- 1Technical Biology, Institute of Process Engineering in Life Science, Karlsruhe Institute of Technology, Karlsruhe, Germany
- 2Faculty of Science, School of Molecular & Cell Biology, University of the Witwatersrand, Johannesburg, South Africa
The microbial production of bulk chemicals from waste gas is becoming a pertinent alternative to industrial strategies that rely on fossil fuels as substrate. Acetogens can use waste gas substrates or syngas (CO, CO2, H2) to produce chemicals, such as acetate or ethanol, but as the feed gas often contains oxygen, which inhibits acetogen growth and product formation, a cost-prohibitive chemical oxygen removal step is necessary. Here, we have developed a two-phase microbial system to facilitate acetate production using a gas mixture containing CO and O2. In the first phase the facultative anaerobic carboxydotroph Parageobacillus thermoglucosidasius was used to consume residual O2 and produce H2 and CO2, which was subsequently utilized by the acetogen Clostridium ljungdahlii for the production of acetate. From a starting amount of 3.3 mmol of CO, 0.52 mmol acetate was produced in the second phase by C. ljungdahlii. In this set-up, the yield achieved was 0.16 mol acetate/mol CO, a 63% of the theoretical maximum. This system has the potential to be developed for the production of a broad range of bulk chemicals from oxygen-containing waste gas by using P. thermoglucosidasius as an oxygen scrubbing tool.
Introduction
The production of value-added chemicals, such as organic acids (e.g., acetate and succinate), glycerol derivatives (e.g., 2,3-butanediol and 1,3-propanediol) and alcohols (e.g., butanol, methanol, and ethanol) is still largely reliant on the use of fossil fuels as substrate (Hatti-Kaul et al., 2007; Zhang et al., 2017). Dwindling reserves and negative environmental effects associated with fossil fuel emissions underpin the necessity to develop novel inexpensive and environmentally friendly means of producing such chemicals. One potential alternative involves the use of synthesis gas (syngas) which consists primarily of hydrogen (H2), carbon dioxide (CO2), and carbon monoxide (CO) (Teixeira et al., 2018). Syngas can be produced from natural gas or coal as well as inexpensive feedstocks, such as lignocellulose (Barnard et al., 2010). Some microorganisms are capable of metabolizing the components from syngas into a wide range of chemical compounds, such as acetate, butanol, lactate, and ethanol (Liou et al., 2005; Drake et al., 2006; Köpke and Dürre, 2011; Daniell et al., 2012). Acetogens are microorganisms that are capable of producing acetyl-CoA out of two molecules of CO2 or CO via the Wood-Ljungdahl (W-L) pathway (Diekert and Wohlfarth, 1994). For example, Clostridium ljungdahlii can ferment CO2/H2 or CO/H2 via the W-L or Acetyl-CoA-pathway into acetyl-CoA. Further conversions lead to acetate as a main product and ethanol, butyrate, butanol and 2,3-butanediol in smaller amounts (Tanner et al., 1993; Köpke and Dürre, 2011). However, C. ljungdahlii and most other syngas fermenters are strict anaerobes, which limits the use of industrial waste gasses containing O2 (Liew et al., 2016). This can be linked to the oxygen sensitivity of many enzymes central to syngas fermentation pathways. For example, the key W-L pathway enzymes pyruvate-ferredoxin oxidoreductase (PFOR) and pyruvate formate lyase (PFL) are sensitive to very low levels of oxygen (Meinecke et al., 1989; Ragsdale and Wood, 1991; Bock et al., 1996; Brown et al., 1998; Becker et al., 1999; Imlay, 2009; Yang et al., 2009; Nakayama et al., 2013; Shen et al., 2017). In order to use microorganism to convert the components of industrial waste gas to a value-added product, O2 has to be removed first, a step which is cost prohibitive (Liew et al., 2016).
Parageobacillus thermoglucosidasius is a facultative anaerobic thermophile which is able to produce H2 and CO2 via the water-gas shift (WGS) when grown in the presence of a gas mixture consisting of CO and air (Mohr et al., 2018a,b) (Figure 1). Initially P. thermoglucosidasius supports its growth via aerobic respiration and once O2 is exhausted, it shifts to the anaerobic WGS pathway (Mohr et al., 2018a,b). This metabolic shift makes P. thermoglucosidasius a potential biological tool for the removal of O2 from syngas mixtures to be utilized in subsequent anaerobic production of value-added products. Furthermore, the CO2 and H2 produced by this organism can serve as substrates for the production of chemical compounds, such as ethanol, butanol, butyric acid, acetate and methane (Drake et al., 2006; Köpke et al., 2010; Liew et al., 2016).
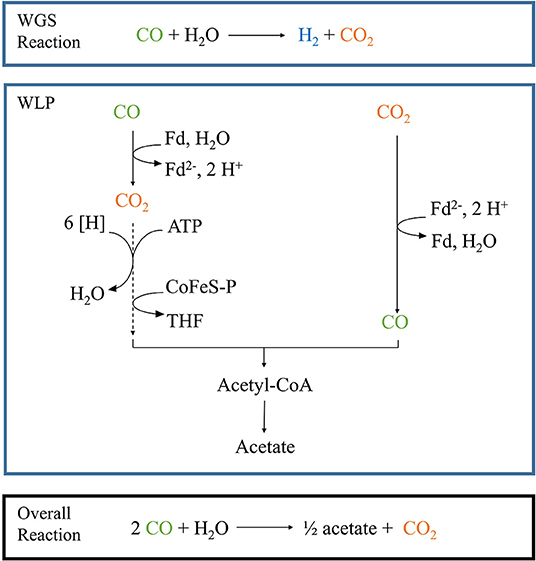
Figure 1. Schematic pathway of the combined WGS reaction and Wood-Ljungdahl pathway. The blue boxes depict each pathway separately, while the black box shows the result of the combined reactions in our particular set-up. Fd, oxidized ferredoxin; Fd2−, reduced ferredoxin; [H], reduction equivalents. Dotted lines depict a multiple-step reaction.
In the present study we have undertaken sequential fermentation with P. thermoglucosidasius and C. ljungdahlii and demonstrate the potential of using an O2-depleting facultative anaerobe to facilitate the anaerobic production of value-added products from an artificial waste gas, a gas mixture containing both CO and O2.
Materials and Methods
Microorganisms and Media
P. thermoglucosidasius DSM 6285 and Clostridium ljungdahlii DSM 13528T were obtained from the Deutsche Sammlung von Mikroorganismen und Zellkulturen (DSMZ, Braunschweig, Germany).
P. thermoglucosidasius DSM 6285 was cultivated in mLB (modified Luria-Bertani) medium (g/l): tryptone (10), yeast extract (5), NaCl (5); 1.25 ml/l NaOH (10% w/v), and 1 ml/l of each of the filter-sterilized stock solutions 1.05 M nitrilotriacetic acid, 0.59 M MgSO4·7H2O, 0.91 M CaCl2·2H2O, and 0.04 M FeSO4·7H2O (Zeigler, 2001). A first (20 ml mLB medium) pre-culture was grown for 24 h and a second (20 ml mLB medium) pre-culture was inoculated to an absorbance (OD600) of 0.1 from the first pre-culture and incubated for 4 h. Both pre-cultures were grown aerobically at 60°C and 120 rpm (Infors Thermotron, Infors AG, Bottmingen, Switzerland) in 20 ml mLB. Serum bottles (Glasgerätebau Ochs, Bovenden, Germany), closed with gas-tight butyl rubber stoppers and secured with an aluminum seal were used (Carl Roth + Co. KG, Karlsruhe, Germany).
Clostridium ljungdahlii DSM 13528T was pre-cultured in modified GA-based medium (Groher and Weuster-Botz, 2016) containing (g/l): 2-(N-morpholino) ethansulfonic acid (MES) (20), / NH4Cl (1), KCl (0.3), KH2PO4 (0.23), MgSO4·7H2O (0.5), NaCl (2.25), yeast extract (2), CaCl2·2H2O (0.15), and resazurin (0.001). The pH of the medium was adjusted to 6.0 with KOH, and distributed in serum bottles. These were then closed with gas-tight butyl rubber stoppers and secured with an aluminum seal, and anaerobized. The anaerobization process was performed as follows: a needle, which was connected to a vacuum/gas line, was inserted through the septum; then, vacuum was applied to a final pressure of 10 psi (absolute), holding for 40 s, followed by pressurizing the bottles to 30 psi (absolute) using a gas mixture containing 20 vol-% carbon dioxide in nitrogen (Air Liquide, France). Following this, vacuum was applied again, and the whole process was repeated for 20 cycles. The bottles were then autoclaved closed. After autoclaving, the following solutions were added to the bottles (g/L): cysteine HCl·H2O (1), fructose (10); 1 ml/l of trace element solution (mg/L): FeSO4·7H2O (4000), Na2SeO3·5H2O (3), Na2WO4·2H2O (4), FeCl2·4H2O (3000), ZnCl2 (140), MnCl2·4H2O (200), H3BO3 (12), CoCl2·6H2O (380), CuCl2·2H2O (4), NiCl2·6H2O (48), Na2MoO4·2H2O (72), and 10 ml/l of vitamin solution (mg/L): biotin (4), folic acid (4), pyridoxine (20), thiamine-HCl·2H2O (10), riboflavin (10), nicotinic acid (10), calcium pantothenate (10), cobalamin (0.2), 4-aminobenzoic acid (10), and lipoic acid (10). To ensure sterility and anaerobic conditions, all additions to the autoclaved bottles were done using sterile syringes and needles, and piercing through the septum. All stock solutions were prepared and anaerobized as described above.
For pre-cultivation of C. ljungdahlii a glycerol stock (1 ml) was transferred anaerobically to a serum bottle containing 50 ml of anaerobic, sterile GA medium (prepared as above) and incubated for 48 h. A total of 5 ml of the latter culture was transferred anaerobically to 50 ml of fresh GA medium and cultivated at 37°C and 120 rpm for 24 h. The latter step was repeated to generate the inoculum for the sequential culture. The glycerol stocks were prepared in sterile, anaerobic hungate-type tubes which were closed with gas-tight rubber septa and secured with a perforated screw cap. In order to anaerobically transfer the glycerol stock and to inoculate the following cultures, sterile syringes and needles were used. The withdrawal of the liquid and its addition to the following serum bottle was performed by piercing through the septum.
Experimental Set Up
Stoppered serum flasks (250 ml), gas-tight and prepared as above, containing 50 ml of modified Luria Bertani (mLB) medium and with an initial gas atmosphere of CO and air (50:50 ratio) were inoculated with 1 ml of second pre-culture of P. thermoglucosidasius and cultivated for 70 h at 60°C and 120 rpm. Subsequently, 5 ml of the C. ljungdahlii pre-culture (OD600 = 2.5) was added to the P. thermoglucosidasius culture. Immediately before inoculating with C. ljungdahlii, 50 μl of GA trace elements, to the same final concentration as the GA medium, were added to each bottle to ensure that all elements necessary for the growth of C. ljungdahlii were present. Incubation of the P. thermoglucosidasius/C. ljungdahlii cultures were performed at 37°C and 120 rpm. The experiments were performed in quadruplicate for a duration of 240 h.
Analytical Methods
Growth was routinely monitored by taking 1 ml culture samples twice per day and performing absorbance (OD600) measurements using an Ultrospec 1100 pro spectrophotometer (Amersham Biosciences, USA). Acetate concentrations were similarly monitored using the Roche Yellow line enzymatic assay (Hoffmann- La Roche, Switzerland). To measure the gas composition in the bottles at each sampling point, a 5 ml gas sample was withdrawn with a syringe from the headspace of the bottle. The bottles were kept at the incubating temperature for the specific microorganism by means of a water bath. The sample was then immediately injected into a 300 Micro GC gas analyzer (Inficon, Bad Ragaz, Switzerland) with columns Molsieve and PLOT Q. Throughout the total analysis time of 180 s, the temperature was maintained constantly at 80°C.
Pressure was measured before and after sample taking using a manometer (GDH 14 AN, Greisinger electronic, Regenstauf, Germany). Gas composition was calculated using the ideal gas law as previously described (Mohr et al., 2018a). The acetate yield was calculated based on Bengelsdorf et al. (2013).
Results
Pre-culturing With P. thermoglucosidasius Supports the Anaerobic Growth of C. ljungdahlii
In the first phase of the sequential fermentation P. thermoglucosidasius was grown in 50 ml modified Luria Bertani (mLB) medium with an initial gas atmosphere of CO and air (50:50) (Figure 2). After 70 h, when all O2 was consumed, the culture reached an absorbance (OD600) of 0.732 ± 0.027 and pH of 6.21 ± 0.04 (Figure 2A). Previously we have observed that when the O2 is consumed, the growth of P. thermoglucosidasius also plateaus (Mohr et al., 2018a,b). To ensure that the increase of OD600 and acetate during the second phase is not due to P. thermoglucosidasius on its own, a control experiment without the addition of C. ljungdahlii was conducted (Figure S1). When C. ljungdahlii was added to the P. thermoglucosidasius culture 70 h after the first phase, the P. thermoglucosidasius/C. ljungdahlii sequential culture reached a maximum absorbance of 1.316 ± 0.157 ~23 h after the latter culture was added (Figure 2A). This indicates that the strict anaerobe C. ljungdahlii is able to grow in the medium after P. thermoglucosidasius exhausts the O2 from the gas atmosphere. The medium pH dropped drastically once C. ljungdahlii was added, from a pH of 6.20 ± 0.04 pre-addition to a pH of 5.61 ± 0.05 post-addition of the latter strain (Figure 2A). However, the pH continued to decline throughout the experiment, which can be correlated to active metabolism and acetate production by C. ljungdahlii.
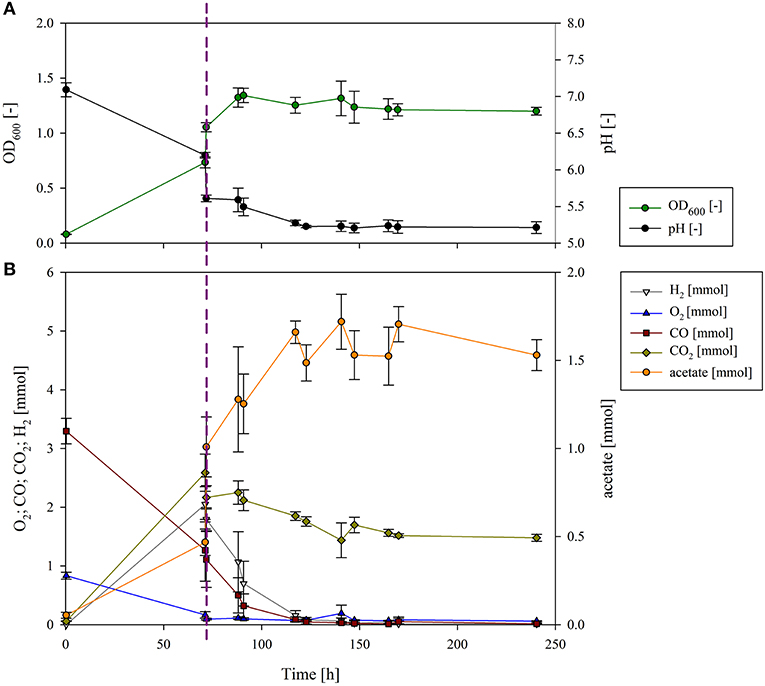
Figure 2. Growth and pH (A) and gas composition and acetate production (B) of the sequential cultivation of P. thermoglucosidasius and C. ljungdahlii. The dotted line presents the inoculation of C. ljungdahlii. (A) The measured OD600 (dark green) increased after 70 h, and at the same time the pH (black) decreased due to the inoculation with C. ljungdahlii. Growth continued until 93 h (23 h after inoculation with the second organism), and then it plateaued. As a result of the metabolic activity, the culture broth was acidified to a pH of 5.2. (B) O2 (blue) had already been consumed before the second phase, but some CO (dark red) was still left. After inoculation with C. ljungdahlii, CO2 (olive), and H2 (gray) did not accumulate any further, since they were used as building blocks by C. ljungdahlii to produce acetate (orange).
Sequential Cultivation With P. thermoglucosidasius and C. ljungdahlii Facilitates Acetate Production
In the post-aerobic phase P. thermoglucosidasius consumed 2.050 ± 0.117 mmol of CO, while 2.055 ± 0.023 and 2.646 ± 0.147 mmol of H2 and CO2 were produced via the WGS, respectively. Here, an equimolar conversion of CO to H2 was achieved. Subsequently, both H2 and CO decreased rapidly, being exhausted ~83 h after C. ljungdahlii was added. Similarly, CO2 decreased, although 1.479 ± 0.058 mmol CO2 were left at the end of the cultivation (after 240 h), due to the fact that 2 moles of H2 are needed per mol of CO2 as per the stoichiometry of the W-L pathway: 2 CO2 + 4 H2 → CH3COOH + 2 H2O (Ragsdale, 2008).
The decrease in the amount of these three gasses correlated with an increase in the amount of acetate. Some acetate (0.47 ± 0.07 mmol) was already observed during the first phase. This may be linked to mixed acid fermentation by P. thermoglucosidasius (Hussein et al., 2015). However, when P. thermoglucosidasius was cultivated on its own, no further increase in acetate was observed (Figure S1). The addition of C. ljungdahlii resulted in a spike in acetate (1.01 ± 0.17 mmol—an increase of 0.54 ± 0.22 mmol). This is associated with acetate production by C. ljungdahlii in the pre-culture in GA medium containing fructose as carbon source (Tirado-Acevedo et al., 2011). To shorten the time of inoculation of C. ljungdahlii, a washing step was not performed to avoid any potential lag phase due to stressing of the cells. Nevertheless, the amount of acetate increased concomitantly with H2, CO and CO2 consumption during the second phase, reaching a final amount of 1.53 ± 0.09 mmol of acetate. The acetate produced exclusively by C. ljungdahlii was, therefore, 0.52 mmol. This suggests that in the absence of additional exogenous carbon sources C. ljungdahlii could successfully use the H2 and CO2 produced by P. thermoglucosidasius as building blocks for acetate via the W-L pathway.
From the WLP, the theoretical maximum yield is 0.25 mol acetate/mol CO (Bengelsdorf et al., 2013). Considering the initial amount of CO in the bottles, 3.3 ± 0.216 mmol in average, a total theoretical maximum of 0.8 mmol of acetate could have been produced. The yield of acetate in the C. ljungdahlii phase in this study was 0.16 mol acetate/mol CO, achieving a 63% of the theoretical maximum.
Discussion and Conclusion
Microbial conversion of syngas into value-added chemicals may provide a sustainable and cost-effective alternative to current industrial strategies. However, most known syngas fermenters are strict anaerobes, which impacts the use of syngas sources which contain even low concentrations of O2. Besides, very few acetogens have been shown to tolerate only trace amounts of O2 (Karnholz et al., 2002; Takors et al., 2018). As such, expensive and often environmentally unfriendly O2 removal steps are necessary to facilitate effective syngas bioconversion (Heijstra et al., 2017). Here we have demonstrated that the facultative anaerobe P. thermoglucosidasius provides a biological means for the removal of toxic concentrations of O2, which allowed for the subsequent growth of the strict anaerobe C. ljungdahlii. Moreover, the production of H2 and CO2 by P. thermoglucosidasius via the WGS reaction provides the building blocks for the synthesis of acetate by C. ljungdahlii via the W-L pathway.
The utilization of a thermophile in the first phase of this process presents some additional advantages in that hot flue gasses resulting from industrial processes will not need to be cooled down to such a great extent. Most pertinently, the consumption of CO enables a near stoichiometric conversion of CO to H2 and CO2, without CO being lost in biomass formation (Mohr et al., 2018a) unlike in other CO-oxidizing organism, where CO is also used for biomass formation (O2 + 2.19 CO → 1.83 CO2 + 0.36 cell carbon) (Ragsdale, 2004). In this study the consumed CO during the first phase was completely converted to H2 and CO2 by P. thermoglucosidasius without CO being used for acetate production or growth. Hence, more substrate for the acetogenesis is available. With the sequential cultivation, a total amount of 1 mmol of acetate was produced. From this, the amount of acetate derived from the initial CO amounted to 0.52 mmol, which represents a 63% of the maximum theoretical yield. The overall yield of the established sequential culture is thus higher than by using other CO metabolizing organisms (King and Weber, 2007).
The sequential fermentation system presented here may thus serve as the basis for establishing as a cost-effective and environmentally friendly methodology for the production of value-added chemicals where it circumvents some of the pitfalls of working with strict anaerobic syngas fermenters while simultaneously linking the fermentative pathways of different taxa for the production of value-added chemicals by a second organism (Figure 1) (Takors et al., 2018). Future research will optimize the set-up and evaluate the application of this sequential fermentation with P. thermoglucosidasius and other mesophilic and thermophilic anaerobic bacteria for the production of a wide variety of bulk chemicals.
Data Availability Statement
The datasets generated for this study are available on request to the corresponding author.
Author Contributions
TM and AI planned the experiments, collected and analyzed the experimental data. LB conducted his Bachelor thesis under the supervision of TM and AI. AN and PM substantially contributed to the conception and design of the experiments. TM, AI, and PM drafted the manuscript. All authors read and approved the final version of the manuscript.
Funding
TM was supported by the German Federal Ministry of Education and Research (grant #031B0180). AI was supported by the German Federal Ministry of Education and Research and the Helmholtz Association of German Research Centers. PM was funded by the National Research Foundation of South Africa (grant #109137).
Conflict of Interest
The authors declare that the research was conducted in the absence of any commercial or financial relationships that could be construed as a potential conflict of interest.
Acknowledgments
We acknowledge support by Deutsche Forschungsgemeinschaft and Open Access Publishing Fund of Karlsruhe Institute of Technology.
Supplementary Material
The Supplementary Material for this article can be found online at: https://www.frontiersin.org/articles/10.3389/fbioe.2019.00433/full#supplementary-material
References
Barnard, D., Casanueva, A., Tuffin, M., and Cowan, D. (2010). Extremophiles in biofuel synthesis. Environ. Technol. 31, 871–888. doi: 10.1080/09593331003710236
Becker, A., Fritz-Wolf, K., Kabsch, W., Knappe, J., Schultz, S., and Volker Wagner, A. F. (1999). Structure and mechanism of the glycyl radical enzyme pyruvate formate-lyase. Nat. Struct. Biol. 6, 969–975. doi: 10.1038/13341
Bengelsdorf, F. R., Straub, M., and Dürre, P. (2013). Bacterial synthesis gas (syngas) fermentation. Environ. Technol. 34, 1639–1651. doi: 10.1080/09593330.2013.827747
Bock, A. K., Kunow, J., Glasemacher, J., and Schönheit, P. (1996). Catalytic properties, molecular composition and sequence alignments of pyruvate:ferredoxin oxidoreductase from the methanogenic archaeon Methanosarcina barkeri (strain Fusaro). Eur. J. Biochem. 237, 35–44. doi: 10.1111/j.1432-1033.1996.0035n.x
Brown, D. M., Upcroft, J. A., Edwards, M. R., and Upcroft, P. (1998). Anaerobic bacterial metabolism in the ancient eukaryote Giardia duodenalis. Int. J. Parasitol. 28, 149–164. doi: 10.1016/s0020-7519(97)00172-0
Daniell, J., Köpke, M., and Simpson, S. D. (2012). Commercial biomass syngas fermentation. Energies 5, 5372–5417. doi: 10.3390/en5125372
Diekert, G., and Wohlfarth, G. (1994). Metabolism of homoacetogens. Antonie Van Leeuwenhoek 66, 209–221. doi: 10.1007/BF00871640
Drake, H. L., Küsel, K., and Matthies, C. (2006). “Acetogenic prokaryotes,” in The Prokaryotes, Vol. 2 (Ecophysiology and Biochemistry), eds. M. Dworkin, S. Falkow, E. Rosenberg, K.-H. Schleifer, and E. Stackebrandt (New York, NY: Springer New York), 354–420. doi: 10.1007/0-387-30742-7_13
Groher, A., and Weuster-Botz, D. (2016). Comparative reaction engineering analysis of different acetogenic bacteria for gas fermentation. J. Biotechnol. 228, 82–94. doi: 10.1016/j.jbiotec.2016.04.032
Hatti-Kaul, R., Törnvall, U., Gustafsson, L., and Börjesson, P. (2007). Industrial biotechnology for the production of bio-based chemicals–a cradle-to-grave perspective. Trends Biotechnol. 25, 119–124. doi: 10.1016/j.tibtech.2007.01.001
Heijstra, B. D., Leang, C., and Juminaga, A. (2017). Gas fermentation: cellular engineering possibilities and scale up. Microb. Cell Fact. 16:60. doi: 10.1186/s12934-017-0676-y
Hussein, A. H., Lisowska, B. K., and Leak, D. J. (2015). The genus Geobacillus and their biotechnological potential. Adv. Appl. Microbiol. 92, 1–48. doi: 10.1016/bs.aambs.2015.03.001
Karnholz, A., Küsel, K., Gößner, A., Schramm, A., and Drake, H. L. (2002). Tolerance and metabolic response of acetogenic bacteria toward oxygen. Appl. Environ. Microbiol. 68, 1005–1009. doi: 10.1128/aem.68.2.1005-1009.2002
King, G. M., and Weber, C. F. (2007). Distribution, diversity and ecology of aerobic CO-oxidizing bacteria. Nat. Rev. Microbiol. 5, 107–118. doi: 10.1038/nrmicro1595
Köpke, M., and Dürre, P. (2011). Biochemical production of biobutanol. Handb. biofuels Prod. Process. Technol. 2011, 221–257. doi: 10.1533/9780857090492.2.199
Köpke, M., Held, C., Hujer, S., Liesegang, H., Wiezer, A., Wollherr, A., et al. (2010). Clostridium ljungdahlii represents a microbial production platform based on syngas. Proc. Natl. Acad. Sci. U.S.A. 107, 13087–13092. doi: 10.1073/PNAS.1004716107
Liew, F. M., Martin, M. E., Tappel, R. C., Heijstra, B. D., Mihalcea, C., and Köpke, M. (2016). Gas fermentation–a flexible platform for commercial scale production of low carbon fuels and chemicals from waste and renewable feedstocks. Front. Microbiol. 7:694. doi: 10.3389/fmicb.2016.00694
Liou, J. S. C., Balkwill, D. L., Drake, G. R., and Tanner, R. S. (2005). Clostridium carboxidivorans sp. nov., a solvent-producing clostridium isolated from an agricultural settling lagoon, and reclassification of the acetogen Clostridium scatologenes strain SL1 as Clostridium drakei sp. nov. Int. J. Syst. Evol. Microbiol. 55, 2085–2091. doi: 10.1099/ijs.0.63482-0
Meinecke, B., Bertram, J., and Gottschalk, G. (1989). Purification and characterization of the pyruvate-ferredoxin oxidoreductase from Clostridium acetobutylicum. Arch. Microbiol. 152, 244–250. doi: 10.1007/BF00409658
Mohr, T., Aliyu, H., Küchlin, R., Polliack, S., Zwick, M., Neumann, A., et al. (2018a). CO-dependent hydrogen production by the facultative anaerobe Parageobacillus thermoglucosidasius. Microb. Cell Fact. 17:108. doi: 10.1186/s12934-018-0954-3
Mohr, T., Aliyu, H., Küchlin, R., Zwick, M., Cowan, D., Neumann, A., et al. (2018b). Comparative genomic analysis of Parageobacillus thermoglucosidasius strains with distinct hydrogenogenic capacities. BMC Genomics 19:880. doi: 10.1186/s12864-018-5302-9
Nakayama, T., Yonekura, S. I., Yonei, S., and Zhang-Akiyama, Q. M. (2013). Escherichia coli pyruvate: flavodoxin oxidoreductase, YdbK–regulation of expression and biological roles in protection against oxidative stress. Genes Genet. Syst. 88, 175–188. doi: 10.1266/ggs.88.175
Ragsdale, S. W. (2004). Life with carbon monoxide. Crit. Rev. Biochem. Mol. Biol. 39, 165–195. doi: 10.1080/10409230490496577
Ragsdale, S. W. (2008). Enzymology of the Wood-Ljungdahl pathway of acetogenesis. Ann. N. Y. Acad. Sci. 1125, 129–136. doi: 10.1196/annals.1419.015
Ragsdale, S. W., and Wood, H. G. (1991). Enzymology of the acetyl-coA pathway of CO2 fixation. Crit. Rev. Biochem. Mol. Biol. 26, 261–300. doi: 10.3109/10409239109114070
Shen, Y., Brown, R. C., and Wen, Z. (2017). Syngas fermentation by Clostridium carboxidivorans P7 in a horizontal rotating packed bed biofilm reactor with enhanced ethanol production. Appl. Energy 187, 585–594. doi: 10.1016/j.apenergy.2016.11.084
Takors, R., Kopf, M., Mampel, J., Bluemke, W., Blombach, B., Eikmanns, B., et al. (2018). Using gas mixtures of CO, CO2 and H2 as microbial substrates: the do's and don'ts of successful technology transfer from laboratory to production scale. Microb. Biotechnol. 11, 606–625. doi: 10.1111/1751-7915.13270
Tanner, R. S., Miller, L. M., and Yang, D. (1993). Clostridium ljungdahlii sp. nov., an acetogenic species in clostridial rRNA homology group I. Int. J. Syst. Bacteriol. 43, 232–236. doi: 10.1099/00207713-43-2-232
Teixeira, L. V., Moutinho, L. F., and Romão-Dumaresq, A. S. (2018). Gas fermentation of C1 feedstocks : commercialization status and future prospects. Biofuels Bioprod. Biorefining 12, 1103–1117. doi: 10.1002/bbb.1912
Tirado-Acevedo, O., Cotter, J. L., Chinn, M. S., and Grunden, A. M. (2011). Influence of carbon source pre-adaptation on Clostridium ljungdahlii growth and product formation. J. Bioprocess. Biotech. 1, 1–5. doi: 10.4172/2155-9821.s2-001
Yang, J., Naik, S. G., Ortillo, D. O., García-Serres, R., Li, M., Broderick, W. E., et al. (2009). The iron-sulfur cluster of pyruvate formate-lyase activating enzyme in whole cells: cluster interconversion and a valence-localized [4Fe-4S]2+ state. Biochemistry 48, 9234–9241. doi: 10.1021/bi9010286
Zeigler, D. R. (2001). “Volume 3: The Genus Geobacillus,” in Bacillus Genetic Stock Center Catalog of Strains (Columbus, OH: The Bacillus Genetic Stock Center). Available online at: http://www.bgsc.org/catalogs.php (accessed August 29, 2019).
Keywords: Parageobacillus thermoglucosidasius, Clostridium ljungdahlii, water-gas shift reaction, anaerobic acetate production, Wood-Ljungdahl pathway, syngas
Citation: Mohr T, Infantes A, Biebinger L, de Maayer P and Neumann A (2019) Acetogenic Fermentation From Oxygen Containing Waste Gas. Front. Bioeng. Biotechnol. 7:433. doi: 10.3389/fbioe.2019.00433
Received: 17 September 2019; Accepted: 05 December 2019;
Published: 20 December 2019.
Edited by:
Fu-Li Li, Qingdao Institute of Bioenergy and Bioprocess Technology (CAS), ChinaReviewed by:
Neng-Zhong Xie, Guangxi Academy of Sciences, ChinaRalph Tanner, University of Oklahoma, United States
Copyright © 2019 Mohr, Infantes, Biebinger, de Maayer and Neumann. This is an open-access article distributed under the terms of the Creative Commons Attribution License (CC BY). The use, distribution or reproduction in other forums is permitted, provided the original author(s) and the copyright owner(s) are credited and that the original publication in this journal is cited, in accordance with accepted academic practice. No use, distribution or reproduction is permitted which does not comply with these terms.
*Correspondence: Alba Infantes, YWxiYS5pbmZhbnRlc0BraXQuZWR1; Anke Neumann, YW5rZS5uZXVtYW5uQGtpdC5lZHU=
†These authors have contributed equally to this work and share first authorship