- 1Tissue Engineering Core—LUCCAR, Morphology, Federal University of Espírito Santo (UFES), Vitória, Brazil
- 2Health Sciences Center, Federal University of Espírito Santo (UFES), Vitória, Brazil
- 3Health Sciences Center, Postgraduate Program in Biotechnology/RENORBIO, Vitória, Brazil
End-stage renal disease (ESRD) is characterized by the progressive deterioration of renal function that may compromise different tissues and organs. The major treatment indicated for patients with ESRD is kidney transplantation. However, the shortage of available organs, as well as the high rate of organ rejection, supports the need for new therapies. Thus, the implementation of tissue bioengineering to organ regeneration has emerged as an alternative to traditional organ transplantation. Decellularization of organs with chemical, physical, and/or biological agents generates natural scaffolds, which can serve as basis for tissue reconstruction. The recellularization of these scaffolds with different cell sources, such as stem cells or adult differentiated cells, can provide an organ with functionality and no immune response after in vivo transplantation on the host. Several studies have focused on improving these techniques, but until now, there is no optimal decellularization method for the kidney available yet. Herein, an overview of the current literature for kidney decellularization and whole-organ recellularization is presented, addressing the pros and cons of the actual techniques already developed, the methods adopted to evaluate the efficacy of the procedures, and the challenges to be overcome in order to achieve an optimal protocol.
Introduction
Chronic kidney disease (CKD) and its complications are of paramount importance in the context of public health (Lai et al., 2013), reaching approximately 8–16% of the adult population worldwide (Jha et al., 2013; United States Renal Data System, 2015). Its overall prevalence progresses at an alarming rate and is correlated with the dramatic increase in obesity in the last 30 years, leading to Type II diabetes, metabolic syndrome, and renal failure—also known as end-stage renal disease (ESRD) (Nguyen and El-Serag, 2010; Yokote et al., 2012). In the USA, it is estimated that one in every ten Americans, approximately 23 million people aged 20 years or older, has some form of CKD [Centers for Disease Control and Prevention (CDC), 2014], and this number is expected to rise given that the elderly population is also growing (Murray et al., 2013).
Although the incidence of CKD is rising, the available therapeutic options remain limited (Levey and Coresh, 2012). Current therapeutic options for renal replacement are limited to peritoneal dialysis, hemodialysis, or kidney transplantation (KT). Renal transplantation is the ideal treatment for patients with ESRD: it improves long-term survival (Wolfe et al., 1999), leads to a better quality of life (Dew et al., 1997; Anil Kumar and Mattoo, 2015), and is effective in terms of cost compared with long-term dialysis (Hutton, 1999). The success of renal transplantation depends on the donor type, age, sex, race, and primary cause of ESRD. In general, the 1-year graft survival and patient survival advantage experienced by living donor transplant recipients persist at 5 and 10 years post-transplant (USRDS, 2016). According to the United States Renal Data System, in 2013, the rate of 5-year graft survival was 73% for recipients of deceased donor transplants, while it was 85% for living donor transplant recipients (United States Renal Data System, 2015). However, KT is often associated with chronic dysfunction linked to immune rejection (National Kidney and Urologic Diseases Information Clearinghouse, 2012). In addition, the significant increase in the use of living kidney donors and cadavers is not compatible with the availability of organs; in the last years, there were more than 100,000 patients in the USA waiting for a transplant with an average of more than 3.6 years (Matas et al., 2015; United States Renal Data System, 2015).
Alternative Management of Kidney Disease
For patients with ESRD, KT is closely associated with an increase in life expectancy, improving the quality of life, and is a cost-effective therapy (Wolfe et al., 1999; Johnson et al., 2000; Ojo et al., 2001; Merion et al., 2005; Tonelli et al., 2011; Rana et al., 2015). To emphasize further this growing disparity between the supply and demand for organs, the total number of KTs performed annually in the United States has remained static over the last decade (Valapour et al., 2014). Furthermore, there are an increasing number of elderly donors and recipients for KT (Wolfe et al., 2010; Abecassis et al., 2012). US initiatives, for example, have resulted in an increase in non-conventional sources of donors, such as expanded criteria donors (ECD) (Port et al., 2002; Stratta et al., 2006; Pascual et al., 2008; Klein et al., 2010), donors after cardiac death donation (DCD) (Howard et al., 2005; Abt et al., 2006; Farney et al., 2011), standard criteria donors (SCD) with warm ischemia times or prolonged cold (CIT) (Roodnat et al., 2003; Kayler et al., 2011; Kim et al., 2013; Debout et al., 2015; Xia et al., 2015), acute kidney injury (AKI) donors (Anil Kumar et al., 2006; Kayler et al., 2009; Farney et al., 2013; Hall et al., 2015; Heilman et al., 2015; Xia et al., 2015), double-kidney transplantation (DKT) and donors at the extremes of age (Johnson et al., 1996; Cruzado et al., 2007; De Serres et al., 2010; Fernández-Lorente et al., 2012). Recently, it was demonstrated that desensitization of the patient and subsequent transplantation with a kidney from an incompatible live donor increase the patient survival rate compared with those who remain on the waiting list for transplantation (Orandi et al., 2016). However, all of these alternatives have the drawback of requiring donors.
Several studies have shown that regenerative therapy using mature adult or stem cells may have success. They have demonstrated that postnatal mammalian kidneys may be partially repaired after resection, and this regeneration is mainly due to the proliferation of mature surviving cells or stem cells present in the kidney, such as glomerular parietal epithelial cells (a type of renal progenitor cell) (Vogetseder et al., 2008; Swetha et al., 2011; Wen et al., 2012; Franquesa et al., 2013). After stress, these cells migrate to the damaged area and proliferate and then differentiate into new somatic cells (Hanna et al., 2010; Yamanaka and Blau, 2010; Wen et al., 2012). These results suggest that regenerative solutions offer great potential for the treatment of ESRD. However, achieving a clinically significant regeneration level has proven to be extremely difficult due to the complexity of this organ (Yu et al., 2014).
Another alternative to the shortage of organs would be xenotransplantation using animal organs (Yokoo and Kawamura, 2009; Wang et al., 2014), through a strategy that combines organ decellularization and the insertion of patient’s cells to avoid the risk of adverse immunological reactions (Barakat et al., 2012). The use of pig kidneys for tissue engineering is an attractive approach, mainly because the size of porcine organs is similar to that of humans, and pig scaffolds may allow better adhesion, survival, and maintenance of human cells than scaffolds obtained from dogs or monkeys (Nakayama et al., 2010; Song and Ott, 2011; Faulk et al., 2014a,b; Habka et al., 2015; Moini et al., 2015; Rana et al., 2017; Lih et al., 2016; McKee and Wingert, 2016). The idea of building a functional kidney using specific stem cells from the patient supports a tangible alternative. In the last 2 years, there has been substantial progress toward this goal (Uzarski et al., 2014). In theory, this approach could increase the range of options for KT, enabling the improvement of more patients and potentially minimizing or eliminating the need for long-term immunosuppressive therapy and reducing the waiting time for treatment.
Tissue Engineering
The increasing number of patients facing end-stage diseases, the shortage of organ donors, and challenges that surround allogenic transplants drive the evolution of tissue engineering and regenerative medicine (Caldas et al., 2011). Tissue engineering is a promising alternative to organ transplantation, with a huge potential in tissue regeneration and repair processes, providing solutions to the conditions in which native tissue is compromised (Lu et al., 2011; He and Callanan, 2013; Teodori et al., 2014). Recent advances in the use of stem cells and regenerative medicine have offered new hope for the treatment of kidney disease, especially ESRD (Krause and Cantley, 2005; Ross et al., 2009; Li et al., 2010).
To reconstruct a new tissue, a triad of components is needed: cells, biomaterials to be used as supports or scaffolds, and growth factors (Ahn et al., 2007; Narayanan et al., 2009; Ng et al., 2011). Biomaterials play a central role because most mammalian cell types are dependent on anchorage (Hollister, 2005; O’Brien, 2011; Cheng et al., 2013; Guan et al., 2015a), and one of the crucial challenges of this area is the selection of the ideal scaffold for cell attachment to ensure its functional integrity. Using a decellularized organ composed of extracellular matrix (ECM), regenerative medicine proposes new horizons (Gilbert et al., 2006; Crapo et al., 2011).
Biological scaffolds consisting of ECM are commonly used for various reconstructive surgical applications, and their use in regenerative medicine for tissue and organ replacement has increased in the last decade. ECM common sources include the dermis, pericardium, small intestine, sub-mucosa, and bladder (Badylak, 2002; Badylak et al., 2009; Rose et al., 2009; Orlando et al., 2011a; Yagi et al., 2013). It is possible to obtain ECM scaffolds from different animals, which can later be recolonized with human cells and then used for transplantation in humans (Cooper et al., 2002).
The ECM is a three-dimensional network that forms a milieu surrounding cells that reciprocally influences cellular function to modulate diverse fundamental aspects of cell biology (Hynes, 2009) such as support for organs and tissues, for cell layers in the form of basement membranes, and for individual cells as substrates for cell motility (Hynes, 2009). Two main classes of extracellular macromolecules make up the matrix (Lai et al., 2013) polysaccharide chains of the class called glycosaminoglycans (GAGs)—a gel-like polysaccharide ground substance, which are usually found covalently linked to protein in the form of proteoglycans, and (United States Renal Data System, 2015) fibrous proteins, including collagen, elastin, and several other molecules (laminins, fibronectins, tenascins) (Brown et al., 2006), which have both structural and adhesive functions (Alberts et al., 2002). GAGs are highly negatively charged and they bind positively charged ions and trap water molecules to form hydrated gels, thereby providing mechanical support to the ECM (Cooper, 2000). In addition, this structure allows nutrition, innervation, cell signaling, and tissue regeneration (Bosman and Stamenkovic, 2003; Naranjo et al., 2009; Ramage, 2011; Keane et al., 2012; Reticker-Flynn et al., 2012). In the kidney cortex, the ECM is present in different anatomical areas, with different functions depending on their molecular components. The ECM present in the interstitial medulla is physiologically more prominent than cortical interstitial ECM and quantitatively greater from the exterior to the interior of the medulla/papilla. The hilar region–renal pelvis (e.g., sub-urothelial basement membrane) and renal capsule also comprise the ECM. Renal interstitial ECM normally consists of collagen type I, III, V, VI, VII, and XV; sulfated- and non-sulfated GAGs; and polysaccharides (Genovese et al., 2014). Specifically, regarding the composition of the ECM of the glomerular basement membrane, the major compounds are laminin, type IV collagen, heparan sulfate proteoglycan (including agrin), and nidogen (Suh and Miner, 2013).
It is possible that scaffolds composed of ECM that integrate the basic structure of the organ that may be used to promote kidney regeneration. In the last decade, numerous studies have demonstrated that constructed ECM scaffolds can support the growth and differentiation of multiple cell types (Reing et al., 2010; Lu et al., 2011; Moroni and Mirabella, 2014). For success clinically, the ECM must be decellularized (Freytes et al., 2008; Choi et al., 2012). It has been shown that decellularized scaffolds may maintain the tri-dimensional composition and biological activity of the ECM; this enables it to be a useful material for cell adhesion, differentiation, and proliferation (Barkan et al., 2010; Vorotnikova et al., 2010; Tien and Nelson, 2014). The advantage of using acellular scaffolds of organs is the possibility not only of using the ECM as a structural scaffold but also allowing the spread of signals that can be exchanged with the cells that adhere to induce migration and differentiation (Gilbert et al., 2007; Gong et al., 2008). The ECM also plays an important role in the maintenance of an adequate network of vessels and growth factors, which allows the appropriate structure of the organ when rebuilt (Ross et al., 2012). These characteristics are indispensable in complex tissues with different cellular components.
Because of the high complexity of the anatomical structure and composition of kidney, renal ECM scaffold has been proposed as a promisor strategy for the reconstruction of a viable kidney. However, obtaining a viable kidney ECM is extremely difficult because of its complex composition, including amino acids, collagens, glycoproteins, GAGs, and microstructures required for filtration (Badylak, 2004; Gagliardini et al., 2010; Mecham, 2001). The appropriate process for the decellularization of tissues/organs must preserve the essential components of the ECM (Song and Ott, 2011). By contrast, synthetic organic scaffolds are inappropriate in terms of complexity. Regarding highly artificial efficient membranes and cell-based bioreactors, there have been successful attempts concerning the miniaturization of the existing bioartificial kidney using various silicon and related thin-film material substrates commonly used in the construction of microelectromechanical systems (MEMS), as well as novel silicon nanopore membranes (SNMs) (Fissell et al., 2006; Fissell and Roy, 2009). The final developmental step based on prior success of the renal tubule cell assist device (RAD) in acute disorders and wearable bioartificial kidney (WEBAK) in preclinical models is the current approach to design, fabricate, and test in preclinical models a functional fully implantable bioartificial kidney (IBAK) (Roy et al., 2011). However, there are still substantial technical challenges regarding the safety and operation of the device, as well as its effectiveness, which need to be overcome (Kooman et al., 2015).
The implantation of renal ECM scaffolds has shown benefit in the promotion of angiogenesis, recruitment of circulating progenitor cells, minimization of immunogenic reactions, and reduction of disease transmission risks (Vavken et al., 2009). Thus, decellularization of an entire organ arises as a novel approach to the generation of a natural 3D architecture.
Methods and Processes Applied for Organ Decellularization
Decellularization is a process that may involve a blend of chemical, physical, and enzymatic treatments (Bolland et al., 2007; Badylak et al., 2009; Lu et al., 2011; He and Callanan, 2013). More specifically, it may employ detergents, salts, enzymes, and/or physical agents for the removal of tissue and organ cells. There are many existing methods for different applications (Gilbert et al., 2006; Badylak et al., 2011; Gilbert, 2012; Ofenbauer et al., 2015). In general, the process removes the cells present in the tissue, preserving partly the proteins of the ECM, such as collagen, with significantly reduced immunogenic potential (Mirmalek-Sani et al., 2013; Caralt et al., 2015). Thus, the ECM can be repopulated with cells extracted from the patient, creating a new organ with minimal probability of being rejected (Hammerman, 2002; Badylak and Gilbert, 2008).
Currently, the most efficient and robust method for decellularization is the use of chemical infusion. The chemical reagents, particularly detergents or acids, remove native cells by disrupting cell membranes and isolating the cellular components of the ECM (Gilbert et al., 2006). For example, peracetic acid (PAA) is commonly used because it removes nucleic acids with minimal effect on the ECM structure (Gilbert et al., 2008; Gao et al., 2015). Triton X-100, a non-ionic detergent, is the most efficient for the removal of cellular debris from tissues such as heart valves (Liao et al., 2008). Sodium dodecyl sulfate (SDS) is reported to be more effective than Triton X-100 to eliminate cells in the medullary regions of dense organs such as the kidney (Nakayama et al., 2010), and sodium deoxycholate (NaDOC), an anionic detergent, can completely remove the cytoplasmic proteins in dense tissues (Wang et al., 2014).
Decellularized kidneys using Triton X-100 retained growth factors and ECM components, although the cells were not properly removed. On the other hand, decellularization with SDS could sufficiently remove cells while preserving the ECM (Matthiesen et al., 2007; Tanemoto et al., 2008; Nakayama et al., 2010, 2011, 2013; Ross et al., 2012; Sullivan et al., 2012; Caralt et al., 2015). Choi et al. (2015), however, obtained opposite results regarding the comparison between the effects of SDS and Triton X-100 on porcine kidneys. They observed that a richer ECM protein and growth factor content were present in the Triton-treated scaffold than in the SDS-treated scaffold; additionally, cell viability, adherence, and proliferation were higher with the Triton-treated scaffold than with the SDS-treated scaffold (Choi et al., 2015). Furthermore, other researchers have demonstrated that the combination of the two agents produces better results (Sugawara et al., 2011). In view of these divergences, there is still a need to identify more suitable decellularizing agents to improve the quality of the obtained scaffolds (Table 1) (Ross et al., 2009, 2012; Peloso et al., 2013; Song et al., 2013).
Elements That Influence the Decellularization Process
The decellularization processes require sensitive methods due to the fragility of the organs and their internal structural complexity. Therefore, it is necessary to develop techniques for decellularization and residual DNA removal simultaneously to preserve the integrity of the ECM. There are several factors that influence the process of decellularization, such as cell density, body weight, lipid content, the organ thickness, and the intrinsic properties of the substances employed to remove the cells (Ross et al., 2009). Furthermore, it is necessary to consider the mechanical forces involved in the process, such as the pressure of perfusion, flow rate, and possibility of the use of retrograde infusion of fluids (Lin et al., 2016). Another point to be considered is that the methods used in the organs of small animals (e.g., mice) have to be adapted to larger animals, such as monkeys and pigs, which resemble humans, at least regarding the size of the organ. In this sense, some studies have already demonstrated good results with the decellularization of monkey’s and pig’s kidneys, with preservation of the ECM scaffolds (Badylak et al., 2009; Orlando et al., 2011b, 2012; Sullivan et al., 2012).
Regarding the types and concentrations of detergents employed in the decellularization process, we considered that each detergent exhibits a distinct pattern of action depending on the tissue involved (Baptista et al., 2009). There are two detergents widely used currently—Triton X-100 and SDS—at concentrations ranging from 0.1 to 5%, perfused alone or in combination (Baptista et al., 2011; Barakat et al., 2012; Sullivan et al., 2012; Nakayama et al., 2013). Recently, He et al. (2016) compared the success of rat kidney decellularization through SDS perfusion in different combinations of time of perfusion (4 and 8 h) and concentration of SDS (0.125, 0.25, 0.5, and 1.0%), with the best result obtained with 0.125% SDS perfused through 4 h. In this condition, histological and immunohistochemical evaluation were not different from other conditions, but growth factor maintenance was more efficient. Another recent approach to decrease SDS exposure time and improve the decellularization process of porcine kidneys employed a combination of freezing/thawing, low concentrations of SDS, incremental increases in flow rate under constant pressure, and applying osmotic shock to the cellular membranes. These procedures preserved the microstructure of the scaffold while still removed 99% of the DNA, enhanced cell–ECM interactions, and allowed the seeded cells to grown more rapidly when compared to kidneys decellularized only with SDS perfusion for longer period (Poornejad et al., 2016a). This same group compared the effect of five different decellularization agents on porcine renal tissue (0.1 N NaOH, 1% PAA, 3% Triton X-100, 1% SDS, and 0.05% trypsin/EDTA). While the NaOH solution was the most efficient on cell removal and cell viability after recellularization, it damaged the ECM components, besides it also produced lots of caustic waste. This could be avoided with the use of HCl to neutralize NaOH. In turn, SDS led to less severe damage to ECM structure, but the cell viability and proliferation after recellularization were not as efficient as the NaOH solution. Triton X-100 and PAA preserved ECM structure and growth factors, although these reagents were not as effective as the SDS and NaOH on cell removal (Poornejad et al., 2016b). In summary, the NaOH solution was considered the best choice if the intention is implantation of cells and rapid biodegradation of the scaffold. Nevertheless, adopting a multistep decellularization protocol using ionic and non-ionic detergent exposure would probably result in better preservation of a relatively intact structure.
Decellularization Assessment
Maintaining the architecture and composition of the ECM is the largest challenge to the success of the decellularization process. With respect to the ECM composition, although many groups have demonstrated the retention of collagen, laminin, elastin, and fibronectin after decellularization (Caralt et al., 2015), the reduction or depletion of ECM proteins and growth factors has also been reported (Singh et al., 2010; Akhyari et al., 2011; Petersen et al., 2012; Wallis et al., 2012; Caralt et al., 2015). The retention of the main components of the ECM, such as collagen and laminin, provides the preservation of the ultrastructure of the scaffold, which can facilitate repopulation by providing the necessary spatial orientation (Scarritt et al., 2015). To evaluate the ECM structure maintenance, a system for histological classification is usually applied to verify whether the fundamental characteristics of renal scaffolds were preserved after decellularization. This system may include assessment of the ECM structure of tubules, glomeruli, and vessels, as well as the level of removal of cells (Figures 1C,D). Transmission electron microscopy has also been used to demonstrate whether the structure of the glomerular basement membrane was preserved after decellularization (Goh et al., 2013; Orlando et al., 2013). As a further evaluation of the retention of ECM components, many researchers have employed the use of traditional engineering techniques such as atomic force microscopy and uni- or bi-axial mechanical tests to evaluate the biophysical properties of the decellularized organ. In many cases, decellularization affects the rigidity of the matrix due to the removal of cells and damage to ECM components (Ott et al., 2008, 2010; Nakayama et al., 2010; Price et al., 2010; Wainwright et al., 2010; Daly et al., 2012; Petersen et al., 2012; Goh et al., 2013). The evaluation of the growth factor retention rate may also be a pattern that indicates the biofunctionality of the ECM (Caralt et al., 2015). Recent studies have shown that preservation of the ECM induces pluripotent cells cultured in the decellularized scaffolds to differentiate into tissue-specific cells (Ross et al., 2009; Cortiella et al., 2010). This cell differentiation linked to ECM intrinsic properties allows the engineering of complex tissues. Moreover, the composition of the ECM is dynamic and constantly changing in response to the metabolic activity of living cells, which modulate their own niche (Badylak et al., 2011). Withal the in silico predication of the genes that encode the ECM components and cell proteins that interact with, or modify the ECM, resulted in a database called “the matrisome” (Naba et al., 2012; Randles et al., 2017). Proteomic analysis by the use of mass spectrometry analysis focused on the matrisome, allow a complete identification and quantification of the ECM components, and already represent powerful resources for researchers (Gessel et al., 2015; Hill et al., 2015; Calle et al., 2016).
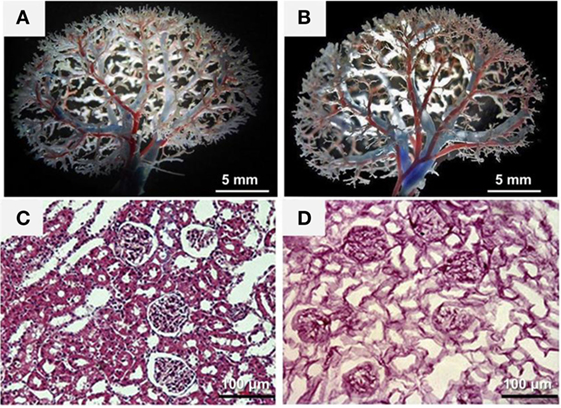
Figure 1. Characterization of the decellularized kidney scaffolds. (A,B) Vascular corrosion casting showed a normal vascular tree of the decellularized kidney scaffold (B) compared with that of the intact kidney (A). (C,D) H&E staining showed the existence of blue-stained nuclei in the intact kidney (C) but not in the decellularized kidney scaffold (D). Reprinted and modified with permission from Yu et al. (2014).
In addition to the parenchymal structures, maintaining the acellular scaffold microvasculature is critical for subsequent recolonization. The perfusion of dyes followed by angiographic analyses with micro computed tomography or vascular corrosion are useful strategies to evaluate the structure of the vascular tree after decellularization and have been performed in kidneys (Figures 1A,B) (Ott et al., 2008; Shupe et al., 2010; Uygun et al., 2010; Baptista et al., 2011; Barakat et al., 2012; Sullivan et al., 2012; Bonvillain et al., 2013; Caralt et al., 2013; Goh et al., 2013; Mirmalek-Sani et al., 2013; Yu et al., 2014). The complete removal of the DNA from the ECM donor is important to prevent a possible inflammatory response in its implementation into the host after recellularization (Badylak et al., 2009; Gilbert et al., 2009). Currently, the techniques for evaluating the presence of DNA in the ECM include spectrophotometric analyses (Rosario et al., 2008; Narayanan et al., 2009), histological stains (Bolland et al., 2007; Rosario et al., 2008; Gilbert et al., 2009), and immunohistochemical methods (Woods and Gratzer, 2005). The quantification of beta-actin gene (one of the main non-muscular cell components) is another reliable alternative method to assess the presence of DNA in the ECM (Kabsch et al., 1990). Furthermore, the beta-actin gene has been widely used as a positive control in polymerase chain reactions (PCRs) (Bellis et al., 2003) and is also configured as a pattern in gene tests due to its constitution and ubiquitous expression (Nygard et al., 2007).
The decellularization techniques developed until the present moment have not been able to remove 100% of the native cellular components without damage to the ECM structure and composition. The residual DNA content may cause cytocompatibility problems in vitro and adverse events in hosts during the reintroduction of cells in vivo (Nagata et al., 2010; Zhang et al., 2010). The threshold concentration of residual cellular components within the ECM, which is sufficient to cause the host response, depends on the (Lai et al., 2013) source of the ECM (United States Renal Data System, 2015), immune response of the receiver, and (Jha et al., 2013) environment in which the ECM will be installed. In this regard, the minimum criteria that reflect a satisfactory decellularization process involve displaying less than 50 ng of double-stranded DNA per mg of ECM (dry weight) with fragments lower than 200 base pairs and the absence of visible nuclear material on optical microscopy stained with 4′,6-diamidino-2-phenylindole (DAPI) or hematoxylin (Figure 1) (Woods and Gratzer, 2005; Crapo et al., 2011). Thus far, there is no consensus regarding the use of a specific detergent because this can degrade collagen, even in similar tissues, thus decreasing the mechanical strength of the organ (Cartmell and Dunn, 2000; Woods and Gratzer, 2005).
Scaffold Sterilization
Prior to use in vitro or in vivo, the ECM scaffold must be sterilized to remove all endotoxins and possible viral or bacterial DNA. The solvents or acidic solutions may be used for this process (Hodde and Hiles, 2002; Gorschewsky et al., 2005). However, these solutions can damage the ECM and affect the adhesion of cells during repopulation (Sun and Leung, 2008). The use of gamma irradiation or ethylene oxide is also reported, but these approaches also alter the ultrastructure of the ECM/scaffold, with the loss of collagen fibers and GAGs, decrease in cell proliferation and alterations in porosity and swelling properties (Poornejad et al., 2016c). Furthermore, exposure to this type of process can activate immune responses from the receptor (Qiu et al., 2009). One alternative for the sterilization of the kidney scaffold without damage to the ECM is the infusion of antibiotic and antifungal solutions, such as sodium azide or a combination of penicillin, streptomycin, and amphotericin, generally diluted in sterile phosphate-buffered saline (PBS). Perfusion with sterile filtered 70% ethanol is also used before perfusion with antibiotics (Nakayama et al., 2010, 2011; Xu et al., 2014; Poornejad et al., 2015). The kidney scaffold is usually perfused with the antimicrobial solution over a period of time to ensure that no microorganisms remain in the scaffold (Nakayama et al., 2010, 2011; Song et al., 2013; Bonandrini et al., 2014). Recently, Poornejad et al., 2016c showed that a 0.2% PAA in 1 M NaCl solution was the best method for decontamination of porcine decellularized ECM over other sterilization solutions such as 70% ethanol and 0.2% PAA in 4% ethanol or gamma-irradiation.
Cell Sources for Kidney Recellularization
The kidney is a complex organ that contains more than 26 types of cells derived from ureteric bud and the metanephrogenic mesenchyme (MM) (Al-Awqati and Oliver, 2002; Nishinakamura, 2008; Gouon-Evans, 2014). The renal corpuscle is composed of endothelial cells, mesangial cells, visceral epithelial cells, also known as podocytes, and parietal epithelial cells, besides the cells that comprise the juxtaglomerular apparatus, such as peripolar cells, juxtaglomerular granular cells, extraglomerular mesangial cells, and cells from the macula densa (Barajas, 1979). The renal proximal tubular cells are epithelial cells that can be distinguished in different cell types, according to the proximal tubular segment in which they are located. In rats, the proximal tubular segments are divided into S1, S2, and S3 segments, and the cells that comprise these segments have been well described by Maunsbach (1966). At the loop of Henle, the epithelial cells are also distinguished by their ultrastructural characteristics and their location within the different regions that form the medulla (Pannabecker, 2012). There are also cells that form the distal tubules, composed of morphologically distinct segments—the thick ascending limb of the loop of Henle, the macula densa, the distal convoluted tubule, and connecting tubules. Each of these segments presents different epithelial cells, with different functions related to their ultrastructural characteristics. Finally, the cells that comprise the collecting duct are the principal cells, type A and type B intercalated cells, and cells that form the inner medullary collecting duct, in addition to the different types of interstitial cells (Little et al., 2007).
In view of this complexity and variety of cells that form the kidneys, different strategies have been used to recellularize this organ. An immortalized human renal cortical-tubular epithelial (RCTE) cell line seeded through the renal artery within a bioreactor has also formed tubular structures and was not found within arterioles, suggesting that cells could translocate into the parenchyma or peri-tubular space to rest on the basement membrane (Caralt et al., 2015).
Another approach to repopulate the kidney scaffold is based on the contribution to urine production from various epithelial cell phenotypes presented in different niches along the nephron (Song et al., 2013). The infusion of suspended human umbilical venous endothelial cells (HUVECs) via the renal artery and a combination of rat neonatal kidney cells (NKCs) via the ureter is a promising strategy. These cells can be maintained in culture media supplemented with in vivo maturation signals such as glucocorticoids and catecholamines to accelerate in vitro nephrogenesis and the maturation of NKCs in acellular kidney matrices. In this sense, repopulation of the renal scaffold with epithelial and endothelial cells with the preservation of glomerular, tubular, and vascular architecture was observed through histologic evaluation after approximately 4 days in culture (Song et al., 2013). HUVECs lined vascular channels throughout the entire scaffold cross section, and the spatial organization of the regenerated epithelium and endothelium resembled the native nephron, which could provide the anatomic basis for renal function, including the processes of water and solute filtration, secretion, and reabsorption (Song et al., 2013).
Primary adult renal cells isolated from kidney cortical tissue by a process of digestion have also been applied to kidney recellularization (Abolbashari et al., 2016). Prior to seeding into the kidney scaffold, the isolated cells were maintained in primary culture, and the expression of aquaporin 1, aquaporin 2, aquaporin 4, ezrin, and podocin was analyzed by immunostaining to identify the cell phenotypes. The majority of the cells were positive for aquaporin1 and ezrin (a protein localized at the brush border membrane of proximal tubules that cross-links plasma membrane proteins with the actin cytoskeleton), indicative of proximal tubular cells, while distal tubular cells and collecting duct cells, expressing aquaporin 2 and aquaporin 4, respectively, represented a small percentage of the total cell population, with very few cells expressing podocin. After the identification of the phenotypes that confirmed the presence of cells from different renal segments, the cells were seeded into the scaffold. Some functional determinations such as electrolyte and protein adsorption, hydrolase activity, and erythropoietin (EPO) production were accessed to evaluate the functional capacity of the engineered kidneys, and the results were encouraging. However, they did not focus on the full characterization of the cultured cells, and cells with vascular phenotypes were not used, leading to the need for future tests to be performed (Abolbashari et al., 2016).
Embryonic stem (ES) cells have been used for organ recellularization with good results (Ross et al., 2009, 2012; Bonandrini et al., 2014). ES cells are especially interesting because of their pluripotency and ability to grow indefinitely. These cells have the potential to form any embryonic organ in vivo, and their capacity to differentiate into any of the adult renal cell types makes these cells great candidates for kidney recellularization (Keller, 2005). Pluripotent ES cells are usually maintained in culture medium in the presence of leukemia inhibitory factor, which reduces spontaneous cell differentiation, until the moment of the recellularization (Garreta et al., 2014). Infusion of the cells in the kidney scaffold through both the renal artery and ureter shows a pattern of distribution of the injected ES cells into tubular and vascular structures and their associated glomeruli, with multiplication of the cells (Song et al., 2013). Furthermore, evidence of embryonic cell differentiation toward epithelial and endothelial phenotypes was obtained by immunohistochemistry and fluorescence microscopy (Ross et al., 2009, 2012; Guan et al., 2015b). When the ES cells are infused only by the renal artery, the cells remain largely in the vascular network, with few cells housed on the tubular segments (Bonandrini et al., 2014).
Despite the good results obtained in applying ES cells for organ recellularization, ethical questions and the teratogenic potential of ES may limit the use of these cells for organ repopulation (Nowacki et al., 2014). One possible alternative is the use of autologous adult stem cells that do not require direct ethical questions, do not lead to immunologic responses, and can be obtained in minimally invasive processes. Bone marrow mesenchymal stem cells (BM-MSCs) have frequently been used for kidney repair and regeneration (Burgkart et al., 2014), but stem cells from other sources such as adipose tissue or amniotic fluid also exhibit promising applications (Hass et al., 2011; Liu et al., 2015b). Induced pluripotent stem cells (iPSCs) can be generated directly from adult somatic cells via the transduction of reprogramming factors and closely resemble ESCs been also suitable for kidney regeneration (Rogers, 2011; Hendry and Little, 2012; Usui et al., 2012). Some studies have achieved highly efficient differentiation of human iPSCs into intermediate mesoderm cells with potential to generate embryonic renal progenitors and adult renal cell types (Mae et al., 2013), as well as iPSC differentiation into podocytes with cytoplasmic contractile response to angiotensin II (AII) and functional ability to albumin intake (Song et al., 2012). The reconstitution of kidney structures in vitro, including efficiently vascularized glomeruli with podocytes, as well as renal proximal and distal tubules with clear lumen have already been generated from iPSCs differentiated to MM (Taguchi et al., 2014). A schematic review detailing the differentiation of MM from iPSCs was published from this same group of researchers (Taguchi and Nishinakamura, 2014). Furthermore, iPSC-derived endothelial cells injected through the renal artery of decellularized kidney were able to outlining branching vasculature and individual glomeruli (Caralt et al., 2015). It can be considered that patient-decellularized kidneys reseeded with patient-derived iPSCs differentiated to the renal precursors and vascular progenitors may represent the most promisor recellularization option to the reconstruction of whole kidneys that can hereafter be used for autologous transplantation.
Whole-Organ Recellularization
Kidney recellularization is usually made by the anterograde infusion of cells through the vasculature or retrograde infusion through the ureter or both (Ross, 2009; Ross et al., 2009, 2012; Song et al., 2013; Bonandrini et al., 2014). The cells can be manually injected (Ross et al., 2009, 2012), or they may be subject to infusion using a syringe pump (Bonandrini et al., 2014) or peristaltic pump (Song et al., 2013; Caralt et al., 2015), which allows for control of the flow rate of infusion. These latter options, coupled to a bioreactor, seem to be more adequate because they ensure the constant infusion of cells without apparent damage to the scaffold. In addition, maintaining a constant or pulsatile perfusion of cell culture medium allows the viability, nutrition, proliferation, migration, and differentiation of the recent seeded cells (Scarritt et al., 2015). Another alternative to seeding the renal scaffolds is the delivery of the cells into the cortical region of renal scaffolds using a needle. The distance between the injection sites and depth of injection has to be optimized to ensure that the whole organ is covered, with cells homogeneously injected into the cortex site (Abolbashari et al., 2016).
The first attempt to recellularize a whole kidney used the incubation of the reseeded organ with static media. This procedure allowed less reproducible growth, significant apoptosis occurred, and this approach did not consistently maintain viability beyond approximately 4 days. Better results were observed with transverse sectioning of the pre-seeded organ and culture of the slices in a multiwell dish, but this approach cannot be extrapolated to rebuild the organ for transplantation (Ross et al., 2009). Still, a peristaltic pump coupled to appropriate tubing could deliver a physiologically normal pressure profile, in a temperature-controlled incubator supplied by regulated medical-grade sterile gases. In this case, there was sufficient cell viability and proliferation with migration into glomeruli and other small vessels, extending from the afferent arterioles and glomerular tufts to the efferent arterioles and peritubular capillary networks (Ross et al., 2009, 2012). Other attempts to seed cells by applying pressure to the collecting system failed to reach the glomerulus, encouraging the development of a bioreactor for the perfusion of cells through the renal artery and retrograde through the ureter, with a vacuum pressure of 40 cmH2O applied to the system (Figure 2). This approach ensured cell seeding with a transrenal gradient and culminating in cell dispersion throughout the entire kidney parenchyma (Song et al., 2013). Thereafter, other studies also used perfusion-based bioreactors for renal repopulation with considerable success (Bonandrini et al., 2014; Caralt et al., 2015; Abolbashari et al., 2016). A table with a summary of some methods and cell sources already used for kidney repopulation can be seen in the review published by Scarritt et al. (2015).
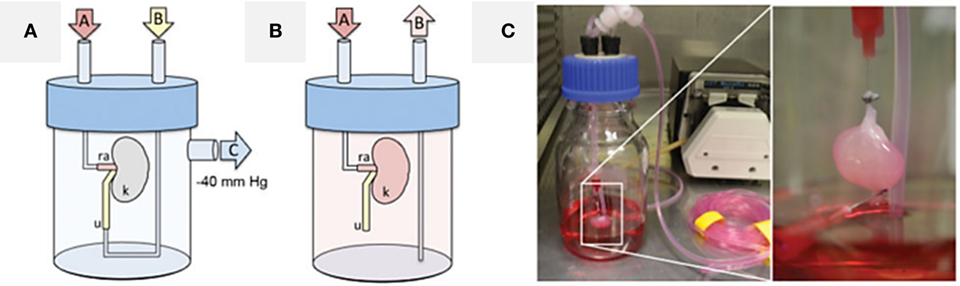
Figure 2. Cell seeding and whole-organ culture of decellularized rat kidneys. (A) Schematic of a cell seeding apparatus enabling endothelial cell seeding via port A attached to the renal artery (ra) and epithelial cell seeding via port B attached to the ureter (u), while negative pressure in the organ chamber is applied to port C, thereby generating a transrenal pressure gradient (left). (B) Schematic of a whole-organ culture in a bioreactor enabling tissue perfusion via port A attached to the renal artery (ra) and drainage to a reservoir via port B (u, ureter; k, kidney). (C) Cell seeding of decellularized rat kidneys in whole-organ culture. Reprinted and modified with permission from Song et al. (2013).
Characterization of the Reseeded Kidney Structure
The characterization of the reseeded kidney with respect to the ECM structure, cell adhesion, proliferation, migration, and differentiation, as well as the regeneration of a new kidney, that resembles the native structure is usually made by histological evaluation. Monitoring the distribution of the cells allows for observation not only of cell proliferation and migration through the scaffold but also the integrity of the vascular basement membranes based on the presence or absence of cells at the Bowman’s space (Ross et al., 2009, 2012; Song et al., 2013; Caralt et al., 2015). Morphometric analysis is also described for repopulated kidney characterization, such as the analysis of the average glomerular diameter, Bowman’s space, and glomerular capillary lumen (Song et al., 2013). Elastin staining gives the notion of vessel and artery integrity (Caralt et al., 2015). Transmission and scanning electron microscopy are also performed to evaluate the final structure of the rebuilt kidney, showing glomerular capillaries with engrafted podocytes and the formation of foot processes (pedicels) (Song et al., 2013; Caralt et al., 2015).
Histological staining with H&E performed for light microscopy allows the analysis of cell distribution, as well as the maintenance of ECM structure (Figure 3). Observations relative to the morphology of the engrafted cells can indicate their cytology features and assessment of the distribution, migration, and grouping tendencies, as well as cell–ECM interactions indicate the success of the chosen method (Ross et al., 2009; Song et al., 2013; Bonandrini et al., 2014; Caralt et al., 2015; Abolbashari et al., 2016). The use of GFP-stained cells for recellularization is also a strategic tool for the characterization of the distribution of the cells along the kidney scaffold by monitoring GFP expression by fluorescence microscopy (Ross et al., 2009, 2012). Other stains are also used to access cell distribution, such as the periodic acid-Schiff (PAS) stain, in addition to fluorescein wheat germ agglutinin (WGA) and DAPI staining, which documents cellular nuclei in the scaffold (Figure 3) (Ross et al., 2009; Bonandrini et al., 2014; Caralt et al., 2015).
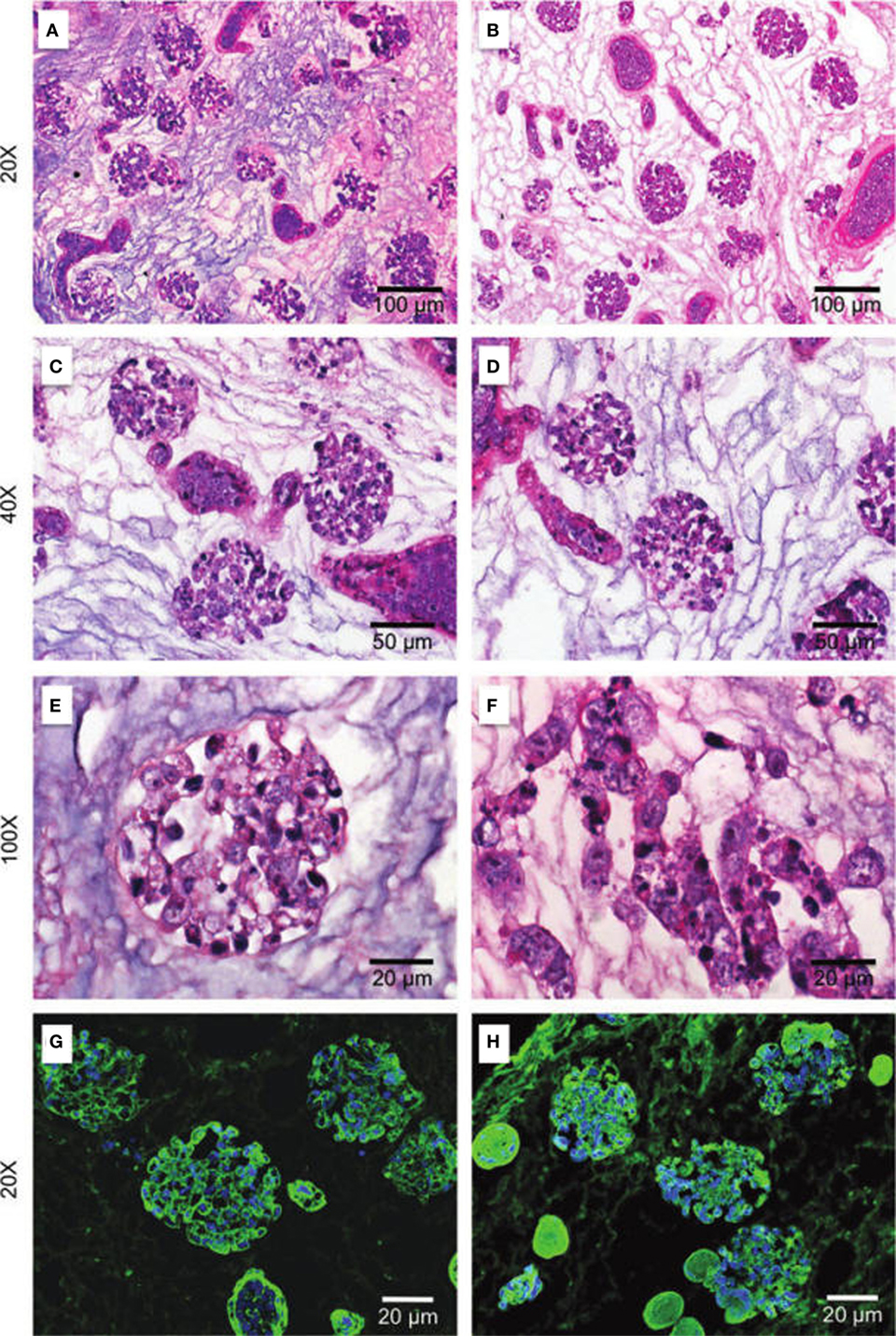
Figure 3. Repopulation of kidney scaffolds with mES cells. Hematoxylin and eosin staining at 24 h (A,C,E) and 72 h (B,D,F) and immunostaining for WGA agglutinin and DAPI at 24 h (G) and 72 h (H) in kidney scaffolds seeded with mES cells show a homogeneous distribution of cells into glomerular and vascular structures, peritubular capillaries, and tubules. mES, murine embryonic stem cells. Reprinted and modified with permission from Bonandrini et al. (2014).
In addition to the histological stains, the evaluations of the expression of cell differentiation and proliferation markers by immunostaining or RT-PCR are also tools for the characterization of the rebuilt kidney. In this regard, there is a range of antibodies available for review of different cell types, resulting in repopulation of kidney scaffolds. Some of the proteins and genes accessed for evaluation of cell differentiation are Oct-4, a marker of embryonic stemness, NCAM, a marker of mesoderm precursors, and the endothelial cell markers Tie-2 and CD3 (Bonandrini et al., 2014). Pan-cytokeratin immunostaining and staining of Pax-2 and Ksp-cadherin, a cell adhesion protein normally expressed in distal nephron tubular cells at later developmental stages, may also be useful in evaluating stem cell differentiation into epithelial tubular cells (Ross et al., 2009). Lectin-specific (BsLB4) staining for murine endothelial cells and the presence of VEGFR2 are indicators of the differentiation of ES cells into endothelial cells in the scaffold’s glomeruli and blood vessels (Ross et al., 2009, 2012). Markers such as Wilms tumor WT-1, podocalyxin like-2, glial cell-derived neurotrophic factor (GDNF), LMX1B, nephrin, and synaptopodinlike-2 are other indications of mature renal cells (Petrosyan et al., 2015). For a more accurate observation of the specific cells differentiated upon recellularization, analysis of the expression of podocin indicates a glomerular epithelial phenotype (Abolbashari et al., 2016), while the polarity of the cells with expression of (Na+)/(K+)-ATPase represents a proximal tubular phenotype (Song et al., 2013). E-cadherin expression can be used as a marker of the distal tubular phenotype, and the expression of β1-integrin indicates glomerular epithelial site-specific cell adhesion to ECM domains. Furthermore, as mentioned previously in the “Cell sources for kidney recellularization” section, the evaluation of the different aquaporins can also indicate the level of differentiation of the tubular cells in the different segments of the kidney (Song et al., 2013; Abolbashari et al., 2016). The expression of aquaporin1 together with ezrin indicates the differentiation of cells into proximal tubular cells, while distal tubular cells and collecting duct cells express aquaporin2 and aquaporin4, respectively (Hatano et al., 2013; Abolbashari et al., 2016).
Cell proliferation and viability are other important parameters to ensure that recellularization is successful. Cell viability is usually assessed by immunostaining of BAX, an apoptotic activator, or cleaved caspase-3, a critical executioner of apoptosis (Ross et al., 2009; Bonandrini et al., 2014). Another approach to assess cell apoptosis is TUNEL staining (Abolbashari et al., 2016). The analysis of cell proliferation, in turn, is made by staining with Ki-67, a nuclear protein necessary for cellular proliferation (Bullwinkel et al., 2006; Ross et al., 2009; Abolbashari et al., 2016) and proliferating cell nuclear antigen (PCNA), a co-factor for DNA polymerase δ activity in eukaryotic cells, that acts as a scaffold for the recruitment of proteins involved in DNA repair, chromatin remodeling, and DNA replication (Moldovan et al., 2007; Bonandrini et al., 2014; Abolbashari et al., 2016). The PCNA/BAX ratio is also used to confirm the success of the cell seeding over time (Petrosyan et al., 2015).
Functional analysis of the rebuilt kidney is also performed. The electrolyte reabsorption can be monitored by sodium uptake assays, with slices from the recellularized kidney cortex incubated with fluorescent sodium dyes and imaged using a fluorescent microscope. For this purpose, ouabain may be used to inhibit (Na+)/(K+)-ATPase on the cells, while normal kidney may be used as a positive control (Abolbashari et al., 2016). Albumin uptake can also be measured using the same methods adapted to sodium uptake, indicating the functionality of proximal tubular cells (Birn and Christensen, 2006). Amino acid transport is another important function performed by renal cells. This activity can be evaluated by accessing the activity of hydrolases, such as leucine aminopeptidase (LAP) and gamma glutamyltranspeptidase (GGT), which play significant roles in amino acid transfer (Meister, 1974; Abolbashari et al., 2016).
Secretome analysis is also an excellent approach to assess the functionality of the recellularized organ (Petrosyan et al., 2015). By analyzing the ability of the cells to secrete various proteins, it is possible to estimate the rate of differentiation of the seeded cells and their metabolic activities. Analysis of metalloproteinases and their inhibitors (MMPs and TIMPs) indicates the induction of cells to produce matrix modulators and their potential to repair and remodel the ECM. The secretion of growth factors (VEGF, TGF, PDGF) is related to different tissue processes, including matrix remodeling, immunomodulation, and angiogenesis. Cytokine (interleukins and GRO) and chemokine (MCP-1, RANTES, Fractalkine) secretion are indicative of multiple processes, including inflammation, angiogenesis, wound healing, and immunomodulation (Peloso et al., 2015; Petrosyan et al., 2015). Another pattern that can be observed to characterize renal function is the EPO production by renal cortical interstitial cells through real-time measurement of secreted EPO into the cell culture medium during bioreactor culture (Ullrich, 1979; Abolbashari et al., 2016).
Finally, transmission electron microscopy images may complement the analyses of cell functions, through the evaluation of the intensity of expression in the endoplasmic reticulum and Golgi apparatus, as well as the presence of secretory granules and vacuoles within the cells, which together are indicative of cellular activity and secretion, demonstrating the ability of cells to synthesize key molecules involved in tissue and organ homeostasis (Petrosyan et al., 2015). Alternatively, the development of non-invasive techniques for monitoring cell behavior such as surface scanning or multiphoton microscopy would be very important to improve the evaluation of the success of the recellularization process (Scarritt et al., 2015). Actually, some currently non-invasive techniques used in regenerative medicine may be used in tissue engineering, such as the resazurin perfusion assay, which allows the repetitive and rapid estimation of viable cell numbers during long-term ex vivo culture without destruction of the organ (Ren et al., 2015). Another non-invasive approach used in ECM scaffold remodeling evaluation is the use of a 19F-MRI (magnetic resonance imaging) contrast agent in 1H-MRI scans, which can adequately monitor the distribution of transplanted cells while allowing an evaluation of the formation of new tissue (Bible et al., 2012).
Future Challenges
We have reviewed one of the promising technologies in regenerative therapies for entire organ recovery. Solid organ regeneration based on perfusion-decellularized native ECM scaffolds has enormous potential in patients with organ failure. The use of biologic scaffolds can represent a potential alternative in the field of tissue engineering and regenerative medicine since the employed methods for decellularization can achieve success in the removal of cells without damaging the ECM. It is now necessary to improve these methods to extrapolate them for large-scale experiments. Figure 4 represents the development of functional organ by decellularization and recellularization, indicating the decellularizing agents, the ECM components to be evaluated post decellularization and the cell sources for recellularization of the organ.
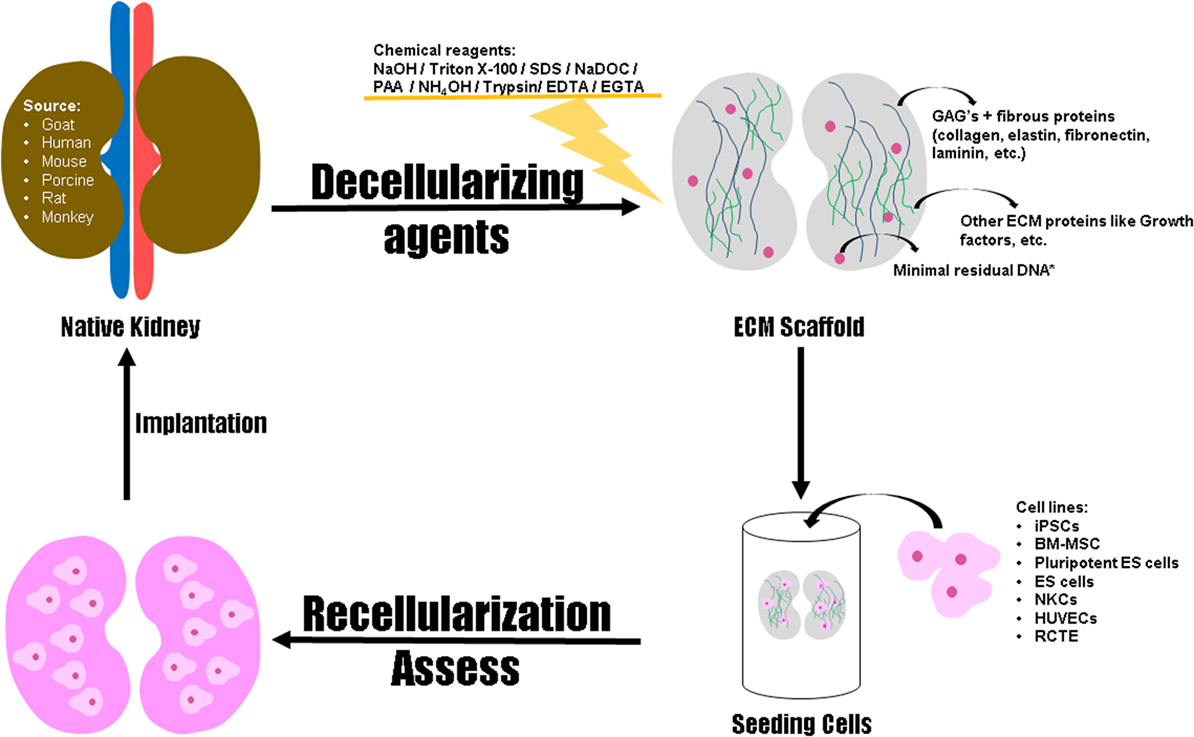
Figure 4. Schematic representation for the development of functional organ by decellularization and recellularization. Native kidneys provided from goat, human, mouse, porcine, rat, and monkey can be decellularized through biological, physical, or chemical methods aiming to obtain a scaffold with preserved structural integrity, retention of ECM proteins and other elements (i.e. growth factors). Regarding the cells types used for repopulation of the scaffold, studies using immortalized human renal cortical-tubular epithelial (RCTE) cell line, human umbilical venous endothelial cells (HUVECs), neonatal kidney cells (NKCs), Embryonic stem (ES) cells, pluripotent ES cells, Bone marrow mesenchymal stem cells (BM-MSCs) and induced pluripotent stem cells (iPSCs) have been driven. The number of adhered cells, the level of cell differentiation, and the functionality of the bioengineered organ must be evaluated for posterior implantation through allotransplantation or xenotransplantation. *Scaffold displaying less than 50 ng of double-stranded DNA per mg of ECM (dry weight) with fragments lower than 200 base pairs.
Many of the present efforts aimed at progressive strategies for cell expansion and differentiation might deliver original solutions applicable to organ engineering in the foreseeable future. Considering the several types of cells that have been used in different approaches for kidney recellularization research, choosing the ideal cell source will determine the effectiveness of the new building organ when in vivo transplantation occurs. Until now, ES cells have seemed to be the most efficient cell source for kidney recellularization. On the other hand, there are some ethical issues that make ES cells an unsuitable option for human organ recellularization and transplantation. With these considerations in mind, there is a need for more studies regarding iPSCs or adult stem cells, such as bone marrow-derived stem cells or adipocyte-derived stem cells, which present no ethical issues but have not yet been used in transplantation post-recellularization. iPSCs represent an ideal alternative to the ES cells, presenting the same ability of ES to efficiently expand in culture, as well as their capacity to differentiate into multiple cell types, with the advantage of having no ethical problems.
Although several methods have been used to characterize the success of kidney regeneration after decellularization, these processes are often directed toward histological characterization; thus, there is a lack of information regarding the functionality of the new kidney. The newly formed organ should mimic the same biological functions of the native organ without impairing its functionality; and this is what is expected for the next decade (Peloso et al., 2016). The in vitro capacity of regenerated kidneys to filter a standardized perfusate, clear metabolites, reabsorb electrolytes and glucose, and generate concentrated urine was assessed by Song et al. (2013), and the results indicate a partial recovered function of endothelial cells, podocytes, and tubular epithelial cells (Song et al., 2013). Their results could be related to incomplete seeding and the immature stage of seeded neonatal epithelial cells (FALK, 1955; Baum, 2009). Despite the functional immaturity observed through in vitro analysis, in vivo transplantation showed that regenerated kidney constructs provided urine production and clearance of metabolites, without the occurrence of bleeding or graft thrombosis (Song et al., 2013). However, this method has presently achieved only short-term functionality in vivo and only in rodent models. Until now, there has been no report of a mature complete regenerated kidney successfully transplanted into a donor with recovery of its functions, an observation that is a challenge in the field of kidney bioengineering at present. Recently, Caralt and colleagues investigated the ability of a decellularized kidney scaffold to hold sutures and support systemic arterial blood flow by implanting it into a rat recipient in an orthotopic position. Although there was an absence of clotting or major bleeding and the kidney scaffolds remained intact in situ for the duration of the study, these results cannot be extrapolated to a real transplantation, considering the absence of cells in the scaffolds (Caralt, 2015; Caralt et al., 2015).
In view of all of the advances observed in kidney bioengineering concerning organ decellularization and repopulation, there is a huge perspective regarding the confirmation of recovery of kidney function after transplantation. The expectation of researchers for the next 10 years is that the studies with decellularized and recellularized kidneys are in the preclinical phase, representing a high potential for translation compared to other tissue engineering approaches to kidney repair, such as renal organoids or 3D bioprinting (Peloso et al., 2016). Nonetheless, the adaptation of the methods described to date for use in human kidney regeneration and transplantation would change the current perspective related to the treatment of kidney diseases in the near future.
Author Contributions
AD and GS: each author has made a valuable contribution to the preparation of this manuscript such as writing, literature review, and table layout. The authors AD and GS contributed equally to this work. BN: conception of this work, drafting the work, and revising it critically for all content.
Conflict of Interest Statement
The authors declare that the research was conducted in the absence of any commercial or financial relationships that could be construed as a potential conflict of interest.
The reviewer, MH, and handling editor declared their shared affiliation, and the handling editor states that the process nevertheless met the standards of a fair and objective review.
Funding
The authors were supported by grants from Fundação de Amparo à Pesquisa e Inovação do Espírito Santo (EDITAL FAPES Nº 006/2014-UNIVERSAL: process number 0606/2015; EDITAL FAPES/CNPq Nº 012/2014-DCR: process number 0832/2015.); Rede Nordeste de Biotecnologia (RENORBIO) and Coordenação de Aperfeiçoamento de Pessoal de Nível Superior (CAPES).
References
Abecassis, M., Bridges, N. D., Clancy, C. J., Dew, M. A., Eldadah, B., Englesbe, M. J., et al. (2012). Solid-organ transplantation in older adults: current status and future research. Am. J. Transplant. 12, 2608–2622. doi: 10.1111/j.1600-6143.2012.04245.x
Abolbashari, M., Agcaoili, S. M., Lee, M.-K., Ko, I. K., Aboushwareb, T., Jackson, J. D., et al. (2016). Repopulation of porcine kidney scaffold using porcine primary renal cells. Acta Biomater. 29, 52–61. doi:10.1016/j.actbio.2015.11.026
Abt, P. L., Fisher, C. A., and Singhal, A. K. (2006). Donation after cardiac death in the US: history and use. J. Am. Coll. Surg. 203, 208–225. doi:10.1016/j.jamcollsurg.2006.03.014
Ahn, H. H., Kim, K. S., Lee, J. H., Lee, M. S., Song, I. B., Cho, M. H., et al. (2007). Porcine small intestinal submucosa sheets as a scaffold for human bone marrow stem cells. Int. J. Biol. Macromol. 41, 590–596. doi:10.1016/j.ijbiomac.2007.07.019
Akhyari, P., Aubin, H., Gwanmesia, P., Barth, M., Hoffmann, S., Huelsmann, J., et al. (2011). The quest for an optimized protocol for whole-heart decellularization: a comparison of three popular and a novel decellularization technique and their diverse effects on crucial extracellular matrix qualities. Tissue Eng. Part C Methods 17, 915–926. doi:10.1089/ten.TEC.2011.0210
Al-Awqati, Q., and Oliver, J. A. (2002). Stem cells in the kidney. Kidney Int. 61, 387–395. doi:10.1046/j.1523-1755.2002.00164.x
Alberts, B., Johnson, A., Lewis, J., Raff, M., Roberts, K., and Walter, P. (2002). “The extracellular matrix of animals,” in Molecular Biology of the Cell, 4th Edn, New York: Garland Science. Available at: https://www.ncbi.nlm.nih.gov/books/NBK26810/
Anil Kumar, B. N., and Mattoo, S. K. (2015). Organ transplant & the psychiatrist: an overview. Indian J. Med. Res. 141, 408–416. doi:10.4103/0971-5916.159268
Anil Kumar, M. S., Khan, S. M., Jaglan, S., Heifets, M., Moritz, M. J., Saeed, M. I., et al. (2006). Successful transplantation of kidneys from deceased donors with acute renal failure: three-year results. Transplantation 82, 1640–1645. doi:10.1097/01.tp.0000250908.62948.8f
Badylak, S. F. (2002). The extracellular matrix as a scaffold for tissue reconstruction. Semin. Cell Dev. Biol. 13, 377–383. doi:10.1016/S1084952102000940
Badylak, S. F. (2004). Xenogeneic extracellular matrix as a scaffold for tissue reconstruction. Transpl. Immunol. 12, 367–377. doi:10.1016/j.trim.2003.12.016
Badylak, S. F., Freytes, D. O., and Gilbert, T. W. (2009). Extracellular matrix as a biological scaffold material: structure and function. Acta Biomater. 5, 1–13. doi:10.1016/j.actbio.2008.09.013
Badylak, S. F., and Gilbert, T. W. (2008). Immune response to biologic scaffold materials. Semin. Immunol. 20, 109–116. doi:10.1016/j.smim.2007.11.003
Badylak, S. F., Taylor, D., and Uygun, K. (2011). Whole-organ tissue engineering: decellularization and recellularization of three-dimensional matrix scaffolds. Annu. Rev. Biomed. Eng. 13, 27–53. doi:10.1146/annurev-bioeng-071910-124743
Baptista, P. M., Orlando, G., Mirmalek-Sani, S.-H. H., Siddiqui, M., Atala, A., and Soker, S. (2009). Whole organ decellularization – a tool for bioscaffold fabrication and organ bioengineering. Conf. Proc. IEEE Eng. Med. Biol. Soc. 2009, 6526–6529. doi:10.1109/IEMBS.2009.5333145
Baptista, P. M., Siddiqui, M. M., Lozier, G., Rodriguez, S. R., Atala, A., and Soker, S. (2011). The use of whole organ decellularization for the generation of a vascularized liver organoid. Hepatology 53, 604–617. doi:10.1002/hep.24067
Barakat, O., Abbasi, S., Rodriguez, G., Rios, J., Wood, R. P., Ozaki, C., et al. (2012). Use of decellularized porcine liver for engineering humanized liver organ. J. Surg. Res. 173, e11–e25. doi:10.1016/j.jss.2011.09.033
Barkan, D., Green, J. E., and Chambers, A. F. (2010). Extracellular matrix: a gatekeeper in the transition from dormancy to metastatic growth. Eur. J. Cancer 46, 1181–1188. doi:10.1016/j.ejca.2010.02.027
Baum, M. (2009). Postnatal developmental renal physiology: a study of historic significance. Am. J. Physiol. Renal. Physiol. 296, F667–F668. doi:10.1152/ajprenal.00037.2009
Bellis, C., Ashton, K. J., Freney, L., Blair, B., and Griffiths, L. R. (2003). A molecular genetic approach for forensic animal species identification. Forensic Sci. Int. 134, 99–108. doi:10.1016/S0379-0738(03)00128-2
Bible, E., Dell’acqua, F., Solanky, B., Balducci, A., Crapo, P. M., Badylak, S. F., et al. (2012). Non-invasive imaging of transplanted human neural stem cells and ECM scaffold remodeling in the stroke-damaged rat brain by 19 F-and diffusion-MRI. Biomaterials 33, 2858–2871. doi:10.1016/j.biomaterials.2011.12.033.Non-invasive
Birn, H., and Christensen, E. I. (2006). Renal albumin absorption in physiology and pathology. Kidney Int. 69, 440–449. doi:10.1038/sj.ki.5000141
Bolland, F., Korossis, S., Wilshaw, S.-P., Ingham, E., Fisher, J., Kearney, J. N., et al. (2007). Development and characterisation of a full-thickness acellular porcine bladder matrix for tissue engineering. Biomaterials 28, 1061–1070. doi:10.1016/j.biomaterials.2006.10.005
Bonandrini, B., Figliuzzi, M., Papadimou, E., Morigi, M., Perico, N., Casiraghi, F., et al. (2014). Recellularization of well-preserved acellular kidney scaffold using embryonic stem cells. Tissue Eng. Part A 20, 1486–1498. doi:10.1089/ten.TEA.2013.0269
Bonvillain, R. W., Scarritt, M. E., Pashos, N. C., Mayeux, J. P., Meshberger, C. L., Betancourt, A. M., et al. (2013). Nonhuman primate lung decellularization and recellularization using a specialized large-organ bioreactor. J. Vis. Exp. 82, e50825. doi:10.3791/50825
Bosman, F. T., and Stamenkovic, I. (2003). Functional structure and composition of the extracellular matrix. J. Pathol. 200, 423–428. doi:10.1002/path.1437
Brown, B., Lindberg, K., Reing, J., Stolz, D. B., and Badylak, S. F. (2006). The basement membrane component of biologic scaffolds derived from extracellular matrix. Tissue Eng. 12, 519–526. doi:10.1089/ten.2006.12.519
Bullwinkel, J., Baron-Lühr, B., Lüdemann, A., Wohlenberg, C., Gerdes, J., and Scholzen, T. (2006). Ki-67 protein is associated with ribosomal RNA transcription in quiescent and proliferating cells. J. Cell. Physiol. 206, 624–635. doi:10.1002/jcp.20494
Burgkart, R., Tron, A., Prodinger, P., Culmes, M., Tuebel, J., van Griensven, M., et al. (2014). Decellularized kidney matrix for perfused bone engineering. Tissue Eng. Part C Methods 20, 553–561. doi:10.1089/ten.TEC.2013.0270
Caldas, H. C., Hayashi, P. C., and Abbud-Filho, M. (2011). Repairing the chronic damaged kidney: the role of regenerative medicine. Transplant. Proc. 43, 3573–3576. doi:10.1016/j.transproceed.2011.10.053
Calle, E. A., Hill, R. C., Leiby, K. L., Le, A. V., Gard, A. L., Madri, J. A., et al. (2016). Targeted proteomics effectively quantifies differences between native lung and detergent-decellularized lung extracellular matrices. Acta Biomater. 46, 91–100. doi:10.1016/j.actbio.2016.09.043
Caralt, M. (2015). Present and future of regenerative medicine: liver transplantation. Transplant. Proc. 47, 2377–2379. doi:10.1016/j.transproceed.2015.08.029
Caralt, M., Iacob, S., Obergfell, K., Akgun, B., Bijonowski, B., Abecassis, M., et al. (2013). A critical evaluation of kidney extracellular matrix after perfusion decellularization as a structural basis for renal tissue engineering. J. Tissue Eng. Regen. Med. 6, 1–429. doi:10.1002/term.1586
Caralt, M., Uzarski, J. S., Iacob, S., Obergfell, K. P., Berg, N., Bijonowski, B. M., et al. (2015). Optimization and critical evaluation of decellularization strategies to develop renal extracellular matrix scaffolds as biological templates for organ engineering and transplantation. Am. J. Transplant. 15, 64–75. doi:10.1111/ajt.12999
Cartmell, J. S., and Dunn, M. G. (2000). Effect of chemical treatments on tendon cellularity and mechanical properties. J. Biomed. Mater. Res. 49, 134–140. doi:10.1002/(SICI)1097-4636(200001)49:1<134::AID-JBM17>3.0.CO;2-D
Centers for Disease Control and Prevention (CDC). (2014). National Chronic Kidney Disease Fact Sheet: General Information and National Estimates on Chronic Kidney Disease in the United States. Atlanta, GA: Centers for Disease Control and Prevention. Available at: http://www.cdc.gov/diabetes/pubs/pdf/kidney_factsheet.pdf (accessed September 21, 2015).
Chae, S. Y., Chun, S. Y., Park, M., Jang, Y.-J., Kim, J. R., Oh, S. H., et al. (2014). Development of renal extracellular matrix (ECM) scaffold for kidney regeneration. Tissue Eng. Regen. Med. 11, 1–7. doi:10.1007/s13770-013-1125-6
Cheng, X., Liu, F., Zhang, Y., and Jiang, Y. (2013). Cloning and expression of a urate oxidase and creatinine hydrolase fusion gene in Escherichia coli. Ren. Fail. 35, 275–278. doi:10.3109/0886022X.2012.745787
Choi, S. H., Chun, S. Y., Chae, S. Y., Kim, J. R., Oh, S. H., Chung, S. K., et al. (2015). Development of a porcine renal extracellular matrix scaffold as a platform for kidney regeneration. J. Biomed. Mater. Res. A 103, 1391–1403. doi:10.1002/jbm.a.35274
Choi, Y. C., Choi, J. S., Kim, B. S., Kim, J. D., Yoon, H. I., and Cho, Y. W. (2012). Decellularized extracellular matrix derived from porcine adipose tissue as a xenogeneic biomaterial for tissue engineering. Tissue Eng. Part C Methods 18, 866–876. doi:10.1089/ten.tec.2012.0009
Cooper, D. K. C., Gollackner, B., and Sachs, D. H. (2002). Will the pig solve the transplantation backlog? Annu. Rev. Med. 53, 133–147. doi:10.1146/annurev.med.53.082901.103900
Cooper, G. M. (2000). “Cell walls and the extracellular matrix,” in The Cell: A Molecular Approach, 2nd Edn. Sunderland, MA: Sinauer Associates. Available at: https://www.ncbi.nlm.nih.gov/books/NBK9874/
Cortiella, J., Niles, J., Cantu, A., Brettler, A., Pham, A., Vargas, G., et al. (2010). Influence of acellular natural lung matrix on murine embryonic stem cell differentiation and tissue formation. Tissue Eng. Part A 16, 2565–2580. doi:10.1089/ten.tea.2009.0730
Crapo, P. M., Gilbert, T. W., and Badylak, S. F. (2011). An overview of tissue and whole organ decellularization processes. Biomaterials 32, 3233–3243. doi:10.1016/j.biomaterials.2011.01.057
Cruzado, J. M., Bestard, O., Riera, L., Torras, J., Gil-Vernet, S., Serón, D., et al. (2007). Immunosuppression for dual kidney transplantation with marginal organs: the old is better yet. Am. J. Transplant. 7, 639–644. doi:10.1111/j.1600-6143.2007.01671.x
Daly, K. A., Liu, S., Agrawal, V., Brown, B. N., Johnson, S. A., Medberry, C. J., et al. (2012). Damage associated molecular patterns within xenogeneic biologic scaffolds and their effects on host remodeling. Biomaterials 33, 91–101. doi:10.1016/j.biomaterials.2011.09.040
De Serres, S. A., Caumartin, Y., Noël, R., Lachance, J.-G., Côté, I., Naud, A., et al. (2010). Dual-kidney transplants as an alternative for very marginal donors: long-term follow-up in 63 patients. Transplantation 90, 1125–1130. doi:10.1097/TP.0b013e3181f8f2b8
Debout, A., Foucher, Y., Trébern-Launay, K., Legendre, C., Kreis, H., Mourad, G., et al. (2015). Each additional hour of cold ischemia time significantly increases the risk of graft failure and mortality following renal transplantation. Kidney Int. 87, 343–349. doi:10.1038/ki.2014.304
Dew, M. A., Switzer, G. E., Goycoolea, J. M., Allen, A. S., DiMartini, A., Kormos, R. L., et al. (1997). Does transplantation produce quality of life benefits? A quantitative analysis of the literature. Transplantation 64, 1261–1273. doi:10.1097/00007890-199711150-00006
Farney, A. C., Hines, M. H., al-Geizawi, S., Rogers, J., and Stratta, R. J. (2011). Lessons learned from a single center’s experience with 134 donation after cardiac death donor kidney transplants. J. Am. Coll. Surg. 212, 440–451; discussion 451–453. doi:10.1016/j.jamcollsurg.2010.12.033
Farney, A. C., Rogers, J., Orlando, G., al-Geizawi, S., Buckley, M., Farooq, U., et al. (2013). Evolving experience using kidneys from deceased donors with terminal acute kidney injury. J. Am. Coll. Surg. 216, 645–655; discussion 655–656. doi:10.1016/j.jamcollsurg.2012.12.020
Faulk, D. M., Wildemann, J. D., and Badylak, S. F. (2014a). Decellularization and cell seeding of whole liver biologic scaffolds composed of extracellular matrix. J. Clin. Exp. Hepatol. 5, 69–80. doi:10.1016/j.jceh.2014.03.043
Faulk, D. M., Johnson, S. A., Zhang, L., and Badylak, S. F. (2014b). Role of the extracellular matrix in whole organ engineering. J. Cell. Physiol. 229, 984–989. doi:10.1002/jcp.24532
Fernández-Lorente, L., Riera, L., Bestard, O., Carrera, M., Gomà, M., Porta, N., et al. (2012). Long-term results of biopsy-guided selection and allocation of kidneys from older donors in older recipients. Am. J. Transplant. 12, 2781–2788. doi:10.1111/j.1600-6143.2012.04153.x
Fissell, W. H., Manley, S., Westover, A., Humes, H. D., Fleischman, A. J., and Roy, S. (2006). Differentiated growth of human renal tubule cells on thin-film and nanostructured materials. ASAIO J. 52, 221–227. doi:10.1097/01.mat.0000205228.30516.9c
Fissell, W. H., and Roy, S. (2009). The implantable artificial kidney. Semin. Dial. 22, 665–670. doi:10.1111/j.1525-139X.2009.00662.x
Franquesa, M., Flaquer, M., Cruzado, J. M., and Grinyó, J. M. (2013). Kidney regeneration and repair after transplantation. Curr. Opin. Organ Transplant 18, 191–196. doi:10.1097/MOT.0b013e32835f0771
Freytes, D. O., Martin, J., Velankar, S. S., Lee, A. S., and Badylak, S. F. (2008). Preparation and rheological characterization of a gel form of the porcine urinary bladder matrix. Biomaterials 29, 1630–1637. doi:10.1016/j.biomaterials.2007.12.014
Gagliardini, E., Conti, S., Benigni, A., Remuzzi, G., and Remuzzi, A. (2010). Imaging of the porous ultrastructure of the glomerular epithelial filtration slit. J. Am. Soc. Nephrol. 21, 2081–2089. doi:10.1681/ASN.2010020199
Gao, R., Wu, W., Xiang, J., Lv, Y., Zheng, X., Chen, Q., et al. (2015). Hepatocyte culture in autologous decellularized spleen matrix. Organogenesis 11, 16–29. doi:10.1080/15476278.2015.1011908
Garreta, E., Melo, E., Navajas, D., and Farre, R. (2014). Low oxygen tension enhances the generation of lung progenitor cells from mouse embryonic and induced pluripotent stem cells. Physiol. Rep. 2, e12075. doi:10.14814/phy2.12075
Genovese, F., Manresa, A. A., Leeming, D. J., Karsdal, M. A., and Boor, P. (2014). The extracellular matrix in the kidney: a source of novel non-invasive biomarkers of kidney fibrosis? Fibrogenesis Tissue Repair 7, 4. doi:10.1186/1755-1536-7-4
Gessel, M., Spraggins, J. M., Voziyan, P., Hudson, B. G., and Caprioli, R. M. (2015). Decellularization of intact tissue enables MALDI imaging mass spectrometry analysis of the extracellular matrix. J. Mass Spectrom. 50, 1288–1293. doi:10.1002/jms.3696
Gilbert, T. W. (2012). Strategies for tissue and organ decellularization. J. Cell. Biochem. 113, 2217–2222. doi:10.1002/jcb.24130
Gilbert, T. W., Freund, J. M., and Badylak, S. F. (2009). Quantification of DNA in biologic scaffold materials. J. Surg. Res. 152, 135–139. doi:10.1016/j.jss.2008.02.013
Gilbert, T. W., Sellaro, T. L., and Badylak, S. F. (2006). Decellularization of tissues and organs. Biomaterials 27, 3675–3683. doi:10.1016/j.biomaterials.2006.02.014
Gilbert, T. W., Stewart-Akers, A. M., Simmons-Byrd, A., and Badylak, S. F. (2007). Degradation and remodeling of small intestinal submucosa in canine achilles tendon repair. J. Bone Joint Surg. Am. 89, 621–630. doi:10.2106/JBJS.E.00742
Gilbert, T. W., Wognum, S., Joyce, E. M., Freytes, D. O., Sacks, M. S., and Badylak, S. F. (2008). Collagen fiber alignment and biaxial mechanical behavior of porcine urinary bladder derived extracellular matrix. Biomaterials 29, 4775–4782. doi:10.1016/j.biomaterials.2008.08.022
Goh, S. K., Bertera, S., Olsen, P., Candiello, J. E., Halfter, W., Uechi, G., et al. (2013). Perfusion-decellularized pancreas as a natural 3D scaffold for pancreatic tissue and whole organ engineering. Biomaterials 34, 6760–6772. doi:10.1016/j.biomaterials.2013.05.066
Gong, J., Sagiv, O., Cai, H., Tsang, S. H., and Del Priore, L. V. (2008). Effects of extracellular matrix and neighboring cells on induction of human embryonic stem cells into retinal or retinal pigment epithelial progenitors. Exp. Eye Res. 86, 957–965. doi:10.1016/j.exer.2008.03.014
Gorschewsky, O., Klakow, A., Riechert, K., Pitzl, M., and Becker, R. (2005). Clinical comparison of the Tutoplast allograft and autologous patellar tendon (bone-patellar tendon-bone) for the reconstruction of the anterior cruciate ligament: 2- and 6-year results. Am. J. Sports Med. 33, 1202–1209. doi:10.1177/0363546504271510
Gouon-Evans, V. (2014). The race for regeneration: pluripotent-stem-cell-derived 3D kidney structures. Cell Stem Cell 14, 5–6. doi:10.1016/j.stem.2013.12.004
Guan, Y., Liu, S., Liu, Y., Sun, C., Cheng, G., Luan, Y., et al. (2015a). Porcine kidneys as a source of ECM scaffold for kidney regeneration. Mater. Sci. Eng. C 56, 451–456. doi:10.1016/j.msec.2015.07.007
Guan, Y., Liu, S., Sun, C., Cheng, G., Kong, F., Luan, Y., et al. (2015b). The effective bioengineering method of implantation decellularized renal extracellular matrix scaffolds. Oncotarget 6, 36126–36138. doi:10.18632/oncotarget.5304
Habka, D., Mann, D., Landes, R., and Soto-Gutierrez, A. (2015). Future economics of liver transplantation: a 20-year cost modeling forecast and the prospect of bioengineering autologous liver grafts. PLoS ONE 10:e0131764. doi:10.1371/journal.pone.0131764
Hall, I. E., Schröppel, B., Doshi, M. D., Ficek, J., Weng, F. L., Hasz, R. D., et al. (2015). Associations of deceased donor kidney injury with kidney discard and function after transplantation. Am. J. Transplant. 15, 1623–1631. doi:10.1111/ajt.13144
Hammerman, M. R. (2002). Xenotransplantation of developing kidneys. Am. J. Physiol. Renal. Physiol. 283, F601–F606. doi:10.1152/ajprenal.00126.2002
Hanna, J. H., Saha, K., and Jaenisch, R. (2010). Pluripotency and cellular reprogramming: facts, hypotheses, unresolved issues. Cell 143, 508–525. doi:10.1016/j.cell.2010.10.008
Hass, R., Kasper, C., Böhm, S., and Jacobs, R. (2011). Different populations and sources of human mesenchymal stem cells (MSC): a comparison of adult and neonatal tissue-derived MSC. Cell Commun. Signal. 9, 12. doi:10.1186/1478-811X-9-12
Hatano, R., Fujii, E., Segawa, H., Mukaisho, K., Matsubara, M., Miyamoto, K., et al. (2013). Ezrin, a membrane cytoskeletal cross-linker, is essential for the regulation of phosphate and calcium homeostasis. Kidney Int. 83, 41–49. doi:10.1038/ki.2012.308
He, M., and Callanan, A. (2013). Comparison of methods for whole-organ decellularization in tissue engineering of bioartificial organs. Tissue Eng. Part B Rev. 19, 194–208. doi:10.1089/ten.teb.2012.0340
He, M., Callanan, A., Lagaras, K., Steele, J. A. M., and Stevens, M. M. (2016). Optimization of SDS exposure on preservation of ECM characteristics in whole organ decellularization of rat kidneys. J. Biomed. Mater. Res. B Appl. Biomater. 1–9. doi:10.1002/jbm.b.33668
Heilman, R. L., Smith, M. L., Kurian, S. M., Huskey, J., Batra, R. K., Chakkera, H. A., et al. (2015). Transplanting kidneys from deceased donors with severe acute kidney injury. Am. J. Transplant. 15, 2143–2151. doi:10.1111/ajt.13260
Hendry, C. E., and Little, M. H. (2012). Reprogramming the kidney: a novel approach for regeneration. Kidney Int. 82, 138–146. doi:10.1038/ki.2012.68
Hill, R. C., Calle, E. A., Dzieciatkowska, M., Niklason, L. E., and Hansen, K. C. (2015). Quantification of extracellular matrix proteins from a rat lung scaffold to provide a molecular readout for tissue engineering. Mol. Cell. Proteomics 1, 961–973. doi:10.1074/mcp.M114.045260
Hodde, J., and Hiles, M. (2002). Virus safety of a porcine-derived medical device: evaluation of a viral inactivation method. Biotechnol. Bioeng. 79, 211–216. doi:10.1002/bit.10281
Hollister, S. J. (2005). Porous scaffold design for tissue engineering. Nat. Mater. 4, 518–524. doi:10.1038/nmat1421
Howard, R. J., Schold, J. D., and Cornell, D. L. (2005). A 10-year analysis of organ donation after cardiac death in the United States. Transplantation 80, 564–568. doi:10.1097/01.tp.0000168156.79847.46
Hutton, J. (1999). The economics of immunosuppression in renal transplantation: a review of recent literature. Transplant. Proc. 31, 1328–1332. doi:10.1016/S0041-1345(98)02017-X
Hynes, R. O. (2009). The extracellular matrix: not just pretty fibrils. Science 326, 1216–1219. doi:10.1126/science.1176009
Jha, V., Garcia-Garcia, G., Iseki, K., Li, Z., Naicker, S., Plattner, B., et al. (2013). Chronic kidney disease: global dimension and perspectives. Lancet 382, 260–272. doi:10.1016/S0140-6736(13)60687-X
Johnson, D. W., Herzig, K., Purdie, D., Brown, A. M., Rigby, R. J., Nicol, D. L., et al. (2000). A comparison of the effects of dialysis and renal transplantation on the survival of older uremic patients. Transplantation 69, 794–799. doi:10.1097/00007890-200003150-00020
Johnson, L. B., Kuo, P. C., Schweitzer, E. J., Ratner, L. E., Klassen, D. K., Hoehn-Saric, E. W., et al. (1996). Double renal allografts successfully increase utilization of kidneys from older donors within a single organ procurement organization. Transplantation 62, 1581–1583. doi:10.1097/00007890-199612150-00009
Kabsch, W., Mannherz, H. G., Suck, D., Pai, E. F., and Holmes, K. C. (1990). Atomic structure of the actin:DNase I complex. Nature 347, 37–44. doi:10.1038/347037a0
Kayler, L. K., Garzon, P., Magliocca, J., Fujita, S., Kim, R. D., Hemming, A. W., et al. (2009). Outcomes and utilization of kidneys from deceased donors with acute kidney injury. Am. J. Transplant. 9, 367–373. doi:10.1111/j.1600-6143.2008.02505.x
Kayler, L. K., Magliocca, J., Zendejas, I., Srinivas, T. R., and Schold, J. D. (2011). Impact of cold ischemia time on graft survival among ECD transplant recipients: a paired kidney analysis. Am. J. Transplant. 11, 2647–2656. doi:10.1111/j.1600-6143.2011.03741.x
Keane, T. J., Londono, R., Turner, N. J., and Badylak, S. F. (2012). Consequences of ineffective decellularization of biologic scaffolds on the host response. Biomaterials 33, 1771–1781. doi:10.1016/j.biomaterials.2011.10.054
Keller, G. (2005). Embryonic stem cell differentiation: emergence of a new era in biology and medicine. Genes Dev. 19, 1129–1155. doi:10.1101/gad.1303605
Kim, S. M., Ahn, S., Min, S. I., Park, D., Park, T., Min, S. K., et al. (2013). Cold ischemic time is critical in outcomes of expanded criteria donor renal transplantation. Clin. Transplant. 27, 132–139. doi:10.1111/ctr.12034
Klein, A. S., Messersmith, E. E., Ratner, L. E., Kochik, R., Baliga, P. K., and Ojo, A. O. (2010). Organ donation and utilization in the United States, 1999-2008. Am. J. Transplant. 10, 973–986. doi:10.1111/j.1600-6143.2009.03008.x
Kooman, J. P., Joles, J. A., and Gerritsen, K. G. (2015). Creating a wearable artificial kidney: where are we now? Expert Rev. Med. Devices 12, 373–376. doi:10.1586/17434440.2015.1053466
Krause, D., and Cantley, L. G. (2005). Bone marrow plasticity revisited: protection or differentiation in the kidney tubule? J. Clin. Invest. 115, 1705–1708. doi:10.1172/JCI25540
Lai, C.-F., Tsai, H.-B., Hsu, S.-H., Chiang, C.-K., Huang, J.-W., and Huang, S.-J. (2013). Withdrawal from long-term hemodialysis in patients with end-stage renal disease in Taiwan. J. Formos. Med. Assoc. 112, 589–599. doi:10.1016/j.jfma.2013.04.009
Levey, A. S., and Coresh, J. (2012). Chronic kidney disease. Lancet 379, 165–180. doi:10.1016/S0140-6736(11)60178-5
Li, B., Cohen, A., Hudson, T. E., Motlagh, D., Amrani, D. L., and Duffield, J. S. (2010). Mobilized human hematopoietic stem/progenitor cells promote kidney repair after ischemia/reperfusion injury. Circulation 121, 2211–2220. doi:10.1161/CIRCULATIONAHA.109.928796
Liao, J., Joyce, E. M., and Sacks, M. S. (2008). Effects of decellularization on the mechanical and structural properties of the porcine aortic valve leaflet. Biomaterials 29, 1065–1074. doi:10.1016/j.biomaterials.2007.11.007
Lih, E., Park, K. W., Chun, S. Y., Kim, H., Kwon, T. G., Joung, Y. K., et al. (2016). Biomimetic porous PLGA scaffolds incorporating decellularized extracellular matrix for kidney tissue regeneration. ACS Appl. Mater. Interfaces 8, 21145–21154. doi:10.1021/acsami.6b03771
Lin, Y.-Q., Wang, L.-R., Pan, L.-L., Wang, H., Zhu, G.-Q., Liu, W.-Y., et al. (2016). Kidney bioengineering in regenerative medicine: an emerging therapy for kidney disease. Cytotherapy 18, 186–197. doi:10.1016/j.jcyt.2015.10.004
Little, M. H., Brennan, J., Georgas, K., Davies, J. A., Davidson, D. R., Baldock, R. A., et al. (2007). A high-resolution anatomical ontology of the developing murine genitourinary tract. Gene Expr. Patterns 7, 680–699. doi:10.1016/j.modgep.2007.03.002
Liu, R., Gao, J., Yang, Y., and Zeng, W. (2015a). Preparation of rat whole-kidney acellular matrix via peristaltic pump. Urol J 12, 2457–2461.
Liu, J., Yu, F., Sun, Y., Jiang, B., Zhang, W., Yang, J., et al. (2015b). Concise reviews: characteristics and potential applications of human dental tissue-derived mesenchymal stem cells. Stem Cells 33, 627–638. doi:10.1002/stem.1909
Lu, H., Hoshiba, T., Kawazoe, N., Koda, I., Song, M., and Chen, G. (2011). Cultured cell-derived extracellular matrix scaffolds for tissue engineering. Biomaterials 32, 9658–9666. doi:10.1016/j.biomaterials.2011.08.091
Mae, S.-I., Shono, A., Shiota, F., Yasuno, T., Kajiwara, M., Gotoda-Nishimura, N., et al. (2013). Monitoring and robust induction of nephrogenic intermediate mesoderm from human pluripotent stem cells. Nat. Commun. 4, 1367. doi:10.1038/ncomms2378
Matas, A. J., Smith, J. M., Skeans, M. A., Thompson, B., Gustafson, S. K., Stewart, D. E., et al. (2015). OPTN/SRTR 2013 Annual Data Report: kidney. Am. J. Transplant. 15(Suppl. 2), 1–34. doi:10.1111/ajt.13195
Matthiesen, T., Ott, H. C., Goh, S.-K., Kren, S. M., and Taylor, D. A. (2007). Abstract 428: creating biocompatible 3-D scaffolds for engineering cardiovascular tissues: heart, lung, and kidney. Circulation 116, II_70–c.
Maunsbach, A. B. (1966). Observations on the segmentation of the proximal tubule in the rat kidney. J. Ultrastruct. Res. 16, 239–258. doi:10.1016/S0022-5320(66)80019-9
McKee, R., and Wingert, R. (2016). Repopulating decellularized kidney scaffolds: an avenue for ex vivo organ generation. Materials (Basel) 9, 190. doi:10.3390/ma9030190
Mecham, R. P. (2001). “Overview of extracellular matrix,” in Current Protocols in Cell Biology. Hoboken, NJ: John Wiley & Sons, Inc., Unit 10.1.
Merion, R. M., Ashby, V. B., Wolfe, R. A., Distant, D. A., Hulbert-Shearon, T. E., Metzger, R. A., et al. (2005). Deceased-donor characteristics and the survival benefit of kidney transplantation. JAMA 294, 2726–2733. doi:10.1001/jama.294.21.2726
Mirmalek-Sani, S.-H., Sullivan, D. C., Zimmerman, C., Shupe, T. D., and Petersen, B. E. (2013). Immunogenicity of decellularized porcine liver for bioengineered hepatic tissue. Am. J. Pathol. 183, 558–565. doi:10.1016/j.ajpath.2013.05.002
Moini, M., Schilsky, M. L., and Tichy, E. M. (2015). Review on immunosuppression in liver transplantation. World J. Hepatol. 7, 1355–1368. doi:10.4254/wjh.v7.i10.1355
Moldovan, G.-L., Pfander, B., and Jentsch, S. (2007). PCNA, the maestro of the replication fork. Cell 129, 665–679. doi:10.1016/j.cell.2007.05.003
Moroni, F., and Mirabella, T. (2014). Decellularized matrices for cardiovascular tissue engineering. Am. J. Stem Cells 3, 1–20.
Murray, C. J. L., Abraham, J., Ali, M. K., Alvarado, M., Atkinson, C., Baddour, L. M., et al. (2013). The state of US health, 1990-2010: burden of diseases, injuries, and risk factors. JAMA 310, 591–608. doi:10.1001/jama.2013.13805
Naba, A., Clauser, K. R., Hoersch, S., Liu, H., Carr, S. A., and Hynes, R. O. (2012). The matrisome: in silico definition and in vivo characterization by proteomics of normal and tumor extracellular matrices. Mol. Cell. Proteomics 11, M111.014647. doi:10.1074/mcp.M111.014647
Nagata, S., Hanayama, R., and Kawane, K. (2010). Autoimmunity and the clearance of dead cells. Cell 140, 619–630. doi:10.1016/j.cell.2010.02.014
Nakayama, K. H., Batchelder, C. A., Lee, C. I., and Tarantal, A. F. (2010). Decellularized rhesus monkey kidney as a three-dimensional scaffold for renal tissue engineering. Tissue Eng. Part A 16, 2207–2216. doi:10.1089/ten.TEA.2009.0602
Nakayama, K. H., Batchelder, C. A., Lee, C. I., and Tarantal, A. F. (2011). Renal tissue engineering with decellularized rhesus monkey kidneys: age-related differences. Tissue Eng. Part A 17, 2891–2901. doi:10.1089/ten.TEA.2010.0714
Nakayama, K. H., Lee, C. C. I., Batchelder, C. A., and Tarantal, A. F. (2013). Tissue specificity of decellularized rhesus monkey kidney and lung scaffolds. PLoS ONE 8:e64134. doi:10.1371/journal.pone.0064134
Naranjo, T. Á., Noguera-Salvá, R., and Fariñas Guerrero, F. (2009). Extracellular matrix: morphology, function and biotensegrity (part I). Rev. Esp. Patol. 42, 249–261.
Narayanan, K., Leck, K.-J., Gao, S., and Wan, A. C. A. (2009). Three-dimensional reconstituted extracellular matrix scaffolds for tissue engineering. Biomaterials 30, 4309–4317. doi:10.1016/j.biomaterials.2009.04.049
National Kidney and Urologic Diseases Information Clearinghouse. (2012). Kidney disease statistics for the United States. Natl. Inst. Health 12, 1–16.
Ng, S. L. J., Narayanan, K., Gao, S., and Wan, A. C. A. (2011). Lineage restricted progenitors for the repopulation of decellularized heart. Biomaterials 32, 7571–7580. doi:10.1016/j.biomaterials.2011.06.065
Nguyen, D. M., and El-Serag, H. B. (2010). The epidemiology of obesity. Gastroenterol. Clin. North Am. 39, 1–7. doi:10.1016/j.gtc.2009.12.014
Nishinakamura, R. (2008). Stem cells in the embryonic kidney. Kidney Int. 73, 913–917. doi:10.1038/sj.ki.5002784
Nowacki, M., Kloskowski, T., Pokrywczyńska, M., Nazarewski, L., Jundziłł, A., Pietkun, K., et al. (2014). Is regenerative medicine a new hope for kidney replacement? J. Artif. Organs 17, 123–134. doi:10.1007/s10047-014-0767-z
Nygard, A.-B., Jørgensen, C. B., Cirera, S., and Fredholm, M. (2007). Selection of reference genes for gene expression studies in pig tissues using SYBR green qPCR. BMC Mol. Biol. 8:67. doi:10.1186/1471-2199-8-67
O’Brien, F. J. (2011). Biomaterials & scaffolds for tissue engineering. Mater. Today 14, 88–95. doi:10.1016/S1369-7021(11)70058-X
Ofenbauer, A., Sebinger, D. D. R., Prewitz, M., Gruber, P., and Werner, C. (2015). Dewaxed ECM: a simple method for analyzing cell behaviour on decellularized extracellular matrices. J. Tissue Eng. Regen. Med. 9, 1046–1055. doi:10.1002/term.1658
Ojo, A. O., Hanson, J. A., Meier-Kriesche, H., Okechukwu, C. N., Wolfe, R. A., Leichtman, A. B., et al. (2001). Survival in recipients of marginal cadaveric donor kidneys compared with other recipients and wait-listed transplant candidates. J. Am. Soc. Nephrol. 12, 589–597.
Orandi, B. J., Luo, X., Massie, A. B., Garonzik-Wang, J. M., Lonze, B. E., Ahmed, R., et al. (2016). Survival benefit with kidney transplants from HLA-incompatible live donors. N. Engl. J. Med. 374, 940–950. doi:10.1056/NEJMoa1508380
Orlando, G., Booth, C., Wang, Z., Totonelli, G., Ross, C. L., Moran, E., et al. (2013). Discarded human kidneys as a source of ECM scaffold for kidney regeneration technologies. Biomaterials 34, 5915–5925. doi:10.1016/j.biomaterials.2013.04.033
Orlando, G., Farney, A. C., Iskandar, S. S., Mirmalek-Sani, S.-H., Sullivan, D. C., Moran, E., et al. (2012). Production and implantation of renal extracellular matrix scaffolds from porcine kidneys as a platform for renal bioengineering investigations. Ann. Surg. 256, 363–370. doi:10.1097/SLA.0b013e31825a02ab
Orlando, G., Wood, K. J., Stratta, R. J., Yoo, J. J., Atala, A., and Soker, S. (2011a). Regenerative medicine and organ transplantation: past, present, and future. Transplantation 91, 1310–1317. doi:10.1097/TP.0b013e318219ebb5
Orlando, G., Baptista, P., Birchall, M., De Coppi, P., Farney, A., Guimaraes-Souza, N. K., et al. (2011b). Regenerative medicine as applied to solid organ transplantation: current status and future challenges. Transpl. Int. 24, 223–232. doi:10.1111/j.1432-2277.2010.01182.x
Ott, H. C., Clippinger, B., Conrad, C., Schuetz, C., Pomerantseva, I., Ikonomou, L., et al. (2010). Regeneration and orthotopic transplantation of a bioartificial lung. Nat. Med. 16, 927–933. doi:10.1038/nm.2193
Ott, H. C., Matthiesen, T. S., Goh, S.-K., Black, L. D., Kren, S. M., Netoff, T. I., et al. (2008). Perfusion-decellularized matrix: using nature’s platform to engineer a bioartificial heart. Nat. Med. 14, 213–221. doi:10.1038/nm1684
Pannabecker, T. L. (2012). Structure and function of the thin limbs of the loop of henle. Compr. Physiol. 2, 2063–2086. doi:10.1002/cphy.c110019
Park, K. M., and Woo, H. M. (2012). Porcine bioengineered scaffolds as new frontiers in regenerative medicine. Transplant. Proc. 44, 1146–1150. doi:10.1016/j.transproceed.2012.03.043
Pascual, J., Zamora, J., and Pirsch, J. D. (2008). A systematic review of kidney transplantation from expanded criteria donors. Am. J. Kidney Dis. 52, 553–586. doi:10.1053/j.ajkd.2008.06.005
Peloso, A., Katari, R., Patel, T., Hemal, S., Zambon, J. P., Salvatori, M., et al. (2013). Considerations on the development of a model of kidney bioengineering and regeneration in rats. Expert Rev. Med. Devices 10, 597–601. doi:10.1586/17434440.2013.827528
Peloso, A., Petrosyan, A., Da Sacco, S., Booth, C., Zambon, J. P., O’Brien, T., et al. (2015). Renal extracellular matrix scaffolds from discarded kidneys maintain glomerular morphometry and vascular resilience and retains critical growth factors. Transplantation 99, 1807–1816. doi:10.1097/TP.0000000000000811
Peloso, A., Tamburrini, R., Edgar, L., Wilm, B., Katari, R., Perin, L., et al. (2016). Extracellular matrix scaffolds as a platform for kidney regeneration. Eur. J. Pharmacol. 790, 21–27. doi:10.1016/j.ejphar.2016.07.038
Petersen, T. H., Calle, E. A., Colehour, M. B., and Niklason, L. E. (2012). Matrix composition and mechanics of decellularized lung scaffolds. Cells Tissues Organs 195, 222–231. doi:10.1159/000324896
Petrosyan, A., Orlando, G., Peloso, A., Wang, Z., Farney, A. C., Rogers, J., et al. (2015). Understanding the bioactivity of stem cells seeded on extracellular matrix scaffolds produced from discarded human kidneys: a critical step towards a new generation bio-artificial kidney. Issues 1. Available at: http://www.researchgate.net/profile/Astgik_Petrosyan/publication/273451480_Understanding_the_bioactivity_of_stem_cells_seeded_on_extracellular_matrix_scaffolds_produced_
from_discarded_human_kidneys_a_critical_step_towards_a_new_generation_bio-artificial_k
Poornejad, N., Frost, T. S., Scott, D. R., Elton, B. B., Reynolds, P. R., Roeder, B. L., et al. (2015). Freezing/thawing without cryoprotectant damages native but not decellularized porcine renal tissue. Organogenesis 11, 30–45. doi:10.1080/15476278.2015.1022009
Poornejad, N., Momtahan, N., Salehi, A. S. M., Scott, D. R., Fronk, C. A., Roeder, B. L., et al. (2016a). Efficient decellularization of whole porcine kidneys improves reseeded cell behavior. Biomed. Mater. 11, 25003. doi:10.1088/1748-6041/11/2/025003
Poornejad, N., Schaumann, L. B., Buckmiller, E. M., Momtahan, N., Gassman, J. R., Ma, H. H., et al. (2016b). The impact of decellularization agents on renal tissue extracellular matrix. J. Biomater. Appl. 31, 521–533. doi:10.1177/0885328216656099
Poornejad, N., Nielsen, J. J., Morris, R. J., Gassman, J. R., Reynolds, P. R., Roeder, B. L., et al. (2016c). Comparison of four decontamination treatments on porcine renal decellularized extracellular matrix structure, composition, and support of human renal cortical tubular epithelium cells. J. Biomater. Appl. 30, 1154–1167. doi:10.1177/0885328215615760
Port, F. K., Bragg-Gresham, J. L., Metzger, R. A., Dykstra, D. M., Gillespie, B. W., Young, E. W., et al. (2002). Donor characteristics associated with reduced graft survival: an approach to expanding the pool of kidney donors. Transplantation 74, 1281–1286. doi:10.1097/01.TP.0000034060.18738.0B
Price, A. P., England, K. A., Matson, A. M., Blazar, B. R., and Panoskaltsis-Mortari, A. (2010). Development of a decellularized lung bioreactor system for bioengineering the lung: the matrix reloaded. Tissue Eng. Part A 16, 2581–2591. doi:10.1089/ten.tea.2009.0659
Qiu, Q.-Q., Leamy, P., Brittingham, J., Pomerleau, J., Kabaria, N., and Connor, J. (2009). Inactivation of bacterial spores and viruses in biological material using supercritical carbon dioxide with sterilant. J. Biomed. Mater. Res. Part B Appl. Biomater. 91, 572–578. doi:10.1002/jbm.b.31431
Rafighdoust, A., Shahri, N. M., and Baharara, J. (2015). Decellularized kidney in the presence of chondroitin sulfate as a natural 3D scaffold for stem cells. Iran J. Basic Med.Sci. 18, 788–798.
Ramage, L. (2011). Integrins and extracellular matrix in mechanotransduction. Cell Health Cytoskeleton 4, 1. doi:10.2147/CHC.S21829
Rana, A., Gruessner, A., Agopian, V. G., Khalpey, Z., Riaz, I. B., Kaplan, B., et al. (2015). Survival benefit of solid-organ transplant in the United States. JAMA Surg. 150, 252–259. doi:10.1001/jamasurg.2014.2038
Rana, D., Zreiqat, H., Benkirane-Jessel, N., Ramakrishna, S., and Ramalingam, M. (2017). Development of decellularized scaffolds for stem cell-driven tissue engineering. J. Tissue Eng. Regen. Med. 11, 942–965. doi:10.1002/term.2061
Randles, M., Humphries, M. J., and Lennon, R. (2017). Proteomic definitions of basement membrane composition in health and disease. Matrix Biol. 57–58, 12–28. doi:10.1016/j.matbio.2016.08.006
Reing, J. E., Brown, B. N., Daly, K. A., Freund, J. M., Gilbert, T. W., Hsiong, S. X., et al. (2010). The effects of processing methods upon mechanical and biologic properties of porcine dermal extracellular matrix scaffolds. Biomaterials 31, 8626–8633. doi:10.1016/j.biomaterials.2010.07.083
Ren, X., Tapias, L. F., Jank, B. J., Mathisen, D. J., Lanuti, M., and Ott, H. C. (2015). Ex vivo non-invasive assessment of cell viability and proliferation in bio-engineered whole organ constructs. Biomaterials 52, 103–112. doi:10.1016/j.biomaterials.2015.01.061
Reticker-Flynn, N. E., Malta, D. F. B., Winslow, M. M., Lamar, J. M., Xu, M. J., Underhill, G. H., et al. (2012). A combinatorial extracellular matrix platform identifies cell-extracellular matrix interactions that correlate with metastasis. Nat. Commun. 3, 1122. doi:10.1038/ncomms2128
Rogers, I. (2011). Induced pluripotent stem cells from human kidney. J. Am. Soc. Nephrol. 22, 1179–1180. doi:10.1681/ASN.2011050501
Roodnat, J. I., Mulder, P. G. H., Van Riemsdijk, I. C., IJzermans, J. N. M., van Gelder, T., and Weimar, W. (2003). Ischemia times and donor serum creatinine in relation to renal graft failure. Transplantation 75, 799–804. doi:10.1097/01.TP.0000056632.00848.8D
Rosario, D. J., Reilly, G. C., Ali Salah, E., Glover, M., Bullock, A. J., and Macneil, S. (2008). Decellularization and sterilization of porcine urinary bladder matrix for tissue engineering in the lower urinary tract. Regen. Med. 3, 145–156. doi:10.2217/17460751.3.2.145
Rose, W., Wood, J. D., Simmons-byrd, A., and Spievack, A. R. (2009). Effect of a xenogeneic urinary bladder injectable bioscaffold on lameness in dogs with osteoarthritis of the coxofemoral joint (Hip): a randomized, double blinded controlled trial. Int. J. Appl. Res. Vet. Med. 7, 13–22.
Ross, E. A. (2009). “Kidney regeneration using stem cells and acellular whole organ scaffolds: perspective and recent developments,” in World Congress on Medical Physics and Biomedical Engineering, September 7–12, 2009, Munich, Germany, 936–939.
Ross, E. A., Abrahamson, D. R., St John, P., Clapp, W. L., Williams, M. J., Terada, N., et al. (2012). Mouse stem cells seeded into decellularized rat kidney scaffolds endothelialize and remodel basement membranes. Organogenesis 8, 49–55. doi:10.4161/org.20209
Ross, E. A., Williams, M. J., Hamazaki, T., Terada, N., Clapp, W. L., Adin, C., et al. (2009). Embryonic stem cells proliferate and differentiate when seeded into kidney scaffolds. J. Am. Soc. Nephrol. 20, 2338–2347. doi:10.1681/ASN.2008111196
Roy, S., Goldman, K., Marchant, R., Zydney, A., Brown, D., Fleischman, A., et al. (2011). Implanted renal replacement for end-stage renal disease. Panminerva Med. 53, 155–166.
Sambi, M., Chow, T., Whiteley, J., Li, M., Chua, S., Raileanu, V., et al. (2017). Acellular mouse kidney ECM can be used as a three-dimensional substrate to test the differentiation potential of embryonic stem cell derived renal progenitors. Stem Cell Rev. doi:10.1007/s12015-016-9712-2
Scarritt, M. E., Pashos, N. C., and Bunnell, B. A. (2015). A review of cellularization strategies for tissue engineering of whole organs. Front. Bioeng. Biotechnol. 3:43. doi:10.3389/fbioe.2015.00043
Shupe, T., Williams, M., Brown, A., Willenberg, B., and Petersen, B. E. (2010). Method for the decellularization of intact rat liver. Organogenesis 6, 134–136. doi:10.4161/org.6.2.11546
Singh, P., Carraher, C., and Schwarzbauer, J. E. (2010). Assembly of fibronectin extracellular matrix. Annu. Rev. Cell Dev. Biol. 26, 397–419. doi:10.1146/annurev-cellbio-100109-104020
Song, B., Smink, A. M., Jones, C. V., Callaghan, J. M., Firth, S. D., Bernard, C. A., et al. (2012). The directed differentiation of human iPS cells into kidney podocytes. PLoS ONE 7:e46453. doi:10.1371/journal.pone.0046453
Song, J. J., Guyette, J. P., Gilpin, S. E., Gonzalez, G., Vacanti, J. P., and Ott, H. C. (2013). Regeneration and experimental orthotopic transplantation of a bioengineered kidney. Nat. Med. 19, 646–651. doi:10.1038/nm.3154
Song, J. J., and Ott, H. C. (2011). Organ engineering based on decellularized matrix scaffolds. Trends Mol. Med. 17, 424–432. doi:10.1016/j.molmed.2011.03.005
Stratta, R. J., Rohr, M. S., Sundberg, A. K., Farney, A. C., Hartmann, E. L., Moore, P. S., et al. (2006). Intermediate-term outcomes with expanded criteria deceased donors in kidney transplantation: a spectrum or specter of quality? Ann. Surg. 243, 594–601; discussion 601–603. doi:10.1097/01.sla.0000216302.43776.1a
Sugawara, M., Ichimura, S., Kokubo, K., Shimbo, T., Hirose, M., Kobayashi, H., et al. (2011). Transplantation: basic science and immune-tolerance. Clin. Kidney J. 4, 4.s2.42. doi:10.1093/ndtplus/4.s2.42
Suh, J. H., and Miner, J. H. (2013). The glomerular basement membrane as a barrier to albumin. Nat. Rev. Nephrol. 9, 470–477. doi:10.1038/nrneph.2013.109
Sullivan, D. C., Mirmalek-Sani, S.-H., Deegan, D. B., Baptista, P. M., Aboushwareb, T., Atala, A., et al. (2012). Decellularization methods of porcine kidneys for whole organ engineering using a high-throughput system. Biomaterials 33, 7756–7764. doi:10.1016/j.biomaterials.2012.07.023
Sun, W. Q., and Leung, P. (2008). Calorimetric study of extracellular tissue matrix degradation and instability after gamma irradiation. Acta Biomater. 4, 817–826. doi:10.1016/j.actbio.2008.02.006
Swetha, G., Chandra, V., Phadnis, S., and Bhonde, R. (2011). Glomerular parietal epithelial cells of adult murine kidney undergo EMT to generate cells with traits of renal progenitors. J. Cell. Mol. Med. 15, 396–413. doi:10.1111/j.1582-4934.2009.00937.x
Taguchi, A., Kaku, Y., Ohmori, T., Sharmin, S., Ogawa, M., Sasaki, H., et al. (2014). Redefining the in vivo origin of metanephric nephron progenitors enables generation of complex kidney structures from pluripotent stem cells. Cell Stem Cell 14, 53–67. doi:10.1016/j.stem.2013.11.010
Taguchi, A., and Nishinakamura, R. (2014). Nephron reconstitution from pluripotent stem cells. Kidney Int. 87, 1–7. doi:10.1038/ki.2014.358
Tanemoto, M., Toyohara, T., Abe, T., and Ito, S. (2008). MAGI-1a functions as a scaffolding protein for the distal renal tubular basolateral K+ channels. J. Biol. Chem. 283, 12241–12247. doi:10.1074/jbc.M707738200
Teodori, L., Costa, A., Marzio, R., Perniconi, B., Coletti, D., Adamo, S., et al. (2014). Native extracellular matrix: a new scaffolding platform for repair of damaged muscle. Front. Physiol. 5:218. doi:10.3389/fphys.2014.00218
Tien, J., and Nelson, C. M. (2014). Microstructured extracellular matrices in tissue engineering and development: an update. Ann. Biomed. Eng. 42, 1413–1423. doi:10.1007/s10439-013-0912-5
Tonelli, M., Wiebe, N., Knoll, G., Bello, A., Browne, S., Jadhav, D., et al. (2011). Systematic review: kidney transplantation compared with dialysis in clinically relevant outcomes. Am. J. Transplant. 11, 2093–2109. doi:10.1111/j.1600-6143.2011.03686.x
Ullrich, K. J. (1979). Sugar, amino acid, and NA+ cotransport in the proximal tubule. Available at: http://www.annualreviews.org/doi/pdf/10.1146/annurev.ph.41.030179.001145
United States Renal Data System. (2015). CKD in the United States: an overview of the USRDS Annual Data Report, volume 1. Am. J. Kidney Dis. 66, S1–S10. doi:10.1053/j.ajkd.2015.04.017
USRDS. (2016). Chapter 7: transplantation. Am. J. Kidney Dis. 67, S227–S238. doi:10.1053/j.ajkd.2016.02.018
Usui, J., Kobayashi, T., Yamaguchi, T., Knisely, A. S., Nishinakamura, R., and Nakauchi, H. (2012). Generation of kidney from pluripotent stem cells via blastocyst complementation. Am. J. Pathol. 180, 2417–2426. doi:10.1016/j.ajpath.2012.03.007
Uygun, B. E., Soto-Gutierrez, A., Yagi, H., Izamis, M.-L., Guzzardi, M. A., Shulman, C., et al. (2010). Organ reengineering through development of a transplantable recellularized liver graft using decellularized liver matrix. Nat. Med. 16, 814–820. doi:10.1038/nm.2170
Uzarski, J. S., Bijonowski, B. M., Wang, B., Ward, H. H., Wandinger-Ness, A., Miller, W. M., et al. (2015). Dual-purpose bioreactors to monitor noninvasive physical and biochemical markers of kidney and liver scaffold recellularization. Tissue Eng. Part C Methods 21, 1032–1043. doi:10.1089/ten.tec.2014.0665
Uzarski, J. S., Xia, Y., Belmonte, J. C. I., and Wertheim, J. A. (2014). New strategies in kidney regeneration and tissue engineering. Curr. Opin. Nephrol. Hypertens. 23, 399–405. doi:10.1097/01.mnh.0000447019.66970.ea
Valapour, M., Skeans, M. A., Heubner, B. M., Smith, J. M., Schnitzler, M. A., Hertz, M. I., et al. (2014). OPTN/SRTR 2012 Annual Data Report: kidney. Am. J. Transplant. 14, 11–44. doi:10.1111/ajt.12579
Vavken, P., Joshi, S., and Murray, M. M. (2009). TRITON-X is most effective among three decellularization agents for ACL tissue engineering. J. Orthop. Res. 27, 1612–1618. doi:10.1002/jor.20932
Vishwakarma, S. K., Bhavani, P. G., Bardia, A., Abkari, A., Murthy, G. S., Venkateshwarulu, J., et al. (2014). Preparation of natural three-dimensional goat kidney scaffold for the development of bioartificial organ. Indian J. Nephrol. 24, 372–375. doi:10.4103/09714065.133008
Vogetseder, A., Picard, N., Gaspert, A., Walch, M., Kaissling, B., and Le Hir, M. (2008). Proliferation capacity of the renal proximal tubule involves the bulk of differentiated epithelial cells. Am. J. Physiol. Cell Physiol. 294, C22–C28. doi:10.1152/ajpcell.00227.2007
Vorotnikova, E., McIntosh, D., Dewilde, A., Zhang, J., Reing, J. E., Zhang, L., et al. (2010). Extracellular matrix-derived products modulate endothelial and progenitor cell migration and proliferation in vitro and stimulate regenerative healing in vivo. Matrix Biol. 29, 690–700. doi:10.1016/j.matbio.2010.08.007
Wainwright, J. M., Czajka, C. A., Patel, U. B., Freytes, D. O., Tobita, K., Gilbert, T. W., et al. (2010). Preparation of cardiac extracellular matrix from an intact porcine heart. Tissue Eng. Part C Methods 16, 525–532. doi:10.1089/ten.tec.2009.0392
Wallis, J. M., Borg, Z. D., Daly, A. B., Deng, B., Ballif, B. A., Allen, G. B., et al. (2012). Comparative assessment of detergent-based protocols for mouse lung de-cellularization and re-cellularization. Tissue Eng. Part C Methods 18, 420–432. doi:10.1089/ten.TEC.2011.0567
Wang, Y., Bao, J., Wu, Q., Zhou, Y., Li, Y., Wu, X., et al. (2014). Method for perfusion decellularization of porcine whole liver and kidney for use as a scaffold for clinical-scale bioengineering engrafts. Xenotransplantation. Available at: http://www.ncbi.nlm.nih.gov/pubmed/25291435
Wen, D., Ni, L., You, L., Zhang, L., Gu, Y., Hao, C.-M., et al. (2012). Upregulation of nestin in proximal tubules may participate in cell migration during renal repair. Am. J. Physiol. Renal. Physiol. 303, F1534–F1544. doi:10.1152/ajprenal.00083.2012
Willenberg, B. J., Oca-Cossio, J., Cai, Y., Brown, A. R., Clapp, W. L., Abrahamson, D. R., et al. (2015). Repurposed biological scaffolds: kidney to pancreas. Organogenesis 11, 47–57. doi:10.1080/15476278.2015.1067354
Wolfe, R. A., Ashby, V. B., Milford, E. L., Ojo, A. O., Ettenger, R. E., Agodoa, L. Y. C., et al. (1999). Comparison of mortality in all patients on dialysis, patients on dialysis awaiting transplantation, and recipients of a first cadaveric transplant. N. Engl. J. Med. 341, 1725–1730. doi:10.1056/NEJM199912023412303
Wolfe, R. A., Roys, E. C., and Merion, R. M. (2010). Trends in organ donation and transplantation in the United States, 1999-2008. Am. J. Transplant. 10, 961–972. doi:10.1111/j.1600-6143.2010.03021.x
Woods, T., and Gratzer, P. F. (2005). Effectiveness of three extraction techniques in the development of a decellularized bone-anterior cruciate ligament-bone graft. Biomaterials 26, 7339–7349. doi:10.1016/j.biomaterials.2005.05.066
Xia, Y., Friedmann, P., Cortes, C. M., Lubetzky, M. L., and Kayler, L. K. (2015). Influence of cold ischemia time in combination with donor acute kidney injury on kidney transplantation outcomes. J. Am. Coll. Surg. 221, 532–538. doi:10.1016/j.jamcollsurg.2015.05.003
Xu, H., Xu, B., Yang, Q., Li, X., Ma, X., Xia, Q., et al. (2014). Comparison of decellularization protocols for preparing a decellularized porcine annulus fibrosus scaffold. PLoS ONE 9:e86723. doi:10.1371/journal.pone.0086723
Yagi, H., Fukumitsu, K., Fukuda, K., Kitago, M., Shinoda, M., Obara, H., et al. (2013). Human-scale whole-organ bioengineering for liver transplantation: a regenerative medicine approach. Cell Transplant. 22, 231–242. doi:10.3727/096368912X654939
Yamanaka, S., and Blau, H. M. (2010). Nuclear reprogramming to a pluripotent state by three approaches. Nature 465, 704–712. doi:10.1038/nature09229
Yokoo, T., and Kawamura, T. (2009). Xenobiotic kidney organogenesis: a new avenue for renal transplantation. J. Nephrol. 22, 312–317.
Yokote, S., Yamanaka, S., and Yokoo, T. (2012). De novo kidney regeneration with stem cells. J. Biomed. Biotechnol. 2012, 453519. doi:10.1155/2012/453519
Yu, Y. L., Shao, Y. K., Ding, Y. Q., Lin, K. Z., Chen, B., Zhang, H. Z., et al. (2014). Decellularized kidney scaffold-mediated renal regeneration. Biomaterials 35, 6822–6828. doi:10.1016/j.biomaterials.2014.04.074
Keywords: kidney transplantation, bioengineering, decellularization, regenerative medicine, stem cell
Citation: Destefani AC, Sirtoli GM and Nogueira BV (2017) Advances in the Knowledge about Kidney Decellularization and Repopulation. Front. Bioeng. Biotechnol. 5:34. doi: 10.3389/fbioe.2017.00034
Received: 18 January 2017; Accepted: 03 May 2017;
Published: 01 June 2017
Edited by:
Paul De Vos, University Medical Center Groningen, NetherlandsReviewed by:
Martin (Marco) Harmsen, University Medical Center Groningen, NetherlandsEmilio Isaac Alarcon, University of Ottawa, Canada
Copyright: © 2017 Destefani, Sirtoli and Nogueira. This is an open-access article distributed under the terms of the Creative Commons Attribution License (CC BY). The use, distribution or reproduction in other forums is permitted, provided the original author(s) or licensor are credited and that the original publication in this journal is cited, in accordance with accepted academic practice. No use, distribution or reproduction is permitted which does not comply with these terms.
*Correspondence: Breno Valentim Nogueira, YnJlbm92YWxlbnRpbUBnbWFpbC5jb20=
†These authors have contributed equally to this work.