- Institute of Biochemical Engineering, University of Stuttgart, Stuttgart, Germany
Carbon dioxide formation mirrors the final carbon oxidation steps of aerobic metabolism in microbial and mammalian cells. As a consequence, dissociation equilibria arise in fermenters by the growing culture. Anaplerotic reactions make use of the abundant levels for refueling citric acid cycle demands and for enabling oxaloacetate-derived products. At the same time, CO2 is released manifold in metabolic reactions via decarboxylation activity. The levels of extracellular depend on cellular activities and physical constraints such as hydrostatic pressures, aeration, and the efficiency of mixing in large-scale bioreactors. Besides, local levels might also act as metabolic inhibitors or transcriptional effectors triggering regulatory events inside the cells. This review gives an overview about fundamental physicochemical properties of in microbial and mammalian cultures effecting cellular physiology, production processes, metabolic activity, and transcriptional regulation.
Introduction
One of the most decisive decisions which needs to be made when developing novel bioprocesses is whether the final process will run under anaerobic or aerobic conditions. While severely reduced investment costs speak in favor of anaerobic production, expected productivities and intracellular energy availabilities are drivers for aerobic approaches. Anaerobic metabolism yields at two net ATP produced in glycolysis per glucose while aerobic counterparts may achieve >12 ATPs. This net ATP yield even represents a conservative estimation considering true ATP per oxygen (P/O) ratios of 1:1.3 which are lower than theoretical maxima of 2–3. Consequently, aerobic processes are often the first choice if ATP-challenging product formation with maximum cell-specific formation rates is targeted.
Carbon dioxide (CO2) is the inevitable product of respiration processes and as such always present in aerobic bioprocesses. This holds also true for the production of commodities, fine chemicals, or therapeutic proteins using microbes or mammalian cells. While therapeutic proteins and fine chemicals are typically produced in bioreactor of 5–20 m3 scale, the production of commodities is usually performed in 50–500 m3 size – or even larger. As an intrinsic property, partial CO2 pressures of these scales differ significantly from those found in lab-scale. This phenomenon is the inherent consequence of high absolute pressures and poor mixing conditions in large-scale bioreactors (Takors, 2012).
CO2 and its hydrated counterpart may not only serve as substrate or product for carboxylating and decarboxylating reactions, the species may also alter physicochemical properties of proteins, acidify the internal pH, and regulate virulence and toxin production in pathogens (Follonier et al., 2013). Therefore, can interact with cellular metabolism and can even create complex transcriptional responses. CO2 not only freely diffuses through the cellular membrane (Gutknecht et al., 1977), it may also accumulate in the same (Jones and Greenfield, 1982; Kuriyama et al., 1993; Bothun et al., 2004), thus increasing its permeability and fluidity which finally leads in the potentially lethal “anesthesia effect” (Isenschmid et al., 1995).
The fact that high pCO2 levels are used to sterilize food (Ballestra et al., 1996; Spilimbergo and Bertucco, 2003; Garcia-Gonzalez et al., 2007) anticipates that elevated pCO2 are not likely to improve the performance of microbial or mammalian production processes. Instead, as it will be shown, high pCO2 levels often coincide with the deterioration of the bioprocess performance. Consequently, thorough scale-up studies should preferably consider the analysis of pCO2 impacts to ensure an equally good performance in large-scale compared to lab-scale expectations. This is especially true for the establishment of novel bioprocesses which are the result of systems metabolic engineering studies performed in lab-scale.
This contribution aims at reviewing fundamental properties, sources, and impacts of CO2 for microbial and mammalian production processes. It yields at bringing together the major puzzle pieces of how interacts with producer cells. It will show that a lot has already been done – but still not everything is fully understood. This holds especially true for regulation of cellular metabolism where apparently serves as an underestimated trigger so far.
Fundamentals – Physicochemical Properties and Mass Transfer
Carbon dioxide (CO2, molar weight: 44.01 g/mol) is a colorless, odorless gas of linear molecular shape with a melting point at −56.6°C. It is present in the Earth atmosphere as a trace compound currently showing levels of about 400 ppm with the tendency of steady increase (http://co2now.org/).
The water solubility can be described applying Henry’s law.
with cCO2,L and pCO2 coding for the equilibrium values of the molar concentration of dissolved CO2 in the liquid L and the related partial CO2 pressure, respectively. For pure water at 25°C the Henry coefficient HCO2 = 34.5 mmol/barL is given (Stumm and Morgan, 1995). Using the Van’t Hoff correlation
the temperature dependency of the equilibrium constant (here: Henry-coefficient HCO2) can be estimated with the standard enthalpy change of the reaction ΔH0, the universal gas constant R, and the absolute temperature T as shown. Noteworthy, K codes for an integration constant that can be derived from reference data e.g., at 25°C. Using equation (2), HCO2(T = 20°C) = 40 mmol/barL and HCO2(T = 37°C) = 25 mmol/barL can be calculated. Decreasing Henry coefficients [as defined by (1)] mirror reducing gas solubility with rising temperature – a typical phenomenon for dissolved gasses at the given temperature range.
Besides temperature, CO2 solubility is also affected by electrolyte concentrations. Following the empirical Sechenov (1889) approach individual contributions of ion strength can be considered to estimate the resulting solubility of a gas in the salt-containing liquid (Noorman et al., 1992). However, the composition of fermentation media is often complex and changes steadily during the course of cultivation. Product and by-product formation, substrate consumption, and the addition of titrating agents are the reasons. Therefore, the most pragmatic approach is to measure CO2 solubility in real cultivation media. Own experimental observations show that real HCO2 values [according to (1)] are often increased, may be even doubled, compared to values for pure water (unpublished data).
Applying typical operating conditions, microbial or mammalian cultivations release exhaust gas with volumetric CO2 fractions of 5–25%. For a conservative estimation, one can assume equilibrium conditions between gas and liquid with HCO2 values for pure water at 37°C. Then dissolved CO2 levels cCO2,L are likely to range between 75 and 375 mg/L. For instance, Blombach et al. (2013) measured pCO2 levels of about 160 mbar (about 360 mg/L) at the end of an aerated (0.1 vvm) 1.5 bar pressured, stirred batch cultivation with 5 gCDW Corynebacterium glutamicum per L. Increasing the aeration to 3 vvm reduced the pCO2 to 40 mbar (about 90 mg/L). Similar values were observed by Buchholz et al. (2014b). By contrast, maximum dissolved oxygen concentrations under atmospheric conditions will typically result at 7.5–8 mg/L (again depending on medium composition). Consequently, dissolved CO2 levels outcompete dissolved O2 levels by far. This finding may be even more pronounced if mass transport characteristics are considered (Figure 1).
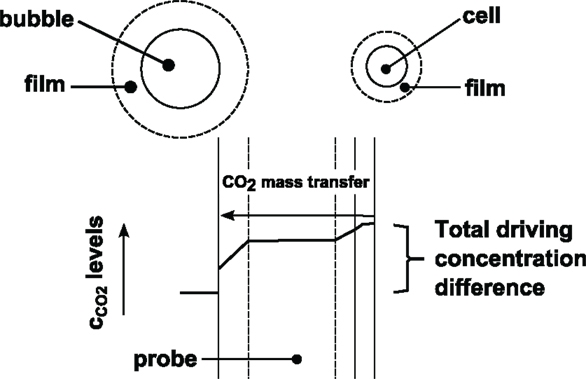
Figure 1. Principles of CO2 mass transfer. CO2 is released by the cells and transported through the surrounding liquid film via diffusion. After leaving this few μm-thick layer dissolved CO2 enters the well-mixed zone and is transported to the bubbles via convection. To reach the bubbles dissolved CO2 must pass the surrounding film by diffusion again. Probes for dissolved CO2 or O2 measurements are installed in the convective zone thus giving the related signal.
Figure 1 shows that maximum cCO2,L levels are found in the proximate microenvironment of the cells. By trend, probes for dissolved gas measurement observe lower levels. This is different compared to dissolved oxygen where cells face the lowest levels along the mass transfer path.
While dissolved carbon dioxide levels may achieve high inhibiting values during the fermentation course, starting conditions might be limiting instead. Assuming equilibrium between inlet aeration and the liquid 0.5 mgCO2/L is present. Noteworthy this low value is likely to persist if too high aeration (with low concentrated CO2) strips out new metabolically produced CO2. Consequently anaplerotic reactions may be limited by substrate supply (see Section “Metabolic Release and Incorporation”) finally resulting at reduced cell growth.
By analogy to oxygen transfer, the CO2 transfer rate CTR (mmol/Lh) can be described according to the following:
with kLaCO2 coding for the CO2 mass transfer coefficient (1/h), for the dissolved CO2 concentration at equilibrium following Henry’s law (mmol/L) and cCO2,L representing the measured concentration (mmol/L).
Measuring true kLaCO2 values in praxis is somewhat challenging. One approach is to assume CTR = CER, i.e., carbon dioxide emission rate CER equals the CO2 stripping rate CTR. By balancing flows of aeration and exhaust gas, related values should be accessible and kLaCO2 can be derived accordingly. Nevertheless, this approach reveals its drawback when mammalian cell cultures are balanced. Here, the exhaust gas signal is a superposition of biological activity and CO2 addition for titration. Alternatively, kLaCO2 could be estimated from kLaO2 according to the following:
Equation (4) results from Higbie’s penetration theory Higbie (1935) and Danckwerts surface renewable model Danckwerts (1951). Apparently, the mass transfer coefficient for CO2 is proportionally linked to the ratio of the diffusion coefficients D for CO2 and O2 in water. As kLaO2 values are relatively easy to measure, the approach offers a straightforward access to kLaCO2. However, CO2 transfer differs fundamentally from O2 transport because dissociation characteristics have been taken into account (see Figure 2).
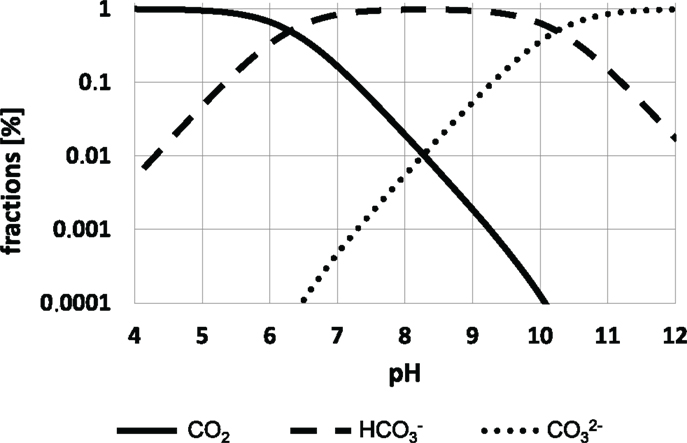
Figure 2. Dissociation equilibria of CO2 species depending on the pH. The equilibrium constants K1 = 10−6.3M and K2 = 10−10.25M were chosen. For details see text.
CO2 dissociates in water depending on pH as follows:
Because the equilibrium of CO2 dissociation is far on the side of the anhydride (99.8%), concentrations of the carbonic acid H2CO3 are low not exceeding one digit micromolar ranges at typical cultivation conditions. Consequently, the apparent equilibrium constant K1 (Bailey and Ollis, 1986):
is formulated and completed by Bailey and Ollis (1986) as follows:
One may safely assume that (de-) protonating reactions of formula (5) are very fast. However, formation and dissociation of carbonic acid from CO2 are suspected to limit the total equilibration process. k1 and k−1 were estimated as 0.03 1/s and 20 1/s, respectively (Bailey and Ollis, 1986).
At typical cultivation conditions (pH 7), 83.3% of the CO2 species are present as only 16.7% as CO2. Hence is about five-fold higher concentrated than CO2. This statement not only holds for the cultivation medium, but it should also be valid for intracellular conditions because cells aim at maintaining their intracellular pH at about this level.
Figure 3 underpins that the full consideration of the individual species CO2, , and is crucial to get accurate values for total CO2 cT dissolved in the fermentation suspension. Recently, Buchholz et al. (2014a) outlined that ignoring the anions leads to a carbon gap of about 20% during the first hours of fermentation. Noteworthy, the dissolved CO2 level is not dependent on pH (see Figure 3). According to Henry’s law only partial pressure (and salt conditions) may effect cCO2. Hence, large-scale bioreactors which have high hydrostatic pressures of 1–1.5 bar possess higher dissolved CO2 levels than comparable laboratory systems. This not only induces regulatory responses in the cells but also affects the buffering capacity of the large-scale suspension. Due to increased levels, pH buffering is severely increased in large scale compared to lab fermentations.
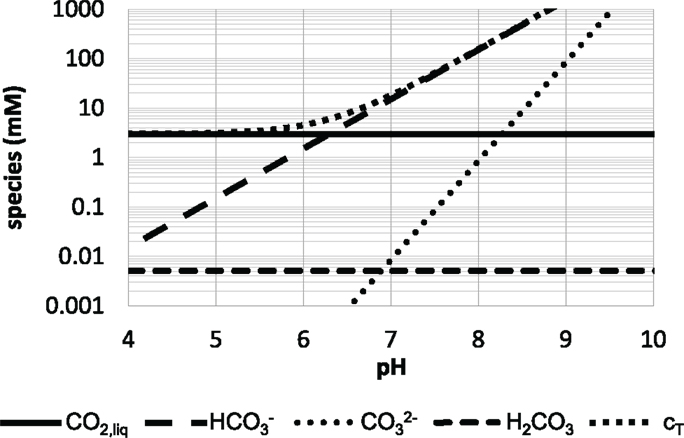
Figure 3. Distribution of CO2 species in water at atmospheric conditions (25°C). The species according to formula (5) are given as well as their sum cT [figure modified after Bailey and Ollis (1986)].
Metabolic Release and Incorporation
Metabolism of all living organisms is equipped with a set of carboxylases incorporating CO2 or bicarbonate into organic molecules and decarboxylases releasing CO2 in the environment. Consequently, these fundamental reactions are directly involved in and/or interconnect anabolism, catabolism, and energy metabolism of the cell. Especially, the phosphoenolpyruvate–pyruvate–oxaloacetate node comprises an organism-specific configuration of carboxylating (e.g., pyruvate carboxylase (PCx), PEP carboxylase, acetyl-CoA carboxylase) and decarboxylating (e.g., PEP carboxykinase; malic enzyme, oxaloacetate decarboxylase; pyruvate dehydrogenase complex, pyruvate:quinone oxidoreductase) reactions (Figure 4) which are of major importance for the carbon flux distribution in the central metabolism. For instance during sugar catabolism anaplerotic C3 (phosphoenolpyruvate (PEP)/pyruvate), carboxylation and decarboxylation of pyruvate to acetyl-CoA are essentially required to maintain TCA flux whereas gluconeogenesis relies on C4 (oxaloacetate/malate) decarboxylation (Sauer and Eikmanns, 2005). Another example is pyruvate decarboxylase of yeast which is the key enzyme in ethanol fermentation and is essentially required to maintain a balanced metabolism in mineral media containing glucose as sole carbon source (Pronk et al., 1996).
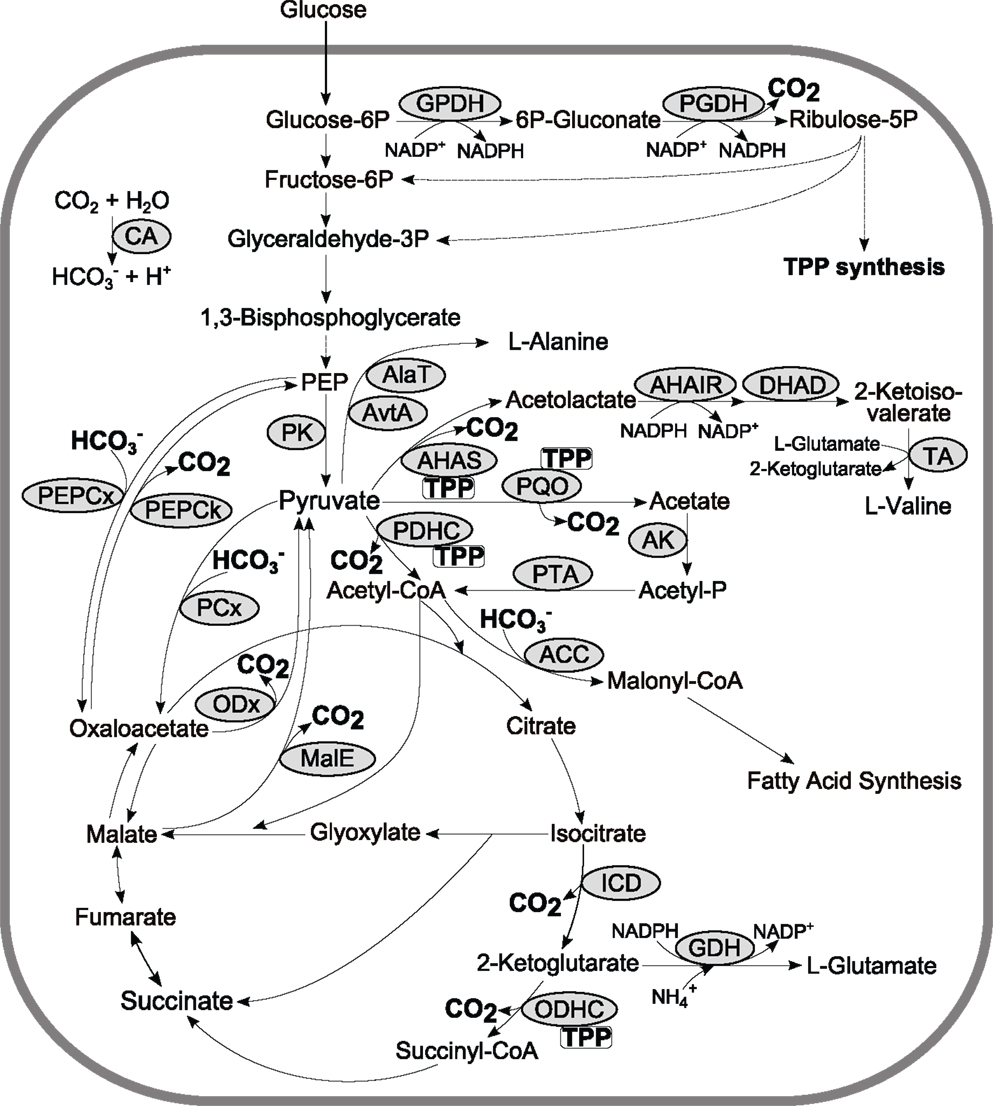
Figure 4. Exemplary, a schematic overview of the central metabolism of C. glutamicum including carboxylases and TPP-dependant decarboxylases. Abbreviations: ACC, acetyl-CoA carboxylase; AHAIR, acetohydroxyacid isomeroreductase; AHAS, acetohydroxyacid synthase; AK, acetate kinase; AlaT, alanine aminotransferase; AvtA, valine-pyruvate aminotransferase; CA, carbonic anhydrase; DHAD, dihydroxyacid dehydratase; GDH, glutamate dehydrogenase; GPDH, glucose-6P dehydrogenase; ICD, isocitrate dehydrogenase; MalE, malic enzyme; ODHC, 2-ketoglutarate dehydrogenase complex; ODx, oxaloacetate decarboxylase; PCx, pyruvate carboxylase; PDHC, pyruvate dehydrogenase complex; PEP phosphoenolpyruvate; PEPCk, PEP carboxykinase; PEPCx, PEP carboxylase; PGDH, 6P-gluconate dehydrogenase; PK, pyruvate kinase; PQO, pyruvate:quinone oxidoreductase; PTA, phosphotransacetylase; TA, transaminase B; TPP, thiamine pyrophosphate.
Carboxylases catalyzing the thermodynamically expensive assimilation of have been classified regarding their physiological function into autotrophic, assimilatory, biosynthetic, anaplerotic, and redox balancing enzymes (Erb, 2011). Currently, six pathways for CO2 fixation have been identified: the reductive pentose phosphate (Calvin–Benson) cycle, the reductive acetyl-CoA (Wood–Ljungdahl) pathway, the reductive citric acid cycle, the 3-hydroxypropionate bicycle, the dicarboxylate/4-hydroxybutyrate cycle, and the 3-hydroxypropionate/4-hydroxybutyrate cycle (Erb, 2011; Fuchs, 2011). Additionally, to increase the carbon fixation rate, novel synthetic pathways have been proposed (Bar-Even et al., 2010). Plants, algae and phototrophic prokaryotes possess ribulose-1,5-bisphosphate carboxylase/oxygenase (RubisCO) quantitatively the most abundant enzyme in the biosphere and the key enzyme in autotrophic CO2 fixation by the Calvin–Benson cycle (Miziorko and Lorimer, 1983; Hartman and Harpel, 1994; Erb, 2011). Notably, the Wood–Ljungdahl pathway is the only one which fixes CO2 and simultaneously generates ATP by conversion of acetyl-CoA to acetate (Fuchs, 2011) rendering this route attractive and promising for CO2-based microbial production purposes (Dürre and Eikmanns, 2015).
Owing to the metabolic key functions carboxylases and decarboxylases fulfill, relevant microbial, mammalian, and plant enzymes have been biochemically characterized and their regulation analyzed [e.g., Miziorko and Lorimer (1983), Chollet et al. (1996), Hanson and Reshef (1997), Nikolau et al. (2003), Sauer and Eikmanns (2005), and Jitrapakdee et al. (2006)]. -related allosteric regulation and biochemical properties of relevant enzymes have been analyzed to some extent (Jones and Greenfield, 1982), however, our understanding is limited by far, yet. The industrially important Gram-positive C. glutamicum possesses the biotin-dependent PCx and the PEP carboxylase, both with Michaelis–Menten constants (KM) for of about 3 mM (Hanke et al., 2005; Chen et al., 2013) which is about 30-fold higher compared to the KM (0.1 mM i.e., 4.4 mg/L) of PEP carboxylase from Escherichia coli as single anaplerotic enzyme (Kai et al., 1999). These differences already point to organism-specific aeration needs to install proper metabolic activity by maintaining sufficient availability at fermentation start when biomass concentrations are still low (Repaske et al., 1974; Talley and Baugh, 1975) or for products requiring a high anaplerotic flux (e.g., succinate, l-lysine, and derived products).
Due to the diffusive potential of CO2 and the rather slow chemical conversion of CO2 to (Kern, 1960), nature has independently evolved three classes (designated as α, β, and γ) of zinc-dependant carbonic anhydrases (CAs) which catalyze with very high turnover numbers (up to 106 s−1) the reversible hydration of CO2 (Figure 4; Tashian, 1989; Tripp et al., 2001). CAs are widespread over all kingdoms of life and play a vital role in various cellular functions such as photosynthesis, ion transport, and pH homeostasis (Smith and Ferry, 2000; Merlin et al., 2003; Mitsuhashi et al., 2004). Essentially, CAs maintain adequate levels for aerobic growth under ordinary atmospheric conditions, since inactivation of CAs in several organisms such as C. glutamicum, E. coli, Ralstonia eutropha, Candida albicans, Saccharomyces cerevisiae, and Aspergillus nidulans is lethal unless the CO2 content in the atmosphere is significantly increased (about 5–10%; Mitsuhashi et al., 2004; Merlin et al., 2003; Kusian et al., 2002; Götz et al., 1999; Cottier et al., 2012).
In mammals, mitochondrial respiration generates CO2 as waste product which has to be actively transported from tissue to the depolluting lungs by the blood. Since is in contrast to CO2 not permeable, mammalian cells are equipped with about 13 genes encoding different types of bicarbonate transporters allowing the intercellular exchange of the former species (Casey, 2006). By contrast, transport in prokaryotes has been rarely observed so far with the well-studied exception of the bicarbonate transport system of the cyanobacteria Synechococcus sp. strain PCC7942. Two different transport mechanisms for maintain with the combined action of CA elevated levels of CO2 in the carboxysomes required for efficient carbon fixation by RubisCO (Ritchie et al., 1996; Badger and Price, 2003).
CO2 Induced Growth Phenotypes
CO2 is the final respiratory product and consequently inevitable in aerobic microbial and mammalian bioprocesses. In exhaust gas flows the CO2 fraction may rise to 15–20% depending on aeration and cellular activity. Considering that head overpressures of microbial fermentations are commonly 0.5–2 bar and 10–15 m bioreactor filling heights create hydrostatic pressures of 1–1.5 bar, pCO2 could achieve maximum values of 0.1–0.6 bar at the bottom of the bioreactor. Notably, these maximum values may be reduced if aeration with fresh air is properly installed there. In principle, the scenario is similar for mammalian cultures although lowered due to reduced cell activities and smaller bioreactor sizes compared to microbial applications (pCO2 at mammalian production: about 0.180 barCO2; Zhu et al., 2005). Noteworthy, cells circulating in large-scale bioreactors experience frequently changing pCO2 levels, a fact that is usually not simulated by pseudo-stationary scale-down tests.
Multiple studies have been performed for elucidating the impact of pCO2 levels on microbial (Dixon and Kell, 1989) and mammalian performance [e.g., Gray et al. (1996)]. Effects on growth, biomass per substrate yields, product formation, cell division, and morphology were analyzed. These were either attributed to elevated CO2 partial pressures alone or in conjunction with co-effects such as changing osmolality in the media. Observed phenotypes are individual. Nevertheless, some characteristic examples are given in the following highlighting basic kinetics of industrially interesting strains:
Bacteria
First indications that bacteria do react on elevated dissolved CO2 levels were published by Jones and Greenfield (1982). Among others, they observed that growth of Bacillus subtilis was inhibited by 40% under pCO2 = 0.17 atm (0.172 bar). Batch studies with E. coli using CO2-enriched aeration revealed that the maximum growth rate was severely reduced and biomass per glucose yields increased for aeration fractions >20% of carbon dioxide (Castan et al., 2002). Baez et al. (2009) studied GFP producing E. coli at constant pCO2 in the range of 20–300 mbar. Their results supported previous findings by measuring more than 30% reduction of the maximal growth rate μmax and doubled acetate formation under pCO2 = 300 mbar compared to the reference. For C. glutamicum, Knoll et al. (2005) investigated the growth rates μ in overpressurized bioreactors (10 bar head pressure) during growth on glucose. They observed μ >0.3 1/h under pCO2 = 0.43 bar. This finding was supported by subsequent studies with an l-lysine producing C. glutamicum strain (Knoll et al., 2007). Additionally, turbidostatic continuous cultivations were performed installing different pCO2 levels. The growth rate of 0.58 1/h turned out to be almost constant until 0.18 bar pCO2 and steadily decreased to 0.36 1/h under 0.8 barCO2 (Bäumchen et al., 2007). In 2013, Blombach et al. studied the growth performance of C. glutamicum in batch cultures. While no significant growth phenotype was found installing pCO2 of about 0.3 bar, low levels smaller than 50 mbarCO2 revealed 3-phase, bi-level growth kinetics of C. glutamicum (Blombach et al., 2013). Recently, Lopes et al. (2014) reviewed some microbial phenotypes as a result of elevated carbon dioxide levels in over-pressurized bioreactors.
Yeast
Chen and Gutmains (1976) reported about growth inhibition of yeast at high CO2 partial pressures. They found “slight” growth inhibition using CO2 aeration fractions of 40% and a severe growth decrease using 50% CO2 enriched air. Later, Kuriyama et al. (1993) underlined these early findings by arguing that cell division of S. cerevisiae may be hampered under pCO2 = 0.5 atm (0.51 bar). Kuriyama et al. (1993) used chemostat approaches for studying the pCO2 impact. They found that an elevated pCO2 coincided with increased ethanol formation which itself may hamper process performance. S. cerevisiae is able to adapt to hyperbaric conditions (10 bar) provided that sufficient time for adaptations is given (Belo et al., 2003). CO2 partial pressures of 0.48 bar had negligible effects on cell viability. This was also observed by Knoll et al. (2007). However, if partial pressures are increased further (0.6 bar) cell budding is hampered (Coelho et al., 2004). Indeed, a growth reduction of 25% was reported by Aguilera et al. (2005) when the CO2 fraction of aeration was increased to 79% in aerobic cultivations. However, growth under anaerobic conditions was much less affected indicating that the respiratory metabolism is likely to be more influenced under high pCO2 levels. This phenomenon was in the focus of recent studies. Richard et al. (2014) outlined that transient metabolic responses are triggered by CO2 shifts e.g., characterized by intermediary increase of respiration rates and the excretion of ethanol and acetate.
Fungi
Similar to bacteria and yeast, inhibition of growth (and product formation) was also observed for fungi such as Penicillium chrysogenum already under pCO2 = 0.08 atm (Jones and Greenfield, 1982). Ho and Smith (1986) specified this early observation by identifying reduced growth and penicillin formation rates using 12.6% CO2 enriched air for cultivation. However, causes and consequences of high pCO2 levels on growth and product formation may not be clearly identifiable. They may rather be a matter of indirect effects finally resulting in morphology changes (McIntyre and McNeil, 1998). Also Gibbs et al. (2000) pinpointed to the chemical interaction of high pCO2 with precursors of penicillin biosynthesis finally deteriorating performance of P. chrysogenum. Nevertheless, under high levels of pCO2 (installed after using 10–15% enriched influent gas) increased climbing and severely reduced penicillin production were observed (El-Sabbagh et al., 2006), not only for P. chrysogenum but also for cephalosporin C producing Acremonium chrysogenum (El-Sabbagh et al., 2008).
Mammalian Cells (e.g., CHO)
Today, mammalian producers are typically derived from tissue cells giving Chinese hamster ovary (CHO) cells an outstanding importance for the production of therapeutic proteins (Pfizenmaier and Takors, 2015). It has been estimated that these cells experience pCO2 levels of 41–72 mbar under physiological conditions (Altman and Dittmer, 1971). However, industrial production environments are likely to impose much higher pCO2, especially when processes are in the focus of ongoing intensification (Ozturk, 1996). pCO2-induced stress usually coincides with the increase of osmolality due to titration for pH control. Hence, the interaction of both effects is often in the foreground of related studies. Kimura and Miller (1996) analyzed recombinant tissue-type plasminogen activator (tPA) production with CHO cells. Under maximum pCO2 of 333 mbar they observed 30% reduction of the growth rate which increased to 45% reduction in combination with high osmolality. Results of Gray et al. (1996) anticipated that an optimum for recombinant protein production exists at 40–100 mbar pCO2. Zhu et al. (2005) showed that industrial osmolality conditions (400–450 mOsm) together with typically high pCO2 (180–213 mar) levels caused a 20% drop of CHO cell viability. Besides, Takuma et al. (2007) outlined that industrial pCO2 values of 293 mbar reduced growth by 60% while cell-specific productivity of antibody IgG1 was almost unchanged. Additionally, there were indications that appropriate glucose limitation could compensate pCO2 triggered growth reduction at “moderate” 190 mbarCO2.
Among others, one reason for the deteriorating performance may be that protein glycosylation patterns reduce in the presence of elevated levels (Zanghi et al., 1999). Besides, DeZengotita et al. (2002) argued that glycolysis was inhibited in a dose-dependent manner when pCO2 levels were studied between 66 and 333 mbar in hybridoma cells. Therefore, pCO2 inhibition is not only a matter of CHO cells alone, but is observed for hybridoma and HEK293S cultures as well (Jardon and Garnier, 2003).
-Induced Regulation
not only serves as substrate or product for enzymes, but also impacts the internal pH, the fluidity and permeability of membranes, and physicochemical properties of proteins, and is regarded as signal for virulence and toxin production in pathogens (Isenschmid et al., 1995; Stretton and Goodman, 1998; Follonier et al., 2013). Due to the multiple involvement of in cellular metabolism, it seems evident that these species are directly or indirectly part of the regulatory machinery.
The human body underlies a complex homeostasis with bicarbonate concentrations up to 140 mM in certain tissues (Arthurs and Sudhakar, 2005; Abuaita and Withey, 2009; Orlowski et al., 2013) representing a striking signal for pathogens invading the host. Although a direct association between CO2 and virulence is missing, Park et al. (2011) found that 10% CO2 stimulated aerobic growth of the human gastric pathogen Helicobacter pylori. CO2 deprivation led to increased intracellular ppGpp levels which might indicate an involvement of the stringent response in CO2-dependent regulation of H. pylori’s metabolism (Park et al., 2011). In Vibrio cholerae bicarbonate activates the regulatory protein ToxT which in turn induces virulence gene expression (Abuaita and Withey, 2009). Another, bicarbonate sensing transcriptional regulator is the AraC-like protein RegA from the mouse enteric pathogen Citrobacter rodentium which in the presence of bicarbonate activates transcription of a number of virulence genes and inhibits expression of several housekeeping genes (Yang et al., 2009). C. albicans a fungal pathogen causing life-threatening infections in immunocompromised patients senses increased levels by the soluble adenylyl cyclase (sAC) Cyr1p which produces cAMP. Then, cAMP activates protein kinase A to trigger filamentous growth which is an important feature for adhesion and invasion of the pathogen (Klengel et al., 2005; Hall et al., 2010). Furthermore, the transcription factor Rca1p of C. albicans was shown to control expression of CA in response to the availability of CO2 (Cottier et al., 2012). Both examples demonstrate the relevancy of a signaling system for global regulation of C. albicans’ metabolism. Regulation by bicarbonate-responsive soluble ACs seems be more widespread across multiple kingdoms since -dependent adjustment of the intracellular cAMP level, initially found in male germ cells, was also identified in mycobacteria, eubacteria, fungi, and cyanobacteria (Chen et al., 2000; Zippin et al., 2001; Bahn and Mühlschlegel, 2006).
Although in large-scale fermentations gradients of dissolved gases occur and high concentrations depending on the process and the production host arise (Hermann, 2003; Takors, 2012), only few studies investigated the effects of altered levels of these species on metabolism and regulation of industrial relevant microbial cells systematically. The already mentioned analysis of Baez et al. (2009) studied the effect of 300 mbar partial pressure on recombinant GFP producing E. coli not only metabolically but also on the transcriptional level. Expression analysis of 16 selected genes revealed only slight changes in transcription. Noteworthy, as response to elevated dissolved CO2 the transcription of acid stress genes (gadA, gadC, and adiA) increased, indicating acidification of the internal pH by CO2 (Baez et al., 2009).
Recently, Follonier et al. (2013) exposed Pseudomonas putida KT2440 to elevated pressure (up to 7 bar) associated with increased concentrations in the bioreactor. They investigated the global transcriptional response by DNA microarrays. Physiology of P. putida KT2440 was hardly affected at increased pressure, however, significant changes in gene transcription were observed: elevated levels activated the heat-shock response and strongly affected expression of cell envelope genes pointing to an altered permeability/fluidity of the membrane (Follonier et al., 2013).
The genome-wide transcriptional response of S. cerevisiae to high CO2 concentrations was analyzed in chemostat cultures under aerobic and anaerobic conditions. Accompanied with a more pronounced sensitivity of respiratory metabolism, high CO2 levels in glucose-limited cultures led to 104 at least two-fold altered transcripts compared to 33 under anaerobic conditions. Interestingly, 50% of the affected transcripts under aerobic conditions encoded mitochondrial proteins such as PEP carboxykinase, PCx, and proteins involved in oxidative phosphorylation (Aguilera et al., 2005).
Recently, we investigated the effects of low (pCO2 < 40 mbar) and high (pCO2 ≥ 300 mbar) levels on growth kinetics and the transcriptional response of C. glutamicum compared to standard conditions. Under high levels growth kinetics were not affected albeit the biomass to substrate yield was increased. However, a complex transcriptional response involving 117 differentially expressed genes was observed. Among those, 60 genes were assigned to the complete DtxR/RipA regulon controlling iron homeostasis in C. glutamicum. The mutant C. glutamicum ΔdtxR showed significantly impaired growth under high conditions (compared to the wildtype) but not under standard conditions. This finding underlines the relevancy of the master regulator for cell fitness under high levels (Blombach et al., 2013). At low levels C. glutamicum showed three distinct growth phases. In the mid-phase with slowest growth, C. glutamicum secreted l-alanine and l-valine into the medium and showed about two times higher activities of glucose-6-P dehydrogenase and 6-phosphoglconate dehydrogenase and a strong transcriptional response (>100 genes with altered expression) including increased transcription of almost all thiamine pyrophosphate (TPP) genes compared to standard conditions. We hypothesized that C. glutamicum counteracts the lack of by triggering TPP biosynthesis for increasing the activities of TPP-dependent enzymes involved in CO2 formation (Figure 4; Blombach et al., 2013).
Industrial scale cells are exposed to various gradients such as pH, substrates, and dissolved gases. To analyze the effects of oscillating levels on the metabolism and transcriptional response of C. glutamicum, a novel three-compartment cascade bioreactor system was developed. pCO2 gradients of 75–315 mbar at industry-relevant residence times of about 3.6 min did not significantly influence the growth kinetics but led to 66 differentially expressed genes compared to control conditions. Interestingly, the overall change in expression was directly linked to the pCO2 gradients and the residence time of the cells in the scale-down device (Buchholz et al., 2014b).
Impacts Production Processes
Production processes on glycolytic substrates rely on the anaplerotic function of PCx and/or PEP carboxylase to replenish citric acid cycle intermediates that are incorporated for anabolic demands and/or product formation. Especially, oxaloacetate-derived products such as l-lysine require a high anaplerotic flux. C. glutamicum is the workhorse in industrial l-lysine production and possesses PCx and PEP carboxylase. Several studies identified PCx and especially deregulated variants as most relevant to improve oxaloacetate supply since inactivation of PCx reduced and overexpression of the corresponding pyc gene significantly improved l-lysine formation in C. glutamicum (Peters-Wendisch et al., 2001; Ohnishi et al., 2002). Furthermore, inactivation of PEP carboxykinase led to an increase in l-lysine production with C. glutamicum (Riedel et al., 2001). Surprisingly, although great efforts have been made to tailor the biosynthetic pathway and to optimize precursor availability (Blombach and Seibold, 2010), the impact of altered levels for aerobic l-lysine production has not been systematically investigated so far.
Apparently too low levels may limit the in vivo activity of anaplerotic reactions. The combination of high aeration and low biomass concentration at the beginning of the fermentation is likely to cause retarded cell growth due to CO2 over-stripping. By analogy, installing non-limiting levels is especially important for zero-growth or resting cell bioprocesses. Examples are the synthesis of organic acids such as malate, fumarate and succinate which are formed anaerobically from oxaloacetate via the reductive arm of the citric acid cycle. Under such conditions only minor amounts of are provided by the metabolism of the cell. However, elevated productivities can be achieved by sparging with CO2 or adding carbonates to the medium to ensure sufficient for C3-carboxylation (Inui et al., 2004; Okino et al., 2005, 2008; Lu et al., 2009; Zelle et al., 2010; Zhang et al., 2010; Wieschalka et al., 2012). Inui et al. (2004) and Okino et al. (2005) showed that addition of NaHCO3 to the medium significantly improved the glucose consumption rate and the succinate production rate with resting cells of C. glutamicum R. Radoš et al. (2014) demonstrated that sparging an anaerobic culture of non-growing C. glutamicum with CO2 improved the succinate and acetate yield, respectively, both at the expense of lactate production. 13C nuclear magnetic resonance analysis of labeling patterns in the end products verified the incorporation of bicarbonate and the formation of succinate mainly via the reductive arm of the citric acid cycle (Radoš et al., 2014). For a dual-phase (aerobic growth, anaerobic production) succinate production process with a recombinant E. coli strain, it was also shown that increasing the CO2 content in the gas phase from 0 to 50% improved the biomass-specific production rate and the succinate yield significantly (Lu et al., 2009). In order to provide additional CO2 and reduction equivalents for anaerobic succinate production from glucose, Litsanov et al. (2012) integrated the fdh gene encoding a formate dehydrogenase from Mycobacterium vaccae into the chromosome of an engineered C. glutamicum strain. Supplementation of formate increased the succinate yield by 20% mainly due to increased NADH availability. However, part of the formed CO2 was incorporated into the product (Litsanov et al., 2012).
The shortage of oil resources and steadily rising oil prices has stimulated efforts to produce chemicals and fuels directly from CO2. Production of ethanol, isobutyraldehyde, and isobutanol from CO2 and light was achieved using engineered photosynthetic bacteria such as Rhodobacter capsulatus and Synechococcus elongates PCC7942 (Wahlund et al., 1996; Atsumi et al., 2009). Li et al. (2012) showed the feasibility of electrochemical supply of electrons to produce isobutanol and 3-methyl-1-butanol from CO2 with engineered R. eutropha H16. However, the low productivity and final titer of such approaches and the reactor design is still a challenge for future industrial application. Alternatively, RubisCO was functionally expressed in heterotrophic S. cerevisiae to incorporate CO2 as co-substrate improving ethanol production and reducing the formation of the by-product glycerol in chemostat cultures (Guadalupe-Medina et al., 2013). An innovative approach is the use of CO2 and hydrogen-containing waste gases or synthesis gas as feedstock for the production of chemicals and fuels with acetogenic and carboxydotrophic bacteria. Aerobic and anaerobic gas fermentation processes have been exploited for their biotechnological potential and commercial plants for ethanol production are already under construction (Dürre and Eikmanns, 2015).
Mammalian producer cells descent from rodents (like mouse or hamster) or human tissues. In case they are used in submerse culture they have undergone a (sometimes) tedious transition to yield at suspended producer cell lines. With this history in mind one may understand why product formation in producer cells such as CHO is often found to be strongly growth de-coupled (Altamirano et al., 2001). This fact is even exploited by temperature shift-down approaches (37 to ~30°C) to arrest cells in G1 phase finally increasing cell-specific protein production. By analogy, osmolality increase results at similar growth and product formation phenotypes (Ozturk and Palsson, 1991; Kumar et al., 2007). As outlined in the foregoing sections, elevated pCO2 environments >100 mbar are likely to inhibit cell growth for CHO cultures. Consequently, therapeutic protein formation kinetics of the (typical) growth de-coupled type are not likely to be affected by high pCO2 environments. Indeed, findings of Takuma et al. (2007) support this conclusion. In case growth-coupled product formation is observed, the impact of increased carbon dioxide partial pressures may be more pronounced. This holds also true for putative interactions of high levels with the cellular membrane or the product proteins. However, more studies are necessary to investigate these individual effects.
Conclusion
Summarizing the impacts of high levels, the reduction of cellular growth is a typical phenomenon. Although the effects are very individual, sensitivities on high CO2 partial pressures are less pronounced in bacteria than they are in fungi or mammalian producer cells. As a rule of thumb pCO2 > 100 mbar marks the beginning of growth inhibition for the later.
On the other hand, too low levels are likely to limit anaplerotic reactions inside the cells. Consequently, downstream precursors such as oxaloacetate could become limiting which affects not only cell growth but also biosynthesis of related metabolic products.
In general, transcriptional responses on high (or low) are by far less studied than metabolic phenotypes. However, (maybe) surprising regulatory mechanisms are waiting to be discovered. An illustrative example is the case of C. glutamicum that aims at counteracting limitation by amplifying TPP biosynthesis, known as an essential co-factor for decarboxylating enzymes. High levels apparently serve as an important stimulus for some pathogenic microbes to identify the host and to trigger related invasion programs. To what extent fragments or derivatives of such regulatory scenarios are also present in other cells also remains to be discovered.
Considering the application of microbes, yeasts, fungi, and mammalian cells in industrial bioreactors some particularities need to be taken into account. High levels do not effect cells as a singular, isolated event. They rather occur in conjunction with changes of osmolality and pH that stimulate the cells manifold. The unequivocal identification of causes and consequences may be hampered intrinsically. Complex networking analysis is necessary to decipher details of impacts. Examples are the link between and productivity with morphology changes in fungi or the osmolality in CHO. On the other side, equal pCO2 levels may serve as a valuable scale-up criterion because they mirror the complex interaction of cellular activities, mixing, and mass transfer (Klinger et al., 2015). Furthermore, one should consider that stimuli occur dynamically under industrial operation conditions. Cells are circulating in large-scale production reactors thus experiencing frequently changing dissolved CO2 levels. Consequently, comprehensive scale-up tests should mirror these conditions to ensure that promising novel producers will perform equally well in large scale – as they should.
Conflict of Interest Statement
The authors declare that the research was conducted in the absence of any commercial or financial relationships that could be construed as a potential conflict of interest.
Acknowledgments
The authors gratefully acknowledge the funding of this work by the Deutsche Forschungsgemeinschaft (DFG), grant TA 241/5-1 and TA 241/5-2. This work was also supported by DFG within the funding programme Open Access Publishing.
References
Abuaita, B. H., and Withey, J. H. (2009). Bicarbonate induces Vibrio cholerae virulence gene expression by enhancing ToxT activity. Infect. Immun. 77, 4111–4120. doi: 10.1128/IAI.00409-09
Aguilera, J., Petit, T., De Winde, J. H., and Pronk, J. T. (2005). Physiological and genome-wide transcriptional responses of Saccharomyces cerevisiae to high carbon dioxide concentrations. FEMS Yeast Res. 5, 579–593. doi:10.1016/j.femsyr.2004.09.009
Altamirano, C., Cairo, J. J., and Godia, F. (2001). Decoupling cell growth and product formation in Chinese hamster ovary cells through metabolic control. Biotechnol. Bioeng. 76, 351–360. doi:10.1002/bit.10096
Altman, P. L., and Dittmer, D. S. (eds) (1971). Biological Handbooks: Respiration and Circulation. Bethesda: Federation of American Societies for Experimental Biology.
Arthurs, G., and Sudhakar, M. (2005). Carbon dioxide transport. Contin. Educ. Anaesth. Crit. Care Pain 5, 207–210. doi:10.1093/bjaceaccp/mki050
Atsumi, S., Higashide, W., and Liao, J. C. (2009). Direct photosynthetic recycling of carbon dioxide to isobutyraldehyde. Nat. Biotechnol. 27, 1177–1180. doi:10.1038/nbt.1586
Badger, M. R., and Price, G. D. (2003). CO2 concentrating mechanisms in cyanobacteria: molecular components, their diversity and evolution. J. Exp. Bot. 54, 609–622. doi:10.1093/jxb/erg076
Baez, A., Flores, N., Bolívar, F., and Ramírez, O. T. (2009). Metabolic and transcriptional response of recombinant Escherichia coli to elevated dissolved carbon dioxide concentrations. Biotechnol. Bioeng. 104, 102–110. doi:10.1002/bit.22379
Bahn, Y. S., and Mühlschlegel, F. A. (2006). CO2 sensing in fungi and beyond. Curr. Opin. Microbiol. 9, 572–578. doi:10.1016/j.mib.2006.09.003
Bailey, J. E., and Ollis, D. F. (1986). Biochemical Engineering Fundamentals. 2nd Edition, McGraw-Hill Chemical Engineering Series. New York, NY: McGraw-Hill.
Ballestra, P., Da Silva, A. A., and Cuq, J. L. (1996). Inactivation of Escherichia coli by carbon dioxide under pressure. J. Food Sci. 61, 829–831. doi:10.1111/j.1365-2621.1996.tb12212.x
Bar-Even, A., Noor, E., Lewis, N. E., and Milo, R. (2010). Design and analysis of synthetic carbon fixation pathways. Proc. Natl. Acad. Sci. U.S.A. 107, 8889–8894. doi:10.1073/pnas.0907176107
Bäumchen, C., Knoll, A., Husemann, B., Seletzky, J., Maier, B., Dietrich, C., et al. (2007). Effect of elevated dissolved carbon dioxide concentrations on growth of Corynebacterium glutamicum on D-glucose and L-lactate. J. Biotechnol. 128, 868–874. doi:10.1016/j.jbiotec.2007.01.001
Belo, I., Pinheiro, R., and Mota, M. (2003). Fed-batch cultivation of Saccharomyces cerevisiae in a hyperbaric bioreactor. Biotechnol. Prog. 19, 665–671. doi:10.1021/bp0257067
Blombach, B., Buchholz, J., Busche, T., Kalinowski, J., and Takors, R. (2013). Impact of different CO2/HCO3- levels on metabolism and regulation in Corynebacterium glutamicum. J. Biotechnol. 168, 331–340. doi:10.1016/j.jbiotec.2013.10.005
Blombach, B., and Seibold, G. M. (2010). Carbohydrate metabolism in Corynebacterium glutamicum and applications for the metabolic engineering of L-lysine production strains. Appl. Microbiol. Biotechnol. 86, 1313–1322. doi:10.1007/s00253-010-2537-z
Bothun, G. D., Knutson, B. L., Berberich, J. A., Strobel, H. J., and Nokes, S. E. (2004). Metabolic selectivity and growth of Clostridium thermocellum in continuous culture under elevated hydrostatic pressure. Appl. Microbiol. Biotechnol. 65, 149–157. doi:10.1007/s00253-004-1554-1
Buchholz, J., Graf, M., Blombach, B., and Takors, R. (2014a). Improving the carbon balance of fermentations by total carbon analyses. Biochem. Eng. J. 90, 162–169. doi:10.1016/j.bej.2014.06.007
Buchholz, J., Graf, M., Freund, A., Busche, T., Kalinowski, J., Blombach, B., et al. (2014b). CO2/HCO3- perturbations of simulated large scale gradients in a scale-down device cause fast transcriptional responses in Corynebacterium glutamicum. Appl. Microbiol. Biotechnol. 98, 8563–8572. doi:10.1007/s00253-014-6014-y
Castan, A., Näsman, A., and Enfors, S.-O. (2002). Oxygen enriched air supply in Escherichia coli processes: production of biomass and recombinant human growth hormone. Enzyme Microb. Technol. 30, 847–854. doi:10.1016/S0141-0229(01)00490-2
Chen, S. L., and Gutmains, F. (1976). Carbon dioxide inhibition of yeast growth in biomass production. Biotechnol. Bioeng. 18, 1455–1462. doi:10.1002/bit.260181012
Chen, Y., Cann, M. J., Litvin, T. N., Iourgenko, V., Sinclair, M. L., Levin, L. R., et al. (2000). Soluble adenylyl cyclase as an evolutionarily conserved bicarbonate sensor. Science 289, 625–628. doi:10.1126/science.289.5479.625
Chen, Z., Bommareddy, R. R., Frank, D., Rappert, S., and Zeng, A.-P. (2013). Deregulation of feedback inhibition of phosphoenolpyruvate carboxylase for improved lysine production in Corynebacterium glutamicum. Appl. Environ. Microbiol. 80, 1388–1393. doi:10.1128/AEM.03535-13
Chollet, R., Vidal, J., and O’Leary, M. H. (1996). Pyruvate carboxylase: a ubiquitous, highly regulated enzyme in plants. Annu. Rev. Plant Physiol. Plant Mol. Biol. 47, 273–298. doi:10.1146/annurev.arplant.47.1.273
Coelho, M. A. Z., Belo, I., Pinheiro, R., Amaral, A. L., Mota, M., Coutinho, J. A. P., et al. (2004). Effect of hyperbaric stress on yeast morphology: study by automated image analysis. Appl. Microbiol. Biotechnol. 66, 318–324. doi:10.1007/s00253-004-1648-9
Cottier, F., Raymond, M., Kurzai, O., Bolstad, M., Leewattanapasuk, W., Jiménez-López, C., et al. (2012). The bZIP transcription factor Rca1p is a central regulator of a novel CO2 sensing pathway in yeast. PLoS Pathog. 8:e1002485. doi:10.1371/journal.ppat.1002485
Danckwerts, P. V. (1951). Significance of liquid-film coefficients in gas absorption. Ind. Eng. Chem. 43, 1460–1467. doi:10.1021/ie50498a055
DeZengotita, V. M., Schmelzer, A. E., and Miller, W. M. (2002). Characterization of hybridoma cell responses to elevated pCO2 and osmolality: intracellular pH, cell size, apoptosis, and metabolism. Biotechnol. Bioeng. 77, 369–380. doi:10.1002/bit.10176
Dixon, N. M., and Kell, D. B. (1989). The inhibition by CO2 of the growth and metabolism of micro-organisms. J. Appl. Bacteriol. 67, 109–136. doi:10.1111/j.1365-2672.1989.tb03387.x
Dürre, P., and Eikmanns, B. J. (2015). C1-carbon sources for chemical and fuel production by microbial gas fermentation. Curr. Opin. Biotechnol. 35, 63–72. doi:10.1016/j.copbio.2015.03.008
El-Sabbagh, N., Harvey, L. M., and McNeil, B. (2008). Effects of dissolved carbon dioxide on growth, nutrient consumption, cephalosporin C synthesis and morphology of Acremonium chrysogenum in batch cultures. Enzyme Microb. Technol. 42, 315–324. doi:10.1016/j.enzmictec.2007.10.012
El-Sabbagh, N., McNeil, B., and Harvey, L. M. (2006). Dissolved carbon dioxide effects on growth, nutrient consumption, penicillin synthesis and morphology in batch cultures of Penicillium chrysogenum. Enzyme Microb. Technol. 39, 185–190. doi:10.1016/j.enzmictec.2005.10.020
Erb, T. J. (2011). Carboxylases in natural and synthetic microbial pathways. Appl. Environ. Microbiol. 77, 8466–8477. doi:10.1128/AEM.05702-11
Follonier, S., Escapa, I. F., Fonseca, P. M., Henes, B., Panke, S., Zinn, M., et al. (2013). New insights on the reorganization of gene transcription in Pseudomonas putida KT2440 at elevated pressure. Microb. Cell Fact. 12, 30. doi:10.1186/1475-2859-12-30
Fuchs, G. (2011). Alternative pathways of carbon dioxide fixation: insights into the early evolution of life? Annu. Rev. Microbiol. 65, 631–658. doi:10.1146/annurev-micro-090110-102801
Garcia-Gonzalez, L., Geeraerd, A. H., Spilimbergo, S., Elst, K., Van Ginneken, L., Debevere, J., et al. (2007). High pressure carbon dioxide inactivation of microorganisms in foods: the past, the present and the future. Int. J. Food Microbiol. 117, 1–28. doi:10.1016/j.ijfoodmicro.2007.02.018
Gibbs, P. A., Seviour, R. J., and Schmid, F. (2000). Growth of filamentous fungi in submerged culture: problems and possible solutions. Crit. Rev. Biotechnol. 20, 17–48. doi:10.1080/07388550091144177
Götz, R., Gnann, A., and Zimmermann, F. K. (1999). Deletion of the carbonic anhydrase-like gene NCE103 of the yeast Saccharomyces cerevisiae causes an oxygen-sensitive growth defect. Yeast 15, 855–864. doi:10.1002/(SICI)1097-0061(199907)15:10A<855:AID-YEA425>3.0.CO;2-C
Gray, D. R., Chen, S., Howarth, W., Inlow, D., and Maiorella, B. L. (1996). CO2 in large-scale and high-density CHO cell perfusion culture. Cytotechnology 22, 65–78. doi:10.1007/BF00353925
Guadalupe-Medina, V., Wisselink, H. W., Luttik, M. A., de Hulster, E., Daran, J.-M., Pronk, J. T., et al. (2013). Carbon dioxide fixation by Calvin-Cycle enzymes improves ethanol yield in yeast. Biotechnol. Biofuels 6, 125. doi:10.1186/1754-6834-6-125
Gutknecht, J., Bisson, M. A., and Tosteson, F. C. (1977). Diffusion of carbon dioxide through lipid bilayer membranes: effects of carbonic anhydrase, bicarbonate, and unstirred layers. J. Gen. Physiol. 69, 779–794. doi:10.1085/jgp.69.6.779
Hall, R. A., de Sordi, L., MacCallum, D. M., Topal, H., Eaton, R., Bloor, J. W., et al. (2010). CO2 acts as a signalling molecule in populations of the fungal pathogen Candida albicans. PLoS Pathog. 6:e1001193. doi:10.1371/journal.ppat.1001193
Hanke, P. D., Sinskey, A. J., and Willis, L. B. (2005). Feedback-Resistant Pyruvate Carboxylase Gene from Corynebacterium. U.S. Patent No 6,965,021.
Hanson, R. W., and Reshef, L. (1997). Regulation of phosphoenolpyruvate carboxykinase (GTP) gene expression. Annu. Rev. Biochem. 66, 581–611. doi:10.1146/annurev.biochem.66.1.581
Hartman, F. C., and Harpel, M. R. (1994). Structure, function, regulation, and assembly of D-ribulose-1,5-bisphosphate carboxylase/oxygenase. Annu. Rev. Biochem. 63, 197–234. doi:10.1146/annurev.bi.63.070194.001213
Hermann, T. (2003). Industrial production of amino acids by coryneform bacteria. J. Biotechnol. 104, 155–172. doi:10.1016/S0168-1656(03)00149-4
Higbie, R. (1935). The rate of absorption of a pure gas into a still liquid during short periods of exposure. Trans. Am. Inst. Chem. Eng. 31, 364–389.
Ho, C. S., and Smith, M. D. (1986). Effect of dissolved carbon dioxide on penicillin fermentations: mycelial growth and penicillin production. Biotechnol. Bioeng. 28, 668–677. doi:10.1002/bit.260280506
Inui, M., Murakami, S., Okino, S., Kawaguchi, H., Vertès, A. A., and Yukawa, H. (2004). Metabolic analysis of Corynebacterium glutamicum during lactate and succinate productions under oxygen deprivation conditions. J. Mol. Microbiol. Biotechnol. 7, 182–196. doi:10.1159/000079827
Isenschmid, A., Marison, I. W., and von Stockar, U. (1995). The influence of pressure and temperature of compressed CO2 on the survival of yeast cells. J. Biotechnol. 39, 229–237. doi:10.1016/0168-1656(95)00018-L
Jardon, M., and Garnier, A. (2003). pH, pCO2, and temperature effect on R-adenovirus production. Biotechnol. Prog. 19, 202–208. doi:10.1021/bp025585a
Jitrapakdee, S., Vidal-Puig, A., and Wallace, J. C. (2006). Anaplerotic roles of pyruvate carboxylase in mammalian tissues. Cell. Mol. Life Sci. 63, 843–854. doi:10.1007/s00018-005-5410-y
Jones, R. P., and Greenfield, P. F. (1982). Effect of carbon dioxide on yeast growth and fermentation. Enzyme Microb. Technol. 4, 210–223. doi:10.1016/0141-0229(82)90034-5
Kai, Y., Matsumura, H., Inoue, T., Terada, K., Nagara, Y., Yoshinaga, T., et al. (1999). Three-dimensional structure of phosphoenolpyruvate carboxylase: a proposed mechanism for allosteric inhibition. Proc. Natl. Acad. Sci. U.S.A. 96, 823–828. doi:10.1073/pnas.96.3.823
Kimura, R., and Miller, W. M. (1996). Effects of elevated pCO2 and/or osmolality on the growth and recombinant tPA production of CHO cells. Biotechnol. Bioeng. 52, 152–160. doi:10.1002/(SICI)1097-0290(19961005)52:1<152:AID-BIT15>3.0.CO;2-Q
Klengel, T., Liang, W. J., Chaloupka, J., Ruoff, C., Schröppel, K., Naglik, J. R., et al. (2005). Fungal adenylyl cyclase integrates CO2 sensing with cAMP signaling and virulence. Curr. Biol. 15, 2021–2026. doi:10.1016/j.cub.2005.10.040
Klinger, C., Schmidberger, C., and Eisenkraetzer, D. (2015). Scale Down Strategies of Cell Culture Processes. Oral Presentation at DECHEMA Conference, Scale-Up and Scale-Down of Bioprocesses. Hamburg.
Knoll, A., Bartsch, S., Husemann, B., Engel, P., Schroer, K., Ribeiro, B., et al. (2007). High cell density cultivation of recombinant yeasts and bacteria under non-pressurized and pressurized conditions in stirred tank bioreactors. J. Biotechnol. 132, 167–179. doi:10.1016/j.jbiotec.2007.06.010
Knoll, A., Maier, B., Tscherrig, H., and Büchs, J. (2005). The oxygen mass transfer, carbon dioxide inhibition, heat removal, and the energy and cost efficiencies of high pressure fermentation. Adv. Biochem. Eng. Biotechnol. 92, 77–99. doi:10.1007/b98918
Kumar, N., Gammell, P., and Clynes, M. (2007). Proliferation control strategies to improve productivity and survival during CHO based production culture: a summary of recent methods employed and the effects of proliferation control in product secreting CHO cell lines. Cytotechnology 53, 33–46. doi:10.1007/s10616-007-9047-6
Kuriyama, H., Mahakarnchanakul, W., Matsui, S., and Kobayashi, H. (1993). The effects of pCO2 on yeast growth and metabolism under continuous fermentation. Biotechnol. Lett. 15, 189–194. doi:10.1007/BF00133022
Kusian, B., Sültemeyer, D., and Bowien, B. (2002). Carbonic anhydrase is essential for growth of Ralstonia eutropha at ambient CO2 concentrations. J. Bacteriol. 184, 5018–5026. doi:10.1128/JB.184.18.5018-5026.2002
Li, H., Opgenorth, P. H., Wernick, D. G., Rogers, S., Wu, T.-Y., Higashide, W., et al. (2012). Integrated electromicrobial conversion of CO2 to higher alcohols. Science 335, 1596. doi:10.1126/science.1217643
Litsanov, B., Brocker, M., and Bott, M. (2012). Towards homosuccinate fermentation: metabolic engineering of Corynebacterium glutamicum for anaerobic succinate production from glucose and formate. Appl. Environ. Microbiol. 78, 3325–3337. doi:10.1128/AEM.07790-11
Lopes, M., Belo, I., and Mota, M. (2014). Over-pressurized bioreactors: application to microbial cell cultures. Biotechnol. Prog. 30, 767–775. doi:10.1002/btpr.1917
Lu, S., Eiteman, M. A., and Altman, E. (2009). Effect of CO2 on succinate production in dual-phase Escherichia coli fermentations. J. Biotechnol. 143, 213–223. doi:10.1016/j.jbiotec.2009.07.012
McIntyre, M., and McNeil, B. (1998). Morphogenetic and biochemical effects of dissolved carbon dioxide on filamentous fungi in submerged cultivation. Appl. Microbiol. Biotechnol. 50, 291–298. doi:10.1007/s002530051293
Merlin, C., Masters, M., McAteer, S., and Coulson, A. (2003). Why is carbonic anhydrase essential to Escherichia coli? J. Bacteriol. 185, 6415–6424. doi:10.1128/JB.185.21.6415-6424.2003
Mitsuhashi, S., Ohnishi, J., Hayashi, M., and Ikeda, M. (2004). A gene homologous to beta-type carbonic anhydrase is essential for the growth of Corynebacterium glutamicum under atmospheric conditions. Appl. Microbiol. Biotechnol. 63, 592–601. doi:10.1007/s00253-003-1402-8
Miziorko, H. M., and Lorimer, G. H. (1983). Ribulose-1, 5-bisphosphate carboxylase-oxygenase. Annu. Rev. Biochem. 52, 507–532. doi:10.1146/annurev.bi.52.070183.002451
Nikolau, B. J., Ohlrogge, J. B., and Wurtele, E. S. (2003). Plant biotin-containing carboxylases. Arch. Biochem. Biophys. 414, 211–222. doi:10.1016/S0003-9861(03)00156-5
Noorman, H. J., Luijkx, G. C. A., Luyben, K. C., and Heijnen, J. J. (1992). Modeling and experimental validation of carbon dioxide evolution in alkalophilic cultures. Biotechnol. Bioeng. 39, 1069–1079. doi:10.1002/bit.260391102
Ohnishi, J., Mitsuhashi, S., Hayashi, M., Ando, S., Yokoi, H., Ochiai, K., et al. (2002). A novel methodology employing Corynebacterium glutamicum genome information to generate a new L-lysine-producing mutant. Appl. Microbiol. Biotechnol. 58, 217–223. doi:10.1007/s00253-001-0883-6
Okino, S., Inui, M., and Yukawa, H. (2005). Production of organic acids by Corynebacterium glutamicum under oxygen deprivation. Appl. Microbiol. Biotechnol. 68, 475–480. doi:10.1007/s00253-005-1900-y
Okino, S., Noburyu, R., Suda, M., Jojima, T., Inui, M., and Yukawa, H. (2008). An efficient succinic acid production process in a metabolically engineered Corynebacterium glutamicum strain. Appl. Microbiol. Biotechnol. 81, 459–464. doi:10.1007/s00253-008-1668-y
Orlowski, A., Vargas, L. A., Aiello, A., and Álvarez, B. V. (2013). Elevated carbon dioxide upregulates NBCn1 Na+/HCO3- cotransporter in human embryonic kidney cells. Am. J. Physiol. Renal Physiol. 305, F1765–F1774. doi:10.1152/ajprenal.00096.2013
Ozturk, S. S. (1996). Engineering challenges in high density cell culture systems. Cytotechnology 22, 3–16. doi:10.1007/BF00353919
Ozturk, S. S., and Palsson, B. O. (1991). Effect of medium osmolarity on hybridoma growth, metabolism, and antibody production. Biotechnol. Bioeng. 37, 989–993. doi:10.1002/bit.260371015
Park, S. A., Ko, A., and Lee, N. G. (2011). Stimulation of growth of the human gastric pathogen Helicobacter pylori by atmospheric level of oxygen under high carbon dioxide tension. BMC Microbiol. 11:96. doi:10.1186/1471-2180-11-96
Peters-Wendisch, P. G., Schiel, B., Wendisch, V. F., Katsoulidis, E., Möckel, B., Sahm, H., et al. (2001). Pyruvate carboxylase is a major bottleneck for glutamate and lysine production by Corynebacterium glutamicum. J. Mol. Microbiol. Biotechnol. 3, 295–300.
Pfizenmaier, J., and Takors, R. (2015). “Host mammalian cell,” in Industrial Biotechnology, eds C. Wittmann and J. Liao (Weinheim: Wiley-VCH Verlag).
Pronk, J. T., Yde Steensma, H., and Van Dijken, J. P. (1996). Pyruvate metabolism in Saccharomyces cerevisiae. Yeast 12, 1607–1633. doi:10.1002/(SICI)1097-0061(199612)12:16<1607:AID-YEA70>3.0.CO;2-4
Radoš, D. X. I., Turner, D. L., Fonseca, L. L., Carvalho, A. L., Blombach, B., Eikmanns, B., et al. (2014). The effect of CO2 on anaerobic succinate production by Corynebacterium glutamicum: carbon flux analysis by 13C-NMR. Appl. Environ. Microbiol. 80, 3015–3024. doi:10.1128/AEM.04189-13
Repaske, R., Repaske, A. C., and Mayer, R. D. (1974). Carbon dioxide control of lag period and growth of Streptococcus sanguis. J. Bacteriol. 117, 652–659.
Richard, L., Guillouet, S. E., and Uribelarrea, J.-L. (2014). Quantification of the transient and long-term response of Saccharomyces cerevisiae to carbon dioxide stresses of various intensities. Process Biochem. 49, 1808–1818. doi:10.1016/j.procbio.2014.07.020
Riedel, C., Rittmann, D., Dangel, P., Möckel, B., Petersen, S., Sahm, H., et al. (2001). Characterization of the phosphoenolpyruvate carboxykinase gene from Corynebacterium glutamicum and significance of the enzyme for growth and amino acid production. J. Mol. Microbiol. Biotechnol. 3, 573–583.
Ritchie, R. J., Nadolny, C., and Larkum, A. (1996). Driving forces for bicarbonate transport in the cyanobacterium Synechococcus R-2 (PCC 7942). Plant Physiol. 112, 1573–1584.
Sauer, U., and Eikmanns, B. J. (2005). The PEP-pyruvate-oxaloacetate node as the switch point for carbon flux distribution in bacteria. FEMS Microbiol. Rev. 29, 765–794. doi:10.1016/j.femsre.2004.11.002
Sechenov, M. (1889). Über die Konstitution der Salzlösungen auf Grund ihres Verhaltens zu Kohlensäure. Z. Phys. Chem. 4, 117–125.
Smith, K. S., and Ferry, J. G. (2000). Prokaryotic carbonic anhydrases. FEMS Microbiol. Rev. 24, 335–366. doi:10.1016/S0168-6445(00)00030-9
Spilimbergo, S., and Bertucco, A. (2003). Non-thermal bacteria inactivation with dense CO2. Biotechnol. Bioeng. 84, 627–638. doi:10.1002/bit.10783
Stretton, S., and Goodman, A. E. (1998). Carbon dioxide as a regulator of gene expression in microorganisms. Antonie Van Leeuwenhoek 73, 79–85. doi:10.1023/A:1000610225458
Stumm, W., and Morgan, J. J. (1995). Aquatic Chemistry: Chemical Equilibria and Rates in Natural Waters, 3rd Edn. New York, NY: Wiley.
Takors, R. (2012). Scale-up of microbial processes: impacts, tools and open questions. J. Biotechnol. 160, 3–9. doi:10.1016/j.jbiotec.2011.12.010
Takuma, S., Hirashima, C., and Piret, J. M. (2007). Dependence on glucose limitation of the pCO2 influences on CHO cell growth, metabolism and IgG production. Biotechnol. Bioeng. 97, 1479–1488. doi:10.1002/bit.21376
Talley, R. S., and Baugh, C. L. (1975). Effects of bicarbonate on growth of Neisseria gonorrhoeae: replacement of gaseous CO2 atmosphere. Appl. Microbiol. 29, 469–471.
Tashian, R. E. (1989). The carbonic anhydrases: widening perspectives on their evolution, expression and function. Bioessays 10, 186–192. doi:10.1002/bies.950100603
Tripp, B. C., Smith, K., and Ferry, J. G. (2001). Carbonic anhydrase: new insights for an ancient Enzyme. J. Biol. Chem. 276, 48615–48618. doi:10.1074/jbc.R100045200
Wahlund, T. M., Conway, T., and Tabita, F. R. (1996). Bioconversion of CO2 to ethanol and other compounds. ACS Div. Fuel Chem. Prepr. 41, 1403–1405.
Wieschalka, S., Blombach, B., Bott, M., and Eikmanns, B. J. (2012). Bio-based production of organic acids with Corynebacterium glutamicum. Microb. Biotechnol. 6, 87–102. doi:10.1111/1751-7915.12013
Yang, J., Dogovski, C., Hocking, D., Tauschek, M., Perugini, M., and Robins-Browne, R. M. (2009). Bicarbonate-mediated stimulation of RegA, the global virulence regulator from Citrobacter rodentium. J. Mol. Biol. 394, 591–599. doi:10.1016/j.jmb.2009.10.033
Zanghi, J. A., Schmelzer, A. E., Mendoza, T. P., Knop, R. H., and Miller, W. M. (1999). Bicarbonate concentration and osmolality are key determinants in the inhibition of CHO cell polysialylation under elevated pCO2 or pH. Biotechnol. Bioeng. 65, 182–191. doi:10.1002/(SICI)1097-0290(19991020)65:2<182:AID-BIT8>3.0.CO;2-D
Zelle, R. M., de Hulster, E., Kloezen, W., Pronk, J. T., and van Maris, A. J. A. (2010). Key process conditions for production of C4 dicarboxylic acids in bioreactor batch cultures of an engineered Saccharomyces cerevisiae strain. Appl. Environ. Microbiol. 76, 744–750. doi:10.1128/AEM.02396-09
Zhang, X., Wang, X., Shanmugam, K. T., and Ingram, L. O. (2010). L-Malate production by metabolically engineered Escherichia coli. Appl. Environ. Microbiol. 77, 427–434. doi:10.1128/AEM.01971-10
Zhu, M. M., Goyal, A., Rank, D. L., Gupta, S. K., Vanden Boom, T., and Lee, S. S. (2005). Effects of elevated pCO2 and osmolality on growth of CHO cells and production of antibody-fusion protein B1: a case study. Biotechnol. Prog. 21, 70–77. doi:10.1021/bp049815s
Keywords: bicarbonate, carbon dioxide, production process, regulation, carboxylation, decarboxylation
Citation: Blombach B and Takors R (2015) CO2 – intrinsic product, essential substrate, and regulatory trigger of microbial and mammalian production processes. Front. Bioeng. Biotechnol. 3:108. doi: 10.3389/fbioe.2015.00108
Received: 22 May 2015; Accepted: 13 July 2015;
Published: 03 August 2015
Edited by:
Hilal Taymaz Nikerel, Bogazici University, TurkeyReviewed by:
Anshu Bhardwaj, Council of Scientific and Industrial Research, IndiaPeter Neubauer, Technische Universität Berlin, Germany
Antonino Baez, Autonomous University of Puebla, Mexico
Copyright: © 2015 Blombach and Takors. This is an open-access article distributed under the terms of the Creative Commons Attribution License (CC BY). The use, distribution or reproduction in other forums is permitted, provided the original author(s) or licensor are credited and that the original publication in this journal is cited, in accordance with accepted academic practice. No use, distribution or reproduction is permitted which does not comply with these terms.
*Correspondence: Ralf Takors, Institute of Biochemical Engineering, University of Stuttgart, Allmandring 31, 70569 Stuttgart, Germany,dGFrb3JzQGlidnQudW5pLXN0dXR0Z2FydC5kZQ==