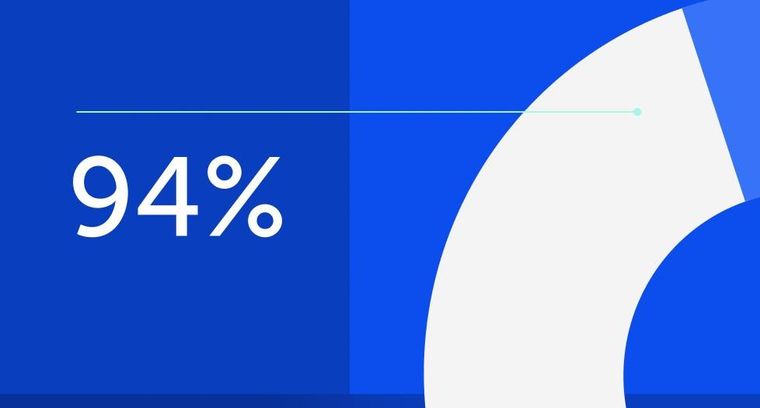
94% of researchers rate our articles as excellent or good
Learn more about the work of our research integrity team to safeguard the quality of each article we publish.
Find out more
REVIEW article
Front. Behav. Neurosci., 09 April 2025
Sec. Motivation and Reward
Volume 19 - 2025 | https://doi.org/10.3389/fnbeh.2025.1553470
This article is part of the Research TopicBrain reward and aversion circuits: progress review on current and future breakthroughsView all 6 articles
The dorsal raphe nucleus is a critical node for affective and motivated circuits in the brain. Though typically known as a serotonergic hub, the dorsal raphe nucleus is also highly enriched in a variety of neuropeptides. Recent advances in biotechnology and behavioral modeling have led to a resurgence in neuropeptide research, allowing investigators to target unique peptide systems with unprecedented clarity. Here, we review and discuss multiple neuropeptide systems in dorsal raphe and consider how their activity may contribute to reward and aversion. While this is not an exhaustive review, this short overview will highlight the many opportunities available to refine our understanding of multiple dorsal raphe neuropeptides. By more thoroughly studying dorsal raphe neuropeptides, we will reveal novel pathways to design more effective therapeutics and tailor treatments for millions of patients.
The dorsal raphe nucleus (DRN) in the midbrain has been implicated in reward processing, mood regulation, and learning (Luo et al., 2015; Luo et al., 2016; McDevitt and Neumaier, 2011). It is one of seven raphe nuclei along the midsagittal plane of the brainstem that are characterized by the presence of serotonin positive (5-hydroxytryptamine; 5-HT) cell bodies (Dahlström and Fuxe, 1964; Taber et al., 1960). Of the raphe nuclei, the DRN provides the largest source of serotonin to the forebrain (Vertes, 1991). As a result, the DRN serotonergic system has been the subject of intense interest, particularly in the realm of affect and motivation. Serotonin was first discovered in 1948 as a vasoconstrictor (Rapport et al., 1948a; Rapport et al., 1948b; Rapport et al., 1948c; Twarog and Page, 1953). Further investigation of serotonergic cells within the raphe found extensive projections throughout the forebrain and cortex, indicating that it could play an important modulatory role in affect, motivation, and learning (Dahlström and Fuxe, 1964). Decades of research more or less corroborate that hypothesis, with the advent of SSRIs and other serotonergic drugs now used regularly in the clinic to treat mood disorders like depression, eating disorders, and anxiety. While clearly a critical node within affective neural circuits, the myopic focus on serotonin has occluded an appreciation for the full neurochemical landscape present within the DRN. Studies find that approximately one to two thirds of DRN neurons are non-serotonergic (Castro et al., 2021; Wiklund et al., 1981), but are neuropeptidergic, leaving unresolved how a significant portion of the dorsal raphe complex may or may not be involved in behavioral phenotypes. For example, despite the presence and high expression of several neuropeptides like the endogenous opioids, cholecystokinin (CCK), and neuropeptide Y (NPY), almost nothing is known regarding the function of these and other neuropeptides in this region.
Neuropeptides are small proteins derived from post-translational processing of precursor proteins that are released from neurons in dense core vesicles to act on receptors, typically G-protein coupled receptors (GPCRs) (Russo, 2017). There are at least 50 neuropeptides expressed in the mammalian brain, each with varying levels of understanding of their physiological roles (Eiden et al., 2022). These signaling molecules can have significant modulatory effects on neuronal transmission and resulting behavioral phenotypes. In many cases, neuropeptides are co-released by neurons along with classical neurotransmitters such as glutamate, GABA, dopamine, or serotonin (Eiden et al., 2022; Nusbaum et al., 2017). Co-release of neuropeptides and neurotransmitters enable highly nuanced circuit flexibility, as peptides can have both neurotransmitter dependent or independent mechanisms of action. Details regarding co-transmission and other peptide-specific signaling mechanisms are reviewed thoroughly elsewhere (Eiden et al., 2022; Nusbaum et al., 2017; Russo, 2017; Svensson et al., 2019), but are critical elements to consider in the context of neuropeptide function in the DRN.
This review aims to highlight the current literature on a selection of neuropeptides within the DRN and describe their known anatomical, physiological, and functional characteristics. Below we outline what is known about the general structure and connections of the DRN, we then summarize the current literature of specific neuropeptides and their functional effects in this region. Overall, we aim to emphasize that DRN neuropeptides act both within and beyond classic serotonergic systems, and provide important contributions to reward, motivation, and affect that could be leveraged in the treatment of neuropsychiatric conditions.
The DRN can be divided into medial and lateral regions as well as rostral and caudal portions. It is located directly below the cerebral aqueduct beginning in the midbrain at the caudal border of the oculomotor nuclei and extending to the mid-pons where the aqueduct meets the fourth ventricle (Franklin and Paxinos, 2007; Jacobs and Azmitia, 1992). It is located directly along the midline with the medial portion extending from the aqueduct superiorly to surround the medial longitudinal fasciculi (MLF) inferiorly. The lateral wings form the largest portion of the DRN and extend out to meet the ventrolateral periaqueductal gray (vlPAG) at the level of the trochlear nuclei until just below the fourth ventricle. Importantly the border between the DRN and vlPAG is not consistently defined; early studies investigating the vlPAG included portions of what would now be considered the DRN (Kyuhou and Gemba, 1999; Monassi et al., 1999; Siegel and Pott, 1988; Yaksh et al., 1976). Recent studies have begun to treat the vlPAG and lateral DRN (LDRN) as a complex of cells that work together, perhaps acting as a functionally unique transition zone (Dougalis et al., 2012; Liu et al., 2023; Xie et al., 2023). These sorts of transitions zones have been described in a variety of mesocorticolimbic systems, including caudal accumbens into rostral bed nucleus of the solitary tract or portions of the extended amygdala (Thompson and Swanson, 2010; Zahm et al., 2013). But for the purposes of this review, we will refer to the LDRN as separate from the vlPAG and adhere to the annotations of Franklin and Paxinos to approximate this border (Figure 1; Franklin and Paxinos, 2007).
Figure 1. Summary of expression patterns of selected neuropeptides throughout the DRN. The coronal sections and annotations are based on the Franklin & Paxinos mouse brain atlas and depict the DRN from –4.36 to –4.72 Bregma. Opioid peptides are depicted as circles (Dynorphin: purple, Enkephalin: green; Nociceptin: blue). The additional peptides discussed in depth are depicted as triangles (Corticotropin releasing factor: yellow; Neuropeptide Y: pink; Galanin: red). The peptides discussed in the Other Neuropeptides section are depicted as squares (Cholecystokinin: green; Neurotensin: orange; Somatostatin: light blue; Substance P: dark blue). Abbreviations: Aq: Aqueduct, VLPAG: ventrolateral periaqueductal grey, DRL: dorsal raphe lateral, DRD: dorsal raphe dorsal, DRV: dorsal raphe ventral, PDR: postero-dorsal raphe, 4N: trochlear nucleus, mlf: medial longitudinal fasciculus.
As would be expected for the primary source of serotonin to the brain, the DRN is highly interconnected with many brain regions with known roles in reward and aversion, including the nucleus accumbens (NAc) (Castro et al., 2021; Pomrenze et al., 2022; Vertes, 1991), ventral tegmental area (VTA) (Abraham et al., 2022), amygdala (Marcinkiewcz et al., 2016; Ren et al., 2018; Ritchie et al., 2024), and PAG (Xu et al., 2021). The projections are generally topographically organized, with more rostral DRN neurons projecting to cortical and subcortical regions and caudal neurons projecting to septohippocampal structures (Commons, 2020). The nonserotonergic neurons have not been specifically characterized for potential functional subgroups yet (Huang et al., 2019). Most tracing studies in the literature have focused on serotonergic cells, but recent work has made efforts to more thoroughly characterize the inputs and outputs of the entire DRN by comparing glutamatergic and GABAergic cell types (Xu et al., 2021). This in-depth mapping of whole-brain connections of the DRN highlights the complexity of interconnectedness present in this highly heterogenous nucleus. For more thorough review of the current understanding of dorsal raphe circuitry we suggest the recent reviews by Steinbusch and colleagues (Steinbusch et al., 2021) or Zhang and colleagues (Zhang et al., 2024a).
As early as the studies of Cajal, the neurons of the DRN as it is known today were grouped into three to four morphological categories: fusiform, multipolar (stellate), and ovoid or triangular cells (Andrade and Haj-Dahmane, 2013; Baker et al., 1990; Michelsen et al., 2007). These neurons have divergent and spiny dendrites with fibers concentrating in either ascending or descending bundles. To date, most efforts into the anatomical classification of dorsal raphe neurons have focused on subtyping serotonin cells, the majority of which are clustered densely along the midline and are present throughout the lateral wings (Andrade and Haj-Dahmane, 2013). Though not well described, one study found that nonserotonergic neurons from the DRN had fewer dendritic spines than serotonin-positive cells. Additionally, nonserotonergic neurons had more heterogenous electrophysiological properties than the slow rhythmic firing that is often used to characterize serotonergic cells (Li et al., 2001). However, more recent studies have found that even serotonin neuron firing patterns may be more heterogenous than traditionally thought (Calizo et al., 2011; Schweimer et al., 2011). These studies underscore the importance of parsing how 5-HT and non-5-HT cells in the DRN can be functionally distinguished from each other.
The advent of single cell sequencing has recently led to several studies attempting a more sophisticated characterization of neuronal subtypes within the DRN. But unfortunately, the focus has once again proven limited with the majority using chemical or genetic manipulations to select for only serotonergic cells in their downstream analyses of neurotransmitter expression (Okaty et al., 2020; Ren et al., 2019), rather than considering other neuronal cell types that are present in the DRN. While there is an active and evolving conversation attempting to define and categorize DRN neurons, consensus has not yet been reached (Andrade and Haj-Dahmane, 2013; Commons, 2020; Huang et al., 2019; Monti, 2010). Whether they are divided by transcriptional profile, projection pattern, or anatomical location, there appears to be a marked heterogeneity to these neurons suggesting complex and integrative functional roles that cannot be simplified to 5-HT expression alone.
Many studies have found expression of neuropeptides such as neuropeptide Y (NPY), Substance P, dynorphin, enkephalin, and corticotropin releasing factor expressed throughout the DRN (Commons et al., 2003; de Quidt and Emson, 1986; Fu et al., 2010; Hökfelt et al., 1977; Hökfelt et al., 1978; Uhl et al., 1979a). However, little effort has been made to distinguish their functions apart from the serotonergic neurons in this region. Recent evidence points to neuropeptides within the DRN having powerful effects on motivation independent of serotonin effects (Castro et al., 2021; Li et al., 2016; Nectow et al., 2017). Here we have summarized what has been revealed thus far about neuropeptide expression and function within the DRN and emphasize the importance of understanding how nonserotonergic signals act in concert and in parallel to traditional serotonergic systems. Due to the lack of specific studies, we have focused on four peptide families with more substantial literature on their role in the DRN and included a final section of “Other Neuropeptides” describing peptides with less extensive investigation into their roles in DRN circuitry. Due to the lack of quantitative data on many of these peptides in the DRN, we have summarized their general expression patterns of their mRNA throughout the DRN in Figure 1 based on Allen Brain Atlas in situ data (Lein et al., 2007).
The endogenous opioid system is comprised of the four precursor peptides proopiomelanocortin (POMC), preproenkephalin (Penk) preprodynorphin (Pdyn), and prepronociceptin (Pnoc). Correspondingly, there are four Gi-coupled receptors, mu opioid receptor (MOPR), delta opioid receptor (DOPR), kappa opioid receptor (KOPR), and nociceptin opioid receptor (NOPR). Decades of research show that opioids can powerfully influence affective and motivated behaviors via their actions in cortex (Castro and Berridge, 2017; Cole et al., 2024; Mena et al., 2013), striatum (Al-Hasani et al., 2015; Bakshi and Kelley, 1993; Castro and Berridge, 2014; Castro et al., 2021; Guy et al., 2011; Massaly et al., 2019; Ragnauth et al., 2000; Toddes et al., 2021), amygdala (Lichtenberg and Wassum, 2017; Nygard et al., 2016), ventral pallidum (Smith and Berridge, 2007; Smith et al., 2009; Wulff et al., 2019), ventral tegmental area (Bals-Kubik et al., 1993; Echo et al., 2002; Margolis et al., 2014; Thomas et al., 2022), and periaqueductal gray (Brandão, 1993; Sante et al., 2000). Like these mesocorticolimbic regions, dorsal raphe is highly enriched in opioids. Both enkephalin and nociceptin appear to be primarily expressed in the lateral wings, extending into vlPAG (Castro et al., 2021). In contrast, dynorphin is exclusively expressed along the midline (Figure 1) and appears to partially colocalize with serotonergic neurons (Pomrenze et al., 2022). Receptor mRNA for MOPR, KOPR and NOPR has been found throughout dorsal raphe, with NOPR additionally having strong expression along the midline (Mansour et al., 1994a; Neal et al., 1999). Surprisingly, DOPRs do not appear to be endemic to dorsal raphe, although that does not preclude potential DOPR expression on incoming terminals. Oppositely, while MOPR mRNA is expressed in a large proportion of dorsal raphe neurons (about 30%) (Castro et al., 2021), receptor binding is fairly low (Mansour et al., 1994b). This could indicate that MOPR proteins may be enriched on downstream terminals rather than cell bodies. These MOPR-expressing DRN neurons have been found to be largely non-overlapping with serotonergic markers, but rather distributed between glutamatergic and GABAergic cells (Castro et al., 2021; Welsch et al., 2023b). Regardless, ample evidence indicates that the dorsal raphe nucleus is enriched in endogenous opioids, suggesting a potential role for this system in regulating behavioral phenotypes.
Dorsal midbrain opioids have been known to modulate behavior for quite some time, but most of these effects have been attributed to the periaqueductal gray (PAG), with the most well-characterized function being the descending modulation of nociception. However, a reevaluation of the literature from the 1980s and 1990s indicates that papers that ostensibly targeted the ventrolateral PAG very likely hit portions of the lateral dorsal raphe (Fardin et al., 1984; Monassi et al., 1999; Sim and Joseph, 1991; Smith et al., 1994). Here, opioid agonists were generally shown to suppress evoked behaviors, such as suppressing electrically stimulated defensive behaviors in cats, vocalizations in guinea pigs, or food intake in rats (Jenck et al., 1987; Kyuhou and Gemba, 1999; Siegel and Pott, 1988). More recent studies using specific targeting have focused mostly on the opioid receptors rather than the peptides. In general, MOPR activation in the DRN appears to potentiate reward behaviors, and produce antinociception (Castro et al., 2021; Ferreira and Menescal-de-Oliveira, 2014; Welsch et al., 2023b). Negative states such as withdrawal and food deprivation may impair the MOPR-expressing neurons in the DRN (Welsch et al., 2023a). Additionally morphine withdrawal studies have indicated that opioid receptors interact with the DRN serotonergic system to modulate behavioral outputs as well as neuronal function (Jolas and Aghajanian, 1997; Li et al., 2024; Lunden and Kirby, 2013; Welsch et al., 2023a). Similar to its role in other regions of the reward system, KOPR agonism in the DRN has been characterized as an opponent process relative to MOPR. For example, whereas MOPR stimulation increases serotonin release, KOPR stimulation suppresses it (Tao and Auerbach, 2005; Tao et al., 2007). NOPR DRN effects have yet to be fully explored, with just a few studies indicating that it is modulated during state changes and antagonism may be protective against antinociceptive tolerance to morphine (Ge et al., 2007; Le Maître et al., 2013; Przydzial et al., 2010).
Unlike opioid receptors, which have been readily targetable with selective pharmacology for decades, opioid peptides have been far more difficult to study. Enkephalin in particular poses difficulty as there are several splice variants that can have different receptor affinities that can have profound effects on functional interpretability. However, recent developments in opioid peptide technology, such as the development of preproenkephalin-cre (Penk-cre) mice and CRISPR-Cas9 knockdown viral vectors, have allowed for the discovery of unique circuit mechanisms important for motivated behaviors. For example, dorsal raphe enkephalinergic projections to the NAc have been found to modulate reward consumption (Castro et al., 2021). Parallel opioid circuit mechanisms have also been described. For example, infusion of nociceptin into the DRN decreases 5-HT neuron activity and downstream serotonin release in the NAc (Nazzaro et al., 2009; Tao et al., 2007). Similarly, a population of dynorphin expressing DRN neurons were found to modulate opioid withdrawal-induced social deficits via KOPR-mediated serotonin release to the NAc (Land et al., 2009). Dynorphin, and its associated KOPR, has been additionally implicated in regulating stress responses, as stress manipulations and CRF injections into the DRN caused dynorphin-dependent KOR activation (Land et al., 2008). Other populations of DRN dynorphin neurons projecting to the VTA activate KOPR to modulate dopamine-dependent enhancement of drug reward learning (Abraham et al., 2022). Ultimately, while several local and circuited-based opioidergic mechanisms have been uncovered over the years, there remains extensive gaps in knowledge on their precise cellular mechanisms. Moving forward, it is imperative that we continue to isolate these mechanisms, as well as integrate their function with known roles for other complementary systems, such as the serotonin and GABA/glutamate.
Corticotropin releasing factor (CRF) has long been studied in the context of stress and motivation (Hupalo et al., 2019; Valentino et al., 2010). First identified in dorsal raphe in 1984 (Merchenthaler, 1984), the peptide and its receptors (CRF1 and CRF2) have been extensively characterized in multiple vertebrates (Alderman and Bernier, 2007; Chappell et al., 1986; Lim et al., 2005; Mancera et al., 1991; Palkovits et al., 1985; Shu et al., 2015). The expression patterns in the DRN appear to be state-dependent, with stress or aversive states modulating expression and localization of both the CRF peptide and its receptors (Chappell et al., 1986; Waselus et al., 2009). In general, CRF precursor mRNA has been found in the medial DRN. In contrast, CRF receptors have a more complex expression pattern that is dependent on the receptor subtype. Specifically, CRF1 is sparsely present and partially colocalized with 5-HT neurons, whereas CRF2 mostly colocalizes with 5-HT in rostral DRN and colocalizes with GABAergic cells in caudal regions (Day et al., 2004).
Early research indicated that DRN CRF was highly sensitive to stressors, potentially indicating that it may interact with serotonin systems to modulate reward or aversive processing. Support for this hypothesis gained traction in the 1990s and 2000s, wherein CRF stimulation was shown to modulate serotonin output and neural activity (Calizo et al., 2011; Kirby et al., 2000; Kovács et al., 2025; Lowry et al., 2000; Lukkes et al., 2009b; Pernar et al., 2004; Price et al., 1998; Thomas et al., 2003). Further work revealed that CRF primarily impacted serotonin activity by increasing GABA release (CRF1 mediated) and sensitivity (CRF1 and CRF2 mediated), as well as effecting other surrounding cell types (Kirby et al., 2008). Functionally, strong associations between generalized stress responses and DRN CRF systems have been well described (Marcinkiewcz et al., 2016). For example, restraint and forced swim stress models elevate dorsal raphe c-fos expression in a CRF dependent manner (Roche et al., 2003; Summers et al., 2018). Maternal aggression and novel object exploration is reduced after CRF administration, and CRF alone is sufficient to generate a conditioned place avoidance. These results potentially point toward CRF increasing anxiety and goal directed avoidance (Clark et al., 2007; Gammie et al., 2004; Land et al., 2008; Wood et al., 2013). Correspondingly, CRF antagonism prevents behavioral deficits due to strong stressors, such as normally increased defensive behaviors in socially defeated hamsters (Cooper and Huhman, 2007) or social deficits after early-life social isolation (Bledsoe et al., 2011; Lukkes et al., 2009a). These and other studies implicating CRF as an important modulator of stress resilience in other brain regions (Chudoba and Dabrowska, 2023) warrant further study of how the DRN CRF system promotes stress coping behaviors. Beyond endogenous or social stressors, DRN CRF also appears to modulate responses to various drugs of abuse, particularly in the context of stress. This appears to hold true for a variety of drugs, including alcohol (Hwa et al., 2013; Knapp et al., 2011; Quadros et al., 2014), psychostimulants (Reinbold Scholl et al., 2014; Verheij et al., 2018; Zorrilla et al., 2012), and opioids (Lunden and Kirby, 2013; Ritchie et al., 2024; Staub et al., 2012). Future work incorporating polysubstance models would be particularly interesting, as this may reveal unexpected synergistic or antagonistic phenotypes.
Though beyond the scope of this review, it is notable that there appears to be regional and anatomical heterogeneity of CRF effects across the DRN (Day et al., 2004; Li et al., 2021; Salvatore et al., 2018; Valentino et al., 2001; Waselus et al., 2005; Waselus et al., 2009). Such functional localization has been observed with other neuropeptides (e.g., opioids) in several other brain regions (Al-Hasani et al., 2015; Castro and Berridge, 2014; Castro and Berridge, 2017; Castro et al., 2016; Parker et al., 2019; Peciña and Berridge, 2005; Smith and Berridge, 2005), but whether these effects map on to DRN-mediated behavioral phenotypes remains to be tested.
Galanin is an evolutionarily conserved peptide that is typically associated with areas like the hypothalamus and locus coeruleus. However, multiple neuroanatomical mapping studies across mammalian and non-mammalian vertebrates show significant galanin peptide and receptor expression in DRN (Figure 1; Araneda et al., 1999; Gundlach et al., 1990; Holmqvist and Carlberg, 1992; Lu et al., 2005b; Melander et al., 1986; Mennicken et al., 2002; Skofitsch and Jacobowitz, 1985; Smith et al., 1994; Sutin and Jacobowitz, 1988). In DRN, galanin primarily colocalizes with serotonergic neurons (Xu and Hökfelt, 1997). However, there is also significant innervation of galanin in DRN that appears to be separate from DRN-derived galanin, indicating that there may be multiple galanin subsystems associated with this region (Xu et al., 1998). Support for this idea can be observed with exogenous galanin infusion in DRN. Here, galanin inhibits 5HT+ neurons and decreases the expression of 5HT1A receptor and galanin mRNA in cell bodies in a GalR3, but not GalR1 dependent manner (Kehr et al., 2002; Razani et al., 2000; Sharkey et al., 2008; Xu et al., 1998). These effects are thought to arise via differential expression of the receptors on presynaptic (GalR1) versus postsynaptic (GalR2/3) membranes (Swanson et al., 2005). However, it should be appreciated that this system is likely highly nuanced, as GalR2 agonism and GalR1/GalR3 antagonism both reduce anxiety and depressive-like phenotypes (de Souza et al., 2018; Lu et al., 2005a; Morais et al., 2016; Silote et al., 2013).
Regardless of the precise mechanism, galanin administration in DRN generally appears to ameliorate aversive phenotypes (García-Durán et al., 2021). Notably, galanin and galanin receptor expression is dynamic and responsive to stress and therapeutic interventions. For example, painful capsaicin exposure increases DRN galanin (Okere and Waterhouse, 2006), traumatic brain injury increases galanin and galanin receptor mRNA (Kawa et al., 2016; Kawa et al., 2020), and galanin receptor mRNA increases after chronic mild stress (Wang et al., 2016). From a clinical perspective, this increase in galanin may be part of a larger response mechanism to rebalance galanin function to increase GalR2 activity and decrease Gal1R activity. For example, the commonly prescribed anti-depressant fluoxetine (a selective serotonin reuptake inhibitor) and electroconvulsive shock increases DRN galanin and GalR2 binding, whereas GalR2 knockout mice show enhanced depressive-like symptoms (Lu et al., 2005a; Lu et al., 2008). In tandem, DRN GalR1 expression is downregulated after stress, and its recruitment appears to account for increases in avoidance-like behaviors (Medel-Matus et al., 2017; Morais et al., 2016). In sum, the galanin system within the DRN has significant control over appetitive and aversive behaviors, with functional interactions between multiple receptor subtypes biasing behavioral responses toward one phenotype or the other. Future studies should clarify how these galanin systems converge in the DRN to impact behavior, as well as dissociate how incoming versus outgoing galanin systems regulate behavioral phenotypes.
Early studies established the presence of Neuropeptide Y (NPY) in the DRN across species (Figure 1), including rats (Smith et al., 1994; Yamazoe et al., 1985), lemurs (Bons et al., 1990), newts (Perroteau et al., 1988), and lampreys (Chiba, 1999). NPY in the DRN often colocalizes with serotonin (5-HT), suggesting functional interactions (Blessing et al., 1986). NPY exerts its effects in the brain mainly through the NPY receptor subtypes Y1, Y2, Y4, Y5, and Y6 (Díaz-Cabiale et al., 2011). Of these receptors, mRNA for Y1, Y2, and Y5 have been found throughout the DRN (Díaz-Cabiale et al., 2011; Gustafson et al., 1997; Kishi et al., 2005; Parker and Herzog, 1999). Detailed colocalization studies are lacking but Y2 receptor binding is enriched on glutamatergic neurons within the DRN, suggesting a possible relationship (Yoon et al., 2013).
NPY exerts diverse effects in the DRN, with implications for stress, feeding, and social behaviors. Receptor-level studies reveal distinct roles for Y1 and Y2 receptors in the DRN. Functional studies suggest that NPY modulates GPCR but not amino acid signaling via Y2 in DRN neurons (Kombian and Colmers, 1992) with Y2 receptor activation suppressing food intake via glutamatergic neurons (Nectow et al., 2017). Y1 receptor activation leads to reduced maternal care in ad libitum-fed dams and suppressed male sexual behavior in fed mice (Inaba et al., 2016; Muroi and Ishii, 2015). Functional effects of Y5 receptors in the DRN have yet to be tested. Recent work found a unique population of NPY-expressing neurons in the DRN that are activated by stress and when they were exogenously activated through either chemogenetics or optogenetics they were able to improve stress resilience, including alleviation of stress-induced hypophagia, by interacting with Y2 receptors in the PVT and lateral hypothalamus (Zhang et al., 2024c). Additional work has found reduced DRN NPY levels in chronic pain and social fear conditioning models, further highlighting its critical role in DRN-mediated behavioral regulation (Hamann et al., 2022; Upadhya et al., 2009). Collectively, these data indicate that NPY within the DRN may act as an “aversion buffering” system. During endogenous recruitment, NPY may facilitate resilience-type phenotypes, whereas its loss (e.g., during chronic pain) allows for unusually high aversion. Future work is needed to elucidate the therapeutic potential of NPY within the DRN.
Although the DRN has been the focus of hundreds of studies, as this review illustrates, there is a significant paucity for what we know regarding DRN neuropeptides. Here, we briefly describe several poorly characterized DRN neuropeptides that are well known to impact reward and aversion in other brain circuits, including cholecystokinin, neurotensin, somatostatin, and Substance P.
Cholecystokinin (CCK) peptide was originally isolated from the gastrointestinal system, but is found throughout the central nervous system and densely in the DRN in rodents (Innis et al., 1979) and birds (Lovell and Mello, 2011). Functional CCK receptors reside within the DRN, and CCK application activates DRN 5-HT neurons primarily via CCKA receptor (Boden et al., 1991). However, CCK itself does not colocalize with 5HT-containing neurons (van der Kooy et al., 1981). There is some variable colocalization of CCK with neurotensin and dopamine within the DRN (Seroogy et al., 1988). This expression pattern suggests that CCK-expressing neurons may be a distinct subpopulation within the DRN that could have unique effects on behavior. Limited behavioral studies have shown that DRN CCK has diverse behavioral effects. For example, CCK-positive neurons have been found to project from the arcuate nucleus to 5-HT cells within the DRN (Sim and Joseph, 1991) and systemic antagonism of CCKA receptor potentiates intra-DRN 5-HT1A agonist-induced food intake (Currie and Coscina, 1995) and activation of DRN CCK+ neurons suppressed food intake (Chowdhury et al., 2025). However, direct administration of CCK to the DRN has no effect on food intake (Blevins et al., 2000), suggesting DRN CCK receptors may have divergent roles from the CCK peptide produced by DRN cell bodies and released downstream elsewhere. The mRNA for CCK is increased in the DRN following stress such as social isolation, chronic pain, or nocebo nausea (Del Bel and Guimarães, 1997; Keay et al., 2021; Zhang et al., 2024b). These DRN CCK-producing neurons have been found to project to the paraventricular thalamus, which is an important node for emotional and motivated behaviors (Bhatnagar et al., 2000; Otake, 2005). Altogether, while evidence indicates a complex role for CCK in DRN, more studies are needed to clarify its precise contributions and mechanisms of action.
Neurotensin expression in DRN was first described in the 1970s and 80s (Beitz, 1982; Jennes et al., 1982; Moyse et al., 1987; Sutin and Jacobowitz, 1988; Uhl et al., 1979b). Its receptors were mapped several decades later, with neurotensin receptor 2, but not neurotensin receptor 1, expressed in DRN (Boudin et al., 1996; Sarret et al., 2003). While its overall pharmacology is complex (Gereau et al., 2023), the net effect of neurotensin stimulation in DRN is to increase the excitability of serotonin neurons (Jolas and Aghajanian, 1996), particularly in the ventromedial portion of DRN. Functionally, neurotensin stimulation increases passive avoidance (Shugalev et al., 2005; Shugalev et al., 2007) and is decreased after adolescent ethanol intake (McClintick et al., 2015). However, intracerebroventricular neurotensin blunts stress evoked increases in serotonin biomarkers, leaving unresolved whether neurotensin primarily increases or decreases raphe activity.
Like neurotensin, descriptions of somatostatin expression in DRN of multiple species can be traced back to the 1980s (Araneda et al., 1999; Cooper et al., 1981; Cotter and Laemle, 1987; Fu et al., 2010; Smith et al., 1994; Spangler and Morley, 1987; Taber-Pierce et al., 1985; Weindl et al., 1984). While somatostatin likely has direct functional effects in DRN, to date very few studies have examined specific roles for the different receptor subtypes. This largely forces us to speculate about potential functions. For example, systemic administration of a somatostatin receptor 4 (SSTR4) agonist leads to an anxiolytic phenotype and increases c-fos in DRN (Scheich et al., 2016), perhaps indicating that somatostatin may promote serotonin activity. In cortex, hippocampus, and hypothalamus slices somatostatin induces the release of 5-HT (Tanaka and Tsujimoto, 1981), suggesting it would do the same in DRN cell bodies, but this remains to be tested. Similarly, ventrolateral PAG/lateral DRN infusions of SST reduces thermal nociception, again reducing aversive responses (Helmchen et al., 1995). In line with the concept that DRN somatostatin may reduce aversive processing, somatostatin, SSTR1, and SSTR2 gene expression is reduced after adolescent ethanol intake (McClintick et al., 2015). Excessive ethanol intake is highly correlated with increased pain and anxiety-like behaviors, but whether there is a causal role for somatostatin in DRN on these psychological phenotypes remains untested.
Substance P is a Gq-coupled receptor that has broad expression throughout the brain. While the peptide is predominantly confined to the ventrolateral PAG, it does infiltrate the DRN (Figure 1) (Baker et al., 1991; Magoul et al., 1988; Smith et al., 1994). The neurokinin receptors (NK1, NK2, NK3) are also expressed in DRN, with NK1 being particularly enriched in DRN (Lacoste et al., 2006; Léger et al., 2002; Maeno et al., 1993). Curiously, both agonism and antagonism of NK1 receptors have been shown to increase serotonin neuron excitability (Conley et al., 2002; Haddjeri and Blier, 2001; Liu et al., 2002; Santarelli et al., 2001), perhaps suggesting that different receptors or cell types may dramatically shift ultimate physiology (Commons and Valentino, 2002; Valentino et al., 2003). Broadly, the Substance P system has been associated with various disease states, including depression and chronic pain (Adell, 2004). It is therefore somewhat surprising that comparatively little has been done to assess how Substance P/NK receptors functionally contribute to behavioral phenotypes. While general antagonism or knockout of NK1 receptors increases serotonin transmission and reduces aversive behavioral profiles, causally linking these phenomena have yet to be tested (Santarelli et al., 2001; Santarelli et al., 2002; Schank et al., 2015).
The dorsal raphe nucleus is an extraordinarily complex structure that has been implicated in mood, affect and motivation for decades. Though initially described in terms of its serotonergic architecture, further anatomical analyses now include the lateral wings and non-serotonergic neuropeptidergic circuits. Unfortunately, despite conscientious descriptions of dorsal raphe neuropeptide anatomy and convincing evidence that neuropeptides can profoundly shape behavioral phenotypes in other limbic brain circuits, comparatively little has been done to study dorsal raphe neuropeptides. By far the most extensive literature relates to the role of CRF, yet even these studies are predominantly framed from a serotonin-centric perspective. We have illustrated this point in Table 1, where we have summarized the currently known effects of each of the discussed neuropeptides in the DRN on either rewarding or aversive behaviors as well as their known effects on serotonin neuron excitability or release. It should be appreciated that all of the peptides have been at least studied in relation to their effect on serotonin, but not on their DRN-specific roles in behavior. This myopic approach could potentially limit our appreciation for how neuropeptides systems may contribute to behavioral phenotypes independent of, or perhaps in parallel with, serotonin.
Table 1. Summary of effects of DRN neuropeptides on rewarding or aversive behaviors and 5-HT signaling.
As experimental neuroscience technology continues to develop, it is imperative that we appreciate how neuropeptides are directly impacting physiology and behavior. Recent advances in biotechnology make it possible to study these neuropeptides with unprecedented specificity in behavioral models. The advent of CRISPR-Cas9 has allowed for the specific knockdown of precursor mRNA for peptides in combination with genetic Cre mouse lines. This technology provides the opportunity to investigate their role in behavioral paradigms and physiological functions in specific circuits or cell types. Previous interrogation of neuropeptidergic circuits was accomplished through pharmacology. Though receptor selective, the inability to target specific cell types pre- or post-synaptically has always limited interpretability. Fortunately, the recent development of DARTs (drugs acutely restricted by tethering) directly address this major confound. Specifically, DARTs use a bacterial enzyme to capture and tether drugs to defined cell surfaces, allowing researchers to pharmacologically target specific proteins on specific cells (Shields et al., 2017; Shields et al., 2024). As this technology continues to be optimized and used in more model systems, it will be interesting to see how it shapes our understanding of neuropeptide signaling. Anatomically, the last decade of in situ hybridization techniques have advanced to allow for much higher resolution and throughput of transcriptomic studies. Since many peptides can be difficult to measure (due to fast degradation, small molecular size, or natural biochemical instability), approaches targeting the mRNA have allowed for much more robust localization within neural circuits with single-cell resolution (DeLaney et al., 2018). Most recently the development of neuropeptide biosensors has invigorated neuropeptide research (Dong et al., 2024; Zhou et al., 2024; Wang et al., 2023). These fluorescent GPCR sensors allow for the detection of neuropeptide binding on a precise sub-second timescale with single cell resolution. Notably, a suite of sensors based on opioid receptors have been developed and validated: δLight, μLight, κLight (Dong et al., 2024) and NOPLight (Zhou et al., 2024). The implementation of these tools is only in its infancy, but they have already been used to confirm that dynorphin is released in the NAc shell in response to aversive stimuli such as a foot shock and revealed a shift in dynorphin dynamics throughout fear learning, wherein the κLight signal became stronger in response to the cue rather than the shock over more trials (Dong et al., 2024). This insight into the release dynamics of dynorphin during behavior can also be applied to the DRN to elucidate the contribution neuropeptides have to its roles in reward and aversion. These and other neuropeptide biosensors are poised to push the field forward and as they are optimized and implemented in various models, it will lead to a deeper understanding of neuropeptide dynamics throughout the brain (Dong et al., 2024; Siuda et al., 2015; Wang et al., 2023; Zhou et al., 2024).
Collectively, the dorsal raphe neuropeptides described in this review appear to converge on a similar functional role. Specifically, they primarily seem to bias responding, rather than generate phenotypes per se. For example, μ opioid or galanin stimulation attenuates aversive responses, whereas CRF promotes it in response to specific stimuli. Perhaps more importantly, the mechanisms through which each of these neuropeptides produces these effects are extraordinarily nuanced. They often include both pre- and post-synaptic mechanisms, as well as serotonergic and non-serotonergic targets. While some of these effects can be explained by biased activity on GABAergic cells or by the selective expression of one receptor versus another, there is much to do to within each neurochemical class to disentangle their respective mechanisms. Further complicating these analyses, some of these neuropeptides have a degree of promiscuity or cross-system interaction. In the case of opioids, individual precursor peptides can be cleaved into a myriad of products, leaving unresolved whether an “enkephalinergic” neuron is behaving as mu opioid receptor (i.e., met-enkephalin) or delta opioid receptor agonist (i.e., leu-enkephalin). Similarly, neurotensin has fairly selective receptors (NTSR1 and NTSR2), but is also known to act on sortilin, a receptor canonically shown to be involved in sorting proteins (Nykjaer and Willnow, 2012). CRF receptors can be activated by both CRF or urocortin, the latter of which has one isoform (urocortin 2) that preferentially binds to CRF2 over CRF1 (Kovács et al., 2025). Such complicated relationships between neuropeptides and their “target” receptors will likely define the future of neuropsychiatric drug development. As our molecular and signaling tools become more sophisticated, we may be able to develop positive or negative allosteric modulators that more efficiently target and bias receptor function without needing to oversaturate unnecessary subsystems. Looking forward, partnerships between medicinal chemists, behavioral neuroscientists, and molecular tool developers will be essential for continuing the advancement of neuropeptidergic research. Ultimately, these collaborations may reveal novel mechanisms or points of access for future therapeutic development and will positively shape the next several decades of neuropsychiatric research.
KB: Conceptualization, Funding acquisition, Visualization, Writing – original draft, Writing – review & editing. DC Conceptualization, Funding acquisition, Writing – original draft, Writing – review & editing.
The author(s) declare that financial support was received for the research and/or publication of this article. This work was funded by the NIH NIMH (R01 MH132504), NIDA (R00 DA049862; F32 DA062455), Diabetes Research Center (P30 DK020579), the McDonnell Center for Systems Neuroscience, and the McDonnell Center for Cellular and Molecular Neurobiology.
The authors declare that the research was conducted in the absence of any commercial or financial relationships that could be construed as a potential conflict of interest.
The authors declare that no Generative AI was used in the creation of this manuscript.
All claims expressed in this article are solely those of the authors and do not necessarily represent those of their affiliated organizations, or those of the publisher, the editors and the reviewers. Any product that may be evaluated in this article, or claim that may be made by its manufacturer, is not guaranteed or endorsed by the publisher.
Abraham, A., Casello, S., Land, B., and Chavkin, C. (2022). Optogenetic stimulation of dynorphinergic neurons within the dorsal raphe activate kappa opioid receptors in the ventral tegmental area and ablation of dorsal raphe prodynorphin or kappa receptors in dopamine neurons blocks stress potentiation of cocaine reward. Addict. Neurosci. 1:100005. doi: 10.1016/j.addicn.2022.100005
Adell, A. (2004). Antidepressant properties of substance P antagonists: Relationship to monoaminergic mechanisms? Curr. Drug Targets CNS Neurol. Disord. 3, 113–121. doi: 10.2174/1568007043482516
Alderman, S., and Bernier, N. (2007). Localization of corticotropin-releasing factor, urotensin I, and CRF-binding protein gene expression in the brain of the zebrafish. Danio rerio. J. Comp. Neurol. 502, 783–793. doi: 10.1002/cne.21332
Al-Hasani, R., McCall, J., Shin, G., Gomez, A., Schmitz, G., Bernardi, J., et al. (2015). Distinct subpopulations of nucleus accumbens dynorphin neurons drive aversion and reward. Neuron 87, 1063–1077. doi: 10.1016/j.neuron.2015.08.019
Andrade, R., and Haj-Dahmane, S. (2013). Serotonin neuron diversity in the dorsal raphe. ACS Chem. Neurosci. 4, 22–25. doi: 10.1021/cn300224n
Araneda, S., Gysling, K., and Calas, A. (1999). Raphe serotonergic neurons projecting to the olfactory bulb contain galanin or somatostatin but not neurotensin. Brain Res. Bull. 49, 209–214.
Baker, K., Halliday, G., and Törk, I. (1990). Cytoarchitecture of the human dorsal raphe nucleus. J. Comp. Neurol. 301, 147–161. doi: 10.1002/cne.903010202
Baker, K., Halliday, G., Hornung, J., Geffen, L., Cotton, R., and Törk, I. (1991). Distribution, morphology and number of monoamine-synthesizing and substance P-containing neurons in the human dorsal raphe nucleus. Neuroscience 42, 757–775. doi: 10.1016/0306-4522(91)90043-n
Bakshi, V., and Kelley, A. (1993). Feeding induced by opioid stimulation of the ventral striatum: Role of opiate receptor subtypes. J. Pharmacol. Exp. Ther. 265, 1253–1260.
Bals-Kubik, R., Ableitner, A., Herz, A., and Shippenberg, T. (1993). Neuroanatomical sites mediating the motivational effects of opioids as mapped by the conditioned place preference paradigm in rats. J. Pharmacol. Exp. Ther. 264, 489–495.
Beitz, A. (1982). The sites of origin brain stem neurotensin and serotonin projections to the rodent nucleus raphe magnus. J. Neurosci. 2, 829–842. doi: 10.1523/JNEUROSCI.02-07-00829.1982
Bhatnagar, S., Viau, V., Chu, A., Soriano, L., Meijer, O., and Dallman, M. F. A. (2000). cholecystokinin-mediated pathway to the paraventricular thalamus is recruited in chronically stressed rats and regulates hypothalamic-pituitary-adrenal function. J. Neurosci. 20, 5564–5573. doi: 10.1523/JNEUROSCI.20-14-05564.2000
Bledsoe, A., Oliver, K., Scholl, J., and Forster, G. (2011). Anxiety states induced by post-weaning social isolation are mediated by CRF receptors in the dorsal raphe nucleus. Brain Res. Bull. 85, 117–122. doi: 10.1016/j.brainresbull.2011.03.003
Blessing, W., Howe, P., Joh, T., Oliver, J., and Willoughby, J. (1986). Distribution of tyrosine hydroxylase and neuropeptide Y-like immunoreactive neurons in rabbit medulla oblongata, with attention to colocalization studies, presumptive adrenaline-synthesizing perikarya, and vagal preganglionic cells. J. Comp. Neurol. 248, 285–300. doi: 10.1002/cne.902480211
Blevins, J., Stanley, B., and Reidelberger, R. (2000). Brain regions where cholecystokinin suppresses feeding in rats. Brain Res. 860, 1–10. doi: 10.1016/s0006-8993(99)02477-4
Boden, P., Woodruff, G., and Pinnock, R. (1991). Pharmacology of a cholecystokinin receptor on 5-hydroxytryptamine neurones in the dorsal raphe of the rat brain. Br. J. Pharmacol. 102, 635–638. doi: 10.1111/j.1476-5381.1991.tb12225.x
Bons, N., Mestre, N., Petter, A., Danger, J., Pelletier, G., and Vaudry, H. (1990). Localization and characterization of neuropeptide Y in the brain of Microcebus murinus (Primate. Lemurian). J. Comp. Neurol. 298, 343–361. doi: 10.1002/cne.902980307
Boudin, H., Pélaprat, D., Rostène, W., and Beaudet, A. (1996). Cellular distribution of neurotensin receptors in rat brain: Immunohistochemical study using an antipeptide antibody against the cloned high affinity receptor. J. Comp. Neurol. 373, 76–89. doi: 10.1002/(SICI)1096-9861(19960909)373:1<76
Brandão, M. (1993). Involvement of opioid mechanisms in the dorsal periaqueductal gray in drug abuse. Rev. Neurosci. 4, 397–406. doi: 10.1515/revneuro.1993.4.4.397
Calizo, L., Akanwa, A., Ma, X., Pan, Y., Lemos, J., Craige, C., et al. (2011). Raphe serotonin neurons are not homogenous: Electrophysiological, morphological and neurochemical evidence. Neuropharmacology 61, 524–543. doi: 10.1016/j.neuropharm.2011.04.008
Castro, D., and Berridge, K. (2014). Opioid hedonic hotspot in nucleus accumbens shell: Mu, delta, and kappa maps for enhancement of sweetness "liking" and "wanting". J. Neurosci. 34, 4239–4250. doi: 10.1523/JNEUROSCI.4458-13.2014
Castro, D., and Berridge, K. (2017). Opioid and orexin hedonic hotspots in rat orbitofrontal cortex and insula. Proc. Natl. Acad. Sci. U S A. 114, E9125–E9134. doi: 10.1073/pnas.1705753114
Castro, D., Oswell, C., Zhang, E., Pedersen, C., Piantadosi, S., Rossi, M., et al. (2021). An endogenous opioid circuit determines state-dependent reward consumption. Nature 598, 646–651. doi: 10.1038/s41586-021-04013-0
Castro, D., Terry, R., and Berridge, K. (2016). Orexin in rostral hotspot of nucleus accumbens enhances sucrose ‘liking’ and intake but scopolamine in caudal shell shifts ‘liking’ toward ‘disgust’ and ‘fear’. Neuropsychopharmacology 41, 2101–2111. doi: 10.1038/npp.2016.10
Chappell, P., Smith, M., Kilts, C., Bissette, G., Ritchie, J., Anderson, C., et al. (1986). Alterations in corticotropin-releasing factor-like immunoreactivity in discrete rat brain regions after acute and chronic stress. J. Neurosci. 6, 2908–2914. doi: 10.1523/JNEUROSCI.06-10-02908.1986
Chiba, A. (1999). Immunohistochemical distribution of neuropeptide Y-related substance in the brain and hypophysis of the arctic lamprey, Lethenteron japonica. Brain Behav. Evol. 53, 102–109. doi: 10.1159/000006586
Chowdhury, S., Kamatkar, N., Wang, W., Akerele, C., Huang, J., Wu, J., et al. (2025). Brainstem neuropeptidergic neurons link a neurohumoral axis to satiation. Cell S0092-8674, 47–49. doi: 10.1016/j.cell.2025.01.018
Chudoba, R., and Dabrowska, J. (2023). Distinct populations of corticotropin-releasing factor (CRF) neurons mediate divergent yet complementary defensive behaviors in response to a threat. Neuropharmacology 228:109461. doi: 10.1016/j.neuropharm.2023.109461
Clark, M., McDevitt, R., Hoplight, B., and Neumaier, J. (2007). Chronic low dose ovine corticotropin releasing factor or urocortin II into the rostral dorsal raphe alters exploratory behavior and serotonergic gene expression in specific subregions of the dorsal raphe. Neuroscience 146, 1888–1905. doi: 10.1016/j.neuroscience.2007.03.032
Cole, R., Moussawi, K., and Joffe, M. (2024). Opioid modulation of prefrontal cortex cells and circuits. Neuropharmacology 248:109891. doi: 10.1016/j.neuropharm.2024.109891
Commons, K. (2020). Dorsal raphe organization. J. Chem. Neuroanat. 110:101868. doi: 10.1016/j.jchemneu.2020.101868
Commons, K., and Valentino, R. (2002). Cellular basis for the effects of substance P in the periaqueductal gray and dorsal raphe nucleus. J. Comp. Neurol. 447, 82–97. doi: 10.1002/cne.10228
Commons, K., Connolley, K., and Valentino, R. J. A. (2003). neurochemically distinct dorsal raphe-limbic circuit with a potential role in affective disorders. Neuropsychopharmacology 28, 206–215. doi: 10.1038/sj.npp.1300045
Conley, R., Cumberbatch, M., Mason, G., Williamson, D., Harrison, T., Locker, K., et al. (2002). Substance P (neurokinin 1) receptor antagonists enhance dorsal raphe neuronal activity. J. Neurosci. 22, 7730–7736. doi: 10.1523/JNEUROSCI.22-17-07730.2002
Cooper, M., and Huhman, K. (2007). Corticotropin-releasing factor receptors in the dorsal raphe nucleus modulate social behavior in Syrian hamsters. Psychopharmacology 194, 297–307. doi: 10.1007/s00213-007-0849-1
Cooper, P., Fernstrom, M., Rorstad, O., Leeman, S., and Martin, J. (1981). The regional distribution of somatostatin, substance P and neurotensin in human brain. Brain Res. 218, 219–232. doi: 10.1016/0006-8993(81)91302-0
Cotter, J., and Laemle, L. (1987). Distribution of somatostatinlike immunoreactivity in the brain of the little brown bat (Myotis lucifugus). Am. J. Anat. 180, 280–294. doi: 10.1002/aja.1001800309
Currie, P., and Coscina, D. (1995). Effect of the CCKA antagonist devazepide on eating stimulated by raphe injection of 8-OH-DPAT. Neuroreport 7, 253–256.
Dahlström, A., and Fuxe, K. (1964). Localization of monoamines in the lower brain stem. Experientia 20, 398–399. doi: 10.1007/BF02147990
Day, H., Greenwood, B., Hammack, S., Watkins, L., Fleshner, M., Maier, S., et al. (2004). Differential expression of 5HT-1A, alpha 1b adrenergic, CRF-R1, and CRF-R2 receptor mRNA in serotonergic, gamma-aminobutyric acidergic, and catecholaminergic cells of the rat dorsal raphe nucleus. J. Comp. Neurol. 474, 364–378. doi: 10.1002/cne.20138
de Quidt, M., and Emson, P. (1986). Distribution of neuropeptide Y-like immunoreactivity in the rat central nervous system–II. Immunohistochemical analysis. Neuroscience 18, 545–618. doi: 10.1016/0306-4522(86)90057-6
de Souza, M., Silote, G., Herbst, L., Funck, V., Joca, S., and Beijamini, V. (2018). The antidepressant-like effect of galanin in the dorsal raphe nucleus of rats involves GAL2 receptors. Neurosci. Lett. 681, 26–30. doi: 10.1016/j.neulet.2018.05.029
Del Bel, E., and Guimarães, F. (1997). Social isolation increases cholecystokinin mRNA in the central nervous system of rats. Neuroreport 8, 3597–3600. doi: 10.1097/00001756-199711100-00035
DeLaney, K., Buchberger, A., Atkinson, L., Gründer, S., Mousley, A., and Li, L. (2018). New techniques, applications and perspectives in neuropeptide research. J. Exp. Biol. 221:jeb151167. doi: 10.1242/jeb.151167
Díaz-Cabiale, Z., Parrado, C., Narváez, M., Puigcerver, A., Millón, C., Santín, L., et al. (2011). Galanin receptor/Neuropeptide Y receptor interactions in the dorsal raphe nucleus of the rat. Neuropharmacology 61, 80–86. doi: 10.1016/j.neuropharm.2011.03.002
Dong, C., Gowrishankar, R., Jin, Y., He, X., Gupta, A., Wang, H., et al. (2024). Unlocking opioid neuropeptide dynamics with genetically encoded biosensors. Nat. Neurosci. 27, 1844–1857. doi: 10.1038/s41593-024-01697-1
Dougalis, A., Matthews, G., Bishop, M., Brischoux, F., Kobayashi, K., and Ungless, M. (2012). Functional properties of dopamine neurons and co-expression of vasoactive intestinal polypeptide in the dorsal raphe nucleus and ventro-lateral periaqueductal grey. Eur. J. Neurosci. 36, 3322–3332. doi: 10.1111/j.1460-9568.2012.08255.x
Echo, J., Lamonte, N., Ackerman, T., and Bodnar, R. (2002). Alterations in food intake elicited by GABA and opioid agonists and antagonists administered into the ventral tegmental area region of rats. Physiol. Behav. 76, 107–116. doi: 10.1016/s0031-9384(02)00690-x
Eiden, L., Hernández, V., Jiang, S., and Zhang, L. (2022). Neuropeptides and small-molecule amine transmitters: Cooperative signaling in the nervous system. Cell. Mol. Life Sci. 79:492. doi: 10.1007/s00018-022-04451-7
Fardin, V., Oliveras, J., and Besson, J. M. (1984). A reinvestigation of the analgesic effects induced by stimulation of the periaqueductal gray matter in the rat. I. The production of behavioral side effects together with analgesia. Brain Res. 306, 105–123. doi: 10.1016/0006-8993(84)90360-3
Ferreira, M., and Menescal-de-Oliveira, L. (2014). Nociceptive vocalization response in guinea pigs modulated by opioidergic, GABAergic and serotonergic neurotransmission in the dorsal raphe nucleus. Brain Res. Bull. 106, 21–29. doi: 10.1016/j.brainresbull.2014.05.001
Franklin, K., and Paxinos, G. (2007). The Mouse Brain in Sterotaxic Coordinates, 3rd Edn. Cambridge, MA: Academic Press.
Fu, W., Le Maître, E., Fabre, V., Bernard, J., David Xu, Z. Q., and Hökfelt, T. (2010). Chemical neuroanatomy of the dorsal raphe nucleus and adjacent structures of the mouse brain. J. Comp. Neurol. 518, 3464–3494. doi: 10.1002/cne.22407
Gammie, S., Negron, A., Newman, S., and Rhodes, J. (2004). Corticotropin-releasing factor inhibits maternal aggression in mice. Behav. Neurosci. 118, 805–814. doi: 10.1037/0735-7044.118.4.805
García-Durán, L., Flores-Burgess, A., Cantero-García, N., Puigcerver, A., Narváez, J. Á, Fuxe, K., et al. (2021). Galanin(1-15) potentiates the antidepressant-like effects induced by escitalopram in a rat model of depression. Int. J. Mol. Sci. 22:10848. doi: 10.3390/ijms221910848
Ge, Z., Li, C., Zhang, L., Zeng, Y., Cao, J., Dai, T., et al. (2007). Involvement of local orphanin FQ in the development of analgesic tolerance induced by morphine microinjections into the dorsal raphe nucleus of rats. Neurosci. Lett. 413, 233–237. doi: 10.1016/j.neulet.2006.11.077
Gereau, G., Garrison, S., and McElligott, Z. (2023). Neurotensin and energy balance. J. Neurochem. 166, 189–200. doi: 10.1111/jnc.15868
Gundlach, A., Wisden, W., Morris, B., and Hunt, S. (1990). Localization of preprogalanin mRNA in rat brain: In situ hybridization study with a synthetic oligonucleotide probe. Neurosci. Lett. 114, 241–247. doi: 10.1016/0304-3940(90)90570-y
Gustafson, E., Smith, K., Durkin, M., Walker, M., Gerald, C., Weinshank, R., et al. (1997). Distribution of the neuropeptide Y Y2 receptor mRNA in rat central nervous system. Brain Res. Mol. Brain Res. 46, 223–235. doi: 10.1016/s0169-328x(97)00017-x
Guy, E., Choi, E., and Pratt, W. (2011). Nucleus accumbens dopamine and mu-opioid receptors modulate the reinstatement of food-seeking behavior by food-associated cues. Behav. Brain Res. 219, 265–272. doi: 10.1016/j.bbr.2011.01.024
Haddjeri, N., and Blier, P. (2001). Sustained blockade of neurokinin-1 receptors enhances serotonin neurotransmission. Biol. Psychiatry 50, 191–199. doi: 10.1016/s0006-3223(01)01162-3
Hamann, C., Bankmann, J., Mora Maza, H., Kornhuber, J., Zoicas, I., and Schmitt-Böhrer, A. (2022). Social fear affects limbic system neuronal activity and gene expression. Int. J. Mol. Sci. 23:8228. doi: 10.3390/ijms23158228
Helmchen, C., Fu, Q., and Sandkühler, J. (1995). Inhibition of spinal nociceptive neurons by microinjections of somatostatin into the nucleus raphe magnus and the midbrain periaqueductal gray of the anesthetized cat. Neurosci. Lett. 187, 137–141. doi: 10.1016/0304-3940(95)11345-2
Hökfelt, T., Ljungdahl, A., Steinbusch, H., Verhofstad, A., Nilsson, G., Brodin, E., et al. (1978). Immunohistochemical evidence of substance P-like immunoreactivity in some 5-hydroxytryptamine-containing neurons in the rat central nervous system. Neuroscience 3, 517–538. doi: 10.1016/0306-4522(78)90017-9
Hökfelt, T., Ljungdahl, A., Terenius, L., Elde, R., and Nilsson, G. (1977). Immunohistochemical analysis of peptide pathways possibly related to pain and analgesia: Enkephalin and substance P. Proc. Natl. Acad. Sci. U S A. 74, 3081–3085. doi: 10.1073/pnas.74.7.3081
Holmqvist, B., and Carlberg, M. (1992). Galanin receptors in the brain of a teleost: Autoradiographic distribution of binding sites in the Atlantic salmon. J. Comp. Neurol. 326, 44–60. doi: 10.1002/cne.903260105
Huang, K., Ochandarena, N., Philson, A., Hyun, M., Birnbaum, J., Cicconet, M., et al. (2019). Molecular and anatomical organization of the dorsal raphe nucleus. Elife 8:e46464. doi: 10.7554/eLife.46464
Hupalo, S., Bryce, C., Bangasser, D., Berridge, C., Valentino, R., and Floresco, S. (2019). Corticotropin-releasing factor (CRF) circuit modulation of cognition and motivation. Neurosci. Biobehav. Rev. 103, 50–59. doi: 10.1016/j.neubiorev.2019.06.010
Hwa, L., Debold, J., and Miczek, K. (2013). Alcohol in excess: crf1 receptors in the rat and mouse VTA and DRN. Psychopharmacology 225, 313–327. doi: 10.1007/s00213-012-2820-z
Inaba, A., Komori, Y., Muroi, Y., Kinoshita, K., and Ishii, T. (2016). Neuropeptide Y signaling in the dorsal raphe nucleus inhibits male sexual behavior in mice. Neuroscience 320, 140–148. doi: 10.1016/j.neuroscience.2016.01.069
Innis, R., Corrêa, F., Uhl, G., Schneider, B., and Snyder, S. (1979). Cholecystokinin octapeptide-like immunoreactivity: Histochemical localization in rat brain. Proc. Natl. Acad. Sci. U S A. 76, 521–525. doi: 10.1073/pnas.76.1.521
Jacobs, B., and Azmitia, E. (1992). Structure and function of the brain serotonin system. Physiol. Rev. 72, 165–229. doi: 10.1152/physrev.1992.72.1.165
Jenck, F., Quirion, R., and Wise, R. (1987). Opioid receptor subtypes associated with ventral tegmental facilitation and periaqueductal gray inhibition of feeding. Brain Res. 423, 39–44. doi: 10.1016/0006-8993(87)90822-5
Jennes, L., Stumpf, W., and Kalivas, P. (1982). Neurotensin: Topographical distribution in rat brain by immunohistochemistry. J. Comp. Neurol. 210, 211–224. doi: 10.1002/cne.902100302
Jolas, T., and Aghajanian, G. (1996). Neurotensin excitation of serotonergic neurons in the dorsal raphe nucleus of the rat in vitro. Eur. J. Neurosci. 8, 153–161. doi: 10.1111/j.1460-9568.1996.tb01176.x
Jolas, T., and Aghajanian, G. (1997). Opioids suppress spontaneous and NMDA-induced inhibitory postsynaptic currents in the dorsal raphe nucleus of the rat in vitro. Brain Res. 755, 229–245. doi: 10.1016/s0006-8993(97)00103-0
Kawa, L., Arborelius, U., Hökfelt, T., and Risling, M. (2020). Sex-specific differences in rodents following a single primary blast exposure: Focus on the monoamine and galanin systems. Front. Neurol. 11:540144. doi: 10.3389/fneur.2020.540144
Kawa, L., Barde, S., Arborelius, U., Theodorsson, E., Agoston, D., Risling, M., et al. (2016). Expression of galanin and its receptors are perturbed in a rodent model of mild, blast-induced traumatic brain injury. Exp. Neurol. 279, 159–167. doi: 10.1016/j.expneurol.2016.02.019
Keay, K., Argueta, M., Zafir, D., Wyllie, P., Michael, G., and Boorman, D. (2021). Evidence that increased cholecystokinin (CCK) in the periaqueductal gray (PAG) facilitates changes in Resident-Intruder social interactions triggered by peripheral nerve injury. J. Neurochem. 158, 1151–1171. doi: 10.1111/jnc.15476
Kehr, J., Yoshitake, T., Wang, F., Razani, H., Gimenez-Llort, L., Jansson, A., et al. (2002). Galanin is a potent in vivo modulator of mesencephalic serotonergic neurotransmission. Neuropsychopharmacology 27, 341–356. doi: 10.1016/S0893-133X(02)00309-3
Kirby, L., Freeman-Daniels, E., Lemos, J., Nunan, J., Lamy, C., Akanwa, A., et al. (2008). Corticotropin-releasing factor increases GABA synaptic activity and induces inward current in 5-hydroxytryptamine dorsal raphe neurons. J. Neurosci. 28, 12927–12937. doi: 10.1523/JNEUROSCI.2887-08.2008
Kirby, L., Rice, K., and Valentino, R. (2000). Effects of corticotropin-releasing factor on neuronal activity in the serotonergic dorsal raphe nucleus. Neuropsychopharmacology 22, 148–162. doi: 10.1016/S0893-133X(99)00093-7
Kishi, T., Aschkenasi, C., Choi, B., Lopez, M., Lee, C., Liu, H., et al. (2005). Neuropeptide Y Y1 receptor mRNA in rodent brain: Distribution and colocalization with melanocortin-4 receptor. J. Comp. Neurol. 482, 217–243. doi: 10.1002/cne.20432
Knapp, D., Overstreet, D., Huang, M., Wills, T., Whitman, B., Angel, R., et al. (2011). Effects of a stressor and corticotrophin releasing factor on ethanol deprivation-induced ethanol intake and anxiety-like behavior in alcohol-preferring P rats. Psychopharmacology 218, 179–189. doi: 10.1007/s00213-011-2366-5
Kombian, S., and Colmers, W. (1992). Neuropeptide Y selectively inhibits slow synaptic potentials in rat dorsal raphe nucleus in vitro by a presynaptic action. J. Neurosci. 12, 1086–1093. doi: 10.1523/JNEUROSCI.12-03-01086.1992
Kovács, A., Tancsics, P., Palotai, M., and Bagosi, Z. (2025). The effects of corticotropin-releasing factor (CRF) and urocortins on the serotonin (hydroxytryptamine, 5HT) released from the raphe nuclei (RN). Neuropeptides 110:102503. doi: 10.1016/j.npep.2025.102503
Kyuhou, S., and Gemba, H. (1999). Injection of orphanin FQ/nociceptin into the periaqueductal gray suppresses the forebrain-elicited vocalization in the guinea pig. Neurosci. Lett. 260, 113–116. doi: 10.1016/s0304-3940(98)00956-2
Lacoste, B., Riad, M., and Descarries, L. (2006). Immunocytochemical evidence for the existence of substance P receptor (NK1) in serotonin neurons of rat and mouse dorsal raphe nucleus. Eur. J. Neurosci. 23, 2947–2958. doi: 10.1111/j.1460-9568.2006.04833.x
Land, B., Bruchas, M., Lemos, J., Xu, M., Melief, E., and Chavkin, C. (2008). The dysphoric component of stress is encoded by activation of the dynorphin kappa-opioid system. J. Neurosci. 28, 407–414. doi: 10.1523/JNEUROSCI.4458-07.2008
Land, B., Bruchas, M., Schattauer, S., Giardino, W., Aita, M., Messinger, D., et al. (2009). Activation of the kappa opioid receptor in the dorsal raphe nucleus mediates the aversive effects of stress and reinstates drug seeking. Proc. Natl. Acad. Sci. U S A. 106, 19168–19173. doi: 10.1073/pnas.0910705106
Le Maître, E., Dourmap, N., Vilpoux, C., Leborgne, R., Janin, F., Bonnet, J., et al. (2013). Acute and subchronic treatments with selective serotonin reuptake inhibitors increase Nociceptin/Orphanin FQ (NOP) receptor density in the rat dorsal raphe nucleus; interactions between nociceptin/NOP system and serotonin. Brain Res. 1520, 51–60. doi: 10.1016/j.brainres.2013.05.005
Léger, L., Gay, N., and Cespuglio, R. (2002). Neurokinin NK1- and NK3-immunoreactive neurons in serotonergic cell groups in the rat brain. Neurosci. Lett. 323, 146–150. doi: 10.1016/s0304-3940(01)02543-5
Lein, E., Hawrylycz, M., Ao, N., Ayres, M., Bensinger, A., Bernard, A., et al. (2007). Genome-wide atlas of gene expression in the adult mouse brain. Nature 445, 168–176. doi: 10.1038/nature05453
Li, C., McCloskey, N., Phillips, J., Simmons, S., and Kirby, L. G. (2021). CRF-5-HT interactions in the dorsal raphe nucleus and motivation for stress-induced opioid reinstatement. Psychopharmacology 238, 29–40. doi: 10.1007/s00213-020-05652-3
Li, C., McElroy, B., Phillips, J., McCloskey, N., Shi, X., Unterwald, E., et al. (2024). Role of α1-GABAA receptors in the serotonergic dorsal raphe nucleus in models of opioid reward, anxiety, and depression. J. Psychopharmacol. 38, 188–199. doi: 10.1177/02698811241227672
Li, C., Sugam, J., Lowery-Gionta, E., McElligott, Z., McCall, N., Lopez, A., et al. (2016). Mu opioid receptor modulation of dopamine neurons in the periaqueductal Gray/dorsal raphe: A role in regulation of pain. Neuropsychopharmacology 41, 2122–2132. doi: 10.1038/npp.2016.12
Li, Y., Li, H., Kaneko, T., and Mizuno, N. (2001). Morphological features and electrophysiological properties of serotonergic and non-serotonergic projection neurons in the dorsal raphe nucleus. An intracellular recording and labeling study in rat brain slices. Brain Res. 900, 110–118. doi: 10.1016/s0006-8993(01)02272-7
Lichtenberg, N., and Wassum, K. (2017). Amygdala mu-opioid receptors mediate the motivating influence of cue-triggered reward expectations. Eur. J. Neurosci. 45, 381–387. doi: 10.1111/ejn.13477
Lim, M., Nair, H., and Young, L. (2005). Species and sex differences in brain distribution of corticotropin-releasing factor receptor subtypes 1 and 2 in monogamous and promiscuous vole species. J. Comp. Neurol. 487, 75–92. doi: 10.1002/cne.20532
Liu, R., Ding, Y., and Aghajanian, G. (2002). Neurokinins activate local glutamatergic inputs to serotonergic neurons of the dorsal raphe nucleus. Neuropsychopharmacology 27, 329–340. doi: 10.1016/S0893-133X(02)00305-6
Liu, X., He, J., Jiang, W., Wen, S., and Xiao, Z. (2023). The roles of periaqueductal gray and dorsal raphe nucleus dopaminergic systems in the mechanisms of thermal hypersensitivity and depression in mice. J. Pain 24, 1213–1228. doi: 10.1016/j.jpain.2023.02.004
Lovell, P., and Mello, C. (2011). Brain expression and song regulation of the cholecystokinin gene in the zebra finch (Taeniopygia guttata). J. Comp. Neurol. 519, 211–237. doi: 10.1002/cne.22513
Lowry, C., Rodda, J., Lightman, S., and Ingram, C. (2000). Corticotropin-releasing factor increases in vitro firing rates of serotonergic neurons in the rat dorsal raphe nucleus: Evidence for activation of a topographically organized mesolimbocortical serotonergic system. J. Neurosci. 20, 7728–7736. doi: 10.1523/JNEUROSCI.20-20-07728.2000
Lu, X., Barr, A., Kinney, J., Sanna, P., Conti, B., Behrens, M., et al. (2005a). A role for galanin in antidepressant actions with a focus on the dorsal raphe nucleus. Proc. Natl. Acad. Sci. U S A. 102, 874–879. doi: 10.1073/pnas.0408891102
Lu, X., Mazarati, A., Sanna, P., Shinmei, S., and Bartfai, T. (2005b). Distribution and differential regulation of galanin receptor subtypes in rat brain: Effects of seizure activity. Neuropeptides 39, 147–152. doi: 10.1016/j.npep.2004.12.011
Lu, X., Ross, B., Sanchez-Alavez, M., Zorrilla, E., and Bartfai, T. (2008). Phenotypic analysis of GalR2 knockout mice in anxiety- and depression-related behavioral tests. Neuropeptides 42, 387–397. doi: 10.1016/j.npep.2008.04.009
Lukkes, J., Summers, C., Scholl, J., Renner, K., and Forster, G. (2009a). Early life social isolation alters corticotropin-releasing factor responses in adult rats. Neuroscience 158, 845–855. doi: 10.1016/j.neuroscience.2008.10.036
Lukkes, J., Vuong, S., Scholl, J., Oliver, H., and Forster, G. (2009b). Corticotropin-releasing factor receptor antagonism within the dorsal raphe nucleus reduces social anxiety-like behavior after early-life social isolation. J. Neurosci. 29, 9955–9960. doi: 10.1523/JNEUROSCI.0854-09.2009
Lunden, J., and Kirby, L. (2013). Opiate exposure and withdrawal dynamically regulate mRNA expression in the serotonergic dorsal raphe nucleus. Neuroscience 254, 160–172. doi: 10.1016/j.neuroscience.2013.08.071
Luo, M., Li, Y., and Zhong, W. (2016). Do dorsal raphe 5-HT neurons encode "beneficialness"? Neurobiol. Learn. Mem. 135, 40–49. doi: 10.1016/j.nlm.2016.08.008
Luo, M., Zhou, J., and Liu, Z. (2015). Reward processing by the dorsal raphe nucleus: 5-ht and beyond. Learn. Mem. 22, 452–460. doi: 10.1101/lm.037317.114
Maeno, H., Kiyama, H., and Tohyama, M. (1993). Distribution of the substance P receptor (NK-1 receptor) in the central nervous system. Mol. Brain Res. 18, 43–58. doi: 10.1016/0169-328x(93)90172-l
Magoul, R., Oblin, A., Calas, A., and Araneda, S. (1988). Serotonergic projections to the spinal cord but not those to the olfactory bulb also contain substance P. A combined immunocytochemical and autoradiographic study following retrograde axonal transport of [3H]serotonin labeled products. Neuroscience 26, 959–969. doi: 10.1016/0306-4522(88)90112-1
Mancera, J., López Avalos, M., Pérez-Fígares, J., and Fernández-Llebrez, P. (1991). The distribution of corticotropin-releasing factor–immunoreactive neurons and nerve fibers in the brain of the snake, Natrix maura. Coexistence with arginine vasotocin and mesotocin. Cell. Tissue Res. 264, 539–548. doi: 10.1007/BF00319043
Mansour, A., Fox, C., Burke, S., Meng, F., Thompson, R., Akil, H., et al. (1994a). Mu, delta, and kappa opioid receptor mRNA expression in the rat CNS: An in situ hybridization study. J. Comp. Neurol. 350, 412–438. doi: 10.1002/cne.903500307
Mansour, A., Fox, C., Thompson, R., Akil, H., and Watson, S. (1994b). mu-Opioid receptor mRNA expression in the rat CNS: Comparison to mu-receptor binding. Brain Res. 643, 245–265. doi: 10.1016/0006-8993(94)90031-0
Marcinkiewcz, C., Mazzone, C., D’Agostino, G., Halladay, L., Hardaway, J., DiBerto, J., et al. (2016). Serotonin engages an anxiety and fear-promoting circuit in the extended amygdala. Nature 537, 97–101. doi: 10.1038/nature19318
Margolis, E., Hjelmstad, G., Fujita, W., and Fields, H. (2014). Direct bidirectional μ-opioid control of midbrain dopamine neurons. J. Neurosci. 34, 14707–14716. doi: 10.1523/JNEUROSCI.2144-14.2014
Massaly, N., Copits, B., Wilson-Poe, A., Hipólito, L., Markovic, T., Yoon, H., et al. (2019). Pain-induced negative affect is mediated via recruitment of the nucleus accumbens kappa opioid system. Neuron 102, 564–573.e6. doi: 10.1016/j.neuron.2019.02.029.
McClintick, J., McBride, W., Bell, R., Ding, Z., Liu, Y., Xuei, X., et al. (2015). Gene expression changes in serotonin, GABA-A receptors, neuropeptides and ion channels in the dorsal raphe nucleus of adolescent alcohol-preferring (P) rats following binge-like alcohol drinking. Pharmacol. Biochem. Behav. 129, 87–96. doi: 10.1016/j.pbb.2014.12.007
McDevitt, R., and Neumaier, J. (2011). Regulation of dorsal raphe nucleus function by serotonin autoreceptors: A behavioral perspective. J. Chem. Neuroanat. 41, 234–246. doi: 10.1016/j.jchemneu.2011.05.001
Medel-Matus, J., Shin, D., Sankar, R., and Mazarati, A. (2017). Galanin contributes to monoaminergic dysfunction and to dependent neurobehavioral comorbidities of epilepsy. Exp. Neurol. 289, 64–72. doi: 10.1016/j.expneurol.2016.12.008
Melander, T., Hökfelt, T., and Rökaeus, A. (1986). Distribution of galaninlike immunoreactivity in the rat central nervous system. J. Comp. Neurol. 248, 475–517. doi: 10.1002/cne.902480404
Mena, J., Selleck, R., and Baldo, B. (2013). Mu-opioid stimulation in rat prefrontal cortex engages hypothalamic orexin/hypocretin-containing neurons, and reveals dissociable roles of nucleus accumbens and hypothalamus in cortically driven feeding. J. Neurosci. 33, 18540–18552. doi: 10.1523/JNEUROSCI.3323-12.2013
Mennicken, F., Hoffert, C., Pelletier, M., Ahmad, S., and O’Donnell, D. (2002). Restricted distribution of galanin receptor 3 (GalR3) mRNA in the adult rat central nervous system. J. Chem. Neuroanat. 24, 257–268. doi: 10.1016/s0891-0618(02)00068-6
Merchenthaler, I. (1984). Corticotropin releasing factor (CRF)-like immunoreactivity in the rat central nervous system. Extrahypothalamic distribution. Peptides 5, 53–69. doi: 10.1016/0196-9781(84)90265-1
Michelsen, K., Schmitz, C., and Steinbusch, H. (2007). The dorsal raphe nucleus–from silver stainings to a role in depression. Brain Res. Rev. 55, 329–342. doi: 10.1016/j.brainresrev.2007.01.002
Monassi, C., Leite-Panissi, C., and Menescal-de-Oliveira, L. (1999). Ventrolateral periaqueductal gray matter and the control of tonic immobility. Brain Res. Bull. 50, 201–208. doi: 10.1016/s0361-9230(99)00192-6
Monti, J. (2010). The structure of the dorsal raphe nucleus and its relevance to the regulation of sleep and wakefulness. Sleep Med. Rev. 14, 307–317. doi: 10.1016/j.smrv.2009.11.004
Morais, J., Souza, M., Campanha, T., Muller, C., Bittencourt, A., Bortoli, V., et al. (2016). Galanin subtype 1 and subtype 2 receptors mediate opposite anxiety-like effects in the rat dorsal raphe nucleus. Behav. Brain Res. 314, 125–133. doi: 10.1016/j.bbr.2016.08.007
Moyse, E., Rostène, W., Vial, M., Leonard, K., Mazella, J., Kitabgi, P., et al. (1987). Distribution of neurotensin binding sites in rat brain: A light microscopic radioautographic study using monoiodo [125I]Tyr3-neurotensin. Neuroscience 22, 525–536. doi: 10.1016/0306-4522(87)90350-2
Muroi, Y., and Ishii, T. (2015). Neuropeptide Y is crucial for nutritional state-dependent regulation of maternal behavior. Psychoneuroendocrinology 51, 392–402. doi: 10.1016/j.psyneuen.2014.09.022
Nazzaro, C., Marino, S., Barbieri, M., and Siniscalchi, A. (2009). Inhibition of serotonin outflow by nociceptin/orphaninFQ in dorsal raphe nucleus slices from normal and stressed rats: Role of corticotropin releasing factor. Neurochem. Int. 54, 378–384. doi: 10.1016/j.neuint.2009.01.004
Neal, C., Mansour, A., Reinscheid, R., Nothacker, H., Civelli, O., Akil, H., et al. (1999). Opioid receptor-like (ORL1) receptor distribution in the rat central nervous system: Comparison of ORL1 receptor mRNA expression with (125)I-[(14)Tyr]-orphanin FQ binding. J. Comp. Neurol. 412, 563–605.
Nectow, A., Schneeberger, M., Zhang, H., Field, B., Renier, N., Azevedo, E., et al. (2017). Identification of a brainstem circuit controlling feeding. Cell 170, 429-442.e11. doi: 10.1016/j.cell.2017.06.045.
Nusbaum, M., Blitz, D., and Marder, E. (2017). Functional consequences of neuropeptide and small-molecule co-transmission. Nat. Rev. Neurosci. 18, 389–403. doi: 10.1038/nrn.2017.56
Nygard, S., Hourguettes, N., Sobczak, G., Carlezon, W., and Bruchas, M. (2016). Stress-induced reinstatement of nicotine preference requires dynorphin/kappa opioid activity in the basolateral amygdala. J. Neurosci. 36, 9937–9948. doi: 10.1523/jneurosci.0953-16.2016
Nykjaer, A., and Willnow, T. (2012). Sortilin: A receptor to regulate neuronal viability and function. Trends Neurosci. 35, 261–270. doi: 10.1016/j.tins.2012.01.003
Okaty, B., Sturrock, N., Escobedo Lozoya, Y., Chang, Y., Senft, R., Lyon, K., et al. (2020). A single-cell transcriptomic and anatomic atlas of mouse dorsal raphe Pet1 neurons. Elife 9:e55523. doi: 10.7554/eLife.55523
Okere, C., and Waterhouse, B. (2006). Activity-dependent heterogeneous populations of nitric oxide synthase neurons in the rat dorsal raphe nucleus. Brain Res. 1086, 117–132. doi: 10.1016/j.brainres.2006.02.107
Otake, K. (2005). Cholecystokinin and substance P immunoreactive projections to the paraventricular thalamic nucleus in the rat. Neurosci. Res. 51, 383–394. doi: 10.1016/j.neures.2004.12.009
Palkovits, M., Brownstein, M., and Vale, W. (1985). Distribution of corticotropin-releasing factor in rat brain. Fed Proc. 44, 215–219.
Parker, K., Pedersen, C., Gomez, A., Spangler, S., Walicki, M., Feng, S., et al. (2019). A paranigral VTA nociceptin circuit that constrains motivation for reward. Cell 178, 653–671.e19. doi: 10.1016/j.cell.2019.06.034.
Parker, R., and Herzog, H. (1999). Regional distribution of Y-receptor subtype mRNAs in rat brain. Eur. J. Neurosci. 11, 1431–1448. doi: 10.1046/j.1460-9568.1999.00553.x
Peciña, S., and Berridge, K. (2005). Hedonic hot spot in nucleus accumbens shell: Where do mu-opioids cause increased hedonic impact of sweetness? J. Neurosci. 25, 11777–11786. doi: 10.1523/JNEUROSCI.2329-05.2005
Pernar, L., Curtis, A., Vale, W., Rivier, J., and Valentino, R. (2004). Selective activation of corticotropin-releasing factor-2 receptors on neurochemically identified neurons in the rat dorsal raphe nucleus reveals dual actions. J. Neurosci. 24, 1305–1311. doi: 10.1523/JNEUROSCI.2885-03.2004
Perroteau, I., Danger, J., Biffo, S., Pelletier, G., Vaudry, H., and Fasolo, A. (1988). Distribution and characterization of neuropeptide Y-like immunoreactivity in the brain of the crested newt. J. Comp. Neurol. 275, 309–325. doi: 10.1002/cne.902750302
Pomrenze, M., Cardozo Pinto, D., Neumann, P., Llorach, P., Tucciarone, J., Morishita, W., et al. (2022). Modulation of 5-HT release by dynorphin mediates social deficits during opioid withdrawal. Neuron 110, 4125–4143.e6. doi: 10.1016/j.neuron.2022.09.024.
Price, M., Curtis, A., Kirby, L., Valentino, R., and Lucki, I. (1998). Effects of corticotropin-releasing factor on brain serotonergic activity. Neuropsychopharmacology 18, 492–502. doi: 10.1016/S0893-133X(97)00197-8
Przydzial, M., Garfield, A., Lam, D., Moore, S., Evans, M., and Heisler, L. (2010). Nutritional state influences Nociceptin/Orphanin FQ peptide receptor expression in the dorsal raphe nucleus. Behav. Brain Res. 206, 313–317. doi: 10.1016/j.bbr.2009.09.017
Quadros, I., Hwa, L., Shimamoto, A., Carlson, J., DeBold, J., and Miczek, K. (2014). Prevention of alcohol-heightened aggression by CRF-R1 antagonists in mice: Critical role for DRN-PFC serotonin pathway. Neuropsychopharmacology 39, 2874–2883. doi: 10.1038/npp.2014.139
Ragnauth, A., Moroz, M., and Bodnar, R. (2000). Multiple opioid receptors mediate feeding elicited by mu and delta opioid receptor subtype agonists in the nucleus accumbens shell in rats. Brain Res. 876, 76–87. doi: 10.1016/s0006-8993(00)02631-7
Rapport, M., Green, A., and Page, I. (1948a). Crystalline serotonin. Science 108, 329–330. doi: 10.1126/science.108.2804.329
Rapport, M., Green, A., and Page, I. (1948b). Partial purification of the vasoconstrictor in beef serum. J. Biol. Chem. 174, 735–741.
Rapport, M., Green, A., and Page, I. (1948c). Serum vasoconstrictor, serotonin; isolation and characterization. J. Biol. Chem. 176, 1243–1251.
Razani, H., Diaz-Cabiale, Z., Fuxe, K., and Ogren, S. (2000). Intraventricular galanin produces a time-dependent modulation of 5-HT1A receptors in the dorsal raphe of the rat. Neuroreport 11, 3943–3948. doi: 10.1097/00001756-200012180-00008
Reinbold Scholl, J., Oliver, K., Watt, M., and Forster, G. (2014). Central CRF2 receptor antagonism reduces anxiety states during amphetamine withdrawal. Neurosci. Res. 89, 37–43. doi: 10.1016/j.neures.2014.08.010
Ren, J., Friedmann, D., Xiong, J., Liu, C., Ferguson, B., Weerakkody, T., et al. (2018). Anatomically defined and functionally distinct dorsal raphe serotonin sub-systems. Cell 175, 472–487.e20. doi: 10.1016/j.cell.2018.07.043.
Ren, J., Isakova, A., Friedmann, D., Zeng, J., Grutzner, S., Pun, A., et al. (2019). Single-cell transcriptomes and whole-brain projections of serotonin neurons in the mouse dorsal and median raphe nuclei. Elife 8:e49424. doi: 10.7554/eLife.49424
Ritchie, J., Qi, S., Soto, D., Swatzell, S., Grenz, H., Pruitt, A., et al. (2024). Dorsal raphe to basolateral amygdala corticotropin-releasing factor circuit regulates cocaine-memory reconsolidation. Neuropsychopharmacology 49, 2077–2086. doi: 10.1038/s41386-024-01892-5
Roche, M., Commons, K., Peoples, A., and Valentino, R. (2003). Circuitry underlying regulation of the serotonergic system by swim stress. J. Neurosci. 23, 970–977. doi: 10.1523/JNEUROSCI.23-03-00970.2003
Russo, A. (2017). Overview of neuropeptides: Awakening the Senses? Headache 57, 37–46. doi: 10.1111/head.13084
Salvatore, M., Wiersielis, K., Luz, S., Waxler, D., Bhatnagar, S., and Bangasser, D. (2018). Sex differences in circuits activated by corticotropin releasing factor in rats. Horm. Behav. 97, 145–153. doi: 10.1016/j.yhbeh.2017.10.004
Santarelli, L., Gobbi, G., Blier, P., and Hen, R. (2002). Behavioral and physiologic effects of genetic or pharmacologic inactivation of the substance P receptor (NK1). J. Clin. Psychiatry 63, 11–17.
Santarelli, L., Gobbi, G., Debs, P., Sibille, E., Blier, P., Hen, R., et al. (2001). Genetic and pharmacological disruption of neurokinin 1 receptor function decreases anxiety-related behaviors and increases serotonergic function. Proc. Natl. Acad. Sci. U S A. 98, 1912–1917. doi: 10.1073/pnas.98.4.1912
Sante, A., Nobre, M., and Brandão, M. (2000). Place aversion induced by blockade of mu or activation of kappa opioid receptors in the dorsal periaqueductal gray matter. Behav. Pharmacol. 11, 583–589. doi: 10.1097/00008877-200011000-00005
Sarret, P., Perron, A., Stroh, T., and Beaudet, A. (2003). Immunohistochemical distribution of NTS2 neurotensin receptors in the rat central nervous system. J. Comp. Neurol. 461, 520–538. doi: 10.1002/cne.10718
Schank, J., Nelson, B., Damadzic, R., Tapocik, J., Yao, M., King, C., et al. (2015). Neurokinin-1 receptor antagonism attenuates neuronal activity triggered by stress-induced reinstatement of alcohol seeking. Neuropharmacology 99, 106–114. doi: 10.1016/j.neuropharm.2015.07.009
Scheich, B., Gaszner, B., Kormos, V., László, K., Ádori, C., Borbély, É, et al. (2016). Somatostatin receptor subtype 4 activation is involved in anxiety and depression-like behavior in mouse models. Neuropharmacology 101, 204–215. doi: 10.1016/j.neuropharm.2015.09.021
Schweimer, J., Mallet, N., Sharp, T., and Ungless, M. (2011). Spike-timing relationship of neurochemically-identified dorsal raphe neurons during cortical slow oscillations. Neuroscience 196, 115–123. doi: 10.1016/j.neuroscience.2011.08.072
Seroogy, K., Ceccatelli, S., Schalling, M., Hökfelt, T., Frey, P., Walsh, J., et al. (1988). A subpopulation of dopaminergic neurons in rat ventral mesencephalon contains both neurotensin and cholecystokinin. Brain Res. 455, 88–98. doi: 10.1016/0006-8993(88)90117-5
Sharkey, L., Madamba, S., Siggins, G., and Bartfai, T. (2008). Galanin alters GABAergic neurotransmission in the dorsal raphe nucleus. Neurochem. Res. 33, 285–291. doi: 10.1007/s11064-007-9524-5
Shields, B., Kahuno, E., Kim, C., Apostolides, P., Brown, J., Lindo, S., et al. (2017). Deconstructing behavioral neuropharmacology with cellular specificity. Science 356:eaaj2161. doi: 10.1126/science.aaj2161
Shields, B., Yan, H., Lim, S., Burwell, S., Cammarata, C., Fleming, E., et al. (2024). DART.2: Bidirectional synaptic pharmacology with thousandfold cellular specificity. Nat. Methods 21, 1288–1297. doi: 10.1038/s41592-024-02292-9
Shu, Y., Ni, R., Sun, Y., Fang, H., and Zhou, J. (2015). Distribution of corticotropin-releasing factor in the tree shrew brain. Brain Res. 1618, 270–285. doi: 10.1016/j.brainres.2015.06.004
Shugalev, I., Ol’shanskiĭ, A., Lenard, L., and Hartmann, G. (2005). Effects of neurotensin on active and passive avoidance performance in rats with lesions of serotoninergic neurons. Zh Vyssh Nerv Deiat Im I P Pavlova 55, 247–252.
Shugalev, N., Stavrovskaia, A., Ol’shnski, A., Hartmann, G., and Lenard, L. (2007). [Involvement of serotoninergic brain structures in the mechanisms of neurotensin effect on passive avoidance in rats]. Zh Vyssh Nerv Deiat Im I P Pavlova. 57, 352–357.
Siegel, A., and Pott, C. (1988). Neural substrates of aggression and flight in the cat. Prog. Neurobiol. 31, 261–283. doi: 10.1016/0301-0082(88)90015-9
Silote, G., Rosal, A., de Souza, M., and Beijamini, V. (2013). Infusion of galanin into the mid-caudal portion of the dorsal raphe nucleus has an anxiolytic effect on rats in the elevated T-maze. Behav. Brain Res. 252, 312–317. doi: 10.1016/j.bbr.2013.06.023
Sim, L., and Joseph, S. (1991). Arcuate nucleus projections to brainstem regions which modulate nociception. J. Chem. Neuroanat. 4, 97–109. doi: 10.1016/0891-0618(91)90034-a
Siuda, E., Copits, B., Schmidt, M., Baird, M., Al-Hasani, R., Planer, W., et al. (2015). Spatiotemporal control of opioid signaling and behavior. Neuron 86, 923–935. doi: 10.1016/j.neuron.2015.03.066
Skofitsch, G., and Jacobowitz, D. (1985). Immunohistochemical mapping of galanin-like neurons in the rat central nervous system. Peptides 6, 509–546. doi: 10.1016/0196-9781(85)90118-4
Smith, G., Savery, D., Marden, C., López Costa, J., Averill, S., Priestley, J., et al. (1994). Distribution of messenger RNAs encoding enkephalin, substance P, somatostatin, galanin, vasoactive intestinal polypeptide, neuropeptide Y, and calcitonin gene-related peptide in the midbrain periaqueductal grey in the rat. J. Comp. Neurol. 350, 23–40. doi: 10.1002/cne.903500103
Smith, K., and Berridge, K. (2005). The ventral pallidum and hedonic reward: Neurochemical maps of sucrose "liking" and food intake. J. Neurosci. 25, 8637–8649. doi: 10.1523/JNEUROSCI.1902-05.2005
Smith, K., and Berridge, K. (2007). Opioid limbic circuit for reward: Interaction between hedonic hotspots of nucleus accumbens and ventral pallidum. J. Neurosci. 27, 1594–1605. doi: 10.1523/JNEUROSCI.4205-06.2007
Smith, K., Tindell, A., Aldridge, J., and Berridge, K. (2009). Ventral pallidum roles in reward and motivation. Behav. Brain Res. 196, 155–167. doi: 10.1016/j.bbr.2008.09.038
Spangler, K., and Morley, B. (1987). Somatostatin-like immunoreactivity in the midbrain of the cat. J. Comp. Neurol. 260, 87–97. doi: 10.1002/cne.902600108
Staub, D., Lunden, J., Cathel, A., Dolben, E., and Kirby, L. (2012). Morphine history sensitizes postsynaptic GABA receptors on dorsal raphe serotonin neurons in a stress-induced relapse model in rats. Psychoneuroendocrinology 37, 859–870. doi: 10.1016/j.psyneuen.2011.10.002
Steinbusch, H., Dolatkhah, M., and Hopkins, D. (2021). Anatomical and neurochemical organization of the serotonergic system in the mammalian brain and in particular the involvement of the dorsal raphe nucleus in relation to neurological diseases. Prog. Brain Res. 261, 41–81. doi: 10.1016/bs.pbr.2021.02.003
Summers, S., Grau, L., Massel, D., Rosas, S., Ong, A., and Hernandez, V. (2018). Opioid use disorders are associated with perioperative morbidity and mortality in the hip fracture population. J. Orthop. Trauma 32, 238–244. doi: 10.1097/BOT.0000000000001118
Sutin, E., and Jacobowitz, D. (1988). Immunocytochemical localization of peptides and other neurochemicals in the rat laterodorsal tegmental nucleus and adjacent area. J. Comp. Neurol. 270, 243–270. doi: 10.1002/cne.902700206
Svensson, E., Apergis-Schoute, J., Burnstock, G., Nusbaum, M., Parker, D., and Schiöth, H. (2019). General principles of neuronal co-transmission: Insights from multiple model systems. Front. Neural Circuits 12:117. doi: 10.3389/fncir.2018.00117
Swanson, C., Blackburn, T., Zhang, X., Zheng, K., Xu, Z., Hökfelt, T., et al. (2005). Anxiolytic- and antidepressant-like profiles of the galanin-3 receptor (Gal3) antagonists SNAP 37889 and SNAP 398299. Proc. Natl. Acad. Sci. U S A. 102, 17489–17494. doi: 10.1073/pnas.0508970102
Taber, E., Brodal, A., and Walberg, F. (1960). The raphe nuclei of the brain stem in the cat. I. Normal topography and cytoarchitecture and general discussion. J. Comp. Neurol. 114, 161–187. doi: 10.1002/cne.901140205
Taber-Pierce, E., Lichentenstein, E., and Feldman, S. (1985). The somatostatin systems of the guinea-pig brainstem. Neuroscience 15, 215–235. doi: 10.1016/0306-4522(85)90133-2
Tanaka, S., and Tsujimoto, A. (1981). Somatostatin facilitates the serotonin release from rat cerebral cortex, hippocampus and hypothalamus slices. Brain Res. 208, 219–222. doi: 10.1016/0006-8993(81)90636-3
Tao, R., and Auerbach, S. (2005). mu-Opioids disinhibit and kappa-opioids inhibit serotonin efflux in the dorsal raphe nucleus. Brain Res. 1049, 70–79. doi: 10.1016/j.brainres.2005.04.076
Tao, R., Ma, Z., Thakkar, M., McCarley, R., and Auerbach, S. (2007). Nociceptin/orphanin FQ decreases serotonin efflux in the rat brain but in contrast to a kappa-opioid has no antagonistic effect on mu-opioid-induced increases in serotonin efflux. Neuroscience 147, 106–116. doi: 10.1016/j.neuroscience.2007.02.011
Thomas, C., Mohammadkhani, A., Rana, M., Qiao, M., Baimel, C., and Borgland, S. (2022). Optogenetic stimulation of lateral hypothalamic orexin/dynorphin inputs in the ventral tegmental area potentiates mesolimbic dopamine neurotransmission and promotes reward-seeking behaviours. Neuropsychopharmacology 47, 728–740. doi: 10.1038/s41386-021-01196-y
Thomas, E., Pernar, L., Lucki, I., and Valentino, R. (2003). Corticotropin-releasing factor in the dorsal raphe nucleus regulates activity of lateral septal neurons. Brain Res. 960, 201–208. doi: 10.1016/s0006-8993(02)03882-9
Thompson, R., and Swanson, L. (2010). Hypothesis-driven structural connectivity analysis supports network over hierarchical model of brain architecture. Proc. Natl. Acad. Sci. U S A. 107, 15235–15239. doi: 10.1073/pnas.1009112107
Toddes, C., Lefevre, E., Brandner, D., Zugschwert, L., and Rothwell, P. (2021). μ-Opioid receptor (Oprm1) copy number influences nucleus accumbens microcircuitry and reciprocal social behaviors. J. Neurosci. 41, 7965–7977. doi: 10.1523/JNEUROSCI.2440-20.2021
Twarog, B., and Page, I. (1953). Serotonin content of some mammalian tissues and urine and a method for its determination. Am. J. Physiol. 175, 157–161. doi: 10.1152/ajplegacy.1953.175.1.157
Uhl, G., Goodman, R., and Snyder, S. (1979b). Neurotensin-containing cell bodies, fibers and nerve terminals in the brain stem of the rat: Immunohistochemical mapping. Brain Res. 167, 77–91. doi: 10.1016/0006-8993(79)90264-6
Uhl, G., Goodman, R., Kuhar, M., Childers, S., and Snyder, S. (1979a). Immunohistochemical mapping of enkephalin containing cell bodies, fibers and nerve terminals in the brain stem of the rat. Brain Res. 166, 75–94. doi: 10.1016/0006-8993(79)90651-6
Upadhya, M., Dandekar, M., Kokare, D., Singru, P., and Subhedar, N. (2009). Involvement of neuropeptide Y in the acute, chronic and withdrawal responses of morphine in nociception in neuropathic rats: Behavioral and neuroanatomical correlates. Neuropeptides 43, 303–314. doi: 10.1016/j.npep.2009.05.003
Valentino, R., Bey, V., Pernar, L., and Commons, K. (2003). Substance P Acts through local circuits within the rat dorsal raphe nucleus to alter serotonergic neuronal activity. J. Neurosci. 23, 7155–7159. doi: 10.1523/JNEUROSCI.23-18-07155.2003
Valentino, R., Liouterman, L., and Van Bockstaele, E. (2001). Evidence for regional heterogeneity in corticotropin-releasing factor interactions in the dorsal raphe nucleus. J. Comp. Neurol. 435, 450–463. doi: 10.1002/cne.1043
Valentino, R., Lucki, I., and Van Bockstaele, E. (2010). Corticotropin-releasing factor in the dorsal raphe nucleus: Linking stress coping and addiction. Brain Res. 1314, 29–37. doi: 10.1016/j.brainres.2009.09.100
van der Kooy, D., Hunt, S., Steinbusch, H., and Verhofstad, A. (1981). Separate populations of cholecystokinin and 5-hydroxytryptamine-containing neuronal cells in the rat dorsal raphe, and their contribution to the ascending raphe projections. Neurosci. Lett. 26, 25–30. doi: 10.1016/0304-3940(81)90420-1
Verheij, M., Contet, C., Karel, P., Latour, J., van der Doelen, R., Geenen, B., et al. (2018). Median and dorsal raphe serotonergic neurons control moderate versus compulsive cocaine intake. Biol. Psychiatry 83, 1024–1035. doi: 10.1016/j.biopsych.2017.10.031
Vertes, R. P. (1991). A PHA-L analysis of ascending projections of the dorsal raphe nucleus in the rat. J. Comp. Neurol. 313, 643–668. doi: 10.1002/cne.903130409
Wang, H., Qian, T., Zhao, Y., Zhuo, Y., Wu, C., Osakada, T., et al. (2023). A tool kit of highly selective and sensitive genetically encoded neuropeptide sensors. Science 382:eabq8173. doi: 10.1126/science.abq8173
Wang, P., Li, H., Barde, S., Zhang, M., Sun, J., Wang, T., et al. (2016). Depression-like behavior in rat: Involvement of galanin receptor subtype 1 in the ventral periaqueductal gray. Proc. Natl. Acad. Sci. U S A. 113, E4726–E4735. doi: 10.1073/pnas.1609198113
Waselus, M., Nazzaro, C., Valentino, R., and Van Bockstaele, E. (2009). Stress-induced redistribution of corticotropin-releasing factor receptor subtypes in the dorsal raphe nucleus. Biol. Psychiatry 66, 76–83. doi: 10.1016/j.biopsych.2009.02.014
Waselus, M., Valentino, R., and Van Bockstaele, E. (2005). Ultrastructural evidence for a role of gamma-aminobutyric acid in mediating the effects of corticotropin-releasing factor on the rat dorsal raphe serotonin system. J. Comp. Neurol. 482, 155–165. doi: 10.1002/cne.20360
Weindl, A., Triepel, J., and Kuchling, G. (1984). Somatostatin in the brain of the turtle Testudo hermanni Gmelin. An immunohistochemical mapping study. Peptides 5, 91–100. doi: 10.1016/0196-9781(84)90268-7
Welsch, L., Colantonio, E., Falconnier, C., Champagnol-DiLiberti, C., Allain, F., Ben Hamida, S., et al. (2023a). Mu opioid receptor-positive neurons in the dorsal raphe nucleus are impaired by morphine abstinence. Biol. Psychiatry 94, 852–862. doi: 10.1016/j.biopsych.2023.06.024
Welsch, L., Colantonio, E., Frison, M., Johnson, D., McClain, S., Mathis, V., et al. (2023b). Mu opioid receptor-expressing neurons in the dorsal raphe nucleus are involved in reward processing and affective behaviors. Biol. Psychiatry 94, 842–851. doi: 10.1016/j.biopsych.2023.05.019
Wiklund, L., Léger, L., and Persson, M. (1981). Monoamine cell distribution in the cat brain stem. A fluorescence histochemical study with quantification of indolaminergic and locus coeruleus cell groups. J. Comp. Neurol. 203, 613–647. doi: 10.1002/cne.902030405
Wood, S., Zhang, X., Reyes, B., Lee, C., Van Bockstaele, E., and Valentino, R. (2013). Cellular adaptations of dorsal raphe serotonin neurons associated with the development of active coping in response to social stress. Biol. Psychiatry 73, 1087–1094. doi: 10.1016/j.biopsych.2013.01.026
Wulff, A., Tooley, J., Marconi, L., and Creed, M. (2019). Ventral pallidal modulation of aversion processing. Brain Res. 1713, 62–69. doi: 10.1016/j.brainres.2018.10.010
Xie, L., Wu, H., Chen, Q., Xu, F., Li, H., Xu, Q., et al. (2023). Divergent modulation of pain and anxiety by GABAergic neurons in the ventrolateral periaqueductal gray and dorsal raphe. Neuropsychopharmacology 48, 1509–1519. doi: 10.1038/s41386-022-01520-0
Xu, Z., and Hökfelt, T. (1997). Expression of galanin and nitric oxide synthase in subpopulations of serotonin neurons of the rat dorsal raphe nucleus. J. Chem. Neuroanat. 13, 169–187. doi: 10.1016/s0891-0618(97)00043-4
Xu, Z., Feng, Z., Zhao, M., Sun, Q., Deng, L., Jia, X., et al. (2021). Whole-brain connectivity atlas of glutamatergic and GABAergic neurons in the mouse dorsal and median raphe nuclei. Elife 10:e65502. doi: 10.7554/eLife.65502
Xu, Z., Zhang, X., Pieribone, V., Grillner, S., and Hökfelt, T. (1998). Galanin-5-hydroxytryptamine interactions: Electrophysiological, immunohistochemical and in situ hybridization studies on rat dorsal raphe neurons with a note on galanin R1 and R2 receptors. Neuroscience 87, 79–94. doi: 10.1016/s0306-4522(98)00151-1
Yaksh, T., Yeung, J., and Rudy, T. (1976). Systematic examination in the rat of brain sites sensitive to the direct application of morphine: Observation of differential effects within the periaqueductal gray. Brain Res. 114, 83–103. doi: 10.1016/0006-8993(76)91009-x
Yamazoe, M., Shiosaka, S., Emson, P., and Tohyama, M. (1985). Distribution of neuropeptide Y in the lower brainstem: An immunohistochemical analysis. Brain Res. 335, 109–120. doi: 10.1016/0006-8993(85)90281-1
Yoon, Y., Lee, J., and Lee, H. (2013). Retrograde study of CART- or NPY-neuronal projection from the hypothalamic arcuate nucleus to the dorsal raphe and/or the locus coeruleus in the rat. Brain Res. 1519, 40–52. doi: 10.1016/j.brainres.2013.04.039
Zahm, D., Parsley, K., Schwartz, Z., and Cheng, A. (2013). On lateral septum-like characteristics of outputs from the accumbal hedonic "hotspot" of Peciña and Berridge with commentary on the transitional nature of basal forebrain "boundaries". J. Comp. Neurol. 521, 50–68. doi: 10.1002/cne.23157
Zhang, H., Li, L., Zhang, X., Ru, G., and Zang, W. (2024a). Role of the dorsal raphe nucleus in pain processing. Brain Sci. 14, 982. doi: 10.3390/brainsci14100982
Zhang, Y., Huang, W., Shan, Z., Zhou, Y., Qiu, T., Hu, L., et al. (2024b). A new experimental rat model of nocebo-related nausea involving double mechanisms of observational learning and conditioning. CNS Neurosci. Ther. 30:e14389. doi: 10.1111/cns.14389
Zhang, Y., Shen, J., Xie, F., Liu, Z., Yin, F., Cheng, M., et al. (2024c). Feedforward inhibition of stress by brainstem neuropeptide Y neurons. Nat. Commun. 15:7603. doi: 10.1038/s41467-024-51956-9
Zhou, X., Stine, C., Prada, P., Fusca, D., Assoumou, K., Dernic, J., et al. (2024). Development of a genetically encoded sensor for probing endogenous nociceptin opioid peptide release. Nat. Commun. 15:5353. doi: 10.1038/s41467-024-49712-0
Keywords: neuropeptide, dorsal raphe nucleus, reward, aversion, behavior
Citation: Braden K and Castro DC (2025) The role of dorsal raphe nucleus neuropeptides in reward and aversion. Front. Behav. Neurosci. 19:1553470. doi: 10.3389/fnbeh.2025.1553470
Received: 30 December 2024; Accepted: 13 March 2025;
Published: 09 April 2025.
Edited by:
Keith B. J. Franklin, McGill University, CanadaReviewed by:
Nivaldo A. P. de Vasconcelos, Federal University of Pernambuco, BrazilCopyright © 2025 Braden and Castro. This is an open-access article distributed under the terms of the Creative Commons Attribution License (CC BY). The use, distribution or reproduction in other forums is permitted, provided the original author(s) and the copyright owner(s) are credited and that the original publication in this journal is cited, in accordance with accepted academic practice. No use, distribution or reproduction is permitted which does not comply with these terms.
*Correspondence: Kathryn Braden, YnJhZGVua0B3dXN0bC5lZHU=
Disclaimer: All claims expressed in this article are solely those of the authors and do not necessarily represent those of their affiliated organizations, or those of the publisher, the editors and the reviewers. Any product that may be evaluated in this article or claim that may be made by its manufacturer is not guaranteed or endorsed by the publisher.
Research integrity at Frontiers
Learn more about the work of our research integrity team to safeguard the quality of each article we publish.