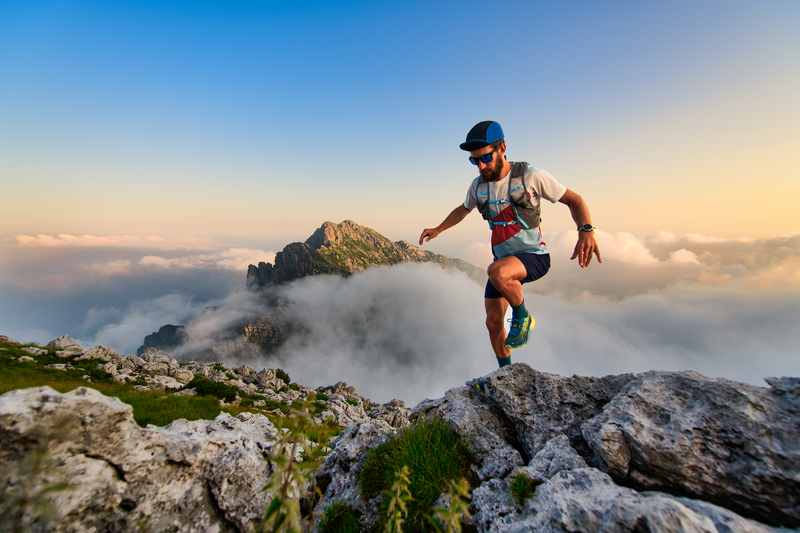
95% of researchers rate our articles as excellent or good
Learn more about the work of our research integrity team to safeguard the quality of each article we publish.
Find out more
ORIGINAL RESEARCH article
Front. Bioeng. Biotechnol. , 24 April 2020
Sec. Bioprocess Engineering
Volume 8 - 2020 | https://doi.org/10.3389/fbioe.2020.00355
Single cell oil (SCO) produced by oleaginous yeasts is considered as a sustainable source for biodiesel and oleochemicals since its production does not compete with food or feed and high yields can be obtained from a wide variety of carbon sources, e.g., acetate or lignocellulose. Downstream processing is still costly preventing the broader application of SCO. Direct transesterification of freeze-dried biomass is widely used for analytical purposes and for biodiesel production but it is energy intensive and, therefore, expensive. Additionally, only fatty acid esters are produced limiting the subsequent applications. The harsh conditions applied during direct esterification might also damage high-value polyunsaturated fatty acids. Unfortunately, universal downstream strategies effective for all yeast species do not exist and methods have to be developed for each yeast species due to differences in cell wall composition. Therefore, the aim of this study was to evaluate three industrially relevant cell disruption methods combined with three extraction systems for the SCO extraction of two novel, unconventional oleaginous yeasts, Saitozyma podzolica DSM 27192 and Apiotrichum porosum DSM 27194, based on cell disruption efficiency, lipid yield, and oil quality. Bead milling (BM) and high pressure homogenization (HPH) were effective cell disruption methods in contrast to sonification. By combining HPH (95% cell disruption efficiency) with ethanol-hexane-extraction 46.9 ± 4.4% lipid/CDW of S. podzolica were obtained which was 2.7 times higher than with the least suitable combination (ultrasound + Folch). A. porosum was less affected by cell disruption attempts. Here, the highest disruption efficiency was 74% after BM and the most efficient lipid recovery method was direct acidic transesterification (27.2 ± 0.5% fatty acid methyl esters/CDW) after freeze drying. The study clearly indicates cell disruption is the decisive step for SCO extraction. At disruption efficiencies of >90%, lipids can be extracted at high yields, whereas at lower cell disruption efficiencies, considerable amounts of lipids will not be accessible for extraction regardless of the solvents used. Furthermore, it was shown that hexane-ethanol which is commonly used for extraction of algal lipids is also highly efficient for yeasts.
Microbial triacylglycerols (SCO) which are produced by oleaginous bacteria, algae, yeast, and fungi are promising sustainable platform chemicals. SCOs are chemically equivalent to plant oils, but can be produced independent of season, climate and location using a wide range of cheap and abundant carbon sources including waste streams from food and other agricultural based industries (Yousuf et al., 2010; Ling et al., 2013; Kot et al., 2017) or renewable carbon sources (Alvarez et al., 1992; Liu et al., 2015; Fei et al., 2016) and therefore do not compete with food or feed. In particular, SCO production with yeasts is advantageous because of their fast growth rate and greater convenience to scale up cultivation than that of autotrophic microalgae since no light is needed (Li et al., 2008; Ageitos et al., 2011; Ochsenreither et al., 2016). Oleaginous yeast lipids can be used to produce biodiesel, and might find application in food industry and as building blocks for biopolymers (Vasconcelos et al., 2019). Pilot plant scale production of yeast SCO increases the potential of these microorganisms. Xue et al. (2010) cultivated Rhodotorula glutinis on starch wastewater in a 300 L scale and reached 35% lipid content. Moreover, Soccol et al. (2017) successfully processed a fed-batch strategy using sugarcane juice with Rhodosporidium toruloides in a 1000 L plant. Thereby, a 6.3 fold higher biodiesel yield was reached compared to standard biodiesel from soybean.
Despite all these advantages, commercial SCO production is restricted to high-value oils containing high amounts of polyunsaturated fatty acids for nutritional purposes (Ratledge, 2004; Mendes et al., 2009; Ji et al., 2014). Production of SCO resembling plant oils is currently too expensive to allow commercialization (Ratledge and Cohen, 2008; Leong et al., 2018). One major obstacle is still the efficient extraction and purification of SCO since high costs arise from energy and labor expenditure to recover the intracellular lipids. The rigidity and robustness of the yeast cell wall mainly contributes to the resistance to organic solvents. These circumstances have an evolutionary background to protect and adapt the cell to environmental conditions by building backbones of cross-linked glucan fibers, mannoprotein, and chitin (Phaff, 1971; Jacob, 1992). On this account, an efficient cell disruption is mandatory for successful lipid recovery and needs to be optimized for each yeast strain, since the cell wall composition may vary considerably between species. In literature lots of attempts using various methods and different species can be found (Jin et al., 2012; Zhang et al., 2014; Meullemiestre et al., 2016; Vasconcelos et al., 2018). However, rating the methods is difficult, as reported results of different laboratories may not be comparable. Although the importance of cell disruption for lipid extraction efficiency has been recognized (Rakesh et al., 2015; Ramola et al., 2019), cell disruption is usually not determined and therefore, thresholds for effective extractions have not been communicated.
In this study, we evaluate combinations of cell disruption and extraction methods on two by Schulze et al. (2014) isolated oleaginous yeasts, Saitozyma podzolica DSM 27192 and Apiotrichum porosum DSM 27194. These unconventional yeasts have been recently characterized as co-producer of intracellular SCO and extracellular gluconic acid, when cultivated on glucose (Schulze et al., 2014; Qian et al., 2019). Additionally, Qian et al. (2020) demonstrated that both yeasts are robust SCO producers capable of using the cheap industrial by-product acetate. However, the interest in these yeasts was only focused on upstream processing. We are the first to provide any kind of insights into lipid downstream methods processed with these new yeasts including an extensive evaluation. The workflow is illustrated in Figure 1. Mechanical cell disruption methods, namely bead-milling (BM) (mechanical disruption by grinding), HPH (mechanical disruption by pressure) and ultrasonification (U) (mechano-physical disruption by cavitation) were implemented because of their great industrial potential and the use at a large scale in other industries (Chisti and Moo-Young, 1986; Rodríguez-Alcalá et al., 2009; Ochsenreither et al., 2016). These disruption methods were applied on frozen, wet yeast biomass prior to chloroform methanol extraction according to Folch (F) (Folch et al., 1957) and Bligh and Dyer (BD) (Bligh and Dyer, 1959), which are the most popular lipid extraction methods in literature and known for their efficiency (Schmid et al., 1973) in lipid recovery at laboratory-scale. Additionally, the ethanol and hexane (EH) system, which is commonly used in food industry (Grima et al., 1994; Cheng and Rosentrater, 2017), was tested in terms of whole lipid extraction yield. The extracted whole lipids, including storage and membrane lipids are applicable for oleochemical building blocks production.
Figure 1. Overview of performed methods for lipid recovery. Red framed boxes were analyzed for both yeasts. The black framed methods were implemented on S. podzolica only. FAMEs, fatty acid methyl esters; FFAs, free fatty acids.
Further investigations were performed on lipid profiles after enzymatic hydrolysis to FFAs and transesterification to FAME via HPLC-ELSD and GC-FID, respectively. All results were also compared with the lipid yield and profile from direct transesterification (DT) of freeze-dried biomass (physico-chemical treatment by heat and alkali or acid), which serve as control as it was the only lipid determination method in previous upstream studies (Schulze et al., 2014; Qian et al., 2019, 2020). The derivatization to FAME directly serves the purpose to produce biodiesel (Li et al., 2008; Sathish et al., 2014; Yousuf et al., 2017), whereas valuable FFA can be applied as nutritional supplements (Whigham et al., 2000; Smedman and Vessby, 2001; Kim et al., 2016).
The potential of new production hosts should be known as precisely as possible, therefore, it is necessary to evaluate product concentration and the effort and costs of processing. The aim is to classify different downstream processing methods in terms of their efficiencies and gentleness of lipid recovery using two new oleaginous yeasts.
The oleaginous basidiomycetes examined in this study were newly screened and deposited at the DSMZ culture collection (Deutsche Sammlung von Mikroorganismen und Zellkulturen, Brunswick, Germany) as Cryptococcus podzolicus DSM 27192 and Trichosporon porosum DSM 27194 by Schulze et al. (2014). After genome sequencing and annotation both yeasts were phylogenetically reclassified to S. podzolica DSM 27192 (Aliyu et al., 2019) and A. porosum DSM 27194 (Gorte et al., 2019), respectively.
All utilized chemicals were purchased either from Carl Roth GmbH & Co. KG (Karlsruhe, Germany) or Sigma-Aldrich Chemie GmbH (Taufkirchen, Germany) if not stated otherwise.
Saitozyma podzolica and A. porosum were cultivated in a 2.5 L Minifors bioreactor (Infors HT, Bottmingen, Switzerland) as described by Schulze et al. (2014). The cultivation of S. podzolica was performed at 22.5°C and pH 4, while A. porosum was grown at 25°C and pH 5. For SCO production, glucose was used as carbon source with initial concentration of 50 g/L. Each day, glucose was replenished manually to 90 g/L after determining the consumed carbon amount. After 96 h the cultivation broth was harvested in 50 mL aliquots by centrifugation at 4800 × g for 10 min. The supernatant was discarded and the biomass was preserved for 1 week at −20°C for downstream processing using BM, HPH, or U combined with chloroform-methanol extraction according to Bligh and Dyer (BD) and Folch (F), or by using ethanol-hexane (EH) as extractants.
Nile red staining was applied according to the staining protocol of Silve et al. (2018). Yeast cells concentration was adjusted to 0.1 g/L in 1 mL of 50 mM potassium phosphate buffer pH 7.4 and centrifuged for 5 min at 6000 × g. Afterward, 200 μL of the supernatant was withdrawn and replenished with 200 μL of nile red stock solution (30 μg/mL in DMSO). The samples were mixed prior to a 10 min incubation period at 40°C. After that, the samples were washed with distilled water. For microscopic imaging the microscope Axioplan 2 (Zeiss, Jena, Germany) was used equipped with a × 63 LD Plan-Neofluar magnifying objective (Zeiss, Jena, Germany) and Axiocam HRc (Zeiss, Jena, Germany). For fluorescence imaging the fluorescence filter set 09 from Zeiss was used, i.e., the following filters: excitation BP 450–490, beam splitter FT 510, emission LP 515.
The amount of total cells before and after disruption was visually counted under a microscope using Improved Neubauer (7178 05) counting chambers, consisting of large squares subdivided into 25 group squares of 0.04 mm2 area. The chamber’s depth is 0.1 mm. Six group squares were counted per each 1:1000 diluted sample. For total cell count calculation, the average amount of cells per group square (N) was determined and used for Eq. (1).
For cell disruption the frozen biomass was thawed and washed twice with distilled water and resuspended in 50 mM potassium phosphate buffer pH 7.4 to a concentration of 100 g/L. After each disruption method the actual concentration of biomass was determined gravimetrically with a precision balance. In a pre-dried and pre-weighed 1.5 mL reaction tube 1 mL disrupted cells solution was provided and dried for 24 h at 100°C. Additionally, the same procedure was performed with 1 mL pure 50 mM potassium phosphate buffer. The weight of the buffer was subtracted from the weight of the biomass according to Eq. (2).
The homogenizer EmulsiFlex-C3 (Avestin Europe GmbH, Mannheim, Germany) was used with a self-established continuous loop system by bridging the distance between the device outlet and the sample funnel with a 1 m silicone hose. During preliminary disruption experiments treatment time was optimized. HPH was processed in a volume of 15 mL of cell suspension at 2000 bar for 5 min (20 s per loop), resulting in 15 passes per sample. Subsequently, the sample was collected in a reaction tube on ice.
All BM experiments were performed using the bead mill MM 300 (Retsch GmbH, Haan, Germany) in 1.5 mL superspin microtubes (20170-030; VWR International GmbH, Darmstadt, Germany) with 425–600 μm acid washed glass beads (G8772; Sigma-Aldrich Chemie GmbH, Taufkirchen, Germany). Sample and glass beads were distributed in 1:1 ratio (v/v) in the microtubes. In preliminary experiments disruption time and frequency was optimized: the milling process was set at the frequency of 30 Hz/s for 20 min. Thereafter, the suspensions were pooled before extraction.
Cell disruption by ultrasonification was operated with the 20 kHz ultrasonic homogenizer Sonopuls HD 3100 equipped with the probe MS 72 (Bandelin electronic GmbH & Co. KG, Berlin, Germany). The optimal amplitude, number of cycles and sonication time were determined in preliminary experiments. For the presented experiments, 30 ml cell suspension cooled on ice was sonicated using the maximum amplitude of 97% in a cycle of 50 s pulsing and 10 s pause for 3 × 10 min.
All extraction experiments were implemented in triplicates. 1 mL of disrupted cell suspension was used per extraction. As negative control, 1 mL of 50 mM potassium phosphate buffer pH 7.4 was processed.
The miniaturized version of the methods of Folch (F) (Folch et al., 1957) and Bligh and Dyer (BD) (Bligh and Dyer, 1959), as adapted by Vasconcelos et al. (2018), were slightly modified in this study. F was performed by combining 1.9 mL potassium phosphate buffer, 1 mL disrupted cells suspension, 9.66 mL chloroform and 4.83 mL methanol. For BD 5.525 mL potassium phosphate buffer were added to 1 mL sample, followed by 7.25 mL chloroform and 7.25 mL methanol. The reaction tubes were inverted 20 times, covered in aluminum foil and were shaken for 30 min. Afterward, the phase separation was accelerated by centrifugation for 5 min and 1400 × g. To collect the entire lower chloroform phase, containing the lipids, a syringe and cannula was instrumentalised to puncture the interphase. The lipid phase was dispensed into preweighed glass tubes before complete evaporation of the chloroform in the vacuum concentrator Laborota 4000 (Heidolph Instruments GmbH & Co. KG, Schwabach, Germany) at 40°C, 700 × g and 10 mbar. For each method blank extractions containing buffer without biomass were performed to exclude artifactual results, as the used plastic consumables were slightly reactive to the solvents. To determine the whole cell lipid, the weight of the remaining lipids was detected gravimetrically and calculated according to Eqs (3) and (4).
After weighting, the lipids were resuspended in 1 mL hexane and stored at −20°C prior to sample preparations for further analytical purposes.
Ethanol hexane (EH) extraction of S. podzolica biomass was conducted as described in Silve et al. (2018) with slight modifications. 15.1 mL ethanol and 6.6 mL hexane were added to 1 mL disrupted cells suspension and mixed by inverting (20 times). The samples were wrapped in aluminum foil and shaken for 3 h at room temperature during which a monophasic suspension was formed. Cell debris was pelleted by centrifugation at 4800 × g for 10 min. Afterward, 10 mL were transferred to new reaction tubes and 5 mL ddH2O and 30 mL hexane were added to induce phase separation. After mixing for 3 min and centrifugation at 4800 × g for 10 min, 20 mL of the upper hexane phase was transferred to pre-weighed glass tubes before solvents were completely evaporated in the vacuum concentrator Laborota 4000 (Heidolph Instruments GmbH & Co. KG, Schwabach, Germany) at 40°C, 700 × g and 10 mbar. Similar to the other extraction methods, blank extractions without biomass were performed to exclude artifacts. The weight of the extracted lipids was determined with a precision balance and the whole cell lipid was calculated using Eqs (3) and (5).
∗Total volume monophase;
∗∗2.9 mL (ratio of hexane in 10 mL monophase) + 30 mL hexane (added for phase separation).
The extracted lipids were resolved in 1 mL hexane and stored at −20°C prior to sample preparations for further analytical purposes.
For enzymatic hydrolysis of the standard triglycerol trilinolein (T1388; TCI Deutschland GmbH, Eschborn, Germany) and extracted lipids, the method of Guauque Torres et al. (2014) was slightly adapted. 25 mg of trilinolein or extracted lipids were mixed with 0.65 g triton X-100, 1.25 mL TRIS-HCl buffer (pH 5.5), 0.5 mL distilled water and 90 mg of Candida antarctica lipase B (Novozym 435, Strem chemicals Europe, Kehl, Germany). The reaction mixture was incubated for 3 h in a NeoLab-rotator with vortex mixer (Heidelberg, Germany) at 50°C using program U2 at 20 rpm. Afterward 200 μL were used for HPLC analysis. All samples were processed in triplicates.
Free fatty acids were determined by reversed-phase HPLC using a Kinetex EVO C18 (2.6 μm, 250 mm × 4.6 mm) from Phenomenex (Aschaffenburg, Germany). The HPLC system consisted of a pump, auto sampler and column oven from Agilent Technologies (Waldbronn, Germany). The fatty acids were separated in a binary gradient of acetonitrile (A) and water (B) with a flow rate of 1 mL/min. The injection volume was set to 10 μL and the temperature of the column oven to 50°C. The elution conditions were the following: 0–7 min isocratic 75A:25B, 7–17 min linear gradient up to 80A:20B, 17–22 min linear gradient up to 95A:5B, 22–30 min isocratic 95A:5B, followed by a reconditioning step of the column to 75A:25B for 5 min. FFAs were detected with an ELSD from Grace (Essen, Germany) at 38.1°C with a gas flow of 1.45 mL/min and the gain was set to 4.
For GC analysis, extracted SCOs were transesterified to FAMEs in a two phase system using two different catalysts. All experiments were performed in triplicates. Transesterification efficiency of both catalysts was determined by using 25 mg trilinolein as control.
For acidic transesterification, 0.5 mL internal standard consisting of 2 mg/mL heptadecanoic acid and additional 0.5 mL hexane were added to 1 mL extracted lipids or 25 mg of standard in hexane. An equal volume of 2 mL 15% H2SO4 in methanol was added as catalyst. The reaction mixtures were incubated for 2 h at 100°C and 1000 rpm in a thermo-shaker (Universal Labortechnik, Leipzig, Germany). Samples were additionally mixed every 30 min by vortexing. To stop the reaction, the tubes were placed on ice for 10 min. To improve phase separation, 1 mL distilled water was added. The upper hexane phase containing FAMEs was transferred for GC analysis.
Alkaline transesterification of extracted lipids and 25 mg standard was done at 60°C and 1000 rpm for 20 min with 2 mL of 5% KOH in methanol as catalyst. 2 mg/mL of methyl benzoate was utilized as internal standard.
For direct transesterification (DT) the standard triglycerol trilinolein and biomass samples were processed. 15 ml of fresh biomass was taken at the end of the cultivation process and washed with 0.9% NaCl. The pellet was freeze-dried for 24 h at −30°C and 0.370 mbar using the BETA 1-8 freeze dryer (Christ, Osterode am Harz, Germany). 20 mg of freeze dried biomass or 25 mg of the trilinolein were applied. Subsequently, an acidic and alkaline transesterification was performed as described above.
The quantitative and qualitative analysis of the transesterified FAMEs were performed gas-chromatographically using the 6890 N Network GC-System (Agilent Technologies Deutschland GmbH, Waldbronn, Germany). The device was coupled with a DB- Wax column (122–7032; l: 30 m d: 0.25 mm, Agilent Technologies Deutschland GmbH, Böblingen, Germany) and the detection was performed with a FID under 1.083 bar working pressure. 1 μL of sample was injected at the initial temperature of 40°C. The separation of the FAMEs was achieved by a temperature gradient from 40 to 250°C with a rate of 8°C/min and was kept for 10 min at 250°C. To identify and quantify the FAMEs the RM3 FAME Mix standard (07256-1AMP; Sigma Aldrich, Taufkirchen, Germany) was used.
Origin Software [version 2019 (9.6)] was used for statistical analysis. One-way ANOVA followed by post hoc-test Tukey were performed using p-value < 0.05.
Different combinations of mechanical cell disruption methods and solvent systems for lipid extraction were applied to both yeasts as outlined in Figure 1. Each step was evaluated for its efficiency and all combinations were subsequently compared with direct transesterification of freeze-dried biomass, which was used as the only analysis method for SCO production with these yeasts in former studies.
For both yeasts, disruption efficiencies of the three applied methods were optically determined and compared via nile red fluorescence assay and light microscopy prior to whole lipid extraction. Figure 2A provides microscopic images of untreated and disrupted cells of S. podzolica. After bead-milling (BM) and HPH mainly cell debris were observed, whereas after treatment with ultrasound (U) most of the cells seemed still to be intact. Total cell count confirmed the microscopical observation: after BM treatment 93% of the cells were found to be disrupted, 95% after HPH, but only 27% after treatment with U, although maximal amplitude was applied. Disruption of the cells probably enables lipid bodies to escape and to aggregate in aqueous buffer solution, which can be observed in the nile red images. Furthermore, HPH treatment might lead to emulsification by reduction of lipid droplet size as it is used for this purpose in the dairy industry. Therefore, fluorescence intensity of S. podzolica seems to be reduced after HPH treatment. The effect of the cell disruption approaches on A. porosum is shown in Figure 2B. Similar to S. podzolica, BM and HPH also seemed to be more efficient compared to U. However, all three treatments appeared to be less destructive to A. porosum than to S. podzolica, which was also confirmed by total cell count. After BM, HPH and U only 74, 53, and 8% of the cells were disrupted, respectively.
Figure 2. Light microscopy pictures of untreated and via BM, HPH and U mechanically disrupted, nile red stained yeast cells. (A) S. podzolica, (B) A. porosum. BM, bead mill; HPH, high pressure homogenization; U, ultrasonic treatment.
After cell disruption whole lipid was extracted either by chloroform and methanol using two different protocols [according to Folch (F) and Bligh and Dyer (BD)] or by ethanol and hexane (EH). Latter extraction was only performed for S. podzolica. Whole cell lipid extraction yields per CDW are illustrated in Table 1. For S. podzolica, whole lipid yield and cell disruption efficiency are clearly correlated. Therefore, highest amounts of whole cell lipid were obtained after HPH, which was the most efficient cell disruption method, followed by BM and U regardless of the solvent system used for extraction. Consistently, extraction efficiency was always superior with EH, followed by BD and F for all cell disruption methods. The best method for whole cell lipid extraction of S. podzolica is therefore the combination of HPH and EH (46.9 ± 4.4%) which is 2.7 times higher than with the least favorable combination (U and F; 17.3 ± 3.1%). Observed differences are in most cases statistically significant, however, no significant difference was detected between HPH-EH and HPH-BD. Hence, both methods, HPH-BD and HPH-EH, are equally well-suited to achieve the best whole cell lipid yield for S. podzolica.
Table 1. Extracted whole cell lipid yields per cell dry weight (CDW) of S. podzolica and A. porosum after different cell disruption methods and solvents.
As expected from low disruption efficiencies, extracted whole cell lipid yields of A. porosum are also generally low (Table 1), i.e., lower than published lipid contents extracted by direct transesterification. The best combination was BM and BD with 20.0 ± 3.2%. Although highest whole lipid yields were also achieved from the more efficient cell disruption method (BM), trends are not as clear as for S. podzolica and observed differences are not statistically significant. The data for extracted whole cell lipid after U are not shown for A. porosum since cell disruption was not sufficient as already mentioned above.
Quantification of lipids can be achieved indirectly via FAMEs by GC or in form of FFAs by HPLC. For both methods, sample preparation is necessary and might have an impact on (calculated) amount and fatty acid composition leading to underestimation of both lipid yield and quality.
Transesterification of fatty acids derived from triacylglycerols/SCOs to FAMEs can be achieved by both acidic and alkaline methylation with H2SO4 or KOH as catalyst, respectively. For both methods transesterification efficiency and impact on polyunsaturated fatty acids were investigated by using the standard triacylglycerol trilinolein. FAME yield with KOH was 92.3 ± 2.8%, whereas, H2SO4 derivatization resulted in a FAME yield of 97.1 ± 3.1%. The results for transesterification of extracted lipids from biomass are shown in Supplementary Table S1. No significant difference was observed between F and BD extractions for both yeasts after transesterification. On that account, Figure 3 exemplifies the comparison with KOH and H2SO4 produced FAMEs after HPH-BD treatment and direct transesterification of yeast biomass. For S. podzolica (Figure 3A) both catalysts achieved an equal yield for prior disrupted and extracted lipids. For direct transesterified biomass the KOH technique is four times less efficient compared to acidic transesterification. Statistically, all three successful methods (disrupted and extracted lipids treated with KOH and H2SO4 and DT-H2SO4) are equally potent to achieve high FAME yields. Figure 3B likewise indicates no difference for A. porosum in transesterification catalyst for recovered lipids. Similarly to S. podzolica, alkaline direct transesterification is not convenient and nine times less potent compared to acidic technique for untreated biomass. Therefore, DT-H2SO4 is the most effective method to produce FAMEs from A. porosum biomass.
Figure 3. Comparison of KOH and H2SO4 catalysts for FAME production after HPH-BD treatment and direct transesterification of yeast biomass. % FAME per CDW from S. podzolica biomass is demonstrated in (A), while (B) presents the same for A. porosum. The standard deviation of three independent experiments are indicated by the error bars. a, b, c illustrate statistical differences (p = 0.05). FAMEs, fatty acid methyl esters; BM, bead mill; HPH, high pressure homogenization; DT, direct transesterification; CDW, cell dry weight; BD, extraction according to Bligh and Dyer.
Since for processed lipids both catalysts are appropriate and for DT the acidic method is more adequate, Figure 4 contrasts the comparison of all disruption methods using BD extraction and DT after acidic transesterification. Figure 4A elucidates for S. podzolica with BM, HPH, and DT a statistically comparable FAME yield at approximately 30% per CDW. On the contrary, U is less efficient with 23.8 ± 2.3% FAME/CDW. However, EH after HPH is significantly the optimal method with the highest lipid yield (Supplementary Table S1). Different findings were observed for A. porosum (Figure 4B). By performing direct transesterification, the highest yield of 27.2 ± 0.5% FAME/CDW was detected. BM with 20.2 ± 1.2 and HPH with 13.4 ± 0.7% FAME/CDW are significant less sufficient methods to extract the lipids from this yeast.
Figure 4. Comparison of all processed lipid recovery methods, using BD extraction and acidic transesterification, and acidic DT method. (A) % FAME per CDW from S. podzolica biomass. (B) % FAME per CDW from A. porosum biomass. The error bars result from the standard deviation of three independent experiments. a, b, c reveal statistical differences (p = 0.05). FAMEs, fatty acid methyl esters; CDW, cell dry weight; BM, bead mill; HPH, high pressure homogenization; DT, direct transesterification; BD, extraction according to Bligh and Dyer.
Enzymatic hydrolysis was used to convert triacylglycerols to FFA prior to HPLC analysis. Investigation of this method using trilinolein showed a conversion rate to FFA of 56.8 ± 2.6%. No statistical difference was observed between the extraction methods F and BD, whereas EH (37.0 ± 1.1% FFA/CDW with BM) was significantly more efficient than BD (18.0 ± 0.4% FFA/CDW with BM) and F (18.6 ± 0.5% FFA/CDW with BM). Cell disruption was shown to have a significant effect on FFA yields. BM (18.6 ± 0.5% FFA/CDW with F) and HPH (16.7 ± 0.9% FFA/CDW with F) resulted in significantly higher yields than U (11.2 ± 1.2% FFA/CDW with F) for S. podzolica (Supplementary Table S1).
For A. porosum BM (16.5% FFA/CDW) was a significantly more efficient method than HPH (7.7% FFA/CDW, Supplementary Table S1). The extraction methods had no statistical influence on the FFA yield. For both yeasts similar tendencies concerning the efficiencies of the investigated methods were observed for FFA with HPLC analysis as for FAME with GC.
In order to judge which method is most suitable for extraction of oxidation sensitive polyunsaturated fatty acids, lipid profiles were determined. In terms of extraction systems, differences in lipid profiles were not observed when comparing the extraction methods F and BD (Supplementary Tables S2, S3). In contrast, extraction with EH resulted in significantly higher amounts of stearic acid and significantly lower amounts of linoleic acid.
With regard to cell disruption, no or only minor differences in lipid profile for S. podzolica biomass were detected when using HPH and BM (Supplementary Table S2). Analysis of the lipid profile of A. porosum, however, revealed significantly more linoleic acid and significantly less stearic acid for BM compared to HPH (Supplementary Table S3).
Additionally, the investigated transesterification techniques did influence the lipid profiles for both yeasts. Figure 5 compares fatty acid profiles of both yeasts obtained by BM + BD and acidic direct transesterified biomass. Extracted and with KOH transesterified lipids of S. podzolica resulted in higher amounts of oleic acid and linolenic acid compared to lipids transesterified with H2SO4 (Figure 5A). However, lowest linoleic acid yields were detected with KOH transesterification of extracted lipids. No significant difference on lipid profile of DT and disrupted and H2SO4 treated lipids were observed for S. podzolica.
Figure 5. Fatty acid distribution of in situ transesterified and BM prior to BD processed biomass analyzed by HPLC and GC. (A) Fatty acid profiles in% per CDW of S. podzolica. (B) Fatty acid distribution in% per CDW of A. porosum. DT, direct transesterification; BD, extraction according to Bligh and Dyer.
Similar to S. podzolica, A. porosum (Figure 5B) showed lower oleic acid yields for disrupted and H2SO4 handled lipids compared to DT or extracted and KOH treated lipids. For A. porosum linoleic acid yield is significant higher for DT than for the other disruption methods.
Comparison of FAME and FFA quantification indicates that yields for the saturated fatty acids, palmitic acid and stearic acid, are significantly higher and linoleic acid amounts are significantly lower represented in FFAs than in FAMEs for both yeasts. No significant difference was observed for oleic acid between FFAs and FAMEs with the exception that significantly higher oleic acid levels were detected with GC KOH for S. podzolica.
Mechanical cell disruption methods ensure cell wall disruption by forces of shear, abrasion and cavitation due to high pressure, velocity, heat, or sonication (Jacob, 1992; Ochsenreither et al., 2016; Probst et al., 2016). In this study the tendencies of all three cell disruption methods on wet, but frozen and thawed, biomass are similar for both yeasts, indicating HPH and BM as potent disruption methods, whereas U resulted to be least effective regardless of the fact, that the maximum amplitude was used. In literature sonication is known to be unsuitable for microorganisms inhabiting rigid and tough cell walls (Jacob, 1992; Yu et al., 2015; Probst et al., 2016). However, due to the different definitions and measurements a comparison of cell disruption methods in literature is difficult. For the ascomycete Yarrowia lipolytica, e.g., ultrasound turned out to be one of the most efficient techniques for lipid recovery (Zhang et al., 2014; Meullemiestre et al., 2016).
The cell wall structure of ascomycetous yeasts usually consists of two layers including an inner layer with polysaccharides and an outer layer bearing glycoproteins covalently bound to the inner layer. In contrast, for some basidiomycetes multilayered cell walls were observed (Van Der Klei et al., 2011). The difference in cell layer quantity may contribute to the higher cell wall rigidity of the basidiomycetes presented in this study compared to ascomycetous yeasts. HPH and BM enforce high shear stress to the cells, either by pressurizing cells through a small valve followed by striking a wall at high velocity in case of HPH or by impact with agitated grinding beads in case of bead-milling (Chisti and Moo-Young, 1986; Middelberg, 1995; Probst et al., 2016). The SCO of the oleaginous yeast Cryptococcus curvatus (Thiru et al., 2011) and the microalgae Nannochloropsis sp. (Halim et al., 2016) were recovered after HPH treatment, though yields of other disruption strategies were not compared. Whereas for Y. lipolytica (Meullemiestre et al., 2016) and for the yeast C. curvatus, the fungus Mortierella isabellina and the microalga Chlorella sorokiniana (Yu et al., 2015) among other methods BM was compared with U, resulting, similarly to this study, in higher effective cell disruption after BM.
Considering the total cell count and the microscopic images after disruption (Figure 2) A. porosum was less affected by mechanical disruption than S. podzolica. This might be due to differences in cell wall compositions of both yeasts. Since both yeasts are newly screened structural analysis of the cell walls does not exist in literature. In general, the yeast cell wall consists of heterogeneous and complex cross-linked polymers of oligosaccharides (glucan, mannoprotein, chitin). The highly elastic β-(1,3)- glucan chains and rigid β-(1,6)-glucan crosslinks contribute to the stability and firmness (Phaff, 1971; Jacob, 1992). In addition, a cell wall is reinforced in stationary growth phase by chitin crosslinking and increased mannoprotein binding (De Nobel et al., 1990; Touhami et al., 2003). Comparisons of cell wall composition and organization indicated high variabilities between different fungal organisms (Free, 2013). Especially remarkable is the ability of some species to form an exterior capsule of carbohydrate polymers (Doering, 2009), which are known to be produced by the members of the family Trichosporonaceae (Duarte-Oliveira et al., 2017) to which A. porosum belongs (Aliyu et al., 2020) and which might also contribute to the challenge to efficiently disrupt the cell.
The most effective lipid recovery method for A. porosum is the acid catalyzed direct transesterification of freeze-dried biomass, in which cell disruption, lipid extraction, and transesterification to FAMEs is combined in a single reaction under harsh conditions at 100°C for 2 h. In contrast, the direct transesterification with KOH using milder conditions (60°C for 20 min) turned out to be insufficient for direct transesterification, which proves the necessity of high temperature for direct FAME production for both tested yeasts. However, freeze drying as pre-treatment of the biomass is critical for this method. Moisture considerably decreases the yield of the direct transesterification (Sathish et al., 2014; Yousuf et al., 2017). The moisture content of wet oleaginous microorganisms is over 80% and therefore, higher than in oilseeds and needs to be removed (Ehimen et al., 2010; Jin et al., 2012). Consequently, freeze drying is absolutely necessary though it is the most energy consuming technique. For biodiesel, a low value product, this strategy contributes mainly to production costs.
In comparison, S. podzolica is easier to break with equal yields after derivatization of both wet biomass, disrupted using HPH and BM, and dry biomass using DT (Figure 4). In fact, this yeast is a potential candidate for biodiesel or oleochemical production. Cell disruption is the decisive step for SCO extraction in yeasts. At an efficiency of over 90% of disrupted cells according to total cell count (S. podzolica: 93 and 95% after BM and HPH, respectively) the conditions are suitable for subsequent extraction, at lower cell disruption efficiencies (A. porosum: 74% by BM and 53% after HPH), only a limited part of the SCO can be extracted.
Depending on the purpose of the extracted lipid a suitable method for downstream processing can be chosen by taking the energy costs and labor into account. In Table 2 the amount of required energy for 1 kg of extracted SCO is presented for each performed downstream method and both yeasts. The energy consumption to extract whole lipid of S. podzolica is about 100 kWh/kg for all tested methods. This is derived from the extraction efficiency, disruption time and the method’s device power consumption, e.g., U is the least efficient but also the least power consuming method, therefore more biomass must be treated to reach 1 kg whole lipid resulting in comparable energy consumption in kWh/kg as with the other methods. However, the most energy efficient methods are BM and HPH prior the EH extraction with 96 and 95 kWh/kg, respectively. For whole lipid extraction from A. porosum nearly twice as much energy is required compared to S. podzolica, which is due to the low achieved cell disruption efficiency of this strain. On this account, A. porosum is less appropriate for potential industrial use.
Regarding the energy consumption for FAME production for application as biodiesel, the most energy saving method is HPH-EH-KOH for S. podzolica with 200 kWh/kg. For A. porosum, 60% more energy is needed for 1 kg FAME even when the most efficient method (BM-BD-KOH) for this yeast is used.
Comparing the energy consumption for FAME production with DT and mechanically treated biomass, DT is clearly the most energy consuming method. By performing HPH-EH-KOH with S. podzolica biomass just 3.6% of energy percentage is needed for one kg FAME compared to DT, for A. porosum biomass treated with BM-BD-KOH just 5.2% of DT’s energy amount is required. This fact results from the different required pre-treatment methods. Although the mechanically treated biomass was frozen for 168 h at −20°C, still less energy was consumed than for 24 h of vacuum freeze-drying. Consequently, freeze-drying is the most energy consuming technique, which is also confirmed by other studies (Meullemiestre et al., 2016). By considering the extrapolated data from laboratory scale in this study, S. podzolica is more suitable for potential industrial application for both, whole lipid recovery for oleochemicals as well as for transesterification for biodiesel production.
Within this study a higher SCO production potential of the yeast S. podzolica is proven, compared to the previous studies performing only DT with this yeasts’ biomass (Schulze et al., 2014; Qian et al., 2019, 2020). Moreover, the downstream process using HPH and ethanol-hexane extraction prior to transesterification is not only more efficient but also more energy saving than DT. However, there is still optimization potential regarding energy efficiency, e.g., shorter pre-treatment times and higher biomass scales need to be investigated.
Oleaginous yeasts produce neutral lipids, such as triacylglycerols as major component, di- and mono-acylglycerols, FFAs and sterols. These molecules are accumulated in lipid bodies surrounded by polar phospholipids and serve for energy storage. Polar lipids, like phospholipids, sphingolipids and glycolipids, are incorporated in the flexible cell membrane (Brown, 2001; Breil et al., 2017). To recover the whole lipid of microorganisms generally a system of polar and non-polar solvents is applied. The polar cosolvent is needed to break up the protein layer surrounding the lipid droplets in order to make the lipids accessible to the non-polar solvent. The most famous and efficient standard techniques are according to Folch (Folch et al., 1957) and Bligh and Dyer (Bligh and Dyer, 1959) using the solvents chloroform and methanol. However, chloroform is a highly toxic and carcinogenic solvent and its usage is therefore prohibited in the food industry (Grima et al., 1994; Breil et al., 2016). In the food industry, EH is used as low-toxicity alternative in lipid extraction of oil seeds and canola (Grima et al., 1994; Biondo et al., 2015). This extraction method is also well-established for algal lipids (Silve et al., 2018). For yeast cells, however chloroform-methanol extraction is still the most common method even though Èertík et al. (1996) already showed that EH is also suitable for Mucor mucedo. In contrast to chloroform, hexane is non-carcinogenic but still neurotoxic (Joshi and Adhikari, 2019). After all, there is no alternative to hexane in lipid extraction (Breil et al., 2016). For this reason, the less harmful solvents ethanol and hexane were also considered for lipid extraction in the present study.
Analyzing the extraction techniques on whole lipid extraction, BD was more effective compared to F for S. podzolica probably because the ratio of the polar methanol is higher in BD leading to a more efficient extraction of polar lipids (Dong et al., 2016; Vasconcelos et al., 2018). For A. porosum both extraction methods showed no significant differences, presumably due to the insufficient cell disruption. After transesterification, however, F und BD extraction revealed the same yield of transesterifiable lipids for both yeasts proving an equal ability of the methods to recover neutral lipids.
The less harmful EH solvent mixture turned out to be highly suitable for SCO purification of S. podzolica. After HPH treatment EH extraction proved to be similarly potent as BD extraction and the highest whole cell lipid yields were achieved. Remarkably, after derivatization of the HPH treated biomass the highest FAME yields resulted from EH extracted lipids with 46.0 ± 6.8% FAME/CDW. Compared to the former standard analysis DT (30.0 ± 1.3% FAME/CDW) with this new approach 53% increase of production potential of S. podzolica was reached. Since HPH is the most effective disruption method for S. podzolica, here the highest amount of lipids was accessible for extraction. Hexane has a lower polarity than chloroform, consequently neutral lipids have a higher affinity to non-polar solvents (Cooney et al., 2009). Thus, more transesterifiable acylglycerols and FFAs should be accessible for EH extraction. Additionally, the EH method was completed in 3 h, whereas BD was carried out in 30 min. The longer extraction time might also contribute to this result. In literature ethanol-hexane extractions are commonly used for microalgae lipid recovery with likewise high extraction effectiveness (Grima et al., 1994; Silve et al., 2018). For the yeast Lipomyces kononenkoae, also the less polar solvent toluene revealed higher extraction yields over chloroform (Vasconcelos et al., 2018). Hence, hazardous chloroform methanol mixtures can be dispensed with less harmful ethanol hexane system without scarifying oil recovery for S. podzolica.
The linoleic acid yield of trilinolein hydrolysis was 56.8% in this study. Candida antarctica lipase B is reported to cleave triglycerides selectively in position sn-1 and sn-3 (Rogalska et al., 1993; Villeneuve et al., 1995; Watanabe et al., 2015). For long chain fatty acids Candida antarctica lipase B has a low sn-1 selectivity and for short chain fatty acids a low sn-3 selectivity compared to a high typoselectivity for short chain fatty acids (Villeneuve et al., 1995). Therefore, enzymatic cleavage of a triglyceride leads to one monoglyceride and two FFAs under the applied conditions. Consequently, 85% of the theoretical yield are reached in this study. Theoretical and experimental FAME yield is higher than FFA yield, respectively. Therefore, FFA production is only economically feasible for high value products that are incompatible with FAME, e.g., in nutraceuticals as linoleic acid showed antiobesity, antiatherosclerosis, anticancerogenic, and immunomodulating effects in vitro and in animal studies (Whigham et al., 2000; Smedman and Vessby, 2001; Kim et al., 2016). The side products, mono acylglycerides, of the applied enzymatic fatty acid production with Candida antarctica lipase B from SCO are of interest for the food industry as they are widely applied as emulsifiers, e.g., in bakery products (Mettler and Seibel, 1993).
Comparison of FAME lipid profiles and FFA lipid profiles revealed a higher content of saturated fatty acids for FFA than for FAME. This might be due to the enzymatic hydrolysis with Candida antarctica lipase B, as this enzyme is reported to hydrolyze stearic acid faster than oleic acid, linoleic acid or linolenic acid (Nalder et al., 2014). In the case of unsaturated acids, linolenic acid is preferred to linoleic acid and oleic acid (Nalder et al., 2014). This could not be confirmed in our study as the quantification of linolenic acid was not possible due to co-elution with the emulsifier used in the reaction.
Alkaline transesterification methods are recommended for lipid analysis as they are faster, more efficient and the reaction is in general more complete than acidic transesterification (Christie, 1993; Liu, 1994; Aldai et al., 2005; Carlini et al., 2014). Another limitation of sulfuric acid catalyzed transesterification, especially for up-scaling, is possible corrosion of reaction vessels due to salt interactions (Carlini et al., 2014). However, with the alkaline methods only transesterification is possible but not the esterification of FFAs. Instead, the acidic catalysis is suitable for both esterification and transesterification (Liu, 1994; Aldai et al., 2005). Another difference between alkaline and acidic transesterification is that N-acyl lipids are not transesterified by alkaline methods but only by acid catalysis (Mayberry, 1981; Galbraith and Wilkinson, 1991; Christie, 1993). A disadvantage of acidic transesterification is that this method could cause isomerization and methoxy artifacts in conjugated fatty acids (Aldai et al., 2005). However, Yamasaki et al. (1999) showed sulfuric acid catalyzed transesterification is a relatively mild method as 71% of conjugated linoleic acid are not isomerized after 120 h of methylation (Yamasaki et al., 1999). Therefore, differences in lipid profiles between alkaline catalysis and acid catalysis might be more likely due to esterification of FFAs and N-acyl lipids with the acidic method, whereas these are not quantified with alkaline transesterification. However, the total amount of FAME is the same for both methods, as alkaline triglyceride transesterification might be more complete and therefore compensate the non-methylated FFAs and N-acyl lipids in regards to total FAME amount.
Significant differences in lipid profile of A. porosum treated with BM and HPH were observed. After HPH linoleic acid content was significantly lower than after BM. To our knowledge, there are no studies existing addressing the lipid profiles of yeasts in relation to cell disruption methods. The influence of BM on fatty acid composition was addressed only in a single publication where no differences in lipid composition of Chlorella vulgaris after BM compared to grinding, ultrasonication, enzymatic lysis, and microwaves was observed (Chi et al., 2011). Studies on the influence of HPH on the fatty acid composition of various foods provide different results. Thus, Rodríguez-Alcalá et al. (2009) reported no influence of HPH on fatty acid composition of milk up to a pressure of 3500 bar. In tomato juice an increase in the oxidation product of unsaturated fatty acids, n-hexanal, was reported after HPH treatment at a pressure of 5000 bar (Porretta et al., 1995). While Kuhn and Cunha (2012) observed a significant increase in primary oxidation products due to HPH treatment of whey protein isolate stabilized oil in water emulsions of flaxseed oil already at a pressure of 800 bar. Therefore, the differences in linoleic acid content after BM and HPH treatment might be due to oxidation of linoleic acid during HPH treatment.
The aim of the presented work was to investigate the impact of cell disruption, extraction, and transesterification methods as well as enzymatic hydrolysis on fatty acid extraction yields and their lipid profile of SCO of two unconventional yeasts, S. podzolica and A. porosum, to assess their potential economic profitability. It was shown that BM and HPH were the most appropriate cell disruption methods for S. podzolica whereas DT was best for A. porosum followed by BM. No differences could be observed between F and BD as extraction methods for derivatized lipids, while the EH system was superior for S. podzolica, which is less harmful in comparison to the highly toxic chloroform-methanol mixture. With regard to the lipid profiles, differences between BM and HPH were observed, which might be due to oxidation of linoleic acid while HPH processing. Comparison of alkaline and acidic transesterification of recovered oil revealed higher linoleic acid yield for H2SO4 catalysis. Both are similarly potent transesterification methods for recovered oil, whereas direct transesterification needs to undergo harsh conditions provided by this study’s acidic transesterification.
We are the first to evaluate the downstream process properties of this new SCO producing yeasts for potential industrial use like biodiesel or oleochemical production. Concluding from this, A. porosum is less suitable compared to S. podzolica. The oil of A. porosum is more difficult to recover and more efficient with high energy consuming pre-treatment process. However, for S. podzolica a 53% higher production potential was shown using HPH-EH prior to transesterification, compared to the former standard analysis DT.
All data generated or analyzed during this study are included in this published article and its additional file.
OG and RH designed and planned the study, analyzed the data and drafted the article supervised by KO. OG performed yeasts cultivation, oil recovery, and GC analysis. RH conducted the enzymatic hydrolysis and the statistical analysis. OG and IP performed the nile red assay. CSt enabled the use of the bead mill. AS, WF, and CSy constructively contributed to the content. All authors critically revised the article.
Bioeconomy International BMBF (grant #031B0452) supported OG. RH was supported by the European Regional Development Fund and the Ministry of Science, Research and the Arts of the State of Baden-Württemberg within the research center ZAFH InSeL (grant#32-7545.24-20/6/3). We acknowledge the support from the Deutsche Forschungsgemeinschaft and the Open Access Publishing Fund of Karlsruhe Institute of Technology.
The authors declare that the research was conducted in the absence of any commercial or financial relationships that could be construed as a potential conflict of interest.
We would like to thank Prof. Dr. Ing. Clemens Posten for the permission to use the bead mill.
The Supplementary Material for this article can be found online at: https://www.frontiersin.org/articles/10.3389/fbioe.2020.00355/full#supplementary-material
BD, Bligh and Dyer; BM, bead milling; CDW, cell dry weight; EH, ethanol hexane extraction system; ELSD, evaporative light scattering detector; F, Folch; FAME, fatty acid methyl ester; FFA, free fatty acid; FID, flame ionization detector; GC, gas chromatography; HPLC, high performance liquid chromatography; HPH, high pressure homogenization; SCO, single cell oil; U, ultrasonification.
Ageitos, J. M., Vallejo, J. A., Veiga-Crespo, P., and Villa, T. G. (2011). Oily yeasts as oleaginous cell factories. Appl. Microbiol. Biotechnol. 90, 1219–1227. doi: 10.1007/s00253-011-3200-z
Aldai, N., Murray, B. E., Nájera, A. I., Troy, D. J., and Osoro, K. (2005). Derivatization of fatty acids and its application for conjugated linoleic acid studies in ruminant meat lipids. J. Sci. Food Agric. 85, 1073–1083. doi: 10.1002/jsfa.2110
Aliyu, H., Gorte, O., Maayer, P., De Neumann, A., and Ochsenreither, K. (2020). Genomic insights into the lifestyles, functional capacities and oleagenicity of members of the fungal family Trichosporonaceae. Sci. Rep. 10:2780. doi: 10.1038/s41598-020-59672-2
Aliyu, H., Gorte, O., Neumann, A., and Ochsenreither, K. (2019). Draft genome sequence of the oleaginous yeast saitozyma podzolica (syn. Cryptococcus podzolicus) DSM 27192. Microbiol. Resour. Announc. 8:e01676-18. doi: 10.1128/MRA.01676-18
Alvarez, R. M., Rodríguez, B., Romano, J. M., Díaz, A. O., Gómez, E., Miró, D., et al. (1992). Lipid accumulation in Rhodotorula glutinis on sugar cane molasses in single-stage continuous culture. World J. Microbiol. Biotechnol. 8, 214–215. doi: 10.1007/BF01195853
Biondo, P. B. F., Dos Santos, V. J., Montanher, P. F., Junior, O. D. O. S., Matsushita, M., Almeida, V. C., et al. (2015). A new method for lipid extraction using low-toxicity solvents developed for canola (Brassica napus L.) and soybean (Glycine max L. Merrill) seeds. Analytical Methods 7, 9773–9778. doi: 10.1039/c5ay02243f
Bligh, E. G., and Dyer, W. J. (1959). A rapid method of total lipid extraction and purification. Can. J. Biochem. Physiol. 37, 911–917. doi: 10.1139/y59-099
Breil, C., Abert Vian, M., Zemb, T., Kunz, W., and Chemat, F. (2017). “Bligh and Dyer” and Folch methods for solid–liquid–liquid extraction of lipids from microorganisms. Comprehension of solvatation mechanisms and towards substitution with alternative solvents. Int. J. Mol. Sci. 18, 1–21. doi: 10.3390/ijms18040708
Breil, C., Meullemiestre, A., Vian, M., and Chemat, F. (2016). Bio-based solvents for green extraction of lipids from oleaginous yeast biomass for sustainable aviation biofuel. Molecules 21, 1–14. doi: 10.3390/molecules21020196
Brown, D. A. (2001). Lipid droplets: proteins floating on a pool of fat. Curr. Biol. 11, 446–449. doi: 10.1016/S0960-9822(01)00257-3
Carlini, M., Castellucci, S., and Cocchi, S. (2014). A pilot-scale study of waste vegetable oil transesterification with alkaline and acidic catalysts. Energy Procedia 45, 198–206. doi: 10.1016/j.egypro.2014.01.022
Èertík, M., Andráši, P., and Šajbidor, J. (1996). Effect of extraction methods on lipid yield and fatty acid composition of lipid classes containing γ-linolenic acid extracted from fungi. J. Am. Oil Chem.Soc. 73, 357–365. doi: 10.1007/BF02523431
Cheng, M. H., and Rosentrater, K. A. (2017). Economic feasibility analysis of soybean oil production by hexane extraction. Ind. Crops Prod. 108, 775–785. doi: 10.1016/j.indcrop.2017.07.036
Chi, Z., Zheng, Y., Ma, J., and Chen, S. (2011). Oleaginous yeast Cryptococcus curvatus culture with dark fermentation hydrogen production effluent as feedstock for microbial lipid production. Int. J. Hydrogen Energy 36, 9542–9550. doi: 10.1016/j.ijhydene.2011.04.124
Chisti, Y., and Moo-Young, M. (1986). Disruption of microbial cells for intracellular products. Enzyme Microb. Technol. 8, 194–204. doi: 10.1016/0141-0229(86)90087-6
Christie, W. W. (1993). Preparation of ester derivatives of fatty acids for chromatographic analysis. Adv. Lipid Methodol. 2, 69–111.
Cooney, M., Young, G., and Nagle, N. (2009). Extraction of bio-oils from microalgae. Sep. Purif. Rev. 38, 291–325. doi: 10.1080/15422110903327919
De Nobel, J. G., Klis, F. M., Priem, J., Munnik, T., and Van Den Ende, H. (1990). The glucanase-soluble mannoproteins limit cell wall porosity in Saccharomyces cerevisiae. Yeast 6, 491–499. doi: 10.1002/yea.320060606
Doering, T. L. (2009). How sweet it is! Cell wall biogenesis and polysaccharide capsule formation in Cryptococcus neoformans. Annu. Rev. Microbiol. 63, 223–247. doi: 10.1146/annurev.micro.62.081307.162753
Dong, T., Knoshaug, E. P., Pienkos, P. T., and Laurens, L. M. L. (2016). Lipid recovery from wet oleaginous microbial biomass for biofuel production: a critical review. Appl. Energy 177, 879–895. doi: 10.1016/j.apenergy.2016.06.002
Duarte-Oliveira, C., Rodrigues, F., Gonçalves, S. M., Goldman, G. H., Arvalho, A., and Cunha, C. (2017). The cell biology of the trichosporon-host interaction. Front. Cell. Infect. Microbiol. 7:118. doi: 10.3389/fcimb.2017.00118
Ehimen, E. A., Sun, Z. F., and Carrington, C. G. (2010). Variables affecting the in situ transesterification of microalgae lipids. Fuel 89, 677–684. doi: 10.1016/j.fuel.2009.10.011
Fei, Q., O’Brien, M., Nelson, R., Chen, X., Lowell, A., and Dowe, N. (2016). Enhanced lipid production by Rhodosporidium toruloides using different fed-batch feeding strategies with lignocellulosic hydrolysate as the sole carbon source. Biotechnol. Biofuels 9, 1–12. doi: 10.1186/s13068-016-0542-x
Folch, J., Lees, M., and Sloane Stanley, G. H. (1957). A simple method for the isolation and purification of total lipides from animal tissues. J. Biol. Chem. 226, 497–509.
Free, S. J. (2013). Fungal cell wall organization and biosynthesis. Adv. Genet. 81, 33–82. doi: 10.1016/B978-0-12-407677-8.00002-6
Galbraith, L., and Wilkinson, S. G. (1991). Polar lipids and fatty acids of Pseudomonas caryophylli, Pseudomonas gladioli and Pseudomonas pickettii. J. Gen. Microbiol. 137, 197–202. doi: 10.1099/00221287-137-1-197
Gorte, O., Aliyu, H., Neumann, A., and Ochsenreither, K. (2019). Draft genome sequence of the oleaginous yeast Apiotrichum Porosum (syn. Trichosporon porosum) DSM 27194. J. Genomics 7, 11–13. doi: 10.7150/jgen.32210
Grima, E. M., Medina, A. R., Gimnez, A. G., Perez, J. A. S., Camacho, F. G., and Sanchez, J. L. G. (1994). Comparison between extraction of lipids and fatty acids from microalgal biomass. J. Am. Oil Chem. Soc. 71, 955–957.
Guauque Torres, M. P., Foresti, M. L., and Ferreira, M. L. (2014). CLEAs of Candida antarctica lipase B (CALB) with a bovine serum albumin (BSA) cofeeder core: study of their catalytic activity. Biochemi. Eng. J. 90, 36–43. doi: 10.1016/j.bej.2014.05.004
Halim, R., Webley, P. A., and Martin, G. J. O. (2016). The CIDES process: fractionation of concentrated microalgal paste for co-production of biofuel, nutraceuticals, and high-grade protein feed. Algal Res. 19, 299–306. doi: 10.1016/j.algal.2015.09.018
Jacob, Z. (1992). Yeast lipids: extraction, quality analysis, and acceptability. Crit. Rev. Biotechnol. 12, 463–491. doi: 10.3109/07388559209114236
Ji, X. J., Ren, L. J., Nie, Z. K., Huang, H., and Ouyang, P. K. (2014). Fungal arachidonic acid-rich oil: research, development and industrialization. Crit. Rev. Biotechnol. 34, 197–214. doi: 10.3109/07388551.2013.778229
Jin, G., Yang, F., Hu, C., Shen, H., and Zhao, Z. K. (2012). Enzyme-assisted extraction of lipids directly from the culture of the oleaginous yeast Rhodosporidium toruloides. Bioresour. Technol. 111, 378–382. doi: 10.1016/j.biortech.2012.01.152
Joshi, D. R., and Adhikari, N. (2019). An overview on common organic solvents and their toxicity. J. Pharm. Res. Int. 28, 1–18. doi: 10.9734/jpri/2019/v28i330203
Kim, J. H., Kim, Y., Kim, Y. J., and Park, Y. (2016). Conjugated linoleic acid: potential health benefits as a functional food ingredient. Annu. Rev. Food Sci. Technol. 7, 221–244. doi: 10.1146/annurev-food-041715-033028
Kot, A. M., Błażejak, S., Kurcz, A., Bryś, J., Gientka, I., Bzducha-Wróbel, A., et al. (2017). Effect of initial pH of medium with potato wastewater and glycerol on protein, lipid and carotenoid biosynthesis by Rhodotorula glutinis. Electron. J. Biotechnol. 27, 25–31. doi: 10.1016/j.ejbt.2017.01.007
Kuhn, K. R., and Cunha, R. L. (2012). Flaxseed oil - Whey protein isolate emulsions: Effect of high pressure homogenization. J. Food Eng. 111, 449–457. doi: 10.1016/j.jfoodeng.2012.01.016
Leong, W. H., Lim, J. W., Lam, M. K., Uemura, Y., and Ho, Y. C. (2018). Third generation biofuels: a nutritional perspective in enhancing microbial lipid production. Ren. Sustainab. Energy Rev. 91, 950–961. doi: 10.1016/j.rser.2018.04.066
Li, Q., Du, W., and Liu, D. (2008). Perspectives of microbial oils for biodiesel production. Appl. Microbiol. Biotechnol. 80, 749–756. doi: 10.1007/s00253-008-1625-9
Ling, J., Nip, S., and Shim, H. (2013). Enhancement of lipid productivity of Rhodosporidium toruloides in distillery wastewater by increasing cell density. Bioresour. Technol. 146, 301–309. doi: 10.1016/j.biortech.2013.07.023
Liu, K. S. (1994). Preparation of fatty acid methyl esters for gas-chromatographic analysis of lipids in biological materials. J. Am. Oil Chem. Soc. 71, 1179–1187. doi: 10.1007/BF02540534
Liu, Y., Wang, Y., Liu, H., and Zhang, J. (2015). Enhanced lipid production with undetoxified corncob hydrolysate by Rhodotorula glutinis using a high cell density culture strategy. Bioresour. Technol. 180, 32–39. doi: 10.1016/j.biortech.2014.12.093
Mayberry, W. R. (1981). Dihydroxy and monohydroxy fatty acids in advances in Legionella pneumophila. J. Bacteriol. 147, 373–381. doi: 10.1128/jb.147.2.373-381.1981
Mendes, A., Reis, A., Vasconcelos, R., Guerra, P., and Lopes Da Silva, T. (2009). Crypthecodinium cohnii with emphasis on DHA production: a review. J. Appl. Phycol. 21, 199–214. doi: 10.1007/s10811-008-9351-3
Mettler, E., and Seibel, W. (1993). Effects of emulsifiers and hydrocolloids on whole wheat bread quality: a response surface methodology study. Cereal Chem. 70, 373–377.
Meullemiestre, A., Breil, C., Abert-Vian, M., and Chemat, F. (2016). Microwave, ultrasound, thermal treatments, and bead milling as intensification techniques for extraction of lipids from oleaginous Yarrowia lipolytica yeast for a biojetfuel application. Bioresour. Technol. 211, 190–199. doi: 10.1016/j.biortech.2016.03.040
Middelberg, A. P. J. (1995). Process-scale disruption of microorganisms. Biotechnol. Adv. 13, 491–551. doi: 10.1016/0734-9750(95)02007-P
Nalder, T. D., Marshall, S., Pfeffer, F. M., and Barrow, C. J. (2014). Characterisation of lipase fatty acid selectivity using novel omega-3 pNP-acyl esters. J. Funct. Foods 6, 259–269. doi: 10.1016/j.jff.2013.10.015
Ochsenreither, K., Glück, C., Stressler, T., Fischer, L., and Syldatk, C. (2016). Production strategies and applications of microbial single cell oils. Front. Microbiol. 7:1539. doi: 10.3389/fmicb.2016.01539
Porretta, S., Birzi, A., Ghizzoni, C., and Vicini, E. (1995). Effects of ultra-high hydrostatic pressure treatments on the quality of tomato juice. Food Chem. 52, 35–41. doi: 10.1016/0308-8146(94)P4178-I
Probst, K. V., Schulte, L. R., Durrett, T. P., Rezac, M. E., and Vadlani, P. V. (2016). Oleaginous yeast: a value-added platform for renewable oils. Crit. Rev. Biotechnol. 36, 942–955. doi: 10.3109/07388551.2015.1064855
Qian, X., Gorte, O., Chen, L., Zhang, W., Dong, W., Ma, J., et al. (2019). Co-production of single cell oil and gluconic acid using oleaginous Cryptococcus podzolicus DSM 27192. Biotechnol. Biofuels 12:127. doi: 10.1186/s13068-019-1469-9
Qian, X., Gorte, O., Chen, L., Zhang, W., Dong, W., Ma, J., et al. (2020). Continuous self-provided fermentation for microbial lipids production from acetate by using oleaginous yeasts Cryptococcus podzolicus and Trichosporon porosum. Ren. Energy 146, 737–743. doi: 10.1016/j.renene.2019.07.013
Rakesh, S., Dhar, D. W., Prasanna, R., Saxena, A. K., Saha, S., Shukla, M., et al. (2015). Cell disruption methods for improving lipid extraction efficiency in unicellular microalgae. Eng. Life Sci. 15, 443–447. doi: 10.1002/elsc.201400222
Ramola, B., Kumar, V., Nanda, M., Mishra, Y., Tyagi, T., Gupta, A., et al. (2019). Evaluation, comparison of different solvent extraction, cell disruption methods and hydrothermal liquefaction of Oedogonium macroalgae for biofuel production. Biotechnol. Rep. 22:e00340. doi: 10.1016/j.btre.2019.e00340
Ratledge, C. (2004). Fatty acid biosynthesis in microorganisms being used for Single Cell Oil production. Biochimie 86, 807–815. doi: 10.1016/j.biochi.2004.09.017
Ratledge, C., and Cohen, Z. (2008). Microbial and algal oils: do they have a future for biodiesel or as commodity oils? Lipid Technol. 20, 155–160. doi: 10.1002/lite.200800044
Rodríguez-Alcalá, L. M., Harte, F., and Fontecha, J. (2009). Fatty acid profile and CLA isomers content of cow, ewe and goat milks processed by high pressure homogenization. Innov. Food Sci. Emerg. Technol. 10, 32–36. doi: 10.1016/j.ifset.2008.10.003
Rogalska, E., Cudrey, C., Ferrato, F., and Verger, R. (1993). Stereoselective hydrolysis of triglycerides by animal and microbial lipases. Chirality 5, 24–30. doi: 10.1002/chir.530050106
Sathish, A., Smith, B. R., and Sims, R. C. (2014). Effect of moisture on in situ transesterification of microalgae for biodiesel production. J. Chem. Technol. Biotechnol. 89, 137–142. doi: 10.1002/jctb.4125
Schmid, P., Hunter, E., and Calvert, J. (1973). Extraction and purification of lipids. III. Serious limitations of chloroform and chloroform-methanol in lipid investigations. Physiol. Chem. Phys. Med. NMR 5, 151–155.
Schulze, I., Hansen, S., Großhans, S., Rudszuck, T., Ochsenreither, K., Syldatk, C., et al. (2014). Characterization of newly isolated oleaginous yeasts - Cryptococcus podzolicus, Trichosporon porosum and Pichia segobiensis. AMB Express 4:24. doi: 10.1186/s13568-014-0024-0
Silve, A., Papachristou, I., Wüstner, R., Sträßner, R., Schirmer, M., Leber, K., et al. (2018). Extraction of lipids from wet microalga Auxenochlorella protothecoides using pulsed electric field treatment and ethanol-hexane blends. Algal Res. 29, 212–222. doi: 10.1016/j.algal.2017.11.016
Smedman, A., and Vessby, B. (2001). Conjugated linoleic acid supplementation in humans—metabolic effects. Lipids 36, 773–781. doi: 10.1007/s11745-001-0784-7
Soccol, C. R., Dalmas Neto, C. J., Soccol, V. T., Sydney, E. B., da Costa, E. S. F., Medeiros, A. B. P., et al. (2017). Pilot scale biodiesel production from microbial oil of Rhodosporidium toruloides DEBB 5533 using sugarcane juice: performance in diesel engine and preliminary economic study. Bioresour. Technol. 223, 259–268. doi: 10.1016/j.biortech.2016.10.055
Thiru, M., Sankh, S., and Rangaswamy, V. (2011). Process for biodiesel production from Cryptococcus curvatus. Bioresour. Technol. 102, 10436–10440. doi: 10.1016/j.biortech.2011.08.102
Touhami, A., Nysten, B., and Dufrêne, Y. F. (2003). Nanoscale mapping of the elasticity of microbial cells by atomic force microscopy. Langmuir 19, 4539–4543. doi: 10.1021/la034136x
Van Der Klei, I., Veenhuis, M., Brul, S., Klis, F. M., De Groot, P. W. J., Müller, W. H., et al. (2011). Cytology, cell walls and septa: a summary of yeast cell biology from a phylogenetic perspective. Yeasts 1, 111–128. doi: 10.1016/B978-0-444-52149-1.00008-2
Vasconcelos, B., Teixeira, J. C., Dragone, G., and Teixeira, J. A. (2018). Optimization of lipid extraction from the oleaginous yeasts Rhodotorula glutinis and Lipomyces kononenkoae. AMB Express 8:126. doi: 10.1186/s13568-018-0658-4
Vasconcelos, B., Teixeira, J. C., Dragone, G., and Teixeira, J. A. (2019). Oleaginous yeasts for sustainable lipid production — from biodiesel to surf boards, a wide range of “green” applications. Appl. Microbiol. Biotechnol. 103, 3651–3667. doi: 10.1007/s00253-019-09742-x
Villeneuve, P., Pina, M., Montet, D., and Graille, J. (1995). Determination of lipase specificities through the use of chiral triglycerides and their racemics. Chem. Phys. Lipids 76, 109–113. doi: 10.1016/0009-3084(95)02421-E
Watanabe, Y., Sato, S., Asada, M., Arishima, T., Iida, Y., Imagi, J., et al. (2015). Enzymatic analysis of positional fatty acid distributions in triacylglycerols by 1(3)-selective transesterification with Candida antarctica lipase β: a collaborative study. J. Oleo Sci. 64, 1193–1205. doi: 10.5650/jos.ess15182
Whigham, L. D., Cook, M. E., and Atkinson, R. L. (2000). Conjugated linoleic acid: implications for human health. Pharmacol. Res. 42, 503–510. doi: 10.1006/phrs.2000.0735
Xue, F., Gao, B., Zhu, Y., Zhang, X., Feng, W., and Tan, T. (2010). Pilot-scale production of microbial lipid using starch wastewater as raw material. Bioresour. Technol. 101, 6092–6095. doi: 10.1016/j.biortech.2010.01.124
Yamasaki, M., Kishihara, K., Ikeda, I., Sugano, M., and Yamada, K. (1999). Recommended esterification method for gas chromatographic measurement of conjugated linoleic acid. J. Am. Oil Chem. Soc. 76, 933–938. doi: 10.1007/s11746-999-0109-0
Yousuf, A., Khan, M. R., Islam, M. A., Wahid, Z. A., and Pirozzi, D. (2017). Technical difficulties and solutions of direct transesterification process of microbial oil for biodiesel synthesis. Biotechnol. Lett. 39, 13–23. doi: 10.1007/s10529-016-2217-x
Yousuf, A., Sannino, F., Addorisio, V., and Pirozzi, D. (2010). Microbial conversion of olive oil mill wastewaters into lipids suitable for biodiesel production. J. Agric. Food Chem. 58, 8630–8635. doi: 10.1021/jf101282t
Yu, X., Dong, T., Zheng, Y., Miao, C., and Chen, S. (2015). Investigations on cell disruption of oleaginous microorganisms: hydrochloric acid digestion is an effective method for lipid extraction. Eur. J. Lipid Sci. Technol. 117, 730–737. doi: 10.1002/ejlt.201400195
Keywords: single cell oil, oleaginous yeasts, downstream processing, cell disruption, lipid extraction, transesterification, Saitozyma podzolica DSM 27192, Apiotrichum porosum DSM 27194
Citation: Gorte O, Hollenbach R, Papachristou I, Steinweg C, Silve A, Frey W, Syldatk C and Ochsenreither K (2020) Evaluation of Downstream Processing, Extraction, and Quantification Strategies for Single Cell Oil Produced by the Oleaginous Yeasts Saitozyma podzolica DSM 27192 and Apiotrichum porosum DSM 27194. Front. Bioeng. Biotechnol. 8:355. doi: 10.3389/fbioe.2020.00355
Received: 12 February 2020; Accepted: 31 March 2020;
Published: 24 April 2020.
Edited by:
Eduardo Jacob-Lopes, Universidade Federal de Santa Maria, BrazilReviewed by:
Bruce S. Dien, Agricultural Research Service, United States Department of Agriculture, United StatesCopyright © 2020 Gorte, Hollenbach, Papachristou, Steinweg, Silve, Frey, Syldatk and Ochsenreither. This is an open-access article distributed under the terms of the Creative Commons Attribution License (CC BY). The use, distribution or reproduction in other forums is permitted, provided the original author(s) and the copyright owner(s) are credited and that the original publication in this journal is cited, in accordance with accepted academic practice. No use, distribution or reproduction is permitted which does not comply with these terms.
*Correspondence: Katrin Ochsenreither, a2F0cmluLm9jaHNlbnJlaXRoZXJAa2l0LmVkdQ==
†These authors have contributed equally to this work and share first authorship
Disclaimer: All claims expressed in this article are solely those of the authors and do not necessarily represent those of their affiliated organizations, or those of the publisher, the editors and the reviewers. Any product that may be evaluated in this article or claim that may be made by its manufacturer is not guaranteed or endorsed by the publisher.
Research integrity at Frontiers
Learn more about the work of our research integrity team to safeguard the quality of each article we publish.