- 1Andalusian Center for Molecular Biology and Regenerative Medicine (CABIMER), Pablo de Olavide University, University of Seville, CSIC, Seville, Spain
- 2Biomedical Research Network on Diabetes and Related Metabolic Diseases (CIBERDEM), Institute of Health Carlos III, Madrid, Spain
- 3School of Medicine, Miguel Hernández University, Alicante, Spain
- 4Pablo de Olavide University, Seville, Spain
Mesenchymal stem cells (MSCs) are among the most frequently used cell type for regenerative medicine. A large number of studies have shown the beneficial effects of MSC-based therapies to treat different pathologies, including neurological disorders, cardiac ischemia, diabetes, and bone and cartilage diseases. However, the therapeutic potential of MSCs in cancer is still controversial. While some studies indicate that MSCs may contribute to cancer pathogenesis, emerging data reported the suppressive effects of MSCs on cancer cells. Because of this reality, a sustained effort to understand when MSCs promote or suppress tumor development is needed before planning a MSC-based therapy for cancer. Herein, we provide an overview on the therapeutic application of MSCs for regenerative medicine and the processes that orchestrates tissue repair, with a special emphasis placed on cancer, including central nervous system tumors. Furthermore, we will discuss the current evidence regarding the double-edged sword of MSCs in oncological treatment and the latest advances in MSC-based anti-cancer agent delivery systems.
Introduction
Mesenchymal stem cells (MSCs), also referred to as mesenchymal stromal cells, are adult stem cells capable of self-renewal and multilineage differentiation (Jiang et al., 2002). They were originally found in the bone marrow (Friedenstein et al., 1970), but they were later identified in other tissues including adipose tissue, muscle, peripheral blood, hair follicles, teeth, placenta and umbilical cord (da Silva Meirelles et al., 2006). Although MSCs may exhibit different characteristics depending on their tissue of origin, they must meet the three minimal criteria defined by the International Society for Cellular Therapy (ISCT) (Dominici et al., 2006). First, MSCs must show plastic-adherence when grown in vitro. Second, MSCs must express the surface antigens CD73, CD90, and CD105 while lacking expression of CD45, CD34, CD14 or CD11b, CD79α or CD19 and HLA-DR. Third, MSCs must differentiate into mesodermal cell types (i.e., adipocytes, chondrocytes, and osteoblasts) when cultured under specific conditions. In addition to mesodermal linage, MSCs are capable of differentiating into cells of non-mesodermal origin (i.e., ectodermal and endodermal lineages), such as neuronal cells, cardiomyocytes, hepatocytes or epithelial cells (Lee et al., 2004; Paunescu et al., 2007; Quevedo et al., 2009; Gervois et al., 2015). This plasticity of MSCs confers benefits in tissue regeneration.
Mesenchymal stem cells have become as the top used stem cell type for clinical application due to numerous advantages (Connick et al., 2012; Gotherstrom et al., 2014; Karantalis et al., 2014; Rushkevich et al., 2015; Thakkar et al., 2015; Vega et al., 2015; Fernandez et al., 2018) (Figure 1). In addition to different source and multilineage differentiation potential, MSCs also possess the capacity to migrate to injured sites in response to environmental signals and promote tissue regeneration mediated by the release of paracrine factors with pleiotropic effects. Through interaction with the host niche, MSCs are able to inhibit the immune system, promote cell survival, or induce angiogenesis among others pleiotropic activities (Salgado et al., 2010). Of these, the immunosuppressive role of MSCs is particularly interesting for clinical use since it confers resistance to rejection by the host immune system after transplantation. Furthermore, MSCs can be obtained from easily accessible sources by minimally invasive methods (e.g., peripheral blood, adipose tissue) and can be rapidly expanded in large-scale for clinical use (Escacena et al., 2015). This allows to produce a patient-specific medicinal product (i.e., autologous medicinal product) within a therapeutic time window. In addition, the possibility of obtaining MSCs from adult tissue circumvent the ethical issues associated with the use of embryonic source (Lo and Parham, 2009; Ramos-Zuriga et al., 2012). All these advantages of MSCs make this cell type a powerful tool for clinical application in regenerative medicine.
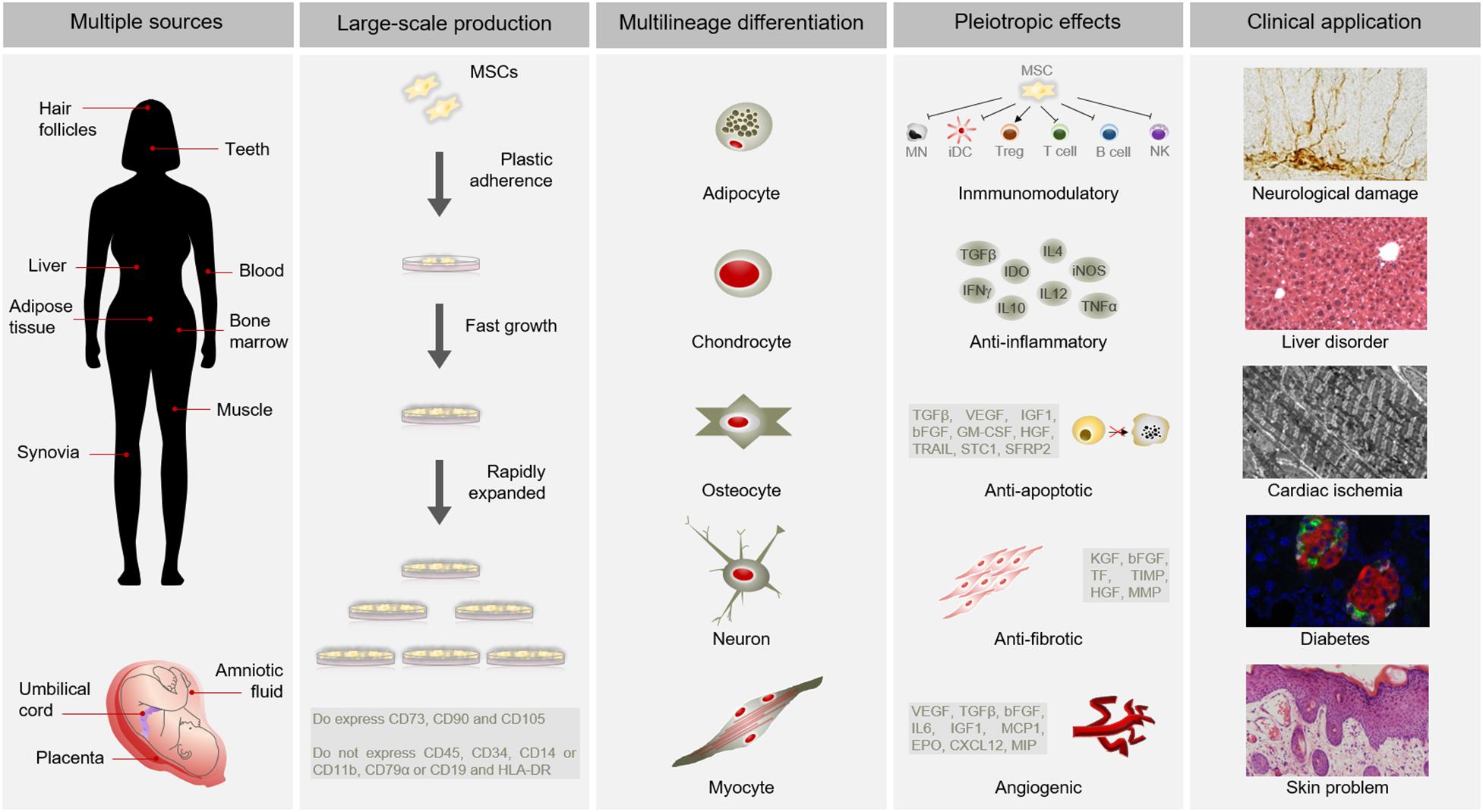
Figure 1. Advantages of MSCs for clinical use. MSCs possess multiple advantages for clinical application. Among other benefits, MSCs can be isolated from several sources, are large-scale produced, differentiate into a variety of cell types and have pleiotropic effects. All these advantages make MSCs suitable for clinical application in different pathological conditions, such as neurological damages, liver disorders, cardiac ischemia, diabetes or skin problems. Abbreviations: HLA-DR, major histocompatibility complex class II DR; MN, monocyte; iDC, immature dendritic Cell; Treg, Regulatory T cell; NK, natural killer cell; TGFβ, transforming growth factor; INFγ, interferon γ; IDO, indoleamine 2,3-dioxygenase; IL10, interleukin 10; IL4, interleukin 4; IL12, interleukin 12; iNOS, inducible nitric oxide synthase; TNFα, tumor necrosis factor α; VEGF, vascular endothelial growth factor; IGF1, insulin like growth factor 1; bFGF, basic fibroblast growth factor; GM-CSF, granulocyte macrophage colony-stimulating factor; HGF, hepatocyte growth factor; TRAIL, TNF-related apoptosis-inducing ligand; STC1, stanniocalcin 1; SFRP2, secreted frizzled related protein 2; KGF, keratinocyte growth factor; TF, tissue factor; TIMP, tissue inhibitor of metalloproteinases; MMP, matrix metalloproteinases; IL6, interleukin 6; MCP1, monocyte chemoatractant protein 1; EPO, erythropoietin; CXCL12, C-X-C motif chemokine 12; MIP, macrophage inflammatory protein.
Although MSCs have shown tremendous therapeutic potential in various diseases, their application for cancer treatment remains controversial. While some studies indicate that MSCs may contribute to cancer pathogenesis, emerging data support the beneficial effects of MSCs for oncological treatment. In this review, we provide an overview on the therapeutic application of MSCs for regenerative medicine and discuss the double-edged sword of MSCs for cancer.
Therapeutic Potential of MSCs
Over the past decades, a large number of studies have emerged using MSC-based therapies in preclinical studies to treat many different pathologies, including neurological disorders, cardiac ischemia, diabetes and bone and cartilage diseases (Si et al., 2012; van Velthoven et al., 2013; Jones et al., 2015; Liu et al., 2016; Ozeki et al., 2016; Capilla-Gonzalez et al., 2018; Chau et al., 2018; Rehorova et al., 2019; Soria et al., 2019). The therapeutic potential of MSCs is firstly mediated by their inherent ability to migrate toward damaged tissues. Then, engrafted cells secrete bioactive mediators, such as growth factors, cytokines and extracellular vesicles that exert immunosuppressive, anti-apoptotic, anti-fibrotic, angiogenic, and anti-inflammatory effects (Salgado et al., 2010). For instance, a study using a neonatal stroke rat model showed that intranasal delivery of bone marrow MSCs reduces infarct size, gray-white matter loss, and motor deficits (van Velthoven et al., 2013). These beneficial effects were in part explained by an increased cell proliferation in the ischemic hemisphere of transplanted rats. In a mouse model of Friedreich’s ataxia, intrathecal injections of bone marrow MSCs improved motor function and delayed neurodegeneration by releasing the neurotrophic factors Neurotrophin-3, Neurotrophin-4, and brain-derived neurotrophic factor, which are implicated in neuronal survival (Jones et al., 2015). Human MSCs derived from umbilical cord showed benefits by improving ventricular function in a porcine model of myocardial ischemia (Liu et al., 2016). In this study, the authors described that MSC-treated pigs exhibit increased angiogenesis, reduced apoptosis and decreased fibrosis in the ischemic heart. Bone marrow-derived MSCs have also shown benefits in improving insulin sensitivity associated with an increased GLUT4 expression in type 2 diabetic rats (Si et al., 2012). More recently, the intranasal application of human adipose-derived MSCs was found to prevent neurocognitive impairments induced by cranial radiation in mice (Soria et al., 2019). The neuroprotective role of intranasally delivered MSCs was mediated by limiting pro-inflammatory processes, restricting oxidative damage accumulation, and reducing neuronal loss after radiation. Another study reported beneficial effects of umbilical cord-derived MSC extracts for atopic dermatitis in a murine model by reducing the T cell response (Song et al., 2019). These reports uncover two main properties of MSCs that determine their therapeutic potential; the capacity to migrate toward the lesion site and the ability to repair damaged tissues.
Migration Toward Damaged Tissues
The success of an advanced therapy medicinal product initially depends on its ability to reach target tissues. MSCs possess inherent tropism toward damaged sites that is controlled by a large number of factors and mechanisms, including chemoattractant signals. For instance, the C-X-C motif chemokine ligand 12 (CXCL12) is a frequent triggering factor at the site of injury. It has been demonstrated that a subpopulation of MSCs expresses the C-X-C chemokine receptor type 4 (CXCR4) that binds to its ligand, the CXCL12, to mediate cell migration (Wynn et al., 2004; Ma et al., 2015). Aside from CXCR4, MSCs express other chemokine receptors, such as CCR1, CCR2, CCR4, CCR7, CCR8, CCR9, CCR10, CXCR1, CXCR2, CXCR3, CXCR4, CXCR5, CXCR6, and CX3CR1 (Sordi et al., 2005; Von Luttichau et al., 2005; Honczarenko et al., 2006; Ringe et al., 2007). These receptors are essential to respond to triggering factors at the site of injury. In addition, MSCs also express cell adhesion molecules, including CD49d, CD44, CD54, CD102, and CD106 (De Ugarte et al., 2003). These chemokines and cell adhesion molecules orchestrate the mobilization of MSCs to sites of injury, in a similar manner to white blood cells do (Kolaczkowska and Kubes, 2013). MSC mobilization is a multistep process that encompasses the attachment of free circulating MSCs in the blood stream to transmigrate between endothelial cells with the ultimate goal of migrate and engraft to the target tissue (Figure 2).
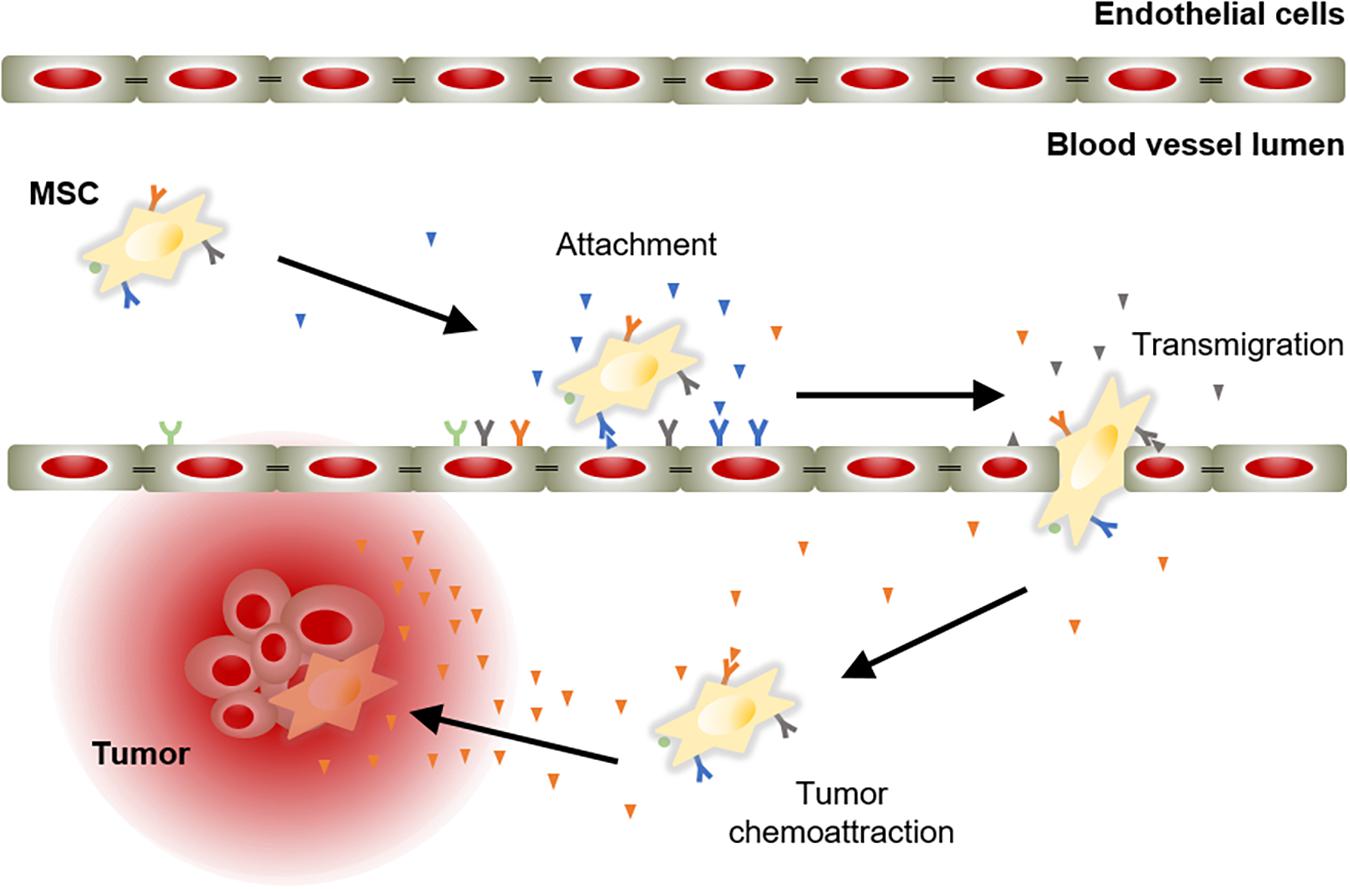
Figure 2. Model of chemoattractant-induced MSC migration toward tumor lesion. Mobilization of MSCs initiates with their incorporation into the circulation. Then, MSCs migrate via the blood stream to areas of injury in response to chemoattractant cues. Ligand-receptor bindings allow MSCs to attach to endothelial cells lining the blood vessels. Subsequently, MSCs activate and initiate the process to cross the endothelium to move toward the target tissue, guided by a chemotactic gradient.
Tissue Repair Ability
Once recruited in the injured site, MSCs contribute to tissue repair and regeneration through activation of several mechanisms. A growing body of research has demonstrated that MSCs display pleiotropic effects, which give them an enormous therapeutic potential (Figure 1). In response to injury signals, MSCs secrete a variety of mediators of tissue repair, including anti-apoptotic, anti-inflammatory, immunomodulatory, anti-fibrotic and angiogenic agents (Caplan and Dennis, 2006; Meirelles Lda et al., 2009; Maltman et al., 2011; Escacena et al., 2015). Among pleiotropic effects, anti-inflammatory and immunomodulatory properties are mainly responsible for the therapeutic benefits of MSCs. As sensors of inflammation, MSCs release soluble factors, such as transforming growth factor β (TGFβ), Indoleamine 2,3-dioxygenase (IDO), Tumor Necrosis Factor α (TNFα), Interleukin 10, and Interferon gamma (INFγ), which interfere with the immune system and modify the inflammatory landscape (Prockop and Oh, 2012). Pivotal studies showed that MSCs inhibit the proliferation of T and B cells (Di Nicola et al., 2002; Corcione et al., 2006; Song et al., 2019), suppress the activation of natural killer cells (Sotiropoulou et al., 2006), and prevent generation and maturation of monocyte-derived dendritic cells (English et al., 2008; Spaggiari et al., 2009). Furthermore, MSCs are able to promote the generation of regulatory T cells (Maccario et al., 2005), which exert immunosuppressive effects. Although soluble factors play a key role in the immunosuppressive activity of MSCs, cell-to-cell contact also influences immune responses (Ren et al., 2010; Li Y. et al., 2019). For instance, direct contact between MSCs and proinflammatory macrophages has been shown to induce immune tolerance through induction of tumor necrosis factor-stimulated gene-6 (TSG-6) production (Li Y. et al., 2019). MSC-mediated modulations of the immune response set in motion essential inflammatory processes that significantly promote tissue repair and regeneration by driving healing, scarring and fibrosis (Julier et al., 2017).
Another typical property of MSCs that is involved in their therapeutic effects is the multilineage differentiation potential. In addition to mesodermal linage, MSCs can differentiate into cells of ectodermal and endodermal origin, such as neuronal cells, cardiomyocytes, hepatocytes or epithelial cells (Lee et al., 2004; Paunescu et al., 2007; Quevedo et al., 2009; Gervois et al., 2015). This ability to differentiate into cell types of non-mesodermal origin has been questioned by researchers claiming that differentiated cells from MSCs are able to dedifferentiate and transdifferentiate into cells of another developmental lineage (Song and Tuan, 2004). Notwithstanding, the versatile differentiation potential of MSCs allows the replacement of damaged or dead cells from different tissues. However, several studies indicate that, after administration, MSCs transiently engraft at the injury site for a short period of time and then disappear. The latter suggest that MSCs must activate mechanisms in the host niche which contribute to tissue repair. For instance, the cross-talk between MSCs and the damaged tissue microenvironment results in the secretion of specific agents involved in proliferation and differentiation of local precursor cells. In this context, a study suggested that systemic administration of MSCs improves radiation-induced intestinal epithelium injury in mice, by increasing the activation of the Wnt/β-catenin signaling pathway that drives proliferation and maintenance of intestinal stem cells (Gong et al., 2016). In a mouse models of Alzheimer’s disease, intravenous administration of MSCs stimulated proliferation and differentiation of hippocampal neuronal progenitor cells into mature neurons by increasing the Wnt signaling pathway (Oh et al., 2015).
Divergent Roles of MSCs in Cancer Treatment
The therapeutic benefits of MSCs have prompt their use in cell-based strategies to treat different diseases, including cancer. Similar to damaged tissues, tumors exert chemoattractant effects on MSCs that influence their recruitment to tumor sites (Figure 2). The CXCL12/CXCR4 axis is one of the most frequently studied signaling pathways in the mobilization of MSCs to tumor microenvironment (Gao et al., 2009; Xu et al., 2009; Lourenco et al., 2015; Wobus et al., 2015; Kalimuthu et al., 2017). However, the ability of MSCs to migrate toward cancerous tissue is also controlled by other agents, including diffusible cytokines such as IL8, growth factors such as TGFβ1 or platelet derived growth factor (PDGF), and extracellular matrix molecules such as matrix metalloproteinase 2 (MMP-2) (Nakamizo et al., 2005; Birnbaum et al., 2007; Bhoopathi et al., 2011). Once the tumor niche is reached, MSCs interact with cancer cells via direct and indirect mechanisms that affect tumor development (Figure 3). The paracrine function of MSCs is one of the main mechanisms involved in cancer regulation and is mediated by multiple factors, including growth factors and cytokines. These paracrine factors affect cellular processes involving tumor cell cycle (i.e., cell proliferation), cell survival, angiogenesis, and immunosuppression/immunomodulation, allowing MSCs to regulate cancer. The paracrine agents can be directly secreted into the extracellular space or packaged into extracellular vesicles to be spread in the tumor milieus (Rani et al., 2015). The interaction of MSCs with tumor cell cycle is the most commonly accepted process by which MSCs exert their therapeutic effects (Fathi et al., 2019). By inhibiting proliferation-related signaling pathways, such as the phosphatidylinositol 3-kinase/protein kinase B (PI3K/AKT), MSCs can induce cell cycle arrest and reduce cancer growth (Lu et al., 2019). In addition, MSCs can undergo differentiation into other cell types, such as cancer-associated fibroblasts (CAFs), to directly contribute to cancer progression (Jotzu et al., 2011; Barcellos-de-Souza et al., 2016; Aoto et al., 2018) (Figure 3).
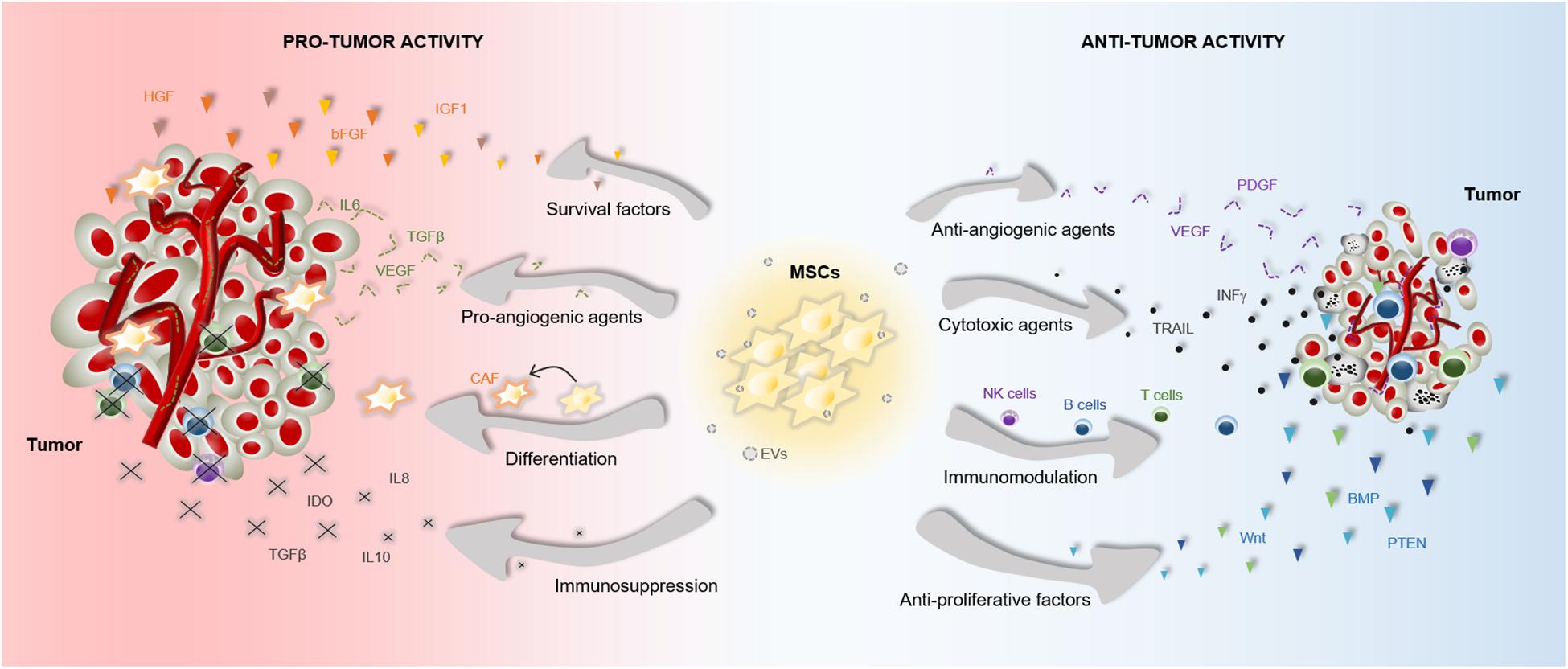
Figure 3. Pro- and anti-tumor effects of MSCs. The particular properties that make MSCs excellent therapeutic agents, can also influence tumor progression. MSCs are able to release multiple agents with pro- and anti-tumor effects, which affect survival, proliferation and angiogenesis among other cell functions. These paracrine agents can be directly secreted into the tumor milieus or secreted via EVs. Furthermore, MSCs can differentiate into CAFs to support tumor progression. Abbreviations: bFGF, basic fibroblast growth factor; BMP, bone morphogenetic protein; CAF, cancer-associated fibroblasts; HGF, hepatocyte growth factor; EVs, extracellular vesicles; IGF1, insulin like growth factor 1; IL6, interleukin 6; IL8, interleukin 8; IL10, interleukin 10; INFγ, interferon gamma; IDO, indoleamine 2,3-dioxygenase; NK, natural killer; PTEN, phosphatidylinositol 3,4,5-trisphosphate 3-phosphatase; PDGF, platelet derived growth factor; TRAIL, TNF-related apoptosis-inducing ligand; TGFβ, transforming growth factor; VEGF, vascular endothelial growth factor.
Accumulating evidences indicate that the cross-talk between MSCs and tumor cells results in both pro-tumor and anti-tumor effects, raising safety concerns for clinical application in oncology (Barkholt et al., 2013) (Figure 3). The discrepancies in the ability of MSCs to promote or suppress tumor development may be attributable to differences in experimental tumor models, MSC tissue source, dose or timing of the MSC treatment, cell delivery method, control group chosen, and other experimental conditions (Bortolotti et al., 2015; Bajetto et al., 2017). In this regard, a study demonstrated that direct (cell-to-cell contact) or indirect (released soluble factors) interaction between umbilical cord MSCs and glioblastoma stem cells produces divergent effects on cell growth, invasion and migration (Bajetto et al., 2017). Additionally, the application of MSCs for cancer patients is a more complex situation in which other factors have to be taken into consideration. For instance, the pathological conditions of each patient may induce cellular and molecular changes in MSCs that interfere with their therapeutic effects (Capilla-Gonzalez et al., 2018; Perez et al., 2018; Rivera et al., 2019). We must, therefore, be cautious in the conclusions we draw from a single study regarding the therapeutic effects of MSCs in cancer.
Pro-tumor Activity
The pleiotropic effects of MSCs that promote tissue repair and regeneration may also confer pro-tumor functions to these cells. For instance, metastatic human breast carcinoma cells were found to induce the secretion of the chemokine (C-C motif) ligand 5 (CCL5) from MSCs, which enhanced tumor invasion (Karnoub et al., 2007). Seminal reports demonstrated that MSCs are also able to inhibit apoptosis in tumor cells by secreting pro-survival factors such as VEGF and basic fibroblast growth factor (bFGF) (Konig et al., 1997; Dias et al., 2002).
Numerous studies converged on the finding that MSCs contribute to cancer pathogenesis by releasing inflammatory factors that promote immunosuppressive effects. For example, an in vitro study showed that MSCs isolated from gastric tumors mediate cancer progression through secretion of Interleukin 8 (IL8) (Li et al., 2015), a pro-inflammatory chemokine that favors the recruitment of leukocytes. It is known that recruited leukocytes, such as macrophages and neutrophils, facilitate cancer initiation and progression (Guo et al., 2017; Powell et al., 2018). Similarly, MSCs are able to secrete TGFβ that promotes macrophages infiltration at the tumor site and facilitates tumor escape from immune surveillance (Kim et al., 2006; Byrne et al., 2008).
Compelling evidences indicate that MSCs can also support tumor angiogenesis, an essential process in cancer progression that supplies tumors with oxygen and nutrients. For instance, MSCs recruited in breast and prostate tumors were found to increase the expression of angiogenic factors, including TGFβ, VEGF and Interleukin 6, which contribute to tumor growth and vascularization (Zhang et al., 2013). Similarly, a correlation between increased expression of TGFβ1 and higher microvessel density was observed in hepatocellular carcinomas of mice receiving intravenous injections of human MSCs (Li et al., 2016). This study further supported that MSCs may enhance tumor angiogenesis via TGFβ.
Furthermore, MSCs can also respond to soluble factors secreted from cancer cells and differentiate into CAFs, a cell type within the tumor microenvironment capable of promoting tumorigenesis (Mishra et al., 2008). In particular, TGFβ secreted from cancer cells plays a key role in the differentiation of MSCs into CAFs (Jotzu et al., 2011; Barcellos-de-Souza et al., 2016; Aoto et al., 2018). It is known that the transition of MSCs into CAFs contributes to tumor progression in part by their active secretome, which includes immune-modulating agents (CXCL12, Granulocyte Macrophage Colony-Stimulating Factor), pro-angiogenic factors (VEGF, TGFβ, PDGF), pro-survival factors (Hepatocyte Growth Factor, Insulin like Growth Factor 1, Interleukin 6), and extracellular matrix modulators (MMP, Tissue Inhibitor of Metalloproteinases) among others (Kalluri, 2016). Cell engulfment has also been identified as an interaction process between MSCs and cancer cells that enhances tumor aggressiveness. A recent report demonstrated that breast cancer cell engulfment of MSCs leads to changes in the transcriptome profile of tumor cells, mainly associated with oncogenic pathways (Chen et al., 2019). This MSC engulfment enhances epithelial-to-mesenchymal transition, stemness, invasion, and metastasis of breast cancer (Chen et al., 2019).
Anti-tumor Activity
Although compelling evidences show a pro-tumorigenic role of MSCs, these cells also have potent tumor suppressive effects that have been exploited as cancer therapeutics. Previous studies have demonstrated that MSCs release cytotoxic agents, such as TNF-Related Apoptosis-Inducing Ligand (TRAIL) that selectively induces apoptosis in different types of cancer (Wiley et al., 1995; Hao et al., 2001; Takeda et al., 2001; Akimoto et al., 2013). Recently, a report indicated that bone marrow MSCs promote apoptosis and suppress growth of glioma U251 cells through downregulation of the PI3K/AKT signaling pathway (Lu et al., 2019). Similarly, intravenously transplanted MSCs were found to suppress tumor growth by blocking AKT activation in a Kaposi sarcoma mouse model (Khakoo et al., 2006). In mammary carcinomas, umbilical cord MSCs attenuated cell growth and triggered apoptosis through inhibiting ERK1/2 and AKT activation (Ganta et al., 2009). The Wnt signaling pathway has also been involved in the ability of MSCs to inhibit tumor cell proliferation (Qiao et al., 2008a, b). A mechanistic study of the inhibitory effect of MSCs on breast cancer cells demonstrated that the protein Dickkopf-1 (Dkk-1) released from MSCs blocks tumor growth via depression of Wnt signaling (Qiao et al., 2008a).
In contrast to investigations describing the pro-angiogenic effect of MSCs (Zhang et al., 2013; Li et al., 2016), the anti-tumor activity of MSCs via inhibition of tumor angiogenesis has also been documented. A study reported that bone marrow MSCs restrict vascular growth in ΔGli36 glioma xenograft through downregulation of the PDGF/PDGFR axis (Ho et al., 2013). In particular, the expression of PDGF-BB protein was significantly reduced in tumor lysates when treated with MSCs, which correlated with reduced levels of activated PDGFR-β and the active isoform of its downstream target AKT (Ho et al., 2013). In a melanoma mouse model, transplanted MSCs inhibited angiogenesis in a concentration-dependent manner, leading to a reduced tumor growth (Otsu et al., 2009). Confirmatory in vitro studies suggested that the anti-angiogenic effect was due to MSC-induced capillary degeneration (Otsu et al., 2009).
Furthermore, MSCs have elicited anti-tumor immune responses through released inflammatory mediators, such as the multifunctional cytokine TGFβ. Similar to several signaling molecules, TGFβ plays a dual role in cancer development (Bierie and Moses, 2006). Besides the aforementioned pro-tumor functions, TGFβ signaling exhibits suppressive effects in cancer (Dong et al., 2007; Guasch et al., 2007). In fact, while the expression of the type III TGFβ receptor (TβRIII) decreases during breast cancer progression, restoring TβRIII expression suppresses tumorigenicity (Dong et al., 2007).
MSCs as Carriers of Anti-Cancer Payloads
Over the past decade, research efforts have focused on investigating the potential of stem cells as Trojan horses to selectively deliver anti-cancer payloads to tumor cells. In this context, MSCs have attracted much attention as therapeutic carriers due to their inherent capacity to migrate to tumor sites. Genetic engineering is one of the most common strategies used to produce MSCs delivering tumor-suppressing agents into cancer cells. Typically, MSCs have been genetically modified with viral particles to express cytokines, such as Interferon β (INFβ) (Studeny et al., 2002; Shen et al., 2016). It has been reported that human umbilical cord MSCs transduced with adenoviral vectors expressing IFNβ effectively inhibit the growth of breast cancer cells through induction of apoptosis (Shen et al., 2016). Interleukins are another group of cytokines used as tumor-suppressing agents (Chen et al., 2008; Liu et al., 2018). A recent study using lentiviral transductions showed that human umbilical cord MSCs expressing interleukin-18 inhibit the proliferation and metastasis of breast cancer in mice (Liu et al., 2018). Genetically engineered MSCs with TRAIL have also shown strong anti-tumor activity in different types of cancer (Ciavarella et al., 2012; Fakiruddin et al., 2014; Guo et al., 2016; Jiang et al., 2016). In a fascinating study, X. Jiang and colleagues developed a non-viral method using nanoparticles to produce human MSCs engineered to express the suicide protein TRAIL for targeting and eradicating intracranial gliomas in mice (Jiang et al., 2016). When transplanted in a mouse model of orthotopic patient-derived glioblastoma xenografts, TRAIL-expressing MSCs inhibited tumor growth, induced apoptosis, reduced the occurrence of microsatellites, and extended animal survival.
Beside cytokines, several other proteins have been used as tumor-suppressing agents in MSC engineering for cancer therapy. For instance, Bone Morphogenetic Protein 4 (BMP4)-expressing MSCs were found to efficiently suppress tumor growth and prolong survival of glioma-bearing mice (Li et al., 2014; Mangraviti et al., 2016). Similarly, MSCs modified to express the tumor-suppressor gene Phosphatidylinositol 3,4,5-Trisphosphate 3-Phosphatase (PTEN) induced cytotoxicity of glioma cells (Guo et al., 2016).
MicroRNAs (miRs) have gained special interest in cancer therapy because of their ability to modulate post-transcriptional gene expression. It is known that MSCs express a variety of miRs that can be packaged into extracellular vesicles, and delivered to neighboring cells to exert therapeutic effects (Collino et al., 2014). Taking advantage of this property, MSCs have been engineered to carry specific miRs with anti-cancer properties (Lee et al., 2013; Lang et al., 2018; Sharif et al., 2018; Li X. et al., 2019). For instance, lentiviral vectors were used to engineer MSCs to produce extracellular vesicles carrying high levels of miR-124a, which had an effective anti-tumor action in multiple patient-derived glioma stem cell lines (Lang et al., 2018).
The loading of MSCs with oncolytic viruses has being used as an effective anti-tumor therapy. MSCs infected with the oncolytic adenovirus ICOVIR5 provided therapeutic benefit for the treatment of lung carcinoma in mice, through inhibition of tumor growth and promotion of T cell recruitment to the tumors (Rincon et al., 2017). Similarly, MSCs carrying the oncolytic adenovirus CRAd5/F11 inhibited tumor progression in a subcutaneous murine xenograft model of colorectal cancer (Guo et al., 2019). Different variants of the oncolytic herpes simplex virus has also been used to arm MSCs that effectively track metastatic tumor lesions and prolong survival of mice with brain metastatic melanomas (Du et al., 2017).
Another cellular Trojan horse that has been used for cancer treatment is MSCs loaded with anti-cancer drugs. For instance, an in vitro study determined that the conditioned media from gingival papilla-derived MSCs primed with Paclitaxel, Doxorubicin or Gemcitabine inhibit squamous carcinoma growth (Cocce et al., 2017). Paclitaxel-loaded MSCs also exhibited anti-tumor effects in glioma-bearing rats (Pacioni et al., 2015). Latest investigations have focused on the development of strategies to improve the payload and delivery capacity of MSCs. In this sense, nanoparticles are a promising approach to increase the anti-tumor efficacy of MSCs loaded with anti-cancer drugs (Layek et al., 2018; Wang et al., 2018; Moku et al., 2019). Drug-encapsulated nanoparticles offer multiple therapeutic benefits by providing preferential accumulation at the target site, preventing burst release and reducing side effects.
Limitations of Msc-Based Therapies for Cancer: a Challenge for Biomaterials
The use of engineered MSCs has emerged as a new therapeutic paradigm to treat cancer. However, efficient engraftment and survival of delivered MSCs remains a potential obstacle that limits their therapeutic application. Biomaterials are used in cell therapy as scaffolds that improve the retention of transplanted cells in specific sites to treat different pathologies. This combined use of biomaterials and stem cells allows from the treatment of restricted defects to the repair and replacement of entire organs (Zeng et al., 2015; Wang et al., 2017; Diomede et al., 2018). In the cancer research area, a recent study described a method for the delivery of therapeutic MSCs on biomaterials to treat postoperative brain cancer (Sheets et al., 2018). This approach bases on the implantation of biodegradable fibrin scaffolds seeded with MSCs into the resection cavity to eradicate residual tumor cells in patients receiving surgical removal, with the ultimate goal of increasing cancer-free survival. Another study used cryogel-housed MSCs that were engineered to release anti-CD33-anti-CD3 bispecific antibody for effective immunotherapy in acute myeloid leukemia (Aliperta et al., 2017). In addition to scaffolds, biomaterials are used to encapsulate cells, protecting them from the host while allowing the diffusion of nutrients and therapeutic agents. Microcapsules designed with alginate, cellulose and agarose have shown benefits in cell-based anti-cancer therapies (Pelegrin et al., 1998; Sakai et al., 2005; Schwenter et al., 2011; Johansson et al., 2013). The group of Simone P. Niclou demonstrated that the interstitial delivery of alginate-encapsulated cells expressing the soluble form of the leucine-rich repeats and immunoglobulin-like domains 1 (Lrig1) inhibited tumor growth in orthotopic patient-derived glioblastoma xenografts mouse model (Johansson et al., 2013).
Biomaterials can be designed to have their own anti-cancer activities. Among biomaterials used in oncology, Gliadel represents one of the major success in the development of interstitial therapies for brain cancer (Brem and Gabikian, 2001). Gliadel is a biodegradable medicinal implant made of polifeprosan that is inserted into the resection cavity and slowly releases the anti-cancer agent carmustine over 2–3 weeks. The use of Gliadel in patients receiving surgical removal of brain tumors is associated with moderated survival benefits (Bregy et al., 2013). Latest investigations have discovered a thermo-responsive biodegradable paste that allows delivering of multiple anti-cancer agents with improved results in glioma patients survival (Smith et al., 2019). Once optimized the composition and design of biomaterials, it may be possible to use them in combination with stem cells to release anti-cancer agents in a cooperative manner. Therefore, the application of biomaterial in MSC-based therapies is a potential approach for the treatment of cancer that merits further investigation.
Clinical Application of MSCs for Cancer Therapy
The last decade has witnessed a rapid development of cell-based therapies for oncological application, being MSCs at the forefront of this new tendency. Aside from their anti-cancer effects, MSCs are of special relevance for personalized cell-based therapies because they can be easily obtained with minimally invasive procedures and rapidly large-scale expanded (Escacena et al., 2015). To date, 25 clinical trials are registered on ClinicalTrials.gov aimed to use MSCs in various cancer conditions. Among these studies, 14 trials are using MSCs as therapeutic agent to directly treat cancer (Table 1). Most of these trials are ongoing phase 1 or 2 studies that are evaluating the safety and efficacy of MSC application in cancer patients. Of special note is a completed phase I/II clinical trial from 2013 that investigated the use of bone marrow-derived autologous MSCs infected with the oncolytic adenovirus ICOVIR5 (CELYVIR) to treat metastatic and refractory solid tumors in children and adults (NCT01844661). This exploratory study evaluated the adverse effects after intravenous infusions of CELYVIR (time frame: 48 h after each infusion) and the clinical outcome (time frame: up to 2 months after the last infusion). The authors concluded that multidoses of CELYVIR have an excellent safety profile and beneficial anti-tumor effects (Garcia-Castro et al., 2010; Melen et al., 2016). Interestingly, they documented a complete remission in one pediatric case 3 years after CELYVIR treatment (Garcia-Castro et al., 2010).
Furthermore, there are nine registered clinical trials that evaluate the application of MSCs to treat a variety of side effects of cancer treatments, such as cardiomyopathy due to anthracyclines (NCT02509156), xerostomia due to radiotherapy (NCT03874572), cisplatin-induced acute renal failure (NCT01275612), erectile dysfunction after prostatectomy (NCT01089387) or radiation-induced hemorrhagic cystitis (NCT02814864). Therefore, MSC-based therapies stand as a good therapeutic option not only to directly target cancer, but also to minimize the side effects of cancer treatments. Consequently, there are two types of participants that would be potential candidates in a clinical trial of MSC-based therapy for cancer; (1) patients with cancer that are receiving or not receiving treatment, and (2) cancer survivors that experience side effects of oncological treatments. Inclusion criteria for these individuals should be either to be diagnosed with cancer, to suffer side effects of cancer treatments or both. Despite advances are being achieved, the lack of published results involving clinical studies hinders the development of further advances in the therapeutic application of MSCs.
Concluding Remarks and Future Perspectives
Mesenchymal stem cells are widely used in the treatment of various diseases due to their ability to home to damaged tissues, their ability to differentiate into various cell types and their pleiotropic effects. However, the therapeutic use of MSCs for cancer has been hampered by contradictory results describing both anti- and pro-tumor effects in preclinical studies. Despite this reality, latest MSC-based therapies bring new hope to cancer patients by offering highly effective anti-cancer treatments in a personalized manner. Among MSC-based therapies, the use of MSCs as Trojan horses to deliver therapeutic factors represents an important step forward to a more efficient cancer treatment. The next challenge is to better understand the interaction between MSCs and cancer cells to improve the clinical safety of MSC-based therapeutic approaches. In this context, the use of MSC-derived extracellular vesicles as a cell-free therapy has emerged as a promising option that circumvent the safety concerns associated with the use of live cells. Further research will shed light on the challenges facing cell-free therapy for cancer. We are definitely moving closer to generate a safe and effective medicinal product for cancer that will improve survival and quality of life of patients suffering this devastating disease.
Author Contributions
VC-G conceived the manuscript. All authors contributed to the manuscript revision and approved the submitted version.
Funding
The authors received financial support from the Andalusian Regional Ministry of Health (PI-0272-2017), the Institute of Health Carlos III, and the Spanish Ministry of Science, Innovation and University, co-funded by Fondos FEDER (CD16/00118, CP19/00046, PI16/00259, BFU2017-83588-P, CP14/00105, PI18/01590, PI17/02104, PIC18/0010, and IC19/0052), the JDRF (2-SRA-2019-837-S-B), and the crowdfunding platform PRECIPITA of the Spanish Foundation for Science and Technology (2018-000237).
Conflict of Interest
The authors declare that the research was conducted in the absence of any commercial or financial relationships that could be construed as a potential conflict of interest.
References
Akimoto, K., Kimura, K., Nagano, M., Takano, S., To’a Salazar, G., Yamashita, T., et al. (2013). Umbilical cord blood-derived mesenchymal stem cells inhibit, but adipose tissue-derived mesenchymal stem cells promote, glioblastoma multiforme proliferation. Stem Cells Dev. 22, 1370–1386. doi: 10.1089/scd.2012.0486
Aliperta, R., Welzel, P. B., Bergmann, R., Freudenberg, U., Berndt, N., Feldmann, A., et al. (2017). Cryogel-supported stem cell factory for customized sustained release of bispecific antibodies for cancer immunotherapy. Sci Rep. 7:42855. doi: 10.1038/srep42855
Aoto, K., Ito, K., and Aoki, S. (2018). Complex formation between platelet-derived growth factor receptor beta and transforming growth factor beta receptor regulates the differentiation of mesenchymal stem cells into cancer-associated fibroblasts. Oncotarget 9, 34090–34102. doi: 10.18632/oncotarget.26124
Bajetto, A., Pattarozzi, A., Corsaro, A., Barbieri, F., Daga, A., Bosio, A., et al. (2017). Different effects of human umbilical cord mesenchymal stem cells on glioblastoma stem cells by direct cell interaction or via released soluble factors. Front. Cell. Neurosci. 11:312. doi: 10.3389/fncel.2017.00312
Barcellos-de-Souza, P., Comito, G., Pons-Segura, C., Taddei, M. L., Gori, V., Becherucci, V., et al. (2016). Mesenchymal stem cells are recruited and activated into carcinoma-associated fibroblasts by prostate cancer microenvironment-derived TGF-beta1. Stem Cell 34, 2536–2547. doi: 10.1002/stem.2412
Barkholt, L., Flory, E., Jekerle, V., Lucas-Samuel, S., Ahnert, P., Bisset, L., et al. (2013). Risk of tumorigenicity in mesenchymal stromal cell-based therapies–bridging scientific observations and regulatory viewpoints. Cytotherapy 15, 753–759. doi: 10.1016/j.jcyt.2013.03.005
Bhoopathi, P., Chetty, C., Gogineni, V. R., Gujrati, M., Dinh, D. H., Rao, J. S., et al. (2011). MMP-2 mediates mesenchymal stem cell tropism towards medulloblastoma tumors. Gene Ther. 18, 692–701. doi: 10.1038/gt.2011.14
Bierie, B., and Moses, H. L. (2006). Tumour microenvironment: TGFbeta: the molecular Jekyll and Hyde of cancer. Nat. Rev. Cancer 6, 506–520. doi: 10.1038/nrc1926
Birnbaum, T., Roider, J., Schankin, C. J., Padovan, C. S., Schichor, C., Goldbrunner, R., et al. (2007). Malignant gliomas actively recruit bone marrow stromal cells by secreting angiogenic cytokines. J. Neurooncol. 83, 241–247. doi: 10.1007/s11060-007-9332-4
Bortolotti, F., Ukovich, L., Razban, V., Martinelli, V., Ruozi, G., Pelos, B., et al. (2015). In vivo therapeutic potential of mesenchymal stromal cells depends on the source and the isolation procedure. Stem Cell Rep. 4, 332–339. doi: 10.1016/j.stemcr.2015.01.001
Bregy, A., Shah, A. H., Diaz, M. V., Pierce, H. E., Ames, P. L., Diaz, D., et al. (2013). The role of Gliadel wafers in the treatment of high-grade gliomas. Expert Rev. Anticancer Ther. 13, 1453–1461. doi: 10.1586/14737140.2013.840090
Brem, H., and Gabikian, P. (2001). Biodegradable polymer implants to treat brain tumors. J. Controll. Release 74, 63–67. doi: 10.1016/s0168-3659(01)00311-x
Byrne, S. N., Knox, M. C., and Halliday, G. M. (2008). TGFbeta is responsible for skin tumour infiltration by macrophages enabling the tumours to escape immune destruction. Immunol. Cell Biol. 86, 92–97. doi: 10.1038/sj.icb.7100116
Capilla-Gonzalez, V., Lopez-Beas, J., Escacena, N., Aguilera, Y., de la Cuesta, A., Ruiz-Salmeron, R., et al. (2018). PDGF restores the defective phenotype of adipose-derived mesenchymal stromal cells from diabetic patients. Mol. Ther. 26, 2696–2709. doi: 10.1016/j.ymthe.2018.08.011
Caplan, A. I., and Dennis, J. E. (2006). Mesenchymal stem cells as trophic mediators. J. Cell. Biochem. 98, 1076–1084. doi: 10.1002/jcb.20886
Chau, M. J., Deveau, T. C., Gu, X., Kim, Y. S., Xu, Y., Yu, S. P., et al. (2018). Delayed and repeated intranasal delivery of bone marrow stromal cells increases regeneration and functional recovery after ischemic stroke in mice. BMC Neurosci. 19:20. doi: 10.1186/s12868-018-0418-z
Chen, X., Lin, X., Zhao, J., Shi, W., Zhang, H., Wang, Y., et al. (2008). A tumor-selective biotherapy with prolonged impact on established metastases based on cytokine gene-engineered MSCs. Mole. Ther. 16, 749–756. doi: 10.1038/mt.2008.3
Chen, Y. C., Gonzalez, M. E., Burman, B., Zhao, X., Anwar, T., Tran, M., et al. (2019). Mesenchymal Stem/Stromal cell engulfment reveals metastatic advantage in Breast Cancer. Cell Rep. 27:e3915. doi: 10.1016/j.celrep.2019.05.084
Ciavarella, S., Grisendi, G., Dominici, M., Tucci, M., Brunetti, O., Dammacco, F., et al. (2012). In vitro anti-myeloma activity of TRAIL-expressing adipose-derived mesenchymal stem cells. Br. J. Haematol. 157, 586–598. doi: 10.1111/j.1365-2141.2012.09082.x
Cocce, V., Farronato, D., Brini, A. T., Masia, C., Gianni, A. B., Piovani, G., et al. (2017). Drug loaded gingival mesenchymal stromal cells (GinPa-MSCs) inhibit in vitro proliferation of oral squamous cell carcinoma. Sci Rep. 7:9376. doi: 10.1038/s41598-017-09175-4
Collino, F., Bruno, S., Lindoso, R. S., and Camussi, G. (2014). miRNA expression in mesenchymal stem cells. Curr. Pathobiol. Rep., 101–107. doi: 10.1007/s40139-014-0045-z
Connick, P., Kolappan, M., Crawley, C., Webber, D. J., Patani, R., Michell, A. W., et al. (2012). Autologous mesenchymal stem cells for the treatment of secondary progressive multiple sclerosis: an open-label phase 2a proof-of-concept study. Lancet Neurol. 11, 150–156. doi: 10.1016/S1474-4422(11)70305-2
Corcione, A., Benvenuto, F., Ferretti, E., Giunti, D., Cappiello, V., Cazzanti, F., et al. (2006). Human mesenchymal stem cells modulate B-cell functions. Blood 107, 367–372.
da Silva Meirelles, L., Chagastelles, P. C., and Nardi, N. B. (2006). Mesenchymal stem cells reside in virtually all post-natal organs and tissues. J. Cell Sci. 119, 2204–2213. doi: 10.1242/jcs.02932
De Ugarte, D. A., Alfonso, Z., Zuk, P. A., Elbarbary, A., Zhu, M., Ashjian, P., et al. (2003). Differential expression of stem cell mobilization-associated molecules on multi-lineage cells from adipose tissue and bone marrow. Immunol. Lett. 89, 267–270. doi: 10.1016/s0165-2478(03)00108-1
Di Nicola, M., Carlo-Stella, C., Magni, M., Milanesi, M., Longoni, P. D., Matteucci, P., et al. (2002). Human bone marrow stromal cells suppress T-lymphocyte proliferation induced by cellular or nonspecific mitogenic stimuli. Blood 99, 3838–3843. doi: 10.1182/blood.v99.10.3838
Dias, S., Shmelkov, S. V., Lam, G., and Rafii, S. (2002). VEGF(165) promotes survival of leukemic cells by Hsp90-mediated induction of Bcl-2 expression and apoptosis inhibition. Blood 99, 2532–2540. doi: 10.1182/blood.v99.7.2532
Diomede, F., Gugliandolo, A., Cardelli, P., Merciaro, I., Ettorre, V., Traini, T., et al. (2018). Three-dimensional printed PLA scaffold and human gingival stem cell-derived extracellular vesicles: a new tool for bone defect repair. Stem Cell Res. Ther. 9:104. doi: 10.1186/s13287-018-0850-0
Dominici, M., Le Blanc, K., Mueller, I., Slaper-Cortenbach, I., Marini, F., Krause, D., et al. (2006). Minimal criteria for defining multipotent mesenchymal stromal cells. the international society for cellular therapy position statement. Cytotherapy 8, 315–317. doi: 10.1080/14653240600855905
Dong, M., How, T., Kirkbride, K. C., Gordon, K. J., Lee, J. D., Hempel, N., et al. (2007). The type III TGF-beta receptor suppresses breast cancer progression. J. Clin. Invest. 117, 206–217.
Du, W., Seah, I., Bougazzoul, O., Choi, G., Meeth, K., Bosenberg, M. W., et al. (2017). Stem cell-released oncolytic herpes simplex virus has therapeutic efficacy in brain metastatic melanomas. Proc. Natl. Acad. Sci. U.S.A. 114, E6157–E6165. doi: 10.1073/pnas.1700363114
English, K., Barry, F. P., and Mahon, B. P. (2008). Murine mesenchymal stem cells suppress dendritic cell migration, maturation and antigen presentation. Immunol. Lett. 115, 50–58. doi: 10.1016/j.imlet.2007.10.002
Escacena, N., Quesada-Hernandez, E., Capilla-Gonzalez, V., Soria, B., and Hmadcha, A. (2015). Bottlenecks in the efficient use of advanced therapy medicinal products based on mesenchymal stromal cells. Stem Cells Int. 2015:895714. doi: 10.1155/2015/895714
Fakiruddin, K. S., Baharuddin, P., Lim, M. N., Fakharuzi, N. A., Yusof, N. A., and Zakaria, Z. (2014). Nucleofection optimization and in vitro anti-tumourigenic effect of TRAIL-expressing human adipose-derived mesenchymal stromal cells. Cancer Cell Int. 14:122. doi: 10.1186/s12935-014-0122-8
Fathi, E., Sanaat, Z., and Farahzadi, R. (2019). Mesenchymal stem cells in acute myeloid leukemia: a focus on mechanisms involved and therapeutic concepts. Blood Res. 54, 165–174. doi: 10.5045/br.2019.54.3.165
Fernandez, O., Izquierdo, G., Fernandez, V., Leyva, L., Reyes, V., Guerrero, M., et al. (2018). Adipose-derived mesenchymal stem cells (AdMSC) for the treatment of secondary-progressive multiple sclerosis: a triple blinded, placebo controlled, randomized phase I/II safety and feasibility study. PLoS One 13:e0195891. doi: 10.1371/journal.pone.0195891
Friedenstein, A. J., Chailakhjan, R. K., and Lalykina, K. S. (1970). The development of fibroblast colonies in monolayer cultures of guinea-pig bone marrow and spleen cells. Cell Tissue Kinet. 3, 393–403. doi: 10.1111/j.1365-2184.1970.tb00347.x
Ganta, C., Chiyo, D., Ayuzawa, R., Rachakatla, R., Pyle, M., Andrews, G., et al. (2009). Rat umbilical cord stem cells completely abolish rat mammary carcinomas with no evidence of metastasis or recurrence 100 days post-tumor cell inoculation. Cancer Res. 69, 1815–1820. doi: 10.1158/0008-5472.CAN-08-2750
Gao, H., Priebe, W., Glod, J., and Banerjee, D. (2009). Activation of signal transducers and activators of transcription 3 and focal adhesion kinase by stromal cell-derived factor 1 is required for migration of human mesenchymal stem cells in response to tumor cell-conditioned medium. Stem Cells. 27, 857–865. doi: 10.1002/stem.23
Garcia-Castro, J., Alemany, R., Cascallo, M., Martinez-Quintanilla, J., Arriero Mdel, M., Lassaletta, A., et al. (2010). Treatment of metastatic neuroblastoma with systemic oncolytic virotherapy delivered by autologous mesenchymal stem cells: an exploratory study. Cancer Gene Ther. 17, 476–483. doi: 10.1038/cgt.2010.4
Gervois, P., Struys, T., Hilkens, P., Bronckaers, A., Ratajczak, J., Politis, C., et al. (2015). Neurogenic maturation of human dental pulp stem cells following neurosphere generation induces morphological and electrophysiological characteristics of functional neurons. Stem Cells Dev. 24, 296–311. doi: 10.1089/scd.2014.0117
Gong, W., Guo, M., Han, Z., Wang, Y., Yang, P., Xu, C., et al. (2016). Mesenchymal stem cells stimulate intestinal stem cells to repair radiation-induced intestinal injury. Cell Death Dis. 7:e2387. doi: 10.1038/cddis.2016.276
Gotherstrom, C., Westgren, M., Shaw, S. W., Astrom, E., Biswas, A., Byers, P. H., et al. (2014). Pre- and postnatal transplantation of fetal mesenchymal stem cells in osteogenesis imperfecta: a two-center experience. Stem Cells Transl. Medi. 3, 255–264. oi: 10.5966/sctm.2013-0090 doi: 10.5966/sctm.2013-0090
Guasch, G., Schober, M., Pasolli, H. A., Conn, E. B., Polak, L., and Fuchs, E. (2007). Loss of TGFbeta signaling destabilizes homeostasis and promotes squamous cell carcinomas in stratified epithelia. Cancer Cell 12, 313–327. doi: 10.1016/j.ccr.2007.08.020
Guo, X., Zhao, Y., Yan, H., Yang, Y., Shen, S., Dai, X., et al. (2017). Single tumor-initiating cells evade immune clearance by recruiting type II macrophages. Genes Dev. 31, 247–259. doi: 10.1101/gad.294348.116
Guo, X. R., Yang, Z. S., Tang, X. J., Zou, D. D., Gui, H., Wang, X. L., et al. (2016). The application of mRNA-based gene transfer in mesenchymal stem cell-mediated cytotoxicity of glioma cells. Oncotarget 7, 55529–55542. doi: 10.18632/oncotarget.10835
Guo, Y., Zhang, Z., Xu, X., Xu, Z., Wang, S., Huang, D., et al. (2019). Menstrual blood-derived stem cells as delivery vehicles for oncolytic adenovirus virotherapy for Colorectal Cancer. Stem Cells Dev. 28, 882–896. doi: 10.1089/scd.2018.0222
Hao, C., Beguinot, F., Condorelli, G., Trencia, A., Van Meir, E. G., Yong, V. W., et al. (2001). Induction and intracellular regulation of tumor necrosis factor-related apoptosis-inducing ligand (TRAIL) mediated apotosis in human malignant glioma cells. Cancer Res. 61, 1162–1170.
Ho, I. A., Toh, H. C., Ng, W. H., Teo, Y. L., Guo, C. M., Hui, K. M., et al. (2013). Human bone marrow-derived mesenchymal stem cells suppress human glioma growth through inhibition of angiogenesis. Stem Cells 31, 146–155. doi: 10.1002/stem.1247
Honczarenko, M., Le, Y., Swierkowski, M., Ghiran, I., Glodek, A. M., and Silberstein, L. E. (2006). Human bone marrow stromal cells express a distinct set of biologically functional chemokine receptors. Stem cells 24, 1030–1041. doi: 10.1634/stemcells.2005-0319
Jiang, X., Fitch, S., Wang, C., Wilson, C., Li, J., Grant, G. A., et al. (2016). Nanoparticle engineered TRAIL-overexpressing adipose-derived stem cells target and eradicate glioblastoma via intracranial delivery. Proc. Natl. Acad. Sci. U.S.A. 113, 13857–13862. doi: 10.1073/pnas.1615396113
Jiang, Y., Jahagirdar, B. N., Reinhardt, R. L., Schwartz, R. E., Keene, C. D., Ortiz-Gonzalez, X. R., et al. (2002). Pluripotency of mesenchymal stem cells derived from adult marrow. Nature 418, 41–49.
Johansson, M., Oudin, A., Tiemann, K., Bernard, A., Golebiewska, A., Keunen, O., et al. (2013). The soluble form of the tumor suppressor Lrig1 potently inhibits in vivo glioma growth irrespective of EGF receptor status. Neuro Oncol 15, 1200–1211. doi: 10.1093/neuonc/not054
Jones, J., Estirado, A., Redondo, C., Pacheco-Torres, J., Sirerol-Piquer, M. S., Garcia-Verdugo, J. M., et al. (2015). Mesenchymal stem cells improve motor functions and decrease neurodegeneration in ataxic mice. Mol. Ther. 23, 130–138. doi: 10.1038/mt.2014.143
Jotzu, C., Alt, E., Welte, G., Li, J., Hennessy, B. T., Devarajan, E., et al. (2011). Adipose tissue derived stem cells differentiate into carcinoma-associated fibroblast-like cells under the influence of tumor derived factors. Cell. Oncol. 34, 55–67. doi: 10.1007/s13402-011-0012-1
Julier, Z., Park, A. J., Briquez, P. S., and Martino, M. M. (2017). Promoting tissue regeneration by modulating the immune system. Acta Biomater. 53, 13–28. doi: 10.1016/j.actbio.2017.01.056
Kalimuthu, S., Oh, J. M., Gangadaran, P., Zhu, L., Lee, H. W., Rajendran, R. L., et al. (2017). In Vivo Tracking of Chemokine Receptor CXCR4-Engineered Mesenchymal Stem Cell Migration by Optical Molecular Imaging. Stem Cells Int. 2017:8085637. doi: 10.1155/2017/8085637
Kalluri, R. (2016). The biology and function of fibroblasts in cancer. Nat. Rev. Cancer 16, 582–598. doi: 10.1038/nrc.2016.73
Karantalis, V., DiFede, D. L., Gerstenblith, G., Pham, S., Symes, J., Zambrano, J. P., et al. (2014). Autologous mesenchymal stem cells produce concordant improvements in regional function, tissue perfusion, and fibrotic burden when administered to patients undergoing coronary artery bypass grafting: the prospective randomized study of mesenchymal stem cell therapy in patients undergoing cardiac surgery (PROMETHEUS) trial. Circ. Res. 114, 1302–1310. doi: 10.1161/CIRCRESAHA.114.303180
Karnoub, A. E., Dash, A. B., Vo, A. P., Sullivan, A., Brooks, M. W., Bell, G. W., et al. (2007). Mesenchymal stem cells within tumour stroma promote breast cancer metastasis. Nature 449, 557–563. doi: 10.1038/nature06188
Khakoo, A. Y., Pati, S., Anderson, S. A., Reid, W., Elshal, M. F., Rovira, I. I., et al. (2006). Human mesenchymal stem cells exert potent antitumorigenic effects in a model of Kaposi’s sarcoma. J. Exp. Med. 203, 1235–1247. doi: 10.1084/jem.20051921
Kim, J. S., Kim, J. G., Moon, M. Y., Jeon, C. Y., Won, H. Y., Kim, H. J., et al. (2006). Transforming growth factor-beta1 regulates macrophage migration via RhoA. Blood 108, 1821–1829. doi: 10.1182/blood-2005-10-009191
Kolaczkowska, E., and Kubes, P. (2013). Neutrophil recruitment and function in health and inflammation. Nat. Rev. Immunol. 13, 159–175. doi: 10.1038/nri3399
Konig, A., Menzel, T., Lynen, S., Wrazel, L., Rosen, A., Al-Katib, A., et al. (1997). Basic fibroblast growth factor (bFGF) upregulates the expression of bcl-2 in B cell chronic lymphocytic leukemia cell lines resulting in delaying apoptosis. Leukemia 11, 258–265. doi: 10.1038/sj.leu.2400556
Lang, F. M., Hossain, A., Gumin, J., Momin, E. N., Shimizu, Y., Ledbetter, D., et al. (2018). Mesenchymal stem cells as natural biofactories for exosomes carrying miR-124a in the treatment of gliomas. Neuro Oncol. 20, 380–390. doi: 10.1093/neuonc/nox152
Layek, B., Sadhukha, T., Panyam, J., and Prabha, S. (2018). Nano-engineered mesenchymal stem cells increase therapeutic efficacy of anticancer drug through true active tumor targeting. Mol. Cancer Ther. 17, 1196–1206. doi: 10.1158/1535-7163.MCT-17-0682
Lee, H. K., Finniss, S., Cazacu, S., Bucris, E., Ziv-Av, A., Xiang, C., et al. (2013). Mesenchymal stem cells deliver synthetic microRNA mimics to glioma cells and glioma stem cells and inhibit their cell migration and self-renewal. Oncotarget 4, 346–361.
Lee, K. D., Kuo, T. K., Whang-Peng, J., Chung, Y. F., Lin, C. T., Chou, S. H., et al. (2004). In vitro hepatic differentiation of human mesenchymal stem cells. Hepatology 40, 1275–1284.
Li, G. C., Zhang, H. W., Zhao, Q. C., Sun, L. I., Yang, J. J., Hong, L., et al. (2016). Mesenchymal stem cells promote tumor angiogenesis via the action of transforming growth factor beta1. Oncol. Lett. 11, 1089–1094. doi: 10.3892/ol.2015.3997
Li, Q., Wijesekera, O., Salas, S. J., Wang, J. Y., Zhu, M., Aprhys, C., et al. (2014). Mesenchymal stem cells from human fat engineered to secrete BMP4 are nononcogenic, suppress brain cancer, and prolong survival. Clin. Cancer Res. 20, 2375–2387. doi: 10.1158/1078-0432.CCR-13-1415
Li, W., Zhou, Y., Yang, J., Zhang, X., Zhang, H., Zhang, T., et al. (2015). Gastric cancer-derived mesenchymal stem cells prompt gastric cancer progression through secretion of interleukin-8. J. Exp. Clin. Cancer Res. 34:52. doi: 10.1186/s13046-015-0172-3
Li, X., Liu, L. L., Yao, J. L., Wang, K., and Ai, H. (2019). Human umbilical cord mesenchymal stem cell-derived extracellular vesicles inhibit endometrial cancer cell proliferation and migration through delivery of exogenous miR-302a. Stem Cells Int. 2019:8108576. doi: 10.1155/2019/8108576
Li, Y., Zhang, D., Xu, L., Dong, L., Zheng, J., Lin, Y., et al. (2019). Cell-cell contact with proinflammatory macrophages enhances the immunotherapeutic effect of mesenchymal stem cells in two abortion models. Cell. Mol. Immunol. 16, 908–920. doi: 10.1038/s41423-019-0204-6
Liu, C. B., Huang, H., Sun, P., Ma, S. Z., Liu, A. H., Xue, J., et al. (2016). Human umbilical cord-derived mesenchymal stromal cells improve left ventricular function, perfusion, and remodeling in a porcine model of chronic myocardial ischemia. Stem Cells Transl. Med. 5, 1004–1013. doi: 10.5966/sctm.2015-0298
Liu, X., Hu, J., Li, Y., Cao, W., Wang, Y., Ma, Z., et al. (2018). Mesenchymal stem cells expressing interleukin-18 inhibit breast cancer in a mouse model. Onco. Lett. 15, 6265–6274. doi: 10.3892/ol.2018.8166
Lo, B., and Parham, L. (2009). Ethical issues in stem cell research. Endocr. Rev. 30, 204–213. doi: 10.1210/er.2008-0031
Lourenco, S., Teixeira, V. H., Kalber, T., Jose, R. J., Floto, R. A., and Janes, S. M. (2015). Macrophage migration inhibitory factor-CXCR4 is the dominant chemotactic axis in human mesenchymal stem cell recruitment to tumors. J. Immunol. 194, 3463–3474. doi: 10.4049/jimmunol.1402097
Lu, L., Chen, G., Yang, J., Ma, Z., Yang, Y., Hu, Y., et al. (2019). Bone marrow mesenchymal stem cells suppress growth and promote the apoptosis of glioma U251 cells through downregulation of the PI3K/AKT signaling pathway. Biomed. Pharmacother. 112:108625. doi: 10.1016/j.biopha.2019.108625
Ma, J., Liu, N., Yi, B., Zhang, X., Gao, B. B., Zhang, Y., et al. (2015). Transplanted hUCB-MSCs migrated to the damaged area by SDF-1/CXCR4 signaling to promote functional recovery after traumatic brain injury in rats. Neurol. Res. 37, 50–56. doi: 10.1179/1743132814Y.0000000399
Maccario, R., Podesta, M., Moretta, A., Cometa, A., Comoli, P., Montagna, D., et al. (2005). Interaction of human mesenchymal stem cells with cells involved in alloantigen-specific immune response favors the differentiation of CD4+ T-cell subsets expressing a regulatory/suppressive phenotype. Haematologica 90, 516–525.
Maltman, D. J., Hardy, S. A., and Przyborski, S. A. (2011). Role of mesenchymal stem cells in neurogenesis and nervous system repair. Neurochem. Int. 59, 347–356. doi: 10.1016/j.neuint.2011.06.008
Mangraviti, A., Tzeng, S. Y., Gullotti, D., Kozielski, K. L., Kim, J. E., Seng, M., et al. (2016). Non-virally engineered human adipose mesenchymal stem cells produce BMP4, target brain tumors, and extend survival. Biomaterials 100, 53–66. doi: 10.1016/j.biomaterials.2016.05.025
Meirelles Lda, S., Fontes, A. M., Covas, D. T., and Caplan, A. I. (2009). Mechanisms involved in the therapeutic properties of mesenchymal stem cells. Cytok.i Growth Factor Revi. 20, 419–427. doi: 10.1016/j.cytogfr.2009.10.002
Melen, G. J., Franco-Luzon, L., Ruano, D., Gonzalez-Murillo, A., Alfranca, A., Casco, F., et al. (2016). Influence of carrier cells on the clinical outcome of children with neuroblastoma treated with high dose of oncolytic adenovirus delivered in mesenchymal stem cells. Cancer Lett. 371, 161–170. doi: 10.1016/j.canlet.2015.11.036
Mishra, P. J., Mishra, P. J., Humeniuk, R., Medina, D. J., Alexe, G., Mesirov, J. P., et al. (2008). Carcinoma-associated fibroblast-like differentiation of human mesenchymal stem cells. Cancer Res. 68, 4331–4339. doi: 10.1158/0008-5472.CAN-08-0943
Moku, G., Layek, B., Trautman, L., Putnam, S., Panyam, J., and Prabha, S. (2019). Improving payload capacity and anti-tumor efficacy of mesenchymal stem cells using TAT peptide functionalized polymeric nanoparticles. Cancers 11:E491. doi: 10.3390/cancers11040491
Nakamizo, A., Marini, F., Amano, T., Khan, A., Studeny, M., Gumin, J., et al. (2005). Human bone marrow-derived mesenchymal stem cells in the treatment of gliomas. Cancer Res. 65, 3307–3318.
Oh, S. H., Kim, H. N., Park, H. J., Shin, J. Y., and Lee, P. H. (2015). Mesenchymal stem cells increase hippocampal neurogenesis and neuronal differentiation by enhancing the wnt signaling pathway in an Alzheimer’s Disease model. Cell Transplant. 24, 1097–1109. doi: 10.3727/096368914X679237
Otsu, K., Das, S., Houser, S. D., Quadri, S. K., Bhattacharya, S., and Bhattacharya, J. (2009). Concentration-dependent inhibition of angiogenesis by mesenchymal stem cells. Blood 113, 4197–4205. doi: 10.1182/blood-2008-09-176198
Ozeki, N., Muneta, T., Koga, H., Nakagawa, Y., Mizuno, M., Tsuji, K., et al. (2016). Not single but periodic injections of synovial mesenchymal stem cells maintain viable cells in knees and inhibit osteoarthritis progression in rats. Osteoarthr. Cartil. 24, 1061–1070. doi: 10.1016/j.joca.2015.12.018
Pacioni, S., D’Alessandris, Q. G., Giannetti, S., Morgante, L., De Pascalis, I., Cocce, V., et al. (2015). Mesenchymal stromal cells loaded with paclitaxel induce cytotoxic damage in glioblastoma brain xenografts. Stem Cell Res. Ther. 6:194. doi: 10.1186/s13287-015-0185-z
Paunescu, V., Deak, E., Herman, D., Siska, I. R., Tanasie, G., Bunu, C., et al. (2007). In vitro differentiation of human mesenchymal stem cells to epithelial lineage. J. Cell. Mol. Med. 11, 502–508.
Pelegrin, M., Marin, M., Noel, D., Del Rio, M., Saller, R., Stange, J., et al. (1998). Systemic long-term delivery of antibodies in immunocompetent animals using cellulose sulphate capsules containing antibody-producing cells. Gene Ther. 5, 828–834. doi: 10.1038/sj.gt.3300632
Perez, L. M., de Lucas, B., and Galvez, B. G. (2018). Unhealthy stem cells: when health conditions upset stem cell properties. Cell. Physiol. Biochem. 46, 1999–2016. doi: 10.1159/000489440
Powell, D., Lou, M., Barros Becker, F., and Huttenlocher, A. (2018). Cxcr1 mediates recruitment of neutrophils and supports proliferation of tumor-initiating astrocytes in vivo. Sci Rep. 8:13285. doi: 10.1038/s41598-018-31675-0
Prockop, D. J., and Oh, J. Y. (2012). Mesenchymal stem/stromal cells (MSCs): role as guardians of inflammation. Mol. Ther. 20, 14–20. doi: 10.1038/mt.2011.211
Qiao, L., Xu, Z. L., Zhao, T. J., Ye, L. H., and Zhang, X. D. (2008a). Dkk-1 secreted by mesenchymal stem cells inhibits growth of breast cancer cells via depression of Wnt signalling. Cancer Lett. 269, 67–77. doi: 10.1016/j.canlet.2008.04.032
Qiao, L., Xu, Z., Zhao, T., Zhao, Z., Shi, M., Zhao, R. C., et al. (2008b). Suppression of tumorigenesis by human mesenchymal stem cells in a hepatoma model. Cell Res. 18, 500–507. doi: 10.1038/cr.2008.40
Quevedo, H. C., Hatzistergos, K. E., Oskouei, B. N., Feigenbaum, G. S., Rodriguez, J. E., Valdes, D., et al. (2009). Allogeneic mesenchymal stem cells restore cardiac function in chronic ischemic cardiomyopathy via trilineage differentiating capacity. Proc. Natl. Acad. Sci. U.S.A. 106, 14022–14027. doi: 10.1073/pnas.0903201106
Ramos-Zuriga, R., Gonzalez-Perez, O., Macedas-Ornelas, A., Capilla-Gonzlez, V., and Quiñones-Hinojosa, A. (2012). Ethical Implications in the Use of Embryonic and Adult Neural Stem Cells. Stem Cells Int. 2012:7.
Rani, S., Ryan, A. E., Griffin, M. D., and Ritter, T. (2015). Mesenchymal stem cell-derived extracellular vesicles: toward cell-free therapeutic applications. Mol. Ther. 23, 812–823. doi: 10.1038/mt.2015.44
Rehorova, M., Vargova, I., Forostyak, S., Vackova, I., Turnovcova, K., Kupcova Skalnikova, H., et al. (2019). A combination of intrathecal and intramuscular application of human mesenchymal stem cells partly reduces the activation of necroptosis in the spinal cord of SOD1(G93A) Rats. Stem Cells Transl. Med. 8, 535–547. doi: 10.1002/sctm.18-0223
Ren, G., Zhao, X., Zhang, L., Zhang, J., L’Huillier, A., Ling, W., et al. (2010). Inflammatory cytokine-induced intercellular adhesion molecule-1 and vascular cell adhesion molecule-1 in mesenchymal stem cells are critical for immunosuppression. J. Immunol. 184, 2321–2328. doi: 10.4049/jimmunol.0902023
Rincon, E., Cejalvo, T., Kanojia, D., Alfranca, A., Rodriguez-Milla, M. A., Gil Hoyos, R. A., et al. (2017). Mesenchymal stem cell carriers enhance antitumor efficacy of oncolytic adenoviruses in an immunocompetent mouse model. Oncotarget 8, 45415–45431. doi: 10.18632/oncotarget.17557
Ringe, J., Strassburg, S., Neumann, K., Endres, M., Notter, M., Burmester, G. R., et al. (2007). Towards in situ tissue repair: human mesenchymal stem cells express chemokine receptors CXCR1. CXCR2 and CCR2, and migrate upon stimulation with CXCL8 but not CCL2. J. Cell. Biochem. 101, 135–146. doi: 10.1002/jcb.21172
Rivera, F. J., de la Fuente, A. G., Zhao, C., Silva, M. E., Gonzalez, G. A., Wodnar, R., et al. (2019). Aging restricts the ability of mesenchymal stem cells to promote the generation of oligodendrocytes during remyelination. Glia 67, 1510–1525. doi: 10.1002/glia.23624
Rushkevich, Y. N., Kosmacheva, S. M., Zabrodets, G. V., Ignatenko, S. I., Goncharova, N. V., Severin, I. N., et al. (2015). The use of autologous mesenchymal stem cells for cell therapy of patients with amyotrophic lateral sclerosis in belarus. Bull. Exp. Biol. Med. 159, 576–581. doi: 10.1007/s10517-015-3017-3
Sakai, S., Kawabata, K., Tanaka, S., Harimoto, N., Hashimoto, I., Mu, C., et al. (2005). Subsieve-size agarose capsules enclosing ifosfamide-activating cells: a strategy toward chemotherapeutic targeting to tumors. Mol. Cancer Ther. 4, 1786–1790. doi: 10.1158/1535-7163.mct-05-0227
Salgado, A. J., Reis, R. L., Sousa, N. J., and Gimble, J. M. (2010). Adipose tissue derived stem cells secretome: soluble factors and their roles in regenerative medicine. Curr. Stem Cell Res. Ther. 5, 103–110. doi: 10.2174/157488810791268564
Schwenter, F., Zarei, S., Luy, P., Padrun, V., Bouche, N., Lee, J. S., et al. (2011). Cell encapsulation technology as a novel strategy for human anti-tumor immunotherapy. Cancer Gene Ther. 18, 553–562. doi: 10.1038/cgt.2011.22
Sharif, S., Ghahremani, M. H., and Soleimani, M. (2018). Delivery of exogenous miR-124 to glioblastoma multiform cells by wharton’s jelly mesenchymal stem cells decreases cell proliferation and migration, and confers chemosensitivity. Stem Cell Rev. 14, 236–246. doi: 10.1007/s12015-017-9788-3
Sheets, K. T., Bago, J. R., and Hingtgen, S. D. (2018). Delivery of Cytotoxic Mesenchymal Stem Cells with Biodegradable Scaffolds for Treatment of Postoperative Brain Cancer. Methods Mol. Biol. 1831, 49–58. doi: 10.1007/978-1-4939-8661-3_5
Shen, C. J., Chan, T. F., Chen, C. C., Hsu, Y. C., Long, C. Y., and Lai, C. S. (2016). Human umbilical cord matrix-derived stem cells expressing interferon-beta gene inhibit breast cancer cells via apoptosis. Oncotarget 7, 34172–34179. doi: 10.18632/oncotarget.8997
Si, Y., Zhao, Y., Hao, H., Liu, J., Guo, Y., Mu, Y., et al. (2012). Infusion of mesenchymal stem cells ameliorates hyperglycemia in type 2 diabetic rats: identification of a novel role in improving insulin sensitivity. Diabetes Metab. Res. Rev. 61, 1616–1625. doi: 10.2337/db11-1141
Smith, S. J., Tyler, B. M., Gould, T., Veal, G. J., Gorelick, N., Rowlinson, J., et al. (2019). Overall survival in malignant glioma is significantly prolonged by neurosurgical delivery of etoposide and temozolomide from a thermo-responsive biodegradable paste. Clin. Cancer Res. 25, 5094–5106. doi: 10.1158/1078-0432.CCR-18-3850
Song, J.-Y., Kang, H. J., Ju, H. M., Park, A., Park, H., Hong, J. S., et al. (2019). Umbilical cord-derived mesenchymal stem cell extracts ameliorate atopic dermatitis in mice by reducing the T cell responses. Sci. Rep. 9:6623. doi: 10.1038/s41598-019-42964-7
Song, L., and Tuan, R. S. (2004). Transdifferentiation potential of human mesenchymal stem cells derived from bone marrow. FASEB J. 18, 980–982. doi: 10.1096/fj.03-1100fje
Sordi, V., Malosio, M. L., Marchesi, F., Mercalli, A., Melzi, R., Giordano, T., et al. (2005). Bone marrow mesenchymal stem cells express a restricted set of functionally active chemokine receptors capable of promoting migration to pancreatic islets. Blood 106, 419–427. doi: 10.1182/blood-2004-09-3507
Soria, B., Martin-Montalvo, A., Aguilera, Y., Mellado-Damas, N., López-Beas, J., Herrera-Herrera, I., et al. (2019). Human mesenchymal stem cells prevent neurological complications of radiotherapy. Front. Cell. Neurosci. 13:204. doi: 10.3389/fncel.2019.00204
Sotiropoulou, P. A., Perez, S. A., Gritzapis, A. D., Baxevanis, C. N., and Papamichail, M. (2006). Interactions between human mesenchymal stem cells and natural killer cells. Stem Cells 24, 74–85. doi: 10.1634/stemcells.2004-0359
Spaggiari, G. M., Abdelrazik, H., Becchetti, F., and Moretta, L. (2009). MSCs inhibit monocyte-derived DC maturation and function by selectively interfering with the generation of immature DCs: central role of MSC-derived prostaglandin E2. Blood 113, 6576–6583. doi: 10.1182/blood-2009-02-203943
Studeny, M., Marini, F. C., Champlin, R. E., Zompetta, C., Fidler, I. J., and Andreeff, M. (2002). Bone marrow-derived mesenchymal stem cells as vehicles for interferon-beta delivery into tumors. Cancer Res. 62, 3603–3608.
Takeda, K., Hayakawa, Y., Smyth, M. J., Kayagaki, N., Yamaguchi, N., Kakuta, S., et al. (2001). Involvement of tumor necrosis factor-related apoptosis-inducing ligand in surveillance of tumor metastasis by liver natural killer cells. Nat. Med. 7, 94–100. doi: 10.1038/83416
Thakkar, U. G., Trivedi, H. L., Vanikar, A. V., and Dave, S. D. (2015). Insulin-secreting adipose-derived mesenchymal stromal cells with bone marrow-derived hematopoietic stem cells from autologous and allogenic sources for type 1 diabetes mellitus. Cytotherapy 17, 940–947. doi: 10.1016/j.jcyt.2015.03.608
van Velthoven, C. T., Sheldon, R. A., Kavelaars, A., Derugin, N., Vexler, Z. S., Willemen, H. L., et al. (2013). Mesenchymal stem cell transplantation attenuates brain injury after neonatal stroke. Stroke 44, 1426–1432. doi: 10.1161/STROKEAHA.111.000326
Vega, A., Martin-Ferrero, M. A., Del Canto, F., Alberca, M., Garcia, V., Munar, A., et al. (2015). Treatment of knee osteoarthritis with allogeneic bone marrow mesenchymal stem cells: a randomized controlled trial. Transplantation 99, 1681–1690. doi: 10.1097/tp.0000000000000678
Von Luttichau, I., Notohamiprodjo, M., Wechselberger, A., Peters, C., Henger, A., Seliger, C., et al. (2005). Human adult CD34- progenitor cells functionally express the chemokine receptors CCR1, CCR4, CCR7, CXCR5, and CCR10 but not CXCR4. Stem Cells Dev. 14, 329–336. doi: 10.1089/scd.2005.14.329
Wang, Q. L., Wang, H. J., Li, Z. H., Wang, Y. L., Wu, X. P., and Tan, Y. Z. (2017). Mesenchymal stem cell-loaded cardiac patch promotes epicardial activation and repair of the infarcted myocardium. J. Cell Mol. Med. 21, 1751–1766. doi: 10.1111/jcmm.13097
Wang, X., Gao, J., Ouyang, X., Wang, J., Sun, X., and Lv, Y. (2018). Mesenchymal stem cells loaded with paclitaxel-poly(lactic-co-glycolic acid) nanoparticles for glioma-targeting therapy. Int. J. Nanomed. 13, 5231–5248. doi: 10.2147/IJN.S167142
Wiley, S. R., Schooley, K., Smolak, P. J., Din, W. S., Huang, C. P., Nicholl, J. K., et al. (1995). Identification and characterization of a new member of the TNF family that induces apoptosis. Immunity 3, 673–682. doi: 10.1016/1074-7613(95)90057-8
Wobus, M., List, C., Dittrich, T., Dhawan, A., Duryagina, R., Arabanian, L. S., et al. (2015). Breast carcinoma cells modulate the chemoattractive activity of human bone marrow-derived mesenchymal stromal cells by interfering with CXCL12. Int. J. Cancer 136, 44–54. doi: 10.1002/ijc.28960
Wynn, R. F., Hart, C. A., Corradi-Perini, C., O’Neill, L., Evans, C. A., Wraith, J. E., et al. (2004). A small proportion of mesenchymal stem cells strongly expresses functionally active CXCR4 receptor capable of promoting migration to bone marrow. Blood 104, 2643–2645. doi: 10.1182/blood-2004-02-0526
Xu, W. T., Bian, Z. Y., Fan, Q. M., Li, G., and Tang, T. T. (2009). Human mesenchymal stem cells (hMSCs) target osteosarcoma and promote its growth and pulmonary metastasis. Cancer Lett. 281, 32–41. doi: 10.1016/j.canlet.2009.02.022
Zeng, X., Qiu, X. C., Ma, Y. H., Duan, J. J., Chen, Y. F., Gu, H. Y., et al. (2015). Integration of donor mesenchymal stem cell-derived neuron-like cells into host neural network after rat spinal cord transection. Biomaterials 53, 184–201. doi: 10.1016/j.biomaterials.2015.02.073
Keywords: mesenchymal stem cells, cancer, cell therapy, therapeutic agents, anti-tumor activity
Citation: Hmadcha A, Martin-Montalvo A, Gauthier BR, Soria B and Capilla-Gonzalez V (2020) Therapeutic Potential of Mesenchymal Stem Cells for Cancer Therapy. Front. Bioeng. Biotechnol. 8:43. doi: 10.3389/fbioe.2020.00043
Received: 27 November 2019; Accepted: 21 January 2020;
Published: 05 February 2020.
Edited by:
Hugo Guerrero-Cazares, Mayo Clinic, United StatesReviewed by:
Rajendra K. Singh, Institute of Tissue Regeneration Engineering (ITREN), South KoreaLia Rimondini, University of Eastern Piedmont, Italy
Copyright © 2020 Hmadcha, Martin-Montalvo, Gauthier, Soria and Capilla-Gonzalez. This is an open-access article distributed under the terms of the Creative Commons Attribution License (CC BY). The use, distribution or reproduction in other forums is permitted, provided the original author(s) and the copyright owner(s) are credited and that the original publication in this journal is cited, in accordance with accepted academic practice. No use, distribution or reproduction is permitted which does not comply with these terms.
*Correspondence: Abdelkrim Hmadcha, a2htYWRjaGFAdXBvLmVz; Vivian Capilla-González, dml2aWFuLmNhcGlsbGFAY2FiaW1lci5lcw==
†Lead contact