP-MSCs can regulate resident immune cell infiltration and resident macrophages thus acting as local immunomodulatory players.
Therefore, the goal of this review is to outline the latest developments of the IFP/synovium complex as a tissue that actively participates in joint homeostasis and disease, while harboring cellular elements that can be harnessed for therapeutic cell-based therapy protocols. In addition, updates regarding recent discoveries in anatomy, cellular composition, function, isolation and harvest, imaging, role in certain pathologies of the knee (most importantly modulation of inflammation in the joint), current therapeutic uses, and future perspectives and goals for IFP use will be discussed.
The IFP is located deep to the patella and occupies the space between the patellar tendon, femoral condyle, and tibial plateau. It attaches to the lower border of the patella, the intercondylar notch within the femur via the ligamentum mucosum, the periosteum of the tibia, and the anterior horn of both menisci (Gallagher et al., 2005). Of note, recent study of IFP anatomy has demonstrated previously undiscovered attachments to the deep quadriceps muscle, which may assist with IFP motion during walking (Woodley et al., 2012).
Although the IFP is intracapsular, it remains extrasynovial despite its constant contact with the synovium (Clockaerts et al., 2010). Increasing evidence has demonstrated that the IFP develops as an outgrowth of the synovial tissue with regard to structure and functionality, which suggests extensive communication between the IFP and the synovium and joint capsule (Figure 1) (Ioan-Facsinay and Kloppenburg, 2013). Furthermore, Macchi et al. (2018) have concluded that the IFP and the synovium should be viewed as one anatomo-functional unit rather than two distinct structures that simply communicate with one another. The authors justified this definition because recent anatomical studies demonstrated the insertion of infrapatellar and medial synovial plicae directly onto the IFP, which suggests that the IFP may not be extrasynovial, but rather an extension of the synovium outside of the joint capsule (Macchi et al., 2018). Nevertheless, the intimate relationship between the synovium and IFP appears highly important in the release of growth factors and cytokines that help to regulate the molecular environment within the joint.
Figure 1. Demonstration of the extensive molecular cross-talk and important cellular components between the IFP and the synovium within the joint capsule which are responsible for inflammation/fibrosis.
The extensive anastomotic vascular network near the IFP involves a combination of the superior and inferior geniculate arteries, the latter which passes through the IFP before supplying the patella. This matrix helps support and promote IFP-MSC proliferation, especially during injury and inflammation. It has also been hypothesized that this network is sufficient to protect the IFP during extensive surgical or arthroscopic procedures that lead to significant manipulation of the structure itself (Kohn et al., 1995).
Innervation to the IFP is just as extensive as its vasculature and typically traverses the same course across the entire tissue. Previous studies have confirmed that posterior articular branches from the tibial, saphenous, recurrent peroneal, and common peroneal nerve provide most of the innervation, however Gardner et al. recently described branches arising from the saphenous and obturator nerves as well (Freeman and Wyke, 1967; Kennedy et al., 1982). This collective peripheral sensory nociceptive innervation pattern (dense in parts of the IFP and synovium) is mediated by nerve fibers equipped with the neurotransmitter Substance P which runs separately but in parallel to sympathetic fibers and it is implicated in knee pain transmission. Additionally, within the IFP tyrosine hydroxylase (TH)-positive sympathetic fibers modulate nociception/pain signaling in sympathetic neurons, through interacting with Substance P-positive fibers (Dragoo et al., 2012; Brumovsky, 2016).
The most prevalent cell is the adipocyte, which is not only responsible for the IFP’s metabolism, but also endocrine and paracrine functions within the knee joint (Coelho et al., 2013; do Amaral et al., 2017). Importantly, adipose cells secrete cytokines, interferons, adipokines, and growth factors, all of which exerting local signaling effects on articular cartilage and synovial cells (Clockaerts et al., 2010).
As shown in Figure 1, other important cellular components of the IFP include fibroblasts, responsible for the production of extracellular matrix, and in less quantities resident monocytes, mast cells, lymphocytes, and perhaps most importantly macrophages (de Lange-Brokaar et al., 2012; Belluzzi et al., 2019; Kouroupis et al., 2019a). Barboza et al. (2017) have demonstrated that macrophages not only permanently reside within the IFP, but lie without phenotypic polarization as either classical M1 or alternative M2 variants until conditions promote their activation and subsequent conversion, such as inflammation.
Resident IFP macrophages are activated by a variety of interleukins and interferons secreted from other resident and infiltrating immune cells and adipose cells within the IFP. When converted to M1 macrophages, the IFP begins secreting vast amounts of pro-inflammatory cytokines, catabolic factors, and adipokines, and with prolonged periods of time, the IFP can also release pro-fibrotic mediators such as CTGF that may contribute to KOA progression (Figure 2) (Clockaerts et al., 2010). This occurs due to tight molecular crosstalk between synovial, IFP, and systemic inflammatory mediators. Consequently, these macrophages are now the target of studies assessing their release of pro-inflammatory molecular mediators (Kouroupis et al., 2019a). On the other hand, also shown in Figure 2, alternatively differentiated M2 macrophages exert anti-inflammatory effects, serving as counterbalance to their M1 cohorts by suppressing their proliferation and inflammatory signaling.
Figure 2. Schematic showing the intricate balance between M1 and M2 macrophages within the IFP and synovium. Differentiated M2 macrophages exhibit anti-inflammatory effects and preserve the health of the joint, whereas an imbalance favoring M1 macrophages promotes the IFP’s secretion of pro-inflammatory cytokines and catabolic factors that are seen within an inflamed/fibrotic knee.
Finally, the IFP harbors a population of MSC (IFP-MSC), which will be discussed in detail later (see section “Synovium-Derived MSC”) as a potential therapeutic tool for cell-based therapy protocols.
Within the intimal synovial lining reside predominantly two synovial cell types: type A (Macrophage-Like synoviocytes – MLS) and type B (Fibroblast-Like-Synoviocytes – FLS) (Tu et al., 2018). The type B synoviocytes, thought to be descendants of cells of mesodermal origin, are far more abundant and display typical fibroblast markers such as surface marker Thy-1 (CD90) and integrins like ICAM1 while secreting specialized matrix constituents including hyaluronan and Type IV and V collagens (Roelofs et al., 2017; Tu et al., 2018). Thus, it can be argued that type B synoviocytes more so than type A counterparts are responsible for maintenance of synovial homeostasis.
Type B synoviocytes are subject to cytokine and growth factor regulation, which can dictate a pro or anti-inflammatory state depending on which factors are expressed in the surrounding synovial environment (Orr et al., 2017). In a chronic inflammatory state, these cells primarily act in a pro-inflammatory role. For example, in patients with rheumatoid arthritis, fibroblasts have been shown to respond to and secrete a combination of TNF-alpha, IL-1, IL-6, and granulocyte-macrophage colony-stimulating factor (GM-CSF), while expressing a multitude of toll-like receptors in order to amplify T-Cell response to TLR activation (Ospelt, 2017). In regard to patients with OA, type B synoviocytes are particularly sensitive to TLR-2, TLR-3, and TLR-4 ligands due to the active expression of CD14, a co-receptor for TLRs (Nair et al., 2012). Type B synoviocytes also secrete a multitude of chemoattractants, including CCL2, CCL5, CCL8, CXCL5, and CXCL10, designed to attract monocytes and macrophages, both resident and peripheral in nature (Bartok and Firestein, 2010). Finally, upon stimulation of TLR-3, these synoviocytes produce large quantities of IL6, B-Cell Activating Factor and proliferation-inducing ligand (Mata-Essayag et al., 2001), promoting the maturation, survival, and antibody production by B cells (Bombardieri et al., 2011). These findings suggest that type B synoviocytes, though non-immune in nature, play a key role in autoimmune and OA disease development due to their inflammatory properties.
Type B synoviocytes can also produce a wide variety of anti-inflammatory factors such as TGF-beta, Type 1 interferons, VEG-F, indoleamine 2,3-dioxygenase (IDO enzyme), and certain prostaglandins, though some of these factors depending on concentration and exposure time may also be pro-inflammatory (Tu et al., 2018). However, the ability to harness the anti-inflammatory properties of these cells remains unknown. The recent proposition that type B synoviocytes may also contain multiple subtypes within the synovial lining which determine their secretory properties provides a future avenue for studies attempting to fully elucidate the role of these cells in arthritis development or modulation (Frank-Bertoncelj et al., 2017).
On the other hand, type A synoviocytes are far less known due to the limited number of these cells in vivo and their poor proliferative potential in vitro. They constitute resident macrophages, derived from both embryonic hematopoietic precursors and from bone marrow, although their definitive origin is still elusive (Tu et al., 2018). These resident macrophages need to be discriminated from monocytes/macrophages that extravasate into the synovium from peripheral circulation after injury or in disease. Nevertheless, it has been established that they have pro-inflammatory tendencies while exhibiting an intimate crosstalk with type B synoviocytes, especially in disease (Tu et al., 2018, 2019). Type A synoviocytes secrete soluble CD14, IL-1β, and TNFα, further potentiating the pro-inflammatory properties of type B synoviocytes and CD4 T helper cells. They also induce monocyte/macrophage-derived osteoclast activity via RANK-L secretion resulting in enhanced bone resorption (Yoshitomi, 2019). It is interesting to note given the above pro-inflammatory properties that the presence and activity levels of these tissue-resident macrophages significantly correlates with advanced stages of OA and poorer clinical outcome scores (Kriegova et al., 2018; Gomez-Aristizabal et al., 2019).
Similar to the IFP, the synovium contains a small population of cells compatible with MSC (sMSC), which will be discussed in detail in section “MSC-Induced Immunomodulation: Focus on Macrophage Polarization.”
Beyond the proximity the IFP and synovium share, there are molecular interactions between both components that support their view as a single anatomical and functional unit. For instance, both IFP and adjacent synovium experience similar structural effects in KOA, including increased inflammatory infiltration, vascularization, and thickness (Favero et al., 2017). The IFP has been shown to release prostaglandin F2a (PGF2α), IL-6, IL-8, and TNFα, inducing a profibrotic effect on the synovial membrane (Bastiaansen-Jenniskens et al., 2013; Eymard et al., 2014). Specifically, Bastiaansen-Jenniskens et al. (2013) cultured human fibroblast -like synoviocytes (type B) obtained from OA patients in conditioned medium derived from IFP tissue with and without inhibitors of TGFβ/activin receptor-like kinase 5 or PGF2α for 4 days in vitro. IFP derived conditioned medium not only increased the migration and proliferation of synoviocytes but also resulted in profibrotic changes including Collagen production and PLOD2 gene expression upregulation. Collagen production in synoviocytes was directly associated with secreted PGF2α levels in IFP derived conditioned medium. On the other hand, as the IFP is mainly composed of adipocytes, it results as a major source of various adipocyte-derived inflammatory mediators including lipids. Previous studies indicated that IFP-derived adipocytes, via secreted lipids, are able to modulate infiltrating macrophages and CD4+ T cells into the OA synovium (Ioan-Facsinay et al., 2013; Klein-Wieringa et al., 2013). In adipocyte-derived conditioned medium obtained from IFP, Ioan-Facsinay et al. (2013) identified free fatty acids that enhance CD4+ T cell proliferation and their capacity to produce IFN-γ. Additionally, free fatty acids secreted from IFP adipocytes can reduce the secretion of IL-12p40 cytokine by macrophages (Klein-Wieringa et al., 2013). According to previous studies (reviewed in Cooper and Khader, 2007), IL-12p40 is a chemoattractant molecule for macrophages, and which promotes inflammation and fibrosis. Furthermore, Mustonen et al. (2019) identified distinct fatty acid signature for IFP in OA and rheumatoid arthritis (RA) patients. Compared to RA, OA patients have higher total n-6, 20:4n-6 and 22:6n-3 polyunsaturated fatty acids (PUFA), and higher product/precursor ratios of n-3 PUFA. In general, n-6 PUFA such as 20:4n-6 (arachidonic acid) are precursors to pro-inflammatory mediators, whereas n-3 PUFA such as 22:6n-3 (docosahexaenoic acid) have anti-inflammatory/anti-catabolic effects (Brouwers et al., 2015). Overall, the major alterations in OA and RA joints compared to control healthy knees are an increase in monounsaturated fatty acids and a simultaneous decrease in n-6 PUFA, effects that should be further investigated in future studies (Mustonen et al., 2019).
Just as IFP influences synovium, Clements et al. (2009) demonstrated that extensive synovial proliferation and fibrosis led to marked loss of adipocytes within the IFP. Specifically, synovium secretion of pro-inflammatory cytokine IL-1β has been associated with catabolic effects in initiation and progression of OA. A previous study showed that exposure of IFP explants from OA patients to IL-1β in vitro result in secretion of large amounts of pro-inflammatory cytokines such as PTGS2, IL-1β, MCP-1, and IL-6. These effects can be partially ameliorated by a PPARα agonist (Clockaerts et al., 2012). Thus, recent literature has not only demonstrated extensive communication between both the IFP and synovium, but that this communication can accelerate development and progression of KOA, as elaborated below.
With the cellular composition of the IFP better elucidated and the occurrence of immune and inflammatory events within the IFP, its role in the pathophysiology of KOA is becoming the focus of multiple studies. For instance, Heilmeier et al. (2019) demonstrated that following ACL acute injury the IFP rapidly releases inflammatory cytokines that promote a sustained inflammatory response lasting for months. Consequently, various theories have emerged explaining the IFP’s role in the regulation of local inflammatory cascades including adipocytes, and more recently resident macrophages as key targets (in the development of post-traumatic OA). We next explore the strengths and limitations of each prevailing theory.
As previously discussed, adipocytes are capable of secreting certain molecular markers and products capable of initiating a local inflammatory response. Given that obesity represents a chronic inflammatory state, many studies have focused on the role of adipocytes as contributors for accelerated development of KOA (Balistreri et al., 2010; Bravo et al., 2019; Jiang et al., 2019). Consistent with this theory, the discovery of IL-1β and other pro-inflammatory cytokine production, together with matrix metalloprotease expression within KOA cartilage by adipocytes, suggests that the IFP may be intimately linked to KOA (Clockaerts et al., 2010; de Boer et al., 2012; Beekhuizen et al., 2013). Furthermore, leptin and adiponectin have been shown to be primarily secreted by IFP adipocytes into synovial fluid, with a key role influencing cartilage and synovial metabolism (Dumond et al., 2003; Toussirot et al., 2007). Therefore, the association of leptin to obesity and inflammation led to the belief that obesity itself plays a role in inducing IFP adipocyte inflammatory propagation and accelerated KOA progression (Ioan-Facsinay and Kloppenburg, 2013). Leptin has been shown to promote production of articular cartilage proteoglycans and collagen while stimulating insulin-like growth factor-1 and other growth factors that subsequently enhance chondrocyte proliferation (Bao et al., 2010, 2014). Lipid-mediated lipoxin A4, which can prevent cartilage degeneration in the knee, is also secreted by IFP adipocytes (Bastiaansen-Jenniskens et al., 2012; Gierman et al., 2013). Leptin facilitates the activation of immune cells, particularly M1 macrophages, via interferon release and nitric oxide production (Matarese et al., 2007). Moreover, recent literature suggests that obese patients with OA have either no difference in the number of M1 macrophages within the IFP, or may even have an increased number of M2 macrophages, compared to that of lean patients (de Jong et al., 2017). Lastly, even with M1 macrophages present within the IFP, the classic M1 macrophage mediated inflammation that usually occurs in abdominal adipose tissue as seen with obesity cannot be recapitulated, suggesting that IFP adipocytes are subject to distinct spatial-temporal metabolic regulation (Barboza et al., 2017).
An alternative mechanism by which obesity may affect the IFP during the progression of KOA is through altered joint mechanics. Ballegaard et al. (2014) have shown that obese patients with KOA demonstrated significantly increased inflammatory signaling within the IFP measured by contrast-enhanced perfusion variables on MRI. Cowan et al. (2015) also demonstrated that patients with patellofemoral OA have a greater IFP volume on MRI compared to healthy knees. Because the IFP resides in a tight anatomical space, the authors suggested that increased IFP volume was an inducer of inflammation, leading to secretion of synovial inflammatory factors (Clements et al., 2009). Therefore, in this alternate hypothesis, adipocyte induced inflammation within the joint may be due to factors other than obesity (King et al., 2013). However, OA also occurs in non-weight bearing joints such as the hand, suggesting that the metabolic effects of obesity may play a greater role than altered joint mechanics (Losina et al., 2011; Yusuf, 2012; Bliddal et al., 2014).
Overall, though there is an established link between obesity and KOA, the explanation that the IFP propagates KOA development because of its primarily adipocyte-based composition remains controversial. Because the IFP has a distinct environment compared to abdominal adipose tissue, the role of this specific adipocyte population in KOA remains unclear and warrants continued investigation, as obesity related features seen in visceral adipose tissue are not present within the IFP of KOA patients (de Jong et al., 2017).
Adipocytes are not the only cellular component with potential to induce or enhance inflammation locally. The IFP and synovium are populated by macrophages, historically viewed as cells that maintain tissue homeostasis with crucial roles in early and late phases of response to injury, while more recently associated with various pathologies (Caspar-Bauguil et al., 2005; Mathis, 2013; Ginhoux and Jung, 2014). Macrophages have distinct origins resulting in significant heterogeneity, beyond the known M1 (classical pro-inflammatory) and M2 (alternative anti-inflammatory) polarization phenotypes (Ginhoux and Jung, 2014; Paul et al., 2015; Wu et al., 2020). A special population of tissue resident macrophages derive from embryonic precursors, exhibit self-renewal, and replenish after injury independently from circulating bone marrow-derived Ly6CHigh monocytes (Ly6CHigh is a murine marker with no current human ortholog identified) (Davies et al., 2011; Gentek et al., 2014; Ginhoux and Guilliams, 2016; Zhao et al., 2018). IFP and synovium show such resident populations with comparable immune cell profiles (Klein-Wieringa et al., 2016), also susceptible to polarize to M1 or M2 phenotypes depending on the status of the joint (Barboza et al., 2017; Sun et al., 2017; Tu et al., 2018; Wu et al., 2020).
Resident M1 pro-inflammatory macrophages are theorized to be an important driver of the host low grade chronic inflammatory state (Mathis, 2013; Kandahari et al., 2015). In fact, patients with KOA show a propensity for the M1 classical phenotype within the IFP/synovium complex, resulting in cytokine, interferon, and TNF-alpha secretion (Klein-Wieringa et al., 2011; Wu et al., 2020). Recent evidence suggests that activation of the mammalian target of rapamycin (Kuptniratsaikul et al., 2009) pathway also plays a role in M1 macrophage polarization and progression of KOA in animal models (Fernandes et al., 2020). Nevertheless, the existence of resident macrophages within the IFP exhibiting an M1 phenotype independent of the presence of local inflammation confirms their potential participation as initiators of KOA (Wu et al., 2020). Thus, the propagation of KOA is not reliant solely on immune cell extravasation, but rather on resident cells from within the IFP/synovium complex, though the precise turning point that leads to KOA still remains unknown.
The aforementioned molecular markers that induce pro-inflammatory states do so in part by promoting extravasation of circulating immune cells into the IFP and synovium. The secretion of related prostaglandins, as well as IL-6 and IL-8 promote the extravasation of immune cells by attracting lymphocytes to the endothelium promoting their migration into the surrounding IFP and synovium (Schnoor et al., 2016). Substance P, a product of nociceptive nerve fibers that transmits pain signals while also modulates local inflammatory processes (i.e., neurogenic inflammation), has also been shown to induce vasodilation of peripheral vessels, thus promoting the extravasation of immune cells from peripheral circulation into surrounding tissue (Clockaerts et al., 2010).
Apinun et al. (2016) described the presence of peripheral CD8 T cells, macrophages, B cells, and mast cells within the IFP of patients with OA undergoing TKA. According to the authors, the infiltration of these cells trended with disease severity (patients with severe radiographic KOA had more CD8 T cell infiltration than patients with mild KOA), thereby leading the authors to conclude that the infiltration of circulating immune cells to the IFP and synovium contribute to disease progression and severity. In addition, Klein-Wieringa et al. (2016) showed that peripheral CD4 T cells also infiltrate the IFP and synovium in a severely osteoarthritic population, and their presence correlated with pain scores (R = 0.53, p < 0.01, N = 76 patients). Thus, pro-inflammatory cells within the IFP and synovium not only promote localized inflammation with resident immune cells, but also promote extravasation of circulating ones potentiating the inflammatory process that are associated with poorer clinical and radiographic outcomes.
The IFP is best visualized on non-contrast magnetic resonance imaging (MRI) in the sagittal plane and intensity of signal alterations have recently been correlated with anterior knee pain and cartilage loss by Han et al. (OR 1.23, p < 0.05, N = 374), supporting the link between changes in IFP and KOA development (Hill et al., 2007; Roemer et al., 2009; Han et al., 2016). Though non-contrast enhanced MRI is the gold standard, contrast-enhanced MRI imaging has recently been employed to show correlations between histological synovial infiltrate and hyperplasia and KOA progression (R = 0.63, p < 0.001, N = 30) (Loeuille et al., 2011). Crema et al. (2013) have shown that peri-patellar synovial thickness on non-contrast-enhanced MRI images could be the culprit for KOA related pain and not the changes in signal alterations within the IFP itself.
Interestingly, the size of the IFP may play an important role in KOA risk and symptom development and intensity. Pan et al. (2015) demonstrated that decreased IFP volume in older women compared to men was significantly associated with increased total knee pain, pain at rest and during movement, and cartilage damage. However, the authors also found that total IFP maximal area appears to have a protective role for knee symptoms in older adult females, but not men. IFP signal intensity was later linked to size of IFP by Han et al. (2016), which provides support for continued use of non-contrast enhanced MRI as the gold standard. Recently, Fontanella et al. (2019) reported changes in the morphometry (i.e., reduced volume, depth, and femoral and tibial arc lengths) and increase of the MRI hypointense signal in the IFP from patients with moderate and end-stage KOA compared to healthy controls. Despite contrasting results from various groups, the description of morphological changes in the IFP by MRI warrants continued investigation into how imaging may play a future in predicting KOA risk or progression.
In 1996 a pioneering study by Maekawa et al. (1996) firstly described a type of fibroblastic cells possessing ‘stem cell-like’ characteristics in synovial tissue near the IFP. Those cells reside mostly in the perivascular space surrounding vessels of small caliber and involved in the fibronectin and laminin production. Recent studies have isolated and phenotypically characterized IFP-MSC positive for CD9, CD10, CD13, CD29, CD44, CD49e, CD59, CD73, CD90, CD105, CD106, CD146, CD166, NG2, and CXCR4 markers, while negative for CD34, CD56, CD200, CD271, 3G5, LepR and STRO-1 markers (Wickham et al., 2003; Khan et al., 2008; Garcia et al., 2016a; Hindle et al., 2017; Kouroupis et al., 2019a). IFP-MSC characteristically have low or no HLA-DR expression, yet a total absent expression of co-stimulatory molecules CD40, CD80, and CD86 (Garcia et al., 2016a; Kouroupis et al., 2020). In a recent study, Hindle et al. identified two distinct IFP-MSC subpopulations within the IFP, characterized as pericytes (CD31–CD45–CD34–CD146+) and adventitial cells (CD31–CD45–CD34+CD146–), representing 3.8 and 21.2% of the IFP stromal vascular fraction, respectively (Hindle et al., 2017).
In general, IFP-MSC have comparable proliferative potential to other MSC types (Dragoo et al., 2003; Jurgens et al., 2009). In comparative studies, IFP-MSC were reported to possess similar growth kinetics to bone marrow-derived MSC (BM-MSC) (English et al., 2007) and higher proliferation to donor-matched synovial fluid-MSC (Garcia et al., 2016a). However, in order to generate clinically relevant cell numbers, IFP-MSC growth rate can be accelerated by various in vitro culturing conditions such as human platelet lysate (hPL) or chemically-reinforced (Ch-R) media expansion, serum and growth factor (TGF-β and FGF-2) stimulation and hypoxia exposure (Marsano et al., 2007; Khan et al., 2008; Buckley and Kelly, 2012; Liu et al., 2012; O’HEireamhoin et al., 2013). Importantly, our group recently showed that hPL and Ch-R formulations can effectively replace FBS to expand IFP-MSC, enhancing phenotypic and functional attributes (Kouroupis et al., 2020).
IFP-MSC multipotentiality toward chondrogenic, osteogenic, and adipogenic lineages has been demonstrated by previous studies (reviewed in Sun et al., 2018). However, there is evidence showing that MSC differentiation capacity is strongly related to the tissue of origin. Therefore, due to the intra-articular localization of IFP tissue, and their anatomical proximity to articular cartilage, it is not surprising that IFP-MSCs exhibit strong chondrogenic differentiation capacity both in vitro and in vivo (Dragoo et al., 2003; Khan et al., 2008; Lee et al., 2008; Jurgens et al., 2009; Buckley et al., 2010; Almeida et al., 2014, 2015, 2016; Liu et al., 2014; Ye et al., 2014). Specifically, in vitro IFP-MSC show stronger chondrogenic differentiation capacity than adipose- derived-, BM-, and UC-MSC (Ding et al., 2015). Others however report that they possess at least comparable chondrogenic capacity to BM-MSC (English et al., 2007) but inferior to native chondrocytes and perivascular IFP-MSC (Marsano et al., 2007; Vinardell et al., 2011; Garcia et al., 2016b; Hindle et al., 2017). On this basis, studies have shown that heterogenous IFP-MSC selection for specific subpopulations may result in further enhanced chondrogenic differentiation capacity. Moreover, perivascular IFP-MSC (CD31–CD45–CD34–CD146+) generate significantly more extracellular matrix than heterogenous “crude” IFP-MSC cultures (Hindle et al., 2017). Also, others reported the positive correlation of CD49c expression of donor-matched chondrocytes, BM-MSC, FP-MSC, and synovial fluid MSC with their chondrogenic capacities in vitro (R = 0.2, p < 0.018, N = 5 samples) (Garcia et al., 2016b). In in vivo settings, freshly isolated uncultured CD44+ IFP-MSC seeded into a TGF-β3 ECM-derived scaffold and subcutaneously implanted in nude mice, are capable of producing a cartilage-like tissue rich of sGAG and Collagen type II (Almeida et al., 2015). Therefore, the selection of specific IFP-MSC subpopulations may result in improved in vivo chondrogenesis.
Given their high proliferation rate and superior chondrogenic differentiation capacity, IFP-MSC may be considered a suitable candidate cell to engineer cartilaginous constructs to resurface focal defects or even an entire OA joint (Liu et al., 2014; Ye et al., 2014; Prabhakar et al., 2016). In that regard, Liu et al. (2014) showed that IFP-MSC obtained from both healthy and OA individuals and cultured on PLLA fiber membranes for 6 weeks can generate robust, flexible cartilage-like grafts of clinically relevant dimensions (≥2 cm in diameter). Of note, the authors did note that donor age variability may affect the robustness of the cultured IFP-MSCs, supporting the idea that the outcome of future IFP-MSC treatments may be substantially different in certain patient populations.
However, the main limitation of MSC-based cartilage constructs is that they progress in differentiation reaching an ultimate hypertrophic phenotype and finally undergoing endochondral ossification in vivo (Farrell et al., 2009, 2011; Scotti et al., 2013; Correa et al., 2015; Feng et al., 2018). To overcome this limitation, co-culture of IFP-MSC with articular chondrocytes in hybrid structures result in a phenotypically stable layer of articular cartilage with reduced mineralization upon implantation in nude mice for 8 weeks (Mesallati et al., 2015). The same group demonstrated that self-assembled IFP-MSC on top of articular cartilage agarose gels result in higher accumulation of sGAG and therefore strongly enhance the development of articular cartilage constructs (Mesallati et al., 2017). Although, articular cartilage tissue engineering is a promising approach, a significant barrier is the generation of constructs with clinically relevant dimensions and in a time/cost efficient manner ready-to-use for in vivo implantation, especially for large compromised surfaces such as in OA. In more simplified approaches, IFP-MSC are directly injected intra-articularly solely or embedded in hydrogel-based delivery systems with and without growth factors. Toghraie et al. (2011) showed that a single dose of intra-articularly injected IFP-MSC result in decreased cartilage degeneration, osteophyte formation, and subchondral sclerosis 20 weeks later in a rabbit OA model. Recently, Muttigi et al. (2018) directly injected IFP-MSC embedded in matrillin-3 (an essential ECM component of cartilage) and 2% hyaluronic acid in an osteochondral defect rat model, with the reasoning that Matrilin-3 alone enhances Collagen II and aggrecan expression in chondrocytes while downregulating matrix degrading enzymes such as matrix metalloproteinase-13 (MMP-13). According to the authors, Matrillin-3, when is co-delivered with IFP-MSC, indeed resulted in greatly enhanced Collagen type II and aggrecan productions, whereas the regenerated defect site possesses similarities with native cartilage (thickness, chondrocyte clustering, and hyaline-like morphology). Overall, IFP-MSC due to their advantageous intra-articular anatomical localization and ease for harvesting along with their enhanced chondrogenic capacity may be an attractive approach for addressing articular cartilage degeneration in OA.
Our group recently reported that intra-articularly injected CD10-rich IFP-MSC reverted induced synovitis and IFP fibrosis in rats which are seen in early OA (Kouroupis et al., 2020). Interestingly, the degree of in vivo efficacy is associated with the degree of expression of CD10 and degradation of Substance P, a local mediator of transmitting pain signals and regulator of neurogenic inflammation.
Located within the synovial intima and sub-intima lie distinct MSC populations similar to IFP-MSC, with an origin still debated (Li et al., 2019; Sivasubramaniyan et al., 2019). Though not populous within the tissue, these cells maintain high proliferative capacity and can differentiate into osteoblasts, adipocytes, and chondrocytes in vitro (Ferro et al., 2019). The origin of synovium-derived MSC (sMSC) in the synovial lining is still not fully defined, with groups supporting the notion of MSC infiltrating from resident vasculature or even from the neighboring bone marrow. However, two recently reports strongly support the hypothesis of the embryonic origin of synovium at the joint interzone, by showing that single or double positive Prg4-lineage and Gdf5-lineage cells, and associated sMSC as contributors to tissue homeostasis and repair in adult life (Decker et al., 2017; Roelofs et al., 2017).
Besides a similar overall immunophenotype of sMSC compared with other MSC (De Bari et al., 2001; Sakaguchi et al., 2005; Hermida-Gomez et al., 2011), CD271, a highly expressed markers in freshly isolated BM-SMC, is absent in healthy sMSC (Karystinou et al., 2009), yet expressed in cells isolated from OA patients (Hermida-Gomez et al., 2011). A topographic analysis of synovium and a full phenotypic description of sMSC are presented in our previous review (Kouroupis et al., 2019b). According to reports, sMSC show a greater proliferation rate and stronger chondrogenic capacity than BM- and adipose-derived MSC whereas they exhibit a reduced hypertrophic differentiation potential (Kubosch et al., 2018).
Previous studies have demonstrated that sMSC and IFP-MSC show comparable chondrogenic differentiation capacity (Mochizuki et al., 2006). In a comparative study of three different MSC types, Mochizuki et al. indicated that sMSC and IFP-MSC have similar chondrogenic capacity between older and younger donors but higher compared to donor-matched subcutaneous fat-derived MSC (Mochizuki et al., 2006). However, Vinardell et al. (2012) reported that when sMSC and IFP-MSC are embedded in agarose hydrogel constructs and chondrogenic induced for 49 days in vitro, sMSC accumulate higher levels of sGAG and Collagen than IFP-MSC. Similarly, to IFP-MSC, sMSC expansion in hPL medium result in increased proliferation rate but lower chondrogenic capacity compared to sMSC grown in the presence of FBS (Nimura et al., 2008).
In preclinical settings, Koizumi et al. (2016) isolated sMSC from both OA and RA patients and assessed their cartilage repair capacity using a scaffold-free tissue engineering approach. Interestingly, 8 weeks post-implantation both OA or RA sMSC-treated groups showed hyaline cartilage-like repair and in general higher histological scores compared to the untreated rats. In addition, various studies using animal sMSC (rabbit-, murine-, equine-, porcine-harvested synovium) showed that sMSC groups are superior to control groups in treating full thickness chondral lesions (To et al., 2019). Therefore, sMSC show good reparative capacity of chondral lesions and no adverse effects after implantation in vivo.
During early phases of OA, both IFP and synovium become infiltrated by immune cells, including T cells, B cells, monocytes/macrophages, and mast cells (Pelletier et al., 2001; Sokolove and Lepus, 2013; Ioan-Facsinay and Kloppenburg, 2017; Kalaitzoglou et al., 2017). These infiltrates complement the local resident cells, especially macrophages, which as seen in Figure 3 polarize into a pro-inflammatory classical M1 phenotype (discussed above in section “Role of IFP/Synovium Resident Macrophages”). MSC exerts immunomodulatory effects, simultaneously influencing multiple immune cells through different mechanisms including cell-cell contact, soluble factors, and released extracellular vesicles (e.g., exosomes). The specific effects of MSC on T cells, B cells and other immune cells have been reviewed extensively elsewhere (Djouad et al., 2005; Hagmann et al., 2013). Herein, we emphasize on the current knowledge regarding the interactions with macrophages given their pivotal role in the initiation and progression of the disease as source of inflammatory and degradative mediators.
Figure 3. Macrophages within the IFP can polarize into M1 (pro-inflammatory) or M2 (anti-inflammatory, tissue repair) variants. Both variants are associated with a multitude of unique surface markers with varying levels of expression that allow for proper identification.
Numerous studies have explored macrophages as potential therapeutic targets, including their pharmacological depletion from synovium and IFP and manipulation of their phenotype [reviewed in Fernandes et al. (2020) and Wu et al. (2020)]. Initial evidence suggests that polarization of macrophages back to an alternative anti-inflammatory M2 phenotype can be induced. M2 macrophages represent the other extreme in terms of functionality, as they play a major role in local tissue repair by secreting low levels of anti-inflammatory cytokines such as IL-10 at a much more accelerated rate compared to unpolarized “naïve” resident macrophages (Figure 3) (Zeyda et al., 2007; Fernandes et al., 2020). In fact, it has been proposed that MSC can indeed promote M2 macrophage polarization in vitro (Harrell et al., 2019). Furthermore, our group recently reported the switch of IFP macrophages from an M1 to an M2 phenotype in vivo, after a single intra-articular injection of a subset of BM-MSC (CD146+) in rats with induced synovitis and IFP fibrosis (Bowles et al., 2020).
The effects of IFP-MSC in macrophage polarization are far less defined. Nevertheless, it has been described that Substance P within IFP actively participates in immune responses and inflammatory cascades (i.e., neurogenic inflammation), enhancing the migration of monocytes to sites of inflammation (Mashaghi et al., 2016; Spitsin et al., 2017; Suvas, 2017). Relatedly, our group recently reported that upon exposure to a pro-inflammatory environment (Kouroupis et al., 2019a) and when manufactured under regulatory-compliant conditions (Kouroupis et al., 2020), IFP-MSC become enriched for CD10/neprilysin, an ectopeptidase that efficiently degrades Substance P both in vitro and in vivo. The resulting CD10-rich IFP-MSC exhibit an innate ability to selectively migrate to areas of active synovitis, reverse inflammation and fibrosis of synovium and IFP. Interestingly, these effects are directly related with the level of positivity for CD10 (Kouroupis et al., 2020). Furthermore, Substance P has been reported to induce the differentiation of pro-inflammatory macrophages into a special phagocytic M2 phenotype (M2SP), different from previously reported M2a and M2c subphenotypes (Lim et al., 2017).
MSC-based therapy to treat OA has received attention based on promising pre-clinical reports. Various cell sources have been successfully used in early-phase clinical trials, including bone marrow (Orozco et al., 2014; Vega et al., 2015; Soler et al., 2016), umbilical cord (Matas et al., 2019), and adipose-derived stromal vascular fraction (Garza et al., 2020). A recent systematic review summarizing available studies testing intra-articular MSC therapy for OA and chondral defects concluded that the therapy is safe with clinical and in some cases imaging improvement (McIntyre et al., 2018). Synovial and IFP-derived MSC are starting to be explored clinically, yet only initial data is available.
In a pioneering case control study, a mean of 1.89 million (range, 1.2–2.3 × 106) IFP-MSC with platelet-rich plasma (PRP) were intra-articularly injected in OA patients after arthroscopic debridement, and controlled against debridement + PRP alone (Koh and Choi, 2012). Patients were followed for up to 12–18 months, reporting no adverse effects and a significant improvement in Patient Reported Outcome Measurements (PROMs) including Lysholm score, visual analog scale (VAS), and Tegner activity were noted in the study group compared with control cohorts. However, Koh et al. (2013) did note some limitations, namely that the control group was significantly different in terms of baseline radiographic and chondral lesion severity, as well as the small sample size with a focus on severe KOA patients.
The same team then in a 24–26-month follow-up study demonstrated that the significant decrease of the Western Ontario and McMaster Universities Osteoarthritis Index (WOMAC) is directly related to the amount of injected IFP-MSC (Koh et al., 2013). The authors also found that study patients demonstrated significantly improved cartilage whole-organ MRI scores that correlated strongly with decreased pain and improved function (R = −0.588 and −0.0536 respectively, p < 0.05, N = 18). Collectively, these initial results indicate the positive effect of intra-articularly injected IFP-MSC in reducing pain and improving knee function in OA patients, when compared with arthroscopic debridement and PRP alone. However, in addition to the various limitations acknowledged by the authors, the study population only included older patients with severe KOA, thus warranting further clinical investigation to determine the efficacy of the procedure as well as it’s applicability to a broader clinical population.
Similarly, sMSC yielded encouraging results in treating symptomatic chondral lesions in patients. Sekiya et al. (2015) expanded sMSC with autologous human serum and intra-articularly injected them to treat femoral condyle chondral lesions in 10 patients. For an average follow-up of 52 months, histologic analyses indicated hyaline and fibrous cartilage formation paralleled by improved Lysholm scores (Sekiya et al., 2015). In another study, autologous sMSC scaffold-free constructs were implanted in five patients to treat 1.5–3.0 cm2 chondral lesions (Shimomura et al., 2018). Forty-eight weeks post-implantation all patients achieved defect filling with tissue integration whereas histological analysis indicated strong cartilaginous tissue formation in all patients, with few spindle-shaped fibroblast-like cells localized only at the new-formed cartilage superficial zone. No adverse effects and significantly clinical improvements were reported at a 24-month follow-up.
These preliminary clinical studies indicated for both IFP-MSC and sMSC the overall significant improvement in cartilage repair without any complications for the patients treated. Nevertheless, the small number of patients involved and the potential confounding effect of parallel products (e.g., PRP) requires the design of prospective randomized, controlled trials to establish efficacy beyond the established safety.
The involvement of immune and inflammatory events within the synovium and IFP during early KOA has led to changes in our thinking of the disease and potential treatment approaches. Furthermore, the identification of resident and infiltrating macrophages as key modulators of those events presents a novel therapeutic target in the treatment of KOA. The ability of locally delivered IFP-MSCs to regulate synovial/IFP inflammation and fibrosis then becomes a promising therapeutic alternative to mitigate disease progression of the disease. Nevertheless, more information is required to solidly connect MSC local effects, macrophage phenotypic polarization and inflammation/fibrosis control with a durable effect limiting KOA progression.
Moreover, one critical aspect to understanding the impact of macrophages in the IFP is to dissect the molecular, cellular, and genetic identities of the heterogeneous tissue. Critically, over the past decade, numerous technologies allowing single-cell RNA sequencing (scRNA-seq) have emerged to provide unprecedented ability to examine gene expression profiles at the single cell level (Svensson et al., 2018). In general, these techniques allow the deconvolution of a heterogeneous tissue into specific cell types and an examination of their abundance. Furthermore, subtle differences between cells of similar lineages can be distinguished on the basis of just a few gene expression changes. Finally, there is the ability to compare cellular profiles and gene expression across samples, conditions, or groups of individuals. While efforts are currently underway to dissect the cellular complexity of tissues throughout the body including articular cartilage during OA progression (Regev et al., 2017; Ji et al., 2019), the IFP is noticeably absent from these efforts. To our knowledge, there is no existing high throughput single cell expression profile of the IFP, either in its nascent state or following injury or in chronic disease. Given its role in the immune responses to these conditions, this remains a topic of importance moving forward in characterizing its importance.
Mechanistically, Substance P targeting and degradation by CD10-rich MSC could become a mechanism to disrupt the sustained chronic inflammation within the IFP and the transmission of nociception signals from the knee to the central nervous system. As such, the reduction of Substance P+ nerve fibers within IFP may possibly be related to control of KOA’s most prevalent clinical presentation, joint pain.
Finally, an emerging approach results from the description of extracellular vesicles (e.g., exosomes) released by MSC, and their involvement in the therapeutic activities of the cells. For instance, our previous reports indicate comparable effects between cells and their supernatant in terms of their ability to degrade Substance P. These observations may support the idea of a “cell-free” product that may recapitulate the therapeutic effects of their parental cells, with manufacturing advantages as previously described (Pachler et al., 2017; Rohde et al., 2019; Witwer et al., 2019). In fact, the potential use of exosome-based cell-free products has already sparked multiple pre-clinical studies assessing potential clinical translation of cell-free products with encouraging results (Kordelas et al., 2014; Mendicino et al., 2014; Karnieli et al., 2017; Hu et al., 2019; Cai et al., 2020; Jiang et al., 2020; Meng and Qiu, 2020).
The knowledge accrued over the last decade regarding the IFP has led to important discoveries that elucidate its role beyond that of a vascular tissue with a biomechanical role in the anterior compartment of the knee. The proposition of the IFP and the synovium functioning as a single unit and the now recognized tight molecular crosstalk between both structures has been shown to promote resident immune cells, immune cell infiltration, and the subsequent production of articular cartilage degradative molecules associated with the propagation of various knee pathologies such as KOA. On the other hand, the presence of IFP-MSC and sMSC suggest that the IFP and synovium act as a reservoir of therapeutic cellular products engaged with repair after exposure to inflammation and subsequent injury. These MSC have also been shown to modulate macrophage phenotypic polarization in favoring immunomodulatory conditions.
The ability of local MSC to regulate synovial/IFP inflammation and fibrosis poses a promising therapeutic target to mitigate disease progression. Therefore, the IFP presents an important target for limiting joint disease progression. More information is required to better understand the connection between the local MSC population and macrophage phenotypic polarization as it relates to controlling the propagation of inflammation/fibrosis and subsequent progression of KOA.
All authors listed have made a substantial, direct and intellectual contribution to the work, and approved it for publication.
The authors are in gratitude with the Soffer Family Foundation and the Diabetes Research Institute Foundation for their generous funding support.
DC is a paid consultant of Lipogems USA, LLC.
The remaining authors declare that the research was conducted in the absence of any commercial or financial relationships that could be construed as a potential conflict of interest.
Almeida, H. V., Cunniffe, G. M., Vinardell, T., Buckley, C. T., O’Brien, F. J., and Kelly, D. J. (2015). Coupling freshly isolated CD44(+) infrapatellar fat pad-derived stromal cells with a TGF-beta3 eluting cartilage ECM-derived scaffold as a single-stage strategy for promoting chondrogenesis. Adv. Healthc. Mater. 4, 1043–1053. doi: 10.1002/adhm.201400687
Almeida, H. V., Eswaramoorthy, R., Cunniffe, G. M., Buckley, C. T., O’Brien, F. J., and Kelly, D. (2016). Fibrin hydrogels functionalized with cartilage extracellular matrix and incorporating freshly isolated stromal cells as an injectable for cartilage regeneration. Acta Biomater. 36, 55–62. doi: 10.1016/j.actbio.2016.03.008
Almeida, H. V., Liu, Y., Cunniffe, G. M., Mulhall, K. J., Matsiko, A., Buckley, C. T., et al. (2014). Controlled release of transforming growth factor-beta3 from cartilage-extra-cellular-matrix-derived scaffolds to promote chondrogenesis of human-joint-tissue-derived stem cells. Acta Biomater. 10, 4400–4409. doi: 10.1016/j.actbio.2014.05.030
Apinun, J., Sengprasert, P., Yuktanandana, P., Ngarmukos, S., Tanavalee, A., and Reantragoon, R. (2016). Immune mediators in osteoarthritis: infrapatellar fat pad-infiltrating CD8+ T Cells are increased in osteoarthritic patients with higher clinical radiographic grading. Int. J. Rheumatol. 2016:9525724.
Attur, M., Samuels, J., Krasnokutsky, S., and Abramson, S. B. (2010). Targeting the synovial tissue for treating osteoarthritis (OA): where is the evidence? Best Pract. Res. Clin. Rheumatol. 24, 71–79. doi: 10.1016/j.berh.2009.08.011
Balistreri, C. R., Caruso, C., and Candore, G. (2010). The role of adipose tissue and adipokines in obesity-related inflammatory diseases. Mediat. Inflamm. 2010:802078.
Ballegaard, C., Riis, R. G., Bliddal, H., Christensen, R., Henriksen, M., Bartels, E. M., et al. (2014). Knee pain and inflammation in the infrapatellar fat pad estimated by conventional and dynamic contrast-enhanced magnetic resonance imaging in obese patients with osteoarthritis: a cross-sectional study. Osteoarthr. Cartilage 22, 933–940. doi: 10.1016/j.joca.2014.04.018
Bao, J. P., Chen, W. P., Feng, J., Hu, P. F., Shi, Z. L., and Wu, L. D. (2010). Leptin plays a catabolic role on articular cartilage. Mol. Biol. Rep. 37, 3265–3272. doi: 10.1007/s11033-009-9911-x
Bao, J. P., Jiang, L. F., Chen, W. P., Hu, P. F., and Wu, L. D. (2014). Expression of vaspin in the joint and the levels in the serum and synovial fluid of patients with osteoarthritis. Int. J. Clin. Exp. Med. 7, 3447–3453.
Barboza, E., Hudson, J., Chang, W. P., Kovats, S., Towner, R. A., Silasi-Mansat, R., et al. (2017). Profibrotic infrapatellar fat pad remodeling without m1 macrophage polarization precedes knee osteoarthritis in mice with diet-induced obesity. Arthritis Rheumatol. 69, 1221–1232. doi: 10.1002/art.40056
Bartok, B., and Firestein, G. S. (2010). Fibroblast-like synoviocytes: key effector cells in rheumatoid arthritis. Immunol. Rev. 233, 233–255. doi: 10.1111/j.0105-2896.2009.00859.x
Bastiaansen-Jenniskens, Y. M., Clockaerts, S., Feijt, C., Zuurmond, A. M., Stojanovic-Susulic, V., Bridts, C., et al. (2012). Infrapatellar fat pad of patients with end-stage osteoarthritis inhibits catabolic mediators in cartilage. Ann. Rheum. Dis. 71, 288–294. doi: 10.1136/ard.2011.153858
Bastiaansen-Jenniskens, Y. M., Wei, W., Feijt, C., Waarsing, J. H., Verhaar, J. A., Zuurmond, A. M., et al. (2013). Stimulation of fibrotic processes by the infrapatellar fat pad in cultured synoviocytes from patients with osteoarthritis: a possible role for prostaglandin f2alpha. Arthritis Rheum. 65, 2070–2080. doi: 10.1002/art.37996
Beekhuizen, M., Gierman, L. M., van Spil, W. E., Van Osch, G. J., Huizinga, T. W., Saris, D. B., et al. (2013). An explorative study comparing levels of soluble mediators in control and osteoarthritic synovial fluid. Osteoarthr. Cartilage 21, 918–922. doi: 10.1016/j.joca.2013.04.002
Belluzzi, E., Stocco, E., Pozzuoli, A., Granzotto, M., Porzionato, A., Vettor, R., et al. (2019). Contribution of infrapatellar fat pad and synovial membrane to knee osteoarthritis pain. Biomed. Res. Int. 2019:6390182.
Benito, M. J., Veale, D. J., FitzGerald, O., van den Berg, W. B., and Bresnihan, B. (2005). Synovial tissue inflammation in early and late osteoarthritis. Ann. Rheum. Dis. 64, 1263–1267. doi: 10.1136/ard.2004.025270
Bliddal, H., Leeds, A. R., and Christensen, R. (2014). Osteoarthritis, obesity and weight loss: evidence, hypotheses and horizons - a scoping review. Obes. Rev. 15, 578–586. doi: 10.1111/obr.12173
Bohnsack, M., Wilharm, A., Hurschler, C., Ruhmann, O., Stukenborg-Colsman, C., and Wirth, C. J. (2004). Biomechanical and kinematic influences of a total infrapatellar fat pad resection on the knee. Am. J. Sports Med. 32, 1873–1880. doi: 10.1177/0363546504263946
Bombardieri, M., Kam, N. W., Brentano, F., Choi, K., Filer, A., Kyburz, D., et al. (2011). A BAFF/APRIL-dependent TLR3-stimulated pathway enhances the capacity of rheumatoid synovial fibroblasts to induce AID expression and Ig class-switching in B cells. Ann. Rheum. Dis. 70, 1857–1865. doi: 10.1136/ard.2011.150219
Bondeson, J., Blom, A. B., Wainwright, S., Hughes, C., Caterson, B., and van den Berg, W. B. (2010). The role of synovial macrophages and macrophage-produced mediators in driving inflammatory and destructive responses in osteoarthritis. Arthritis Rheum. 62, 647–657. doi: 10.1002/art.27290
Bowles, A. C., Willman, M. A., Perucca Orfei, C., Agarwal, A., and Correa, D. (2020). Signature quality attributes of CD146+ mesenchymal stem/stromal cells correlate to high therapeutic and secretory potency. Stem Cells [Epub ahead of print]. doi: 10.1002/stem.3196
Bravo, B., Guisasola, M. C., Vaquero, J., Tirado, I., Gortazar, A. R., and Forriol, F. (2019). Gene expression, protein profiling, and chemotactic activity of infrapatellar fat pad mesenchymal stem cells in pathologies of the knee joint. J. Cell Physiol. 234, 18917–18927. doi: 10.1002/jcp.28532
Brouwers, H., von Hegedus, J., Toes, R., Kloppenburg, M., and Ioan-Facsinay, A. (2015). Lipid mediators of inflammation in rheumatoid arthritis and osteoarthritis. Best Pract. Res. Clin. Rheumatol. 29, 741–755. doi: 10.1016/j.berh.2016.02.003
Brumovsky, P. R. (2016). Dorsal root ganglion neurons and tyrosine hydroxylase–an intriguing association with implications for sensation and pain. Pain 157, 314–320. doi: 10.1097/j.pain.0000000000000381
Buckley, C. T., and Kelly, D. J. (2012). Expansion in the presence of FGF-2 enhances the functional development of cartilaginous tissues engineered using infrapatellar fat pad derived MSCs. J. Mech. Behav. Biomed. Mater. 11, 102–111. doi: 10.1016/j.jmbbm.2011.09.004
Buckley, C. T., Vinardell, T., Thorpe, S. D., Haugh, M. G., Jones, E., McGonagle, D., et al. (2010). Functional properties of cartilaginous tissues engineered from infrapatellar fat pad-derived mesenchymal stem cells. J. Biomech. 43, 920–926. doi: 10.1016/j.jbiomech.2009.11.005
Cai, J., Wu, J., Wang, J., Li, Y., Hu, X., Luo, S., et al. (2020). Extracellular vesicles derived from different sources of mesenchymal stem cells: therapeutic effects and translational potential. Cell Biosci. 10:69.
Caplan, A. I., and Correa, D. (2011). The MSC: an injury drugstore. Cell Stem Cell. 9, 11–15. doi: 10.1016/j.stem.2011.06.008
Caspar-Bauguil, S., Cousin, B., Galinier, A., Segafredo, C., Nibbelink, M., Andre, M., et al. (2005). Adipose tissues as an ancestral immune organ: site-specific change in obesity. FEBS Lett. 579, 3487–3492. doi: 10.1016/j.febslet.2005.05.031
Clements, K. M., Ball, A. D., Jones, H. B., Brinckmann, S., Read, S. J., and Murray, F. (2009). Cellular and histopathological changes in the infrapatellar fat pad in the monoiodoacetate model of osteoarthritis pain. Osteoarthr. Cartilage 17, 805–812. doi: 10.1016/j.joca.2008.11.002
Clockaerts, S., Bastiaansen-Jenniskens, Y. M., Feijt, C., De Clerck, L., Verhaar, J. A., Zuurmond, A. M., et al. (2012). Cytokine production by infrapatellar fat pad can be stimulated by interleukin 1beta and inhibited by peroxisome proliferator activated receptor alpha agonist. Ann. Rheum. Dis. 71, 1012–1018. doi: 10.1136/annrheumdis-2011-200688
Clockaerts, S., Bastiaansen-Jenniskens, Y. M., Runhaar, J., Van Osch, G. J., Van Offel, J. F., Verhaar, J. A., et al. (2010). The infrapatellar fat pad should be considered as an active osteoarthritic joint tissue: a narrative review. Osteoarthr. Cartilage 18, 876–882. doi: 10.1016/j.joca.2010.03.014
Coelho, M., Oliveira, T., and Fernandes, R. (2013). Biochemistry of adipose tissue: an endocrine organ. Arch. Med. Sci. 9, 191–200. doi: 10.5114/aoms.2013.33181
Cooper, A. M., and Khader, S. A. (2007). IL-12p40: an inherently agonistic cytokine. Trends Immunol. 28, 33–38. doi: 10.1016/j.it.2006.11.002
Correa, D., Somoza, R. A., Lin, P., Greenberg, S., Rom, E., Duesler, L., et al. (2015). Sequential exposure to fibroblast growth factors (FGF) 2, 9 and 18 enhances hMSC chondrogenic differentiation. Osteoarthr. Cartilage 23, 443–453. doi: 10.1016/j.joca.2014.11.013
Cowan, S. M., Hart, H. F., Warden, S. J., and Crossley, K. M. (2015). Infrapatellar fat pad volume is greater in individuals with patellofemoral joint osteoarthritis and associated with pain. Rheumatol. Int. 35, 1439–1442. doi: 10.1007/s00296-015-3250-0
Crema, M. D., Felson, D. T., Roemer, F. W., Niu, J., Marra, M. D., Zhang, Y., et al. (2013). Peripatellar synovitis: comparison between non-contrast-enhanced and contrast-enhanced MRI and association with pain. The MOST study. Osteoarthr. Cartilage 21, 413–418. doi: 10.1016/j.joca.2012.12.006
Davies, L. C., Rosas, M., Smith, P. J., Fraser, D. J., Jones, S. A., and Taylor, P. R. (2011). A quantifiable proliferative burst of tissue macrophages restores homeostatic macrophage populations after acute inflammation. Eur. J. Immunol. 41, 2155–2164. doi: 10.1002/eji.201141817
De Bari, C., Dell’Accio, F., Tylzanowski, P., and Luyten, F. P. (2001). Multipotent mesenchymal stem cells from adult human synovial membrane. Arthritis Rheum. 44, 1928–1942. doi: 10.1002/1529-0131(200108)44:8<1928::aid-art331>3.0.co;2-p
de Boer, T. N., van Spil, W. E., Huisman, A. M., Polak, A. A., Bijlsma, J. W., Lafeber, F. P., et al. (2012). Serum adipokines in osteoarthritis; comparison with controls and relationship with local parameters of synovial inflammation and cartilage damage. Osteoarthr. Cartilage 20, 846–853. doi: 10.1016/j.joca.2012.05.002
de Jong, A. J., Klein-Wieringa, I. R., Andersen, S. N., Kwekkeboom, J. C., Herb-van Toorn, L., de Lange-Brokaar, B. J. E., et al. (2017). Lack of high BMI-related features in adipocytes and inflammatory cells in the infrapatellar fat pad (IFP). Arthritis Res. Ther. 19, 186.
de Lange-Brokaar, B. J., Ioan-Facsinay, A., van Osch, G. J., Zuurmond, A. M., Schoones, J., Toes, R. E., et al. (2012). Synovial inflammation, immune cells and their cytokines in osteoarthritis: a review. Osteoarthr. Cartilage 20, 1484–1499. doi: 10.1016/j.joca.2012.08.027
Decker, R. S., Um, H. B., Dyment, N. A., Cottingham, N., Usami, Y., Enomoto-Iwamoto, M., et al. (2017). Cell origin, volume and arrangement are drivers of articular cartilage formation, morphogenesis and response to injury in mouse limbs. Dev. Biol. 426, 56–68. doi: 10.1016/j.ydbio.2017.04.006
Ding, D. C., Wu, K. C., Chou, H. L., Hung, W. T., Liu, H. W., and Chu, T. Y. (2015). Human infrapatellar fat pad-derived stromal cells have more potent differentiation capacity than other mesenchymal cells and can be enhanced by hyaluronan. Cell Transplant. 24, 1221–1232. doi: 10.3727/096368914x681937
Djouad, F., Bony, C., Haupl, T., Uze, G., Lahlou, N., Louis-Plence, P., et al. (2005). Transcriptional profiles discriminate bone marrow-derived and synovium-derived mesenchymal stem cells. Arthritis Res. Ther. 7, R1304–R1315.
do Amaral, R., Almeida, H. V., Kelly, D. J., O’Brien, F. J., and Kearney, C. J. (2017). Infrapatellar fat pad stem cells: from developmental biology to cell therapy. Stem Cells Int. 2017:6843727.
Dragoo, J. L., Johnson, C., and McConnell, J. (2012). Evaluation and treatment of disorders of the infrapatellar fat pad. Sports Med. 42, 51–67. doi: 10.2165/11595680-000000000-00000
Dragoo, J. L., Samimi, B., Zhu, M., Hame, S. L., Thomas, B. J., Lieberman, J. R., et al. (2003). Tissue-engineered cartilage and bone using stem cells from human infrapatellar fat pads. J. Bone Joint Surg. Br. 85, 740–747. doi: 10.1302/0301-620x.85b5.13587
Dumond, H., Presle, N., Terlain, B., Mainard, D., Loeuille, D., Netter, P., et al. (2003). Evidence for a key role of leptin in osteoarthritis. Arthritis Rheum. 48, 3118–3129. doi: 10.1002/art.11303
English, A., Jones, E. A., Corscadden, D., Henshaw, K., Chapman, T., Emery, P., et al. (2007). A comparative assessment of cartilage and joint fat pad as a potential source of cells for autologous therapy development in knee osteoarthritis. Rheumatology 46, 1676–1683. doi: 10.1093/rheumatology/kem217
Eymard, F., Pigenet, A., Citadelle, D., Flouzat-Lachaniette, C. H., Poignard, A., Benelli, C., et al. (2014). Induction of an inflammatory and prodegradative phenotype in autologous fibroblast-like synoviocytes by the infrapatellar fat pad from patients with knee osteoarthritis. Arthritis Rheumatol. 66, 2165–2174. doi: 10.1002/art.38657
Farrell, E., Both, S. K., Odorfer, K. I., Koevoet, W., Kops, N., O’Brien, F. J., et al. (2011). In-vivo generation of bone via endochondral ossification by in-vitro chondrogenic priming of adult human and rat mesenchymal stem cells. BMC Musculoskelet. Disord. 12:31. doi: 10.1186/1471-2474-12-31
Farrell, E., van der Jagt, O. P., Koevoet, W., Kops, N., van Manen, C. J., Hellingman, C. A., et al. (2009). Chondrogenic priming of human bone marrow stromal cells: a better route to bone repair? Tissue Eng. Part C Methods 15, 285–295. doi: 10.1089/ten.tec.2008.0297
Favero, M., El-Hadi, H., Belluzzi, E., Granzotto, M., Porzionato, A., Sarasin, G., et al. (2017). Infrapatellar fat pad features in osteoarthritis: a histopathological and molecular study. Rheumatology 56, 1784–1793. doi: 10.1093/rheumatology/kex287
Felson, D. T., Niu, J., Neogi, T., Goggins, J., Nevitt, M. C., Roemer, F., et al. (2016). Synovitis and the risk of knee osteoarthritis: the MOST Study. Osteoarthr. Cartilage 24, 458–464. doi: 10.1016/j.joca.2015.09.013
Feng, X., Li, Z., Wei, J., Feng, Z., Wu, W., and Zhao, Y. (2018). Injectable cartilaginous template transformed BMSCs into vascularized bone. Sci. Rep. 8:8244.
Fernandes, T. L., Gomoll, A. H., Lattermann, C., Hernandez, A. J., Bueno, D. F., and Amano, M. T. (2020). Macrophage: a potential target on cartilage regeneration. Front. Immunol. 11:111. doi: 10.3389/fimmu.2020.00111
Ferro, T., Santhagunam, A., Madeira, C., Salgueiro, J. B., da Silva, C. L., and Cabral, J. M. S. (2019). Successful isolation and ex vivo expansion of human mesenchymal stem/stromal cells obtained from different synovial tissue-derived (biopsy) samples. J. Cell Physiol. 234, 3973–3984. doi: 10.1002/jcp.27202
Fontanella, C. G., Belluzzi, E., Rossato, M., Olivotto, E., Trisolino, G., Ruggieri, P., et al. (2019). Quantitative MRI analysis of infrapatellar and suprapatellar fat pads in normal controls, moderate and end-stage osteoarthritis. Ann. Anat. 221, 108–114. doi: 10.1016/j.aanat.2018.09.007
Frank-Bertoncelj, M., Trenkmann, M., Klein, K., Karouzakis, E., Rehrauer, H., Bratus, A., et al. (2017). Epigenetically-driven anatomical diversity of synovial fibroblasts guides joint-specific fibroblast functions. Nat. Commun. 8:14852.
Freeman, M. A., and Wyke, B. (1967). The innervation of the knee joint. An anatomical and histological study in the cat. J. Anat. 101(Pt 3), 505–532.
Galipeau, J., Krampera, M., Barrett, J., Dazzi, F., Deans, R. J., DeBruijn, J., et al. (2016). International society for cellular therapy perspective on immune functional assays for mesenchymal stromal cells as potency release criterion for advanced phase clinical trials. Cytotherapy 18, 151–159. doi: 10.1016/j.jcyt.2015.11.008
Gallagher, J., Tierney, P., Murray, P., and O’Brien, M. (2005). The infrapatellar fat pad: anatomy and clinical correlations. Knee Surg. Sports Traumatol. Arthrosc. 13, 268–272. doi: 10.1007/s00167-004-0592-7
Garcia, J., Mennan, C., McCarthy, H. S., Roberts, S., Richardson, J. B., and Wright, K. T. (2016a). Chondrogenic potency analyses of donor-matched chondrocytes and mesenchymal stem cells derived from bone marrow, infrapatellar fat pad, and subcutaneous fat. Stem Cells Int. 2016:6969726.
Garcia, J., Wright, K., Roberts, S., Kuiper, J. H., Mangham, C., Richardson, J., et al. (2016b). Characterisation of synovial fluid and infrapatellar fat pad derived mesenchymal stromal cells: the influence of tissue source and inflammatory stimulus. Sci. Rep. 6:24295.
Garza, J. R., Campbell, R. E., Tjoumakaris, F. P., Freedman, K. B., Miller, L. S., Santa Maria, D., et al. (2020). Clinical efficacy of intra-articular mesenchymal stromal cells for the treatment of knee osteoarthritis: a double-blinded prospective randomized controlled clinical trial. Am. J. Sports Med. 48, 588–598. doi: 10.1177/0363546519899923
Gentek, R., Molawi, K., and Sieweke, M. H. (2014). Tissue macrophage identity and self-renewal. Immunol. Rev. 262, 56–73. doi: 10.1111/imr.12224
Gierman, L. M., Wopereis, S., van El, B., Verheij, E. R., Werff-van, der Vat, B. J., et al. (2013). Metabolic profiling reveals differences in concentrations of oxylipins and fatty acids secreted by the infrapatellar fat pad of donors with end-stage osteoarthritis and normal donors. Arthritis Rheum. 65, 2606–2614.
Ginhoux, F., and Guilliams, M. (2016). Tissue-resident macrophage ontogeny and homeostasis. Immunity 44, 439–449. doi: 10.1016/j.immuni.2016.02.024
Ginhoux, F., and Jung, S. (2014). Monocytes and macrophages: developmental pathways and tissue homeostasis. Nat. Rev. Immunol. 14, 392–404. doi: 10.1038/nri3671
Gomez-Aristizabal, A., Gandhi, R., Mahomed, N. N., Marshall, K. W., and Viswanathan, S. (2019). Synovial fluid monocyte/macrophage subsets and their correlation to patient-reported outcomes in osteoarthritic patients: a cohort study. Arthritis Res. Ther. 21:26.
Hagmann, S., Gotterbarm, T., Muller, T., Baesig, A. M., Gantz, S., Dreher, T., et al. (2013). The influence of bone marrow- and synovium-derived mesenchymal stromal cells from osteoarthritis patients on regulatory T cells in co-culture. Clin. Exp. Immunol. 173, 454–462. doi: 10.1111/cei.12122
Han, W., Aitken, D., Zhu, Z., Halliday, A., Wang, X., Antony, B., et al. (2016). Signal intensity alteration in the infrapatellar fat pad at baseline for the prediction of knee symptoms and structure in older adults: a cohort study. Ann. Rheum. Dis. 75, 1783–1788. doi: 10.1136/annrheumdis-2015-208360
Harrell, C. R., Markovic, B. S., Fellabaum, C., Arsenijevic, A., and Volarevic, V. (2019). Mesenchymal stem cell-based therapy of osteoarthritis: current knowledge and future perspectives. Biomed. Pharmacother. 109, 2318–2326. doi: 10.1016/j.biopha.2018.11.099
Heilmeier, U., Mamoto, K., Amano, K., Eck, B., Tanaka, M., Bullen, J. A., et al. (2019). Infrapatellar fat pad abnormalities are associated with a higher inflammatory synovial fluid cytokine profile in young adults following ACL tear. Osteoarthr. Cartilage 28, 82–91. doi: 10.1016/j.joca.2019.09.001
Hermida-Gomez, T., Fuentes-Boquete, I., Gimeno-Longas, M. J., Muinos-Lopez, E., Diaz-Prado, S., de Toro, F. J., et al. (2011). Quantification of cells expressing mesenchymal stem cell markers in healthy and osteoarthritic synovial membranes. J. Rheumatol. 38, 339–349. doi: 10.3899/jrheum.100614
Hill, C. L., Hunter, D. J., Niu, J., Clancy, M., Guermazi, A., Genant, H., et al. (2007). Synovitis detected on magnetic resonance imaging and its relation to pain and cartilage loss in knee osteoarthritis. Ann. Rheum. Dis. 66, 1599–1603. doi: 10.1136/ard.2006.067470
Hindle, P., Khan, N., Biant, L., and Peault, B. (2017). The infrapatellar fat pad as a source of perivascular stem cells with increased chondrogenic potential for regenerative medicine. Stem Cells Transl. Med. 6, 77–87. doi: 10.5966/sctm.2016-0040
Hu, P., Yang, Q., Wang, Q., Shi, C., Wang, D., Armato, U., et al. (2019). Mesenchymal stromal cells-exosomes: a promising cell-free therapeutic tool for wound healing and cutaneous regeneration. Burns Trauma 7:38.
Ioan-Facsinay, A., and Kloppenburg, M. (2013). An emerging player in knee osteoarthritis: the infrapatellar fat pad. Arthritis Res. Ther. 15:225. doi: 10.1186/ar4422
Ioan-Facsinay, A., and Kloppenburg, M. (2017). Osteoarthritis: inflammation and fibrosis in adipose tissue of osteoarthritic joints. Nat. Rev. Rheumatol. 13, 325–326. doi: 10.1038/nrrheum.2017.53
Ioan-Facsinay, A., Kwekkeboom, J. C., Westhoff, S., Giera, M., Rombouts, Y., van Harmelen, V., et al. (2013). Adipocyte-derived lipids modulate CD4+ T-cell function. Eur. J. Immunol. 43, 1578–1587. doi: 10.1002/eji.201243096
Ji, Q., Zheng, Y., Zhang, G., Hu, Y., Fan, X., Hou, Y., et al. (2019). Single-cell RNA-seq analysis reveals the progression of human osteoarthritis. Ann. Rheum. Dis. 78, 100–110. doi: 10.1136/annrheumdis-2017-212863
Jiang, L. F., Fang, J. H., and Wu, L. D. (2019). Role of infrapatellar fat pad in pathological process of knee osteoarthritis: future applications in treatment. World J. Clin. Cases 7, 2134–2142. doi: 10.12998/wjcc.v7.i16.2134
Jiang, T., Wang, Z., and Sun, J. (2020). Human bone marrow mesenchymal stem cell-derived exosomes stimulate cutaneous wound healing mediates through TGF-beta/Smad signaling pathway. Stem Cell Res. Ther. 11:198.
Jurgens, W. J., van Dijk, A., Doulabi, B. Z., Niessen, F. B., Ritt, M. J., van Milligen, F. J., et al. (2009). Freshly isolated stromal cells from the infrapatellar fat pad are suitable for a one-step surgical procedure to regenerate cartilage tissue. Cytotherapy 11, 1052–1064. doi: 10.3109/14653240903219122
Kalaitzoglou, E., Griffin, T. M., and Humphrey, M. B. (2017). Innate immune responses and osteoarthritis. Curr. Rheumatol. Rep. 19:45.
Kandahari, A. M., Yang, X., Dighe, A. S., Pan, D., and Cui, Q. (2015). Recognition of immune response for the early diagnosis and treatment of osteoarthritis. J. Immunol. Res. 2015:192415.
Karnieli, O., Friedner, O. M., Allickson, J. G., Zhang, N., Jung, S., Fiorentini, D., et al. (2017). A consensus introduction to serum replacements and serum-free media for cellular therapies. Cytotherapy 19, 155–169. doi: 10.1016/j.jcyt.2016.11.011
Karystinou, A., Dell’Accio, F., Kurth, T. B., Wackerhage, H., Khan, I. M., Archer, C. W., et al. (2009). Distinct mesenchymal progenitor cell subsets in the adult human synovium. Rheumatology 48, 1057–1064. doi: 10.1093/rheumatology/kep192
Kennedy, J. C., Alexander, I. J., and Hayes, K. C. (1982). Nerve supply of the human knee and its functional importance. Am. J. Sports Med. 10, 329–335. doi: 10.1177/036354658201000601
Khan, W. S., Tew, S. R., Adesida, A. B., and Hardingham, T. E. (2008). Human infrapatellar fat pad-derived stem cells express the pericyte marker 3G5 and show enhanced chondrogenesis after expansion in fibroblast growth factor-2. Arthritis Res. Ther. 10:R74.
King, L. K., March, L., and Anandacoomarasamy, A. (2013). Obesity & osteoarthritis. Indian J. Med. Res. 138, 185–193.
Klein-Wieringa, I. R., Andersen, S. N., Kwekkeboom, J. C., Giera, M., de Lange-Brokaar, B. J., van Osch, G. J., et al. (2013). Adipocytes modulate the phenotype of human macrophages through secreted lipids. J. Immunol. 191, 1356–1363. doi: 10.4049/jimmunol.1203074
Klein-Wieringa, I. R., de Lange-Brokaar, B. J., Yusuf, E., Andersen, S. N., Kwekkeboom, J. C., Kroon, H. M., et al. (2016). Inflammatory cells in patients with endstage knee osteoarthritis: a comparison between the synovium and the infrapatellar fat pad. J. Rheumatol. 43, 771–778. doi: 10.3899/jrheum.151068
Klein-Wieringa, I. R., Kloppenburg, M., Bastiaansen-Jenniskens, Y. M., Yusuf, E., Kwekkeboom, J. C., El-Bannoudi, H., et al. (2011). The infrapatellar fat pad of patients with osteoarthritis has an inflammatory phenotype. Ann. Rheum. Dis. 70, 851–857. doi: 10.1136/ard.2010.140046
Koh, Y. G., and Choi, Y. J. (2012). Infrapatellar fat pad-derived mesenchymal stem cell therapy for knee osteoarthritis. Knee 19, 902–907. doi: 10.1016/j.knee.2012.04.001
Koh, Y. G., Jo, S. B., Kwon, O. R., Suh, D. S., Lee, S. W., Park, S. H., et al. (2013). Mesenchymal stem cell injections improve symptoms of knee osteoarthritis. Arthroscopy 29, 748–755. doi: 10.1016/j.arthro.2012.11.017
Kohn, D., Deiler, S., and Rudert, M. (1995). Arterial blood supply of the infrapatellar fat pad. Anatomy and clinical consequences. Arch. Orthop. Trauma Surg. 114, 72–75. doi: 10.1007/bf00422828
Koizumi, K., Ebina, K., Hart, D. A., Hirao, M., Noguchi, T., Sugita, N., et al. (2016). Synovial mesenchymal stem cells from osteo- or rheumatoid arthritis joints exhibit good potential for cartilage repair using a scaffold-free tissue engineering approach. Osteoarthr. Cartilage 24, 1413–1422. doi: 10.1016/j.joca.2016.03.006
Kordelas, L., Rebmann, V., Ludwig, A. K., Radtke, S., Ruesing, J., Doeppner, T. R., et al. (2014). MSC-derived exosomes: a novel tool to treat therapy-refractory graft-versus-host disease. Leukemia 28, 970–973. doi: 10.1038/leu.2014.41
Kouroupis, D., Bowles, A. C., Willman, M. A., Perucca Orfei, C., Colombini, A., Best, T. M., et al. (2019a). Infrapatellar fat pad-derived MSC response to inflammation and fibrosis induces an immunomodulatory phenotype involving CD10-mediated Substance P degradation. Sci. Rep. 9:10864.
Kouroupis, D., Sanjurjo-Rodriguez, C., Jones, E., and Correa, D. (2019b). Mesenchymal stem cell functionalization for enhanced therapeutic applications. Tissue Eng. Part B Rev. 25, 55–77. doi: 10.1089/ten.teb.2018.0118
Kouroupis, D. B. A., Best, T. M., Kaplan, L. D., and Correa, D. (2020). CD10/neprilysin enrichment in infrapatellar fat pad-derived MSC under regulatory-compliant conditions: implications for efficient synovitis and fat pad fibrosis reversal. Am. J. Sports Med. 48, 2013–2027. doi: 10.1177/0363546520917699
Kriegova, E., Manukyan, G., Mikulkova, Z., Gabcova, G., Kudelka, M., Gajdos, P., et al. (2018). Gender-related differences observed among immune cells in synovial fluid in knee osteoarthritis. Osteoarthr. Cartilage 26, 1247–1256. doi: 10.1016/j.joca.2018.04.016
Kubosch, E. J., Lang, G., Furst, D., Kubosch, D., Izadpanah, K., Rolauffs, B., et al. (2018). The potential for synovium-derived stem cells in cartilage repair. Curr. Stem Cell Res. Ther. 13, 174–184. doi: 10.2174/1574888x12666171002111026
Kuptniratsaikul, V., Thanakhumtorn, S., Chinswangwatanakul, P., Wattanamongkonsil, L., and Thamlikitkul, V. (2009). Efficacy and safety of Curcuma domestica extracts in patients with knee osteoarthritis. J. Altern. Complement. Med. 15, 891–897. doi: 10.1089/acm.2008.0186
Lee, S. Y., Nakagawa, T., and Reddi, A. H. (2008). Induction of chondrogenesis and expression of superficial zone protein (SZP)/lubricin by mesenchymal progenitors in the infrapatellar fat pad of the knee joint treated with TGF-beta1 and BMP-7. Biochem. Biophys. Res. Commun. 376, 148–153. doi: 10.1016/j.bbrc.2008.08.138
Li, F., Tang, Y., Song, B., Yu, M., Li, Q., Zhang, C., et al. (2019). Nomenclature clarification: synovial fibroblasts and synovial mesenchymal stem cells. Stem Cell Res. Ther. 10:260.
Li, Y. S., Luo, W., Zhu, S. A., and Lei, G. H. (2017). T Cells in osteoarthritis: alterations and beyond. Front. Immunol. 8:356. doi: 10.3389/fimmu.2017.00356
Lieberthal, J., Sambamurthy, N., and Scanzello, C. R. (2015). Inflammation in joint injury and post-traumatic osteoarthritis. Osteoarthr. Cartilage 23, 1825–1834. doi: 10.1016/j.joca.2015.08.015
Lim, J. E., Chung, E., and Son, Y. (2017). A neuropeptide, Substance-P, directly induces tissue-repairing M2 like macrophages by activating the PI3K/Akt/mTOR pathway even in the presence of IFNgamma. Sci. Rep. 7:9417.
Liu, Y., Buckley, C. T., Almeida, H. V., Mulhall, K. J., and Kelly, D. J. (2014). Infrapatellar fat pad-derived stem cells maintain their chondrogenic capacity in disease and can be used to engineer cartilaginous grafts of clinically relevant dimensions. Tissue Eng. Part A 20, 3050–3062. doi: 10.1089/ten.tea.2014.0035
Liu, Y., Buckley, C. T., Downey, R., Mulhall, K. J., and Kelly, D. J. (2012). The role of environmental factors in regulating the development of cartilaginous grafts engineered using osteoarthritic human infrapatellar fat pad-derived stem cells. Tissue Eng. Part A 18, 1531–1541. doi: 10.1089/ten.tea.2011.0575
Loeuille, D., Sauliere, N., Champigneulle, J., Rat, A. C., Blum, A., and Chary-Valckenaere, I. (2011). Comparing non-enhanced and enhanced sequences in the assessment of effusion and synovitis in knee OA: associations with clinical, macroscopic and microscopic features. Osteoarthr. Cartilage 19, 1433–1439. doi: 10.1016/j.joca.2011.08.010
Losina, E., Walensky, R. P., Reichmann, W. M., Holt, H. L., Gerlovin, H., Solomon, D. H., et al. (2011). Impact of obesity and knee osteoarthritis on morbidity and mortality in older Americans. Ann. Intern. Med. 154, 217–226.
Macchi, V., Stocco, E., Stecco, C., Belluzzi, E., Favero, M., Porzionato, A., et al. (2018). The infrapatellar fat pad and the synovial membrane: an anatomo-functional unit. J. Anat. 233, 146–154. doi: 10.1111/joa.12820
Maekawa, K., Furukawa, H., Kanazawa, Y., Hijioka, A., Suzuki, K., and Fujimoto, S. (1996). Electron and immunoelectron microscopy on healing process of the rat anterior cruciate ligament after partial transection: the roles of multipotent fibroblasts in the synovial tissue. Histol. Histopathol. 11, 607–619.
Marsano, A., Millward-Sadler, S. J., Salter, D. M., Adesida, A., Hardingham, T., Tognana, E., et al. (2007). Differential cartilaginous tissue formation by human synovial membrane, fat pad, meniscus cells and articular chondrocytes. Osteoarthr. Cartilage 15, 48–58. doi: 10.1016/j.joca.2006.06.009
Mashaghi, A., Marmalidou, A., Tehrani, M., Grace, P. M., Pothoulakis, C., and Dana, R. (2016). Neuropeptide substance P and the immune response. Cell Mol. Life Sci. 73, 4249–4264. doi: 10.1007/s00018-016-2293-z
Mata-Essayag, S., Magaldi, S., de Capriles, C. H., Henao, L., Garrido, L., and Pacillo, V. (2001). Mucor indicus necrotizing fasciitis. Int. J. Dermatol. 40, 406–408. doi: 10.1046/j.1365-4362.2001.01246-3.x
Matarese, G., Leiter, E. H., and La Cava, A. (2007). Leptin in autoimmunity: many questions, some answers. Tissue Antigens 70, 87–95. doi: 10.1111/j.1399-0039.2007.00886.x
Matas, J., Orrego, M., Amenabar, D., Infante, C., Tapia-Limonchi, R., Cadiz, M. I., et al. (2019). Umbilical cord-derived mesenchymal stromal cells (MSCs) for knee osteoarthritis: repeated MSC dosing is superior to a single msc dose and to hyaluronic acid in a controlled randomized phase I/II Trial. Stem Cells Transl. Med. 8, 215–224. doi: 10.1002/sctm.18-0053
Mathiessen, A., and Conaghan, P. G. (2017). Synovitis in osteoarthritis: current understanding with therapeutic implications. Arthritis Res. Ther. 19, 18.
Mathis, D. (2013). Immunological goings-on in visceral adipose tissue. Cell Metab. 17, 851–859. doi: 10.1016/j.cmet.2013.05.008
McIntyre, J. A., Jones, I. A., Han, B., and Vangsness, C. T. Jr. (2018). Intra-articular mesenchymal stem cell therapy for the human joint: a systematic review. Am. J. Sports Med. 46, 3550–3563. doi: 10.1177/0363546517735844
Mendicino, M., Bailey, A. M., Wonnacott, K., Puri, R. K., and Bauer, S. R. (2014). MSC-based product characterization for clinical trials: an FDA perspective. Cell Stem Cell. 14, 141–145. doi: 10.1016/j.stem.2014.01.013
Meng, Q., and Qiu, B. (2020). Exosomal MicroRNA-320a derived from mesenchymal stem cells regulates rheumatoid arthritis fibroblast-like synoviocyte activation by suppressing CXCL9 expression. Front. Physiol. 11:441. doi: 10.3389/fphys.2020.00441
Mesallati, T., Buckley, C. T., and Kelly, D. J. (2017). Engineering cartilaginous grafts using chondrocyte-laden hydrogels supported by a superficial layer of stem cells. J. Tissue Eng. Regen. Med. 11, 1343–1353. doi: 10.1002/term.2033
Mesallati, T., Sheehy, E. J., Vinardell, T., Buckley, C. T., and Kelly, D. J. (2015). Tissue engineering scaled-up, anatomically shaped osteochondral constructs for joint resurfacing. Eur. Cell Mater. 30, 163–85; discussion85–86.
Mizuno, H., Tobita, M., and Uysal, A. C. (2012). Concise review: adipose-derived stem cells as a novel tool for future regenerative medicine. Stem Cells 30, 804–810. doi: 10.1002/stem.1076
Mizuno, M., Katano, H., Mabuchi, Y., Ogata, Y., Ichinose, S., Fujii, S., et al. (2018). Specific markers and properties of synovial mesenchymal stem cells in the surface, stromal, and perivascular regions. Stem Cell Res. Ther. 9:123.
Mochizuki, T., Muneta, T., Sakaguchi, Y., Nimura, A., Yokoyama, A., Koga, H., et al. (2006). Higher chondrogenic potential of fibrous synovium- and adipose synovium-derived cells compared with subcutaneous fat-derived cells: distinguishing properties of mesenchymal stem cells in humans. Arthritis Rheum. 54, 843–853. doi: 10.1002/art.21651
Mustonen, A. M., Kakela, R., Lehenkari, P., Huhtakangas, J., Turunen, S., Joukainen, A., et al. (2019). Distinct fatty acid signatures in infrapatellar fat pad and synovial fluid of patients with osteoarthritis versus rheumatoid arthritis. Arthritis Res. Ther. 21:124. doi: 10.3109/08916934.2015.1113267
Muttigi, M. S., Kim, B. J., Choi, B., Yoshie, A., Kumar, H., Han, I., et al. (2018). Matrilin-3 codelivery with adipose-derived mesenchymal stem cells promotes articular cartilage regeneration in a rat osteochondral defect model. J. Tissue Eng. Regen. Med. 12, 667–675. doi: 10.1002/term.2485
Nair, A., Kanda, V., Bush-Joseph, C., Verma, N., Chubinskaya, S., Mikecz, K., et al. (2012). Synovial fluid from patients with early osteoarthritis modulates fibroblast-like synoviocyte responses to toll-like receptor 4 and toll-like receptor 2 ligands via soluble CD14. Arthritis Rheum. 64, 2268–2277. doi: 10.1002/art.34495
Nimura, A., Muneta, T., Koga, H., Mochizuki, T., Suzuki, K., Makino, H., et al. (2008). Increased proliferation of human synovial mesenchymal stem cells with autologous human serum: comparisons with bone marrow mesenchymal stem cells and with fetal bovine serum. Arthritis Rheum. 58, 501–510. doi: 10.1002/art.23219
O’HEireamhoin, S., Buckley, C. T., Jones, E., McGonagle, D., Mulhall, K. J., and Kelly, D. J. (2013). Recapitulating aspects of the oxygen and substrate environment of the damaged joint milieu for stem cell-based cartilage tissue engineering. Tissue Eng. Part C Methods 19, 117–127. doi: 10.1089/ten.tec.2012.0142
Orozco, L., Munar, A., Soler, R., Alberca, M., Soler, F., Huguet, M., et al. (2014). Treatment of knee osteoarthritis with autologous mesenchymal stem cells: two-year follow-up results. Transplantation 97, e66–e68. doi: 10.1097/tp.0000000000000167
Orr, C., Vieira-Sousa, E., Boyle, D. L., Buch, M. H., Buckley, C. D., Canete, J. D., et al. (2017). Synovial tissue research: a state-of-the-art review. Nat. Rev. Rheumatol. 13:630.
Pachler, K., Lener, T., Streif, D., Dunai, Z. A., Desgeorges, A., Feichtner, M., et al. (2017). A good manufacturing practice-grade standard protocol for exclusively human mesenchymal stromal cell-derived extracellular vesicles. Cytotherapy 19, 458–472. doi: 10.1016/j.jcyt.2017.01.001
Pan, F., Han, W., Wang, X., Liu, Z., Jin, X., Antony, B., et al. (2015). A longitudinal study of the association between infrapatellar fat pad maximal area and changes in knee symptoms and structure in older adults. Ann. Rheum. Dis. 74, 1818–1824. doi: 10.1136/annrheumdis-2013-205108
Paul, F., Arkin, Y., Giladi, A., Jaitin, D. A., Kenigsberg, E., Keren-Shaul, H., et al. (2015). Transcriptional heterogeneity and lineage commitment in myeloid progenitors. Cell 163, 1663–1677. doi: 10.1016/j.cell.2015.11.013
Pelletier, J. P., Martel-Pelletier, J., and Abramson, S. B. (2001). Osteoarthritis, an inflammatory disease: potential implication for the selection of new therapeutic targets. Arthritis Rheum. 44, 1237–1247. doi: 10.1002/1529-0131(200106)44:6<1237::aid-art214>3.0.co;2-f
Prabhakar, A., Lynch, A. P., and Ahearne, M. (2016). Self-assembled infrapatellar fat-pad progenitor cells on a poly-epsilon-caprolactone film for cartilage regeneration. Artif. Organs 40, 376–384. doi: 10.1111/aor.12565
Regev, A., Teichmann, S. A., Lander, E. S., Amit, I., Benoist, C., Birney, E., et al. (2017). The human cell atlas. eLife 6:e27041.
Roelofs, A. J., Zupan, J., Riemen, A. H. K., Kania, K., Ansboro, S., White, N., et al. (2017). Joint morphogenetic cells in the adult mammalian synovium. Nat. Commun. 8:15040.
Roemer, F. W., Guermazi, A., Zhang, Y., Yang, M., Hunter, D. J., Crema, M. D., et al. (2009). Hoffa’s fat pad: evaluation on unenhanced mr images as a measure of patellofemoral synovitis in osteoarthritis. Am. J. Roentgenol. 192, 1696–1700. doi: 10.2214/ajr.08.2038
Rohde, E., Pachler, K., and Gimona, M. (2019). Manufacturing and characterization of extracellular vesicles from umbilical cord-derived mesenchymal stromal cells for clinical testing. Cytotherapy 21, 581–592. doi: 10.1016/j.jcyt.2018.12.006
Sakaguchi, Y., Sekiya, I., Yagishita, K., and Muneta, T. (2005). Comparison of human stem cells derived from various mesenchymal tissues: superiority of synovium as a cell source. Arthritis Rheum. 52, 2521–2529. doi: 10.1002/art.21212
Scanzello, C. R., and Goldring, S. R. (2012). The role of synovitis in osteoarthritis pathogenesis. Bone 51, 249–257. doi: 10.1016/j.bone.2012.02.012
Schnoor, M., Alcaide, P., Voisin, M. B., and van Buul, J. D. (2016). Recruitment of immune cells into inflamed tissues: consequences for endothelial barrier integrity and tissue functionality. Mediat. Inflamm. 2016:1561368.
Scotti, C., Piccinini, E., Takizawa, H., Todorov, A., Bourgine, P., Papadimitropoulos, A., et al. (2013). Engineering of a functional bone organ through endochondral ossification. Proc. Natl. Acad. Sci. U.S.A. 110, 3997–4002. doi: 10.1073/pnas.1220108110
Sekiya, I., Muneta, T., Horie, M., and Koga, H. (2015). Arthroscopic transplantation of synovial stem cells improves clinical outcomes in knees with cartilage defects. Clin. Orthop. Relat. Res. 473, 2316–2326. doi: 10.1007/s11999-015-4324-8
Shimomura, K., Yasui, Y., Koizumi, K., Chijimatsu, R., Hart, D. A., Yonetani, Y., et al. (2018). First-in-human pilot study of implantation of a scaffold-free tissue-engineered construct generated from autologous synovial mesenchymal stem cells for repair of knee chondral lesions. Am. J. Sports Med. 46, 2384–2393. doi: 10.1177/0363546518781825
Siciliano, C., Bordin, A., Ibrahim, M., Chimenti, I., Cassiano, F., Gatto, I., et al. (2016). The adipose tissue of origin influences the biological potential of human adipose stromal cells isolated from mediastinal and subcutaneous fat depots. Stem Cell Res. 17, 342–351. doi: 10.1016/j.scr.2016.07.010
Sivasubramaniyan, K., Koevoet, W., Hakimiyan, A. A., Sande, M., Farrell, E., Hoogduijn, M. J., et al. (2019). Cell-surface markers identify tissue resident multipotential stem/stromal cell subsets in synovial intimal and sub-intimal compartments with distinct chondrogenic properties. Osteoarthr. Cartilage 27, 1831–1840. doi: 10.1016/j.joca.2019.08.006
Sokolove, J., and Lepus, C. M. (2013). Role of inflammation in the pathogenesis of osteoarthritis: latest findings and interpretations. Ther. Adv. Musculoskelet. Dis. 5, 77–94. doi: 10.1177/1759720x12467868
Soler, R., Orozco, L., Munar, A., Huguet, M., Lopez, R., Vives, J., et al. (2016). Final results of a phase I-II trial using ex vivo expanded autologous Mesenchymal Stromal Cells for the treatment of osteoarthritis of the knee confirming safety and suggesting cartilage regeneration. Knee 23, 647–654. doi: 10.1016/j.knee.2015.08.013
Spitsin, S., Meshki, J., Winters, A., Tuluc, F., Benton, T. D., and Douglas, S. D. (2017). Substance P-mediated chemokine production promotes monocyte migration. J. Leukoc. Biol. 101, 967–973. doi: 10.1189/jlb.1ab0416-188rr
Stagg, J., and Galipeau, J. (2013). Mechanisms of immune modulation by mesenchymal stromal cells and clinical translation. Curr. Mol. Med. 13, 856–867. doi: 10.2174/1566524011313050016
Sun, A. R., Panchal, S. K., Friis, T., Sekar, S., Crawford, R., Brown, L., et al. (2017). Obesity-associated metabolic syndrome spontaneously induces infiltration of pro-inflammatory macrophage in synovium and promotes osteoarthritis. PLoS One 12:e0183693. doi: 10.1371/journal.pone.0183693
Sun, Y., Chen, S., and Pei, M. (2018). Comparative advantages of infrapatellar fat pad: an emerging stem cell source for regenerative medicine. Rheumatology 57, 2072–2086. doi: 10.1093/rheumatology/kex487
Suvas, S. (2017). Role of substance P neuropeptide in inflammation, wound healing, and tissue homeostasis. J. Immunol. 199, 1543–1552. doi: 10.4049/jimmunol.1601751
Svensson, V., Vento-Tormo, R., and Teichmann, S. A. (2018). Exponential scaling of single-cell RNA-seq in the past decade. Nat. Protoc. 13, 599–604. doi: 10.1038/nprot.2017.149
Tangchitphisut, P., Srikaew, N., Numhom, S., Tangprasittipap, A., Woratanarat, P., Wongsak, S., et al. (2016). Infrapatellar fat pad: an alternative source of adipose-derived mesenchymal stem cells. Arthritis 2016:4019873.
To, K., Zhang, B., Romain, K., Mak, C., and Khan, W. (2019). Synovium-derived mesenchymal stem cell transplantation in cartilage regeneration: a PRISMA review of in vivo studies. Front. Bioeng. Biotechnol. 7:314. doi: 10.3389/fbioe.2019.00314
Toghraie, F. S., Chenari, N., Gholipour, M. A., Faghih, Z., Torabinejad, S., Dehghani, S., et al. (2011). Treatment of osteoarthritis with infrapatellar fat pad derived mesenchymal stem cells in Rabbit. Knee 18, 71–75. doi: 10.1016/j.knee.2010.03.001
Toussirot, E., Streit, G., and Wendling, D. (2007). The contribution of adipose tissue and adipokines to inflammation in joint diseases. Curr. Med. Chem. 14, 1095–1100. doi: 10.2174/092986707780362826
Tu, J., Hong, W., Guo, Y., Zhang, P., Fang, Y., Wang, X., et al. (2019). Ontogeny of synovial macrophages and the roles of synovial macrophages from different origins in arthritis. Front. Immunol. 10:1146. doi: 10.3389/fimmu.2019.01146
Tu, J., Hong, W., Zhang, P., Wang, X., Korner, H., and Wei, W. (2018). Ontology and function of fibroblast-like and macrophage-like synoviocytes: how do they talk to each other and can they be targeted for rheumatoid arthritis therapy? Front. Immunol. 9:1467. doi: 10.3389/fimmu.2018.01467
Uccelli, A., and de Rosbo, N. K. (2015). The immunomodulatory function of mesenchymal stem cells: mode of action, and pathways. Ann. N. Y. Acad. Sci. 1351, 114–126. doi: 10.1111/nyas.12815
Vega, A., Martin-Ferrero, M. A., Del Canto, F., Alberca, M., Garcia, V., Munar, A., et al. (2015). Treatment of knee osteoarthritis with allogeneic bone marrow mesenchymal stem cells: a randomized controlled trial. Transplantation 99, 1681–1690. doi: 10.1097/tp.0000000000000678
Vilalta, M., Degano, I. R., Bago, J., Gould, D., Santos, M., Garcia-Arranz, M., et al. (2008). Biodistribution, long-term survival, and safety of human adipose tissue-derived mesenchymal stem cells transplanted in nude mice by high sensitivity non-invasive bioluminescence imaging. Stem Cells Dev. 17, 993–1003.
Vinardell, T., Buckley, C. T., Thorpe, S. D., and Kelly, D. J. (2011). Composition–function relations of cartilaginous tissues engineered from chondrocytes and mesenchymal stem cells isolated from bone marrow and infrapatellar fat pad. J. Tissue Eng. Regen. Med. 5, 673–683. doi: 10.1002/term.357
Vinardell, T., Sheehy, E. J., Buckley, C. T., and Kelly, D. J. (2012). A comparison of the functionality and in vivo phenotypic stability of cartilaginous tissues engineered from different stem cell sources. Tissue Eng. Part A 18, 1161–1170. doi: 10.1089/ten.tea.2011.0544
Wickham, M. Q., Erickson, G. R., Gimble, J. M., Vail, T. P., and Guilak, F. (2003). Multipotent stromal cells derived from the infrapatellar fat pad of the knee. Clin. Orthop. Relat. Res. 412, 196–212. doi: 10.1097/01.blo.0000072467.53786.ca
Witwer, K. W., Van Balkom, B. W. M., Bruno, S., Choo, A., Dominici, M., Gimona, M., et al. (2019). Defining mesenchymal stromal cell (MSC)-derived small extracellular vesicles for therapeutic applications. J. Extracell Vesicles 8:1609206. doi: 10.1080/20013078.2019.1609206
Woodley, S. J., Latimer, C. P., Meikle, G. R., and Stringer, M. D. (2012). Articularis genus: an anatomic and MRI study in cadavers. J. Bone Joint Surg. Am. 94, 59–67. doi: 10.2106/jbjs.k.00157
Wu, C. L., Harasymowicz, N. S., Klimak, M. A., Collins, K. H., and Guilak, F. (2020). The role of macrophages in osteoarthritis and cartilage repair. Osteoarthr. Cartilage 28, 544–554. doi: 10.1016/j.joca.2019.12.007
Ye, K., Felimban, R., Traianedes, K., Moulton, S. E., Wallace, G. G., Chung, J., et al. (2014). Chondrogenesis of infrapatellar fat pad derived adipose stem cells in 3D printed chitosan scaffold. PLoS One 9:e99410. doi: 10.1371/journal.pone.0099410
Yoshitomi, H. (2019). Regulation of immune responses and chronic inflammation by fibroblast-like synoviocytes. Front. Immunol. 10:1395. doi: 10.3389/fimmu.2019.01395
Yusuf, E. (2012). Metabolic factors in osteoarthritis: obese people do not walk on their hands. Arthritis Res. Ther. 14:123. doi: 10.1186/ar3894
Zeyda, M., Farmer, D., Todoric, J., Aszmann, O., Speiser, M., Gyori, G., et al. (2007). Human adipose tissue macrophages are of an anti-inflammatory phenotype but capable of excessive pro-inflammatory mediator production. Int. J. Obes. 31, 1420–1428. doi: 10.1038/sj.ijo.0803632
Keywords: infrapatellar fat pad, synovium, mesenchymal stem/stromal cells, macrophages, osteoarthritis
Citation: Greif DN, Kouroupis D, Murdock CJ, Griswold AJ, Kaplan LD, Best TM and Correa D (2020) Infrapatellar Fat Pad/Synovium Complex in Early-Stage Knee Osteoarthritis: Potential New Target and Source of Therapeutic Mesenchymal Stem/Stromal Cells. Front. Bioeng. Biotechnol. 8:860. doi: 10.3389/fbioe.2020.00860
Received: 07 May 2020; Accepted: 03 July 2020;
Published: 28 July 2020.
Edited by:
Roberto Narcisi, Erasmus University Rotterdam, NetherlandsReviewed by:
Cristóbal Orellana, Corporació Sanitària Parc Taulí, SpainCopyright © 2020 Greif, Kouroupis, Murdock, Griswold, Kaplan, Best and Correa. This is an open-access article distributed under the terms of the Creative Commons Attribution License (CC BY). The use, distribution or reproduction in other forums is permitted, provided the original author(s) and the copyright owner(s) are credited and that the original publication in this journal is cited, in accordance with accepted academic practice. No use, distribution or reproduction is permitted which does not comply with these terms.
*Correspondence: Diego Correa, ZHhjODIxQG1lZC5taWFtaS5lZHU=
†These authors have contributed equally to this work
‡ORCID: Diego Correa orcid.org/0000-0002-9004-4300
Disclaimer: All claims expressed in this article are solely those of the authors and do not necessarily represent those of their affiliated organizations, or those of the publisher, the editors and the reviewers. Any product that may be evaluated in this article or claim that may be made by its manufacturer is not guaranteed or endorsed by the publisher.
Research integrity at Frontiers
Learn more about the work of our research integrity team to safeguard the quality of each article we publish.