- 1Faculty of Science, Institute of Biological Sciences, University of Malaya, Kuala Lumpur, Malaysia
- 2Department of Plant and Environmental Sciences, University of Copenhagen, Frederiksberg, Denmark
- 3Department of Biotechnology and Biomedicine, Technical University of Denmark, Kongens Lyngby, Denmark
- 4Laboratory of Plant Physiology, Wageningen University, Wageningen, Netherlands
- 5Plant Hormone Biology Lab, Swammerdam Institute for Life Sciences, University of Amsterdam, Amsterdam, Netherlands
Malaria is a real and constant danger to nearly half of the world’s population of 7.4 billion people. In 2015, 212 million cases were reported along with 429,000 estimated deaths. The World Health Organization recommends artemisinin-based combinatorial therapies, and the artemisinin for this purpose is mainly isolated from the plant Artemisia annua. However, the plant supply of artemisinin is irregular, leading to fluctuation in prices. Here, we report the development of a simple, sustainable, and scalable production platform of artemisinin. The five genes involved in artemisinin biosynthesis were engineered into the moss Physcomitrella patens via direct in vivo assembly of multiple DNA fragments. In vivo biosynthesis of artemisinin was obtained without further modifications. A high initial production of 0.21 mg/g dry weight artemisinin was observed after only 3 days of cultivation. Our study shows that P. patens can be a sustainable and efficient production platform of artemisinin that without further modifications allow for industrial-scale production. A stable supply of artemisinin will lower the price of artemisinin-based treatments, hence become more affordable to the lower income communities most affected by malaria; an important step toward containment of this deadly disease threatening millions every year.
Introduction
Physcomitrella patens is a non-vascular plant that has been well established as a model organism to be used in basic research and in applied biotechnology (Simonsen et al., 2009; Buttner-Mainik et al., 2011; Ikram et al., 2015; Reski et al., 2015). The genome is fully sequenced and the haploid life cycle and efficient homologous recombination makes P. patens an attractive industrial production system compared to other plant hosts (Schaefer and Zrÿd, 1997; Reski, 1998). Additionally, a novel transformation technology involving in vivo assembly of multiple DNA fragments in P. patens has been established, further increasing the potential as a photosynthetic chassis for synthetic biology (King et al., 2016). Currently, several recombinant pharmaceutical proteins and molecules of commercial value are being produced in this system (Anterola et al., 2009; Zhan et al., 2014; Pan et al., 2015; Reski et al., 2015).
Artemisinin originates from the plant Artemisinia annua and is the first-choice treatment for malaria (Novotny et al., 1966). Chemically, artemisinin is a sesquiterpene lactone bearing a unique endoperoxide structure and its complex structure makes it difficult and not economically feasible to be chemically synthesized (Pandey and Pandey-Rai, 2016). Several efforts have been made to obtain artemisinin from a stable source, such as yeast (Ro et al., 2006), where a production of artemisinic acid followed by a three-step chemical synthesis to artemisinin was established (Paddon and Keasling, 2014). It was previously shown that artemisinin could be mass-produced by cultivation of A. annua or semi-synthetically in microorganisms producing artemisinic acid (Paddon and Keasling, 2014). Besides these efforts, extensive bioengineering of modified Nicotiana plants (Malhotra et al., 2016; Wang et al., 2016) has provided limited production of artemisinin. However, these have not yet been used for large-scale production. Currently, there are no reports on the stable heterologous biosynthesis of artemisinin in photosynthetic organisms that can be grown in bioreactors.
In order to produce artemisinin in P. patens, we introduced the five genes responsible for the biosynthesis of dihydroartemisinic acid (Figure 1), where amorpha-4,11-diene synthase, ADS (Komatsu et al., 2010) catalyzes the first step. This was followed by the second step, the cytochrome P450, CYP71AV1 (Ro et al., 2006) which we linked with the third step, the alcohol dehydrogenase 1 (ADH1) (Paddon et al., 2013) by the hybrid LP4/2A peptide linker (François et al., 2004). Finally, we introduced the fourth step catalyzed by double-bond reductase 2 (DBR2) (Zhang et al., 2008) and the fifth step, being the aldehyde dehydrogenase 1 (ALDH1) (Teoh et al., 2009). These five genes would yield dihydroartemisinic acid. The final conversion of dihydroartemisinic acid into artemisinin is thought to occur by photooxidation (Sy and Brown, 2002), thus the enzymatic end product is dihydroartemisinic acid. The five genes were transformed into P. patens using in vivo homologous recombination that allows multiple DNA fragments to be transformed at once into the genome (King et al., 2016). Here, we show that engineered P. patens can produce significant levels of artemisinin. This could become a sustainable and efficient production platform of artemisinin, which could potentially help to stabilize the supply of artemisinin and aid in containing malaria.
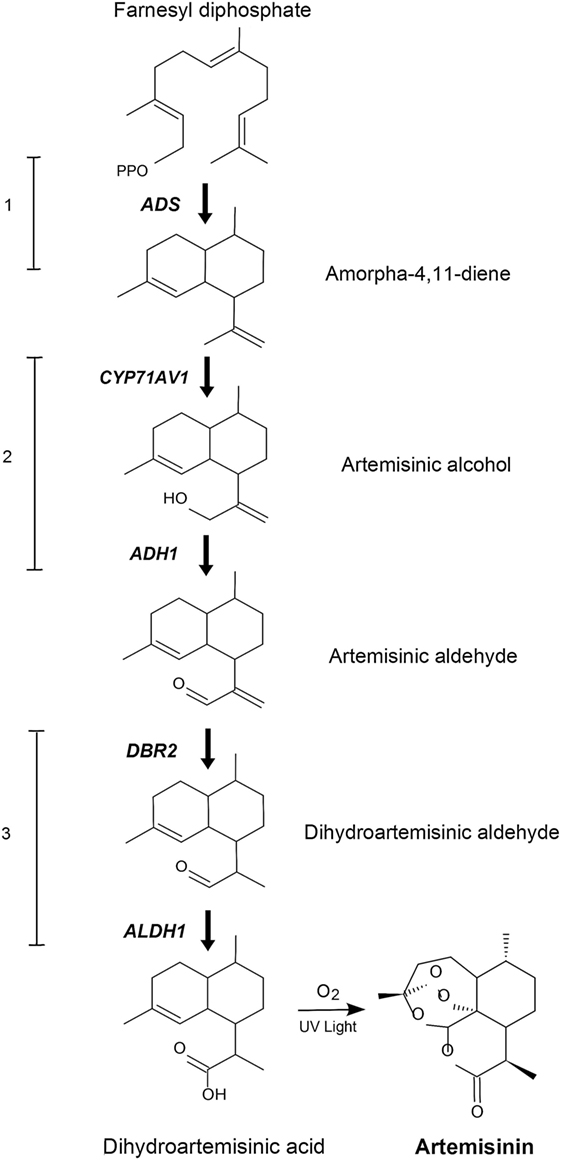
Figure 1. Schematic representation of the engineered artemisinin biosynthetic pathway in Physcomitrella patens. The five genes that encode the dihydroartemisinic acid biosynthetic pathway are ADS, amorphadiene synthase; CYP71AV1, amorphadiene oxidase; ADH1, alcohol dehydrogenase 1; DBR2, artemisinic aldehyde double-bond reductase; ALDH1, aldehyde dehydrogenase 1. The numbers 1–3 indicates transformation sets.
Materials and Methods
P. patens Material and Growth Conditions
Physcomitrella patens (Gransden ecotype, International Moss Stock Center #40001) was grown on solid and liquid PhyB media under sterile conditions, at 25°C with continuous 20–50 W/m2 light intensity. For PhyB media mix 800 mg Ca(NO3)2, 250 mg MgSO4⋅7H2O, 12.5 mg FeSO4⋅7H20, 0.5 g (NH4)2C4H4O, 10 mL KH2PO4 buffer (25 g KH2PO4 per liter and adjusted to pH 6.5 with 4 M KOH), and 0.25 mL trace element solution (110 mg CuSO4⋅5H20, 110 mg ZnSO4⋅7H2O, 1,228 mg H3BO3, 778 mg MnCl2⋅4H2O, 110 mg CoCl2⋅6H2O, 53 mg KI, 50 mg Na2MoO4⋅2H2O per liter). The medium can be solidified with 0.7% (w/v) agar and is sterilized by autoclaving at 121°C (Bach et al., 2014).
Transformation of P. patens
A detailed description of P. patens transformation was previously published (Bach et al., 2014). Five days cultured P. patens with approximately 1.5 g (fresh weight) was digested by adding 1 mL of 0.5% DriselaseR enzyme solution in 8.5% mannitol (Sigma D9515) for every 40 mg of P. patens tissue. The tissue was incubated at room temperature with occasional gentle shaking for 30–60 min before filtering through a 100-µm pored mesh-filter. The filtrate was collected by centrifugation at 150–200 × g for 4 min with slow breaking. The pellet was washed twice with protoplast wash solution (8.5% mannitol, 10 mM CaCl2). Protoplast density was measured using a hemocytometer, and resuspended in MMM solution (9.1% d-mannitol, 10% MES, and 15 mM MgCl2) at a concentration of 1.6 × 106 protoplasts/mL. 300 µL of the protoplast suspension and 300 µL of PEG solution were added to a 15-mL tube containing 10 µg total DNA followed by incubation in a water bath for 5 min at 45°C and another 5 min at room temperature. 300 µL of 8.5% d-mannitol were added five times followed by another five times dilutions with 1 mL of 8.5% d-mannitol. Transformed protoplasts were pelleted by centrifugation and resuspended in 500 µL of 8.5% d-mannitol and 2.5 mL of protoplast regeneration media (top layer; PRMT). 1 mL of the mixture was dispensed on three plates containing protoplast regeneration media (bottom layer; PRMB) overlaid with cellophane. The plates were incubated in continuous light for 5–7 days at 25°C. The cellophane and regenerating protoplasts were then transferred to PhyB media containing the appropriate selection marker for 2 weeks, before transferring on PhyB media without antibiotics for another 2 weeks. This process was repeated twice, after which the stable transformants was kept on PhyB media with biweekly subcultivation including blending in sterile water until further use.
DNA Fragments and Genes
The Pp108 locus homologous recombination flanking regions were amplified from P. patens genomic DNA. The ADS gene was a kind gift from Assoc. Prof. Dae Kyun Ro, University of Calgary, Canada. The synthetic genes CYP71AV1 (DQ268763), ADH1 (JF910157.1), DBR2 (EU704257.1), and ALDH1 (FJ809784.1) were codon-optimized according to the P. patens codon usage by GenScript, USA. The Ubiquitin promoter and Ubiquitin terminator from Arabidopsis thaliana (CP002686.1) synthetic genes were also purchased from GenScript. The Maize Ubiquitin 1 promoter and G418 selection cassettes were obtained from the pMP1355 vector, a kind gift from Prof. Mark Estelle, University of California San Diego, USA. The rice actin promoter and hygromycin selection cassette were obtained from the pZAG1 vector, a kind gift from Assoc. Prof. Yuji Hiwatashi from Miyagi University, Japan.
PCR, DNA Purification and Concentration
The DNA fragments were amplified using PhusionR High-Fidelity DNA Polymerase (New England Biolabs). The primers used are listed in Table S1 in Supplementary Material. PCR conditions and annealing temperatures were modified depending on primers and templates used in the reaction. PCR reactions using plasmid DNA as template were digested with DpnI (NEB, USA) for 1 h at 37°C followed by inactivation at 65°C for 20 min to lower background after transformation. PCR products were purified using QIAquick PCR Purification Kit (QIAGEN GmbH, Germany). The DNA fragments for transformations were concentrated via ethanol precipitation to a final concentration of ~1 μg/μL, determined using NanoDrop2000 (Thermo Fisher Scientific).
GC–MS Analysis of Amorpha-4,11-Diene
Amorpha-4,11-diene production was measured using a Shimadzu GCMS-QP2010 Plus (GC-2010). The initial screening was performed by HS-SPME (Headspace-Solid Phase Micro-Extraction) (Drew et al., 2012; Andersen et al., 2015). Quantification of amorpha-4,11-diene was performed according to a published protocol (Rodriguez et al., 2014). One-week-old P. patens was blended in sterile water using a Polytron (PT 1200 E, Kinematic AG) to a final concentration of 0.2 g/mL (fresh weight). Two milliliters of the blended P. patens were inoculated into 20 mL liquid PhyB media and cultivated on a shaker under standard conditions for 4 days. Then, 2 mL of decane were added into the P. patens culture and cultivation was continued for up to 2 weeks. After 2 weeks, 100 µL of decane was harvested and diluted twice in ethyl acetate spiked with an internal standard (trans-caryophyllene), and analyzed by GC–MS. 1 µL of the extract was injected in split mode and separated with HP-5MS UI column (20.0 m × 0.18 mm × 0.18 µm) with hydrogen as a carrier gas. The GC program was as follows, injection temperature of 250°C, oven temperatures of 60°C for 3 min and 60–320°C at 40°C/min. The amorpha-4,11-diene concentration was calculated based on the calibration curve of the internal standard run in parallel (Rodriguez et al., 2014).
P. patens Growth and Biomass Measurements
Physcomitrella patens lines were blended in sterile water and subcultivated onto PhyB. After 1 week, the P. patens was blended for 30 s in sterile water, normalized to a concentration of 0.2 g/mL (fresh weight) as previously described (Zhan et al., 2014; Pan et al., 2015). 2 mL of the blended P. patens was inoculated into 20 mL liquid PhyB media and cultivated on a horizontal shaker under standard conditions without aeration. The P. patens cultures were prepared in 3 replicates and harvested in 3, 6, 12, 15, and 18 days. The harvested P. patens tissue was dried overnight in an oven at 90°C and weighed.
Metabolite Extraction and UPLC-MRM-MS Analysis
Physcomitrella patens lines were blended in sterile water and subcultivated onto PhyB. After 1 week the fresh P. patens samples were harvested, snap-frozen, and ground into a fine powder. Samples of 3,000 mg were extracted with 3 mL citrate phosphate buffer, pH 5.4, followed by vortexing and sonication for 15 min. 1 mL Viscozyme (Sigma V2010) was added and samples were incubated at 37°C. The whole mixture was then extracted three times with 3 mL ethyl acetate, concentrated to a volume of 1 mL, and stored at −20°C. For liquid culture extracts, 500 mL of liquid culture was harvested, passed through a filter paper and extracted with 200 mL of ethyl acetate in a separation funnel. Ethyl acetate was concentrated to a volume of 1 mL and stored at −20°C. Ethyl acetate of both liquid culture and P. patens sample extracts were then dried under a flow of N2 and resuspended into 300 µL of 75% MeOH:H2O (V:V). Extracts were passed through a 0.45-µm membrane filter (Minisart® RC4, Sartorius, Germany) before analysis.
Artemisinin and artemisinin biosynthesis pathway intermediates were measured in a targeted approach by using a Waters Xevo tandem quadrupole mass spectrometer equipped with an electrospray ionization source and coupled to an Acuity UPLC system (Waters), essentially as described before (Ting et al., 2013). A BEH C18 column (100 mm × 2.1 mm × 1.7 µm; Waters) was used for chromatographic separation by applying a water:acetonitrile gradient. The gradient started with 5% (v/v) acetonitrile in water with formic acid [1:1,000 (v/v)] for 1.25 min, then raised to 50% in 2.35 min and further raised to 90% at 3.65 min. This was kept for 0.75 min before returning to the 5% acetonitrile/water (v/v) with formic acid [1:1,000 (v/v)] by using a 0.15-min gradient. The same solvent composition was used to equilibrate the column for 1.85 min. The flow rate was 0.5 mL/min, and the column temperature was maintained at 50°C. Injection volume was set to 10 µL. Desolvation and cone gas flow were set to 1,000 and 50 L/h, and the mass spectrometer was operated in positive ionization mode. Capillary voltage was set at 3.0 kV. Desolvation and source temperatures were set at 650 and 150°C, respectively. The cone voltage was optimized for all metabolites using the Waters IntelliStart MS Console. Fragmentation by collision-induced dissociation was done in the ScanWave collision cell using argon. Multiple Reaction Monitoring (MRM) was used for detection and quantification of artemisinin. MRM transitions for artemisinin and pathway intermediates measurement settings were optimized for MRM channels, which are presented in (Table S2 in Supplementary Material). Artemisinin and dihydroartemisinic acid were gifts from Dafra Pharma (Belgium). Other precursors were synthesized from dihydroartemisinic acid by Chiralix (Nijmegen, the Netherlands) and were then examined by NMR (>98% purity). External calibration curves were measured by using reference standards. The metabolite profiling was performed twice with 2 months apart, using the same original cell lines that had been subcultivated four times on PhyB media in between the analysis.
Analysis of Conjugated Artemisinin Biosynthesis Pathway Intermediates by LC-QTOF-MS
In order to analyze the putative conjugated forms of artemisinin biosynthesis pathway intermediates, P. patens lines were blended in sterile water and subcultivated onto PhyB. After 1 week, 100 mg of fresh P. patens was ground in liquid nitrogen and extracted with 300 µL MeOH:formic acid [1,000:1 (v/v)]. Samples were briefly vortexed and sonicated for 15 min, followed by 15 min centrifugation at 13,000 × g. Extracts were passed through a 0.45-µm membrane filter (Minisart® RC4, Sartorius, Germany) before analysis on a Water alliance 2795 HPLC connected to a QTOF Ultima V 4.00.00 mass spectrometer (Waters. MS technologies, UK). The mass spectrometer was operated in negative ionization mode. A precoloumn of 2.0 mm × 4 mm (Phenomenex, USA) was connected to the C18 analytical column (Luna 3 µm C18/2 100A; 2.0 mm × 150 mm; Phenomenex, USA). Degassed eluent A and B were HPLC-grade water:formic acid [1,000:1 (v/v)] and acetonitrile:formic acid [1,000:1 (v/v)], respectively. The flow rate was 19 mL/min. The HPLC gradient started from 5% eluent B and linearly increased to 75% in 45 min. after that the column was equilibrated for 15 min with 5% eluent B. 5 µL of each sample was used for injection.
P. patens Cell Components Isolation and Extraction
Physcomitrella patens protoplasts were isolated according to a previously published protocol (Bach et al., 2014). The P. patens lines were blended in sterile water and subcultivated onto PhyB. After 1 week, protoplast were isolated and pelleted by centrifugation at 150–200 × g for 4 min with slow breaking. Supernatant, which contained the apoplast (AP) was harvested and 10 mL of ultra-pure water was added to the pelleted protoplast to lyse the cells. Lysed cells were centrifuged at 6,000 × g for 10 min, and 7 mL of the supernatant was mixed with an equal amount of sucrose to a final concentration of 0.3 M. The mixture was loaded into an ultracentrifuge tube with a 2 mL over-lay of water and centrifuged at 135,000 × g for 40 min at room temperature using a swinging bucket rotor. The bottom layers containing the cytoplasm (CT) and the cell pellet (CP) was harvested. The top layer (1.5 mL) containing neutral lipid bodies (LBs) was transferred to another centrifuge tube, mixed with sucrose to a final concentration of 0.3 M, overlaid with 2 mL of water as before, and 1 mL of the top-most layer of the LBs after centrifugation at 135,000 x g for 40 min. The harvested samples; AP, CT, LBs, and CP was then extracted with 3 mL ethyl acetate and concentrated to a volume of 1 mL and stored at −20°C. The samples were then dried under a flow of N2 and resuspended into 300 µL of 75% MeOH:H2O (V:V). Extracts were passed through a 0.45-µm membrane filter (Minisart® RC4, Sartorius, Germany) before analysis.
Results and Discussions
Engineering of P. patens
We introduced ADS under the control of the strong constitutively expressed promoter, Ubiquitin1 from Zea mays with geneticin (G418) as resistance cassette. After two rounds of selection the ADS product amorpha-4,11-diene was detected in 11 lines with an average content of 200 mg/L, which is higher than in A. annua (Ma et al., 2009), Nicotiana tabacum (Wallaart et al., 2001), E. coli (Martin et al., 2003), and yeast (Ro et al., 2006). An encouraging initial result was achieved without further optimization of terpenoid biosynthesis. The best producing line was selected for further transformation with CYP71AV1 and ADH1. The CYP71AV1-LP4/2A-ADH1 construct was controlled by the rice actin promoter with hygromycin resistance cassette. The genome homologous overhang was targeted to remove the previously integrated G418 cassette during the recombination event. Of 47 transformed lines, 11 were chemo- and genotyped and one line was selected for transformation with the final genes, DBR2 and ALDH1. The DBR2-LP4/2A-ALDH1 construct was controlled by the Arabidopsis Ubiquitin promoter and the G418 selection cassette was used again. The hygromycin cassette was targeted for recombination to remove this selection marker. Three independent transformants were recovered, and genotyping showed that the five genes in the biosynthesis of dihydroartemisinic acid were integrated into the genome (Figure S1 in Supplementary Material). PCR analysis showed there was no untargeted integration for the selected lines. This showed that the selected P. patens lines have a uniform integration of the five genes and one copy of each.
Metabolite Profiling and Growth of Engineered Strains
Extracts of the transgenic P. patens lines containing all five genes were analyzed using ultra-high performance liquid chromatography coupled with a triple quadrupole mass spectrometer operated in MRM mode (MRM) (UPLC-MRM-MS). MRM traces identical with the artemisinin standard were detected in the P. patens extracts, but not in the liquid culture medium or in extracts of wild-type P. patens (WT) (Figure 2; Figure S2 in Supplementary Material). The analysis was performed twice with 2 months apart in triplicates each time. The presence of artemisinin, but no intermediates, in P. patens was confirmed by comparison with an artemisinin standard (Figure S2 in Supplementary Material). Quantification using external calibration showed that the artemisinin yield was 0.21 mg/g dry weight (DW), which is in the range of native A. annua (0.001–1.54 mg/g DW) (Bhakuni et al., 2001) and in cross-bred plants (up to 10 mg/g DW) and higher in genetically engineered (up to 30 mg/g DW) (Tang et al., 2014). The amount of artemisinin in our P. patens lines is higher than what was initially obtained via heterologous expression in N. tabacum (0.0068 mg/g DW) (Farhi et al., 2011) and in Nicotiana benthamiana (0.003 mg/g DW) (Wang et al., 2016). Recent efforts of targeting the biosynthesis to the chloroplast elevated the yield in N. tabacum to 0.8 mg/g DW (Malhotra et al., 2016), since glycosylation of the precursors was avoided. Glycosylation and glutathione conjugation was previously shown to influence the yield in N. benthamiana (Mukanganyama et al., 2001; van Herpen et al., 2010; Ting et al., 2013; Liu et al., 2014). We also explored the presence of sugar and glutathione conjugated products by liquid chromatography coupled to quadrupole time-of-flight mass spectrometry (LC-QTOF-MS). However, no glycosylated or glutathione conjugated products were detected in the culture medium nor in the P. patens extracts. The lack of glycosides may be explained by the fact that P. patens only has 20 glycosyltransferase 1 (GT1) genes that encode for enzymes involved in deactivation or detoxification of secondary metabolites, while higher vascular plants usually have hundreds (Yonekura-Sakakibara and Hanada, 2011). As no pathway intermediates (conjugated or not) were detected in our extracts, we conclude that the pathway operates efficiently in P. patens.
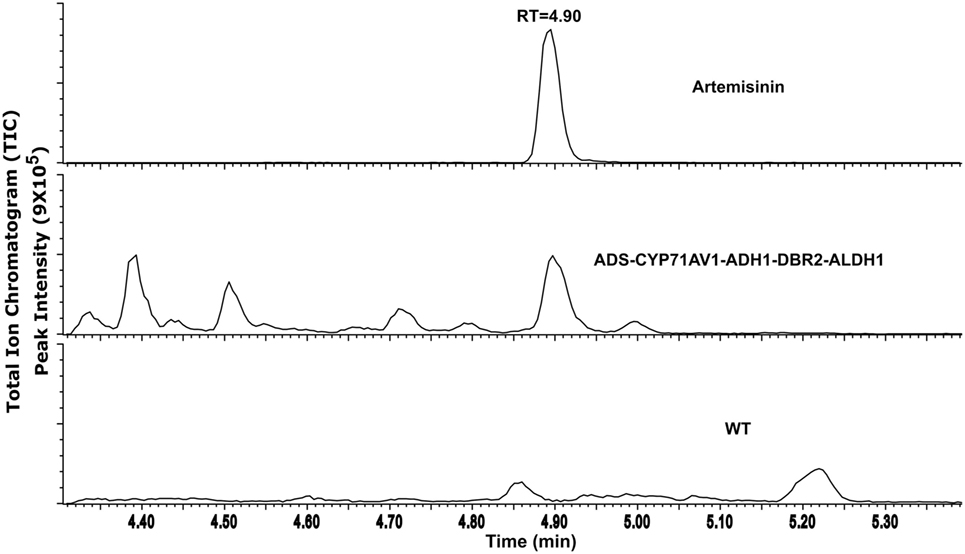
Figure 2. UPLC-MRM-MS analysis of artemisinin produced from transgenic Physcomitrella patens, an internal standard (artemisinin) and WT as control with retention time. TIC represents the sum of multiple reaction monitoring channels used for the detection of artemisinin: m/z 283.19 > 219.21; 283.19 > 247.19; and 283.19 > 265.22.
A slight reduction in growth rate was observed after day 12, resulting in 11% lower biomass in the transgenic line after 18 days (Figure 3A). This indicates that there is just a small disruption of other metabolic pathways responsible for P. patens growth and fitness. The effect of artemisinin biosynthesis on P. patens growth is less, but follows and suggests the same pattern as was previously observed (Simonsen et al., 2009; Zhan et al., 2014; Pan et al., 2015). The lesser growth is likely due to toxicity of the product and to depletion of nutrients in the media, but this requires further studies in semi-continuous cultures. The highest concentration (0.21 mg/g DW) of artemisinin was observed after 3 days of cultivation (Figure 3B), whereas the highest accumulative amount of artemisinin (2.5 mg artemisinin) was observed at day 12. This is mainly due to the increase in biomass. This show that the primary production of artemisinin in P. patens happens within the first 2 weeks after subcultivation. This rapid production of artemisinin is very valuable for future industrial production and suggests that a semi-continuous batch cultivation with weekly extraction and disruption of the cells can provide high amounts of artemisinin.

Figure 3. Growth and production of artemisinin in Physcomitrella patens. (A) Time course analysis of growth of transgenic and WT P. patens in liquid culture. D0 indicates the initial sub-culturing day. (B) Time course analysis of artemisinin production in P. patens. Error bars denote the SE of triplicate shake-flask liquid culture. (C) Production of artemisinin after 18 days of cultivation in different cellular component of AP, apoplast; CT, cytoplasm; LBs, lipid bodies; and CP, cell pellet.
Conversion of dihydroartemisinic acid to artemisinin in A. annua has been suggested to occur via photooxidation and induced by oxygen (Sy and Brown, 2002). In the present study, P. patens was grown under 24-h light and ambient air, which seems to facilitate the conversion to artemisinin as dihydroartemisinic acid was not detected in the extract. The reduction in artemisinin content from day 12 to day 18 might be due to chemical degradation of the compound, but no obvious breakdown products was found in the analysis; thus, this drop requires further studies.
Storage of Artemisinin in P. patens
Artemisinin biosynthesis occurs in the glandular trichomes of A. annua, but P. patens does not have trichomes. To identify the storage location of artemisinin in our transgenic P. patens, extracts of the AP, CT, LBs, and CPs were analyzed by UPLC-MRM-MS. Artemisinin was detected in all the extracts except for the CPs (Figure 3C). The highest accumulation was in the AP at 0.04 mg/g DW (after 18 days of cultivation, analyzed at 18 days to obtain enough biomass for the analysis) with 10- and 20-fold less in the CT and LBs. In the native plant A. annua, dihydroartemisinic acid is transported to the subcuticular space of the glandular trichomes before photooxidation into artemisinin (Brown, 2010). The high accumulation of artemisinin in the P. patens AP indicates a transport of pathway products over the cell membrane, as also shown N. benthamiana (Wang et al., 2016).
This work demonstrates a stable production of artemisinin in a photosynthetic organism that allows for large-scale industrial production. The production will not be affected by environmental and ecological variables and overall have a lower environmental impact than field production (water usages, fertilizers, petrol usages, etc.). It should be noted that besides using codon-optimized sequences, no other enhancement, e.g. increasing terpenoid precursor supply or multi copy gene integration was applied. The high flux through the terpenoid pathway is likely to be due to the metabolic robustness of P. patens (Schaefer and Zrÿd, 1997). In contrast, yeast needed extensive and complex precursor pathway engineering prior to the introduction of the artemisinic acid pathway genes. Further optimization of the metabolic network in P. patens has been shown to optimize sesquiterpenoid production (Zhan et al., 2014) and possibly also artemisinin production. Possible targets include overexpression of the key enzymes, 3-hydroxy-3-methylglutaryl-CoA reductase (HMGR), and farnesyl diphosphate synthase (FPS), which improved terpenoid production in other plants (van Herpen et al., 2010) and microbial (Ro et al., 2006; Paddon et al., 2013) hosts. Another possibility is targeting biosynthesis to different cellular compartments, which has also been shown to improve artemisinin and other sesquiterpenoid yield up to 1,000-fold in N. tabacum (Wu et al., 2006; Malhotra et al., 2016), which alone in P. patens would lead to a yield of 210 mg/g DW.
Optimization of artemisinin yield in P. patens can potentially result in a stable, sustainable, environmentally friendly, and commercially viable production platform. A considerable advantage of P. patens as an artemisinin production platform is that the extract only requires simple purification steps (Figure 2). This is different from the current yeast production platform that requires further chemical synthesis to yield artemisinin (Paddon et al., 2013; Turconi et al., 2014) and the production in N. tabacum was only described as semi-pure extracts (Malhotra et al., 2016). The use of P. patens could lead to a reduced price for artemisinin-based treatments, allowing lower income communities most affected by malaria, to contain malaria. Furthermore, scaling up the production of artemisinin in plant-based bioreactors would expand the use of plant cells in bioreactors.
Conclusion
All five artemisinin biosynthetic pathway genes were engineered into the moss P. patens. In vivo biosynthesis of artemisinin was obtained without further modifications and a high initial production of 0.21 mg/g DW artemisinin was observed after only 3 days of cultivation. This bioengineering achievement expands the frontiers of synthetic biotechnology, offering a genetically robust plant-based platform, which can be scaled up for industrial production of other complex high-value plant-based compounds. P. patens uses light as an energy source, thus is potentially more cost effective than other carbon supplemented biotechnological platforms.
Author Contributions
NI planned and performed the experiments, analyzed the data, and wrote the manuscript. ABK performed the UPLC-MRM-MS and LC-QTOF-MS, analyzed the data, and reviewed the manuscript. AP performed the P. patens cell components isolation experiments and reviewed the manuscript. ARvdK and HB reviewed the manuscript. HS planned the experiment and supervised and reviewed the manuscript.
Conflict of Interest Statement
All authors declare that they have no conflict of interest. HS is co-founder of Mosspiration Biotech IVS that aim to produce fragrances in P. patens, but not artemisinin.
Acknowledgments
NI was supported by a grant from the Ministry of Higher Education, Malaysia and the University of Malaya. AP and HS was supported by The Danish Council for Independent Research (#4005-00158B). The authors would like to thank Professor Mark Estelle, Assoc. Prof. Yuji Hiwatashi, and Assoc. Prof. Dae Kyun Ro for kindly providing the pMP1355, and PZAG1 vector, and the ADS template.
Supplementary Material
The Supplementary Material for this article can be found online at http://journal.frontiersin.org/article/10.3389/fbioe.2017.00047/full#supplementary-material.
References
Andersen, T. B., Cozzi, F., and Simonsen, H. T. (2015). Optimization of biochemical screening methods for volatile and unstable sesquiterpenoids using HS-SPME-GC-MS. Chromatography 2, 277–292. doi: 10.3390/chromatography2020277
Anterola, A., Shanle, E., Perroud, P.-F., and Quatrano, R. (2009). Production of taxa-4(5),11(12)-diene by transgenic Physcomitrella patens. Transgenic Res. 18, 655–660. doi:10.1007/s11248-009-9252-5
Bach, S. S., King, B. C., Zhan, X., Simonsen, H. T., and Hamberger, B. (2014). “Heterologous stable expression of terpenoid biosynthetic genes using the moss Physcomitrella patens,” in Plant Isoprenoids, ed. M. Rodríguez-Concepción (New York, USA: Springer), 257–271.
Bhakuni, R., Jain, D., Sharma, R., and Kumar, S. (2001). Secondary metabolites of Artemisia annua and their biological activity. Curr. Sci. 80, 35–48.
Brown, G. D. (2010). The biosynthesis of artemisinin (Qinghaosu) and the phytochemistry of Artemisia annua L.(Qinghao). Molecules 15, 7603–7698. doi:10.3390/molecules15117603
Buttner-Mainik, A., Parsons, J., Jerome, H., Hartmann, A., Lamer, S., Schaaf, A., et al. (2011). Production of biologically active recombinant human factor H in Physcomitrella. Plant Biotechnol. J. 9, 373–383. doi:10.1111/j.1467-7652.2010.00552.x
Drew, D. P., Rasmussen, S. K., Avato, P., and Simonsen, H. T. (2012). A comparison of headspace solid-phase microextraction and classic hydrodistillation for the identification of volatile constituents from Thapsia spp. provides insights into guaianolide biosynthesis in Apiaceae. Phytochem. Anal. 23, 44–51. doi:10.1002/pca.1323
Farhi, M., Marhevka, E., Ben-Ari, J., Algamas-Dimantov, A., Liang, Z., Zeevi, V., et al. (2011). Generation of the potent anti-malarial drug artemisinin in tobacco. Nat. Biotechnol. 29, 1072–1074. doi:10.1038/nbt.2054
François, I. E. J. A., Van Hemelrijck, W., Aerts, A. M., Wouters, P. F. J., Proost, P., Broekaert, W. F., et al. (2004). Processing in Arabidopsis thaliana of a heterologous polyprotein resulting in differential targeting of the individual plant defensins. Plant Sci. 166, 113–121. doi:10.1016/j.plantsci.2003.09.001
Ikram, N. K. B. K., Zhan, X., Pan, X., King, B. C., and Simonsen, H. T. (2015). Stable heterologous expression of biologically active terpenoids in green plant cells. Front. Plant Sci. 6:129. doi:10.3389/fpls.2015.00129
King, B. C., Vavitsas, K., Ikram, N. K. B. K., Schrøder, J., Scharff, L. B., Hamberger, B., et al. (2016). In vivo assembly of DNA-fragments in the moss, Physcomitrella patens. Sci. Rep. 6, 25030. doi:10.1038/srep25030
Komatsu, M., Uchiyama, T., Ōmura, S., Cane, D. E., and Ikeda, H. (2010). Genome-minimized Streptomyces host for the heterologous expression of secondary metabolism. Proc. Natl. Acad. Sci. U.S.A. 107, 2646–2651. doi:10.1073/pnas.0914833107
Liu, Q., Manzano, D., Tanic, N., Pesic, M., Bankovic, J., Pateraki, I., et al. (2014). Elucidation and in planta reconstitution of the parthenolide biosynthetic pathway. Metab. Eng. 23, 145–153. doi:10.1016/j.ymben.2014.03.005
Ma, C., Wang, H., Lu, X., Wang, H., Xu, G., and Liu, B. (2009). Terpenoid metabolic profiling analysis of transgenic Artemisia annua L. by comprehensive two-dimensional gas chromatography time-of-flight mass spectrometry. Metabolomics 5, 497–506. doi:10.1007/s11306-009-0170-6
Malhotra, K., Subramaniyan, M., Rawat, K., Kalamuddin, M., Qureshi, M. I., Malhotra, P., et al. (2016). Compartmentalized metabolic engineering for artemisinin biosynthesis and effective malaria treatment by oral delivery of plant cells. Mol. Plant. 9, 1464–1477. doi:10.1016/j.molp.2016.09.013
Martin, V. J., Pitera, D. J., Withers, S. T., Newman, J. D., and Keasling, J. D. (2003). Engineering a mevalonate pathway in Escherichia coli for production of terpenoids. Nat. Biotechnol. 21, 796–802. doi:10.1038/nbt833
Mukanganyama, S., Naik, Y. S., Widersten, M., Mannervik, B., and Hasler, J. A. (2001). Proposed reductive metabolism of artemisinin by glutathione transferases in vitro. Free Radic. Res. 35, 427–434. doi:10.1080/10715760100300941
Novotny, L., Samek, Z., and Sorm, F. (1966). The Structure of bisabolangelone a sesquiterpenic ketoalcohol from Angelica silvestris L. seeds. Tetrahedron Lett. 7, 3541–46. doi:10.1016/S0040-4039(01)82825-2
Paddon, C. J., and Keasling, J. D. (2014). Semi-synthetic artemisinin: a model for the use of synthetic biology in pharmaceutical development. Nat. Rev. Microbiol. 12, 355–367. doi:10.1038/nrmicro3240
Paddon, C. J., Westfall, P. J., Pitera, D. J., Benjamin, K., Fisher, K., Mcphee, D., et al. (2013). High-level semi-synthetic production of the potent antimalarial artemisinin. Nature 496, 528–532. doi:10.1038/nature12051
Pan, X.-W., Han, L., Zhang, Y.-H., Chen, D.-F., and Simonsen, H. (2015). Sclareol production in the moss Physcomitrella patens and observations on growth and terpenoid biosynthesis. Plant Biotechnol. Rep. 9, 1–11. doi:10.1007/s11816-015-0353-8
Pandey, N., and Pandey-Rai, S. (2016). Updates on artemisinin: an insight to mode of actions and strategies for enhanced global production. Protoplasma 253, 15–30. doi:10.1007/s00709-015-0805-6
Reski, R. (1998). Development, genetics and molecular biology of mosses. Plant Biol. 111, 1–15. doi:10.1111/j.1438-8677.1998.tb00670.x
Reski, R., Parsons, J., and Decker, E. L. (2015). Moss-made pharmaceuticals: from bench to bedside. Plant Biotechnol. J. 13, 1191–1198. doi:10.1111/pbi.12401
Ro, D. K., Paradise, E. M., Ouellet, M., Fisher, K. J., Newman, K. L., Ndungu, J. M., et al. (2006). Production of the antimalarial drug precursor artemisinic acid in engineered yeast. Nature 440, 940–943. doi:10.1038/nature04640
Rodriguez, S., Kirby, J., Denby, C. M., and Keasling, J. D. (2014). Production and quantification of sesquiterpenes in Saccharomyces cerevisiae, including extraction, detection and quantification of terpene products and key related metabolites. Nat. Protoc. 9, 1980–1996. doi:10.1038/nprot.2014.132
Schaefer, D. G., and Zrÿd, J. P. (1997). Efficient gene targeting in the moss Physcomitrella patens. Plant J. 11, 1195–1206. doi:10.1046/j.1365-313X.1997.11061195.x
Simonsen, H. T., Drew, D. P., and Lunde, C. (2009). Perspectives on using Physcomitrella Patens as an alternative production platform for thapsigargin and other terpenoid drug candidates. Perspect. Medicin. Chem. 3, 1–6.
Sy, L.-K., and Brown, G. D. (2002). The mechanism of the spontaneous autoxidation of dihydroartemisinic acid. Tetrahedron 58, 897–908. doi:10.1016/S0040-4020(01)01192-9
Tang, K., Shen, Q., Yan, T., and Fu, X. (2014). Transgenic approach to increase artemisinin content in Artemisia annua L. Plant Cell Rep. 33, 605–615. doi:10.1007/s00299-014-1566-y
Teoh, K. H., Polichuk, D. R., Reed, D. W., and Covello, P. S. (2009). Molecular cloning of an aldehyde dehydrogenase implicated in artemisinin biosynthesis in Artemisia annua. Botany 87, 635–642. doi:10.1139/B09-032
Ting, H.-M., Wang, B., Rydén, A.-M., Woittiez, L., Van Herpen, T., Verstappen, F. W. A., et al. (2013). The metabolite chemotype of Nicotiana benthamiana transiently expressing artemisinin biosynthetic pathway genes is a function of CYP71AV1 type and relative gene dosage. New Phytol. 199, 352–366. doi:10.1111/nph.12274
Turconi, J., Griolet, F., Guevel, R., Oddon, G., Villa, R., Geatti, A., et al. (2014). Semisynthetic artemisinin, the chemical path to industrial production. Org. Process Res. Dev. 18, 417–422. doi:10.1021/op5001397
van Herpen, T. W., Cankar, K., Nogueira, M., Bosch, D., Bouwmeester, H. J., and Beekwilder, J. (2010). Nicotiana benthamiana as a production platform for artemisinin precursors. PLoS ONE 5:e14222. doi:10.1371/journal.pone.0014222
Wallaart, T. E., Bouwmeester, H. J., Hille, J., Poppinga, L., and Maijers, N. C. (2001). Amorpha-4,11-diene synthase: cloning and functional expression of a key enzyme in the biosynthetic pathway of the novel antimalarial drug artemisinin. Planta 212, 460–465. doi:10.1007/s004250000428
Wang, B., Kashkooli, A. B., Sallets, A., Ting, H.-M., De Ruijter, N. C., Olofsson, L., et al. (2016). Transient production of artemisinin in Nicotiana benthamiana is boosted by a specific lipid transfer protein from A. annua. Metab. Eng. 38, 159–169. doi:10.1016/j.ymben.2016.07.004
Wu, S., Schalk, M., Clark, A., Miles, R. B., Coates, R., and Chappell, J. (2006). Redirection of cytosolic or plastidic isoprenoid precursors elevates terpene production in plants. Nat. Biotechnol. 24, 1441–1447. doi:10.1038/nbt1251
Yonekura-Sakakibara, K., and Hanada, K. (2011). An evolutionary view of functional diversity in family 1 glycosyltransferases. Plant J. 66, 182–193. doi:10.1111/j.1365-313X.2011.04493.x
Zhan, X., Zhang, Y., Chen, D., and Simonsen, H. T. (2014). Metabolic engineering of the moss Physcomitrella patens to produce the sesquiterpenoids patchoulol and α/β-santalene. Front. Plant Sci. 5:636. doi:10.3389/fpls.2014.00636
Zhang, Y., Teoh, K. H., Reed, D. W., Maes, L., Goossens, A., Olson, D. J., et al. (2008). The molecular cloning of artemisinic aldehyde Δ11(13) reductase and its role in glandular trichome-dependent biosynthesis of artemisinin in Artemisia annua. J. Biol. Chem. 283, 21501–21508. doi:10.1074/jbc.M803090200
Keywords: Physcomitrella patens, malaria, artemisinin, in vivo assembly, bioengineering
Citation: Khairul Ikram NKB, Beyraghdar Kashkooli A, Peramuna AV, van der Krol AR, Bouwmeester H and Simonsen HT (2017) Stable Production of the Antimalarial Drug Artemisinin in the Moss Physcomitrella patens. Front. Bioeng. Biotechnol. 5:47. doi: 10.3389/fbioe.2017.00047
Received: 19 April 2017; Accepted: 28 July 2017;
Published: 15 August 2017
Edited by:
Pamela J. Weathers, Worcester Polytechnic Institute, United StatesCopyright: © 2017 Khairul Ikram, Beyraghdar Kashkooli, Peramuna, van der Krol, Bouwmeester and Simonsen. This is an open-access article distributed under the terms of the Creative Commons Attribution License (CC BY). The use, distribution or reproduction in other forums is permitted, provided the original author(s) or licensor are credited and that the original publication in this journal is cited, in accordance with accepted academic practice. No use, distribution or reproduction is permitted which does not comply with these terms.
*Correspondence: Henrik Toft Simonsen, aGV0c0BkdHUuZGs=