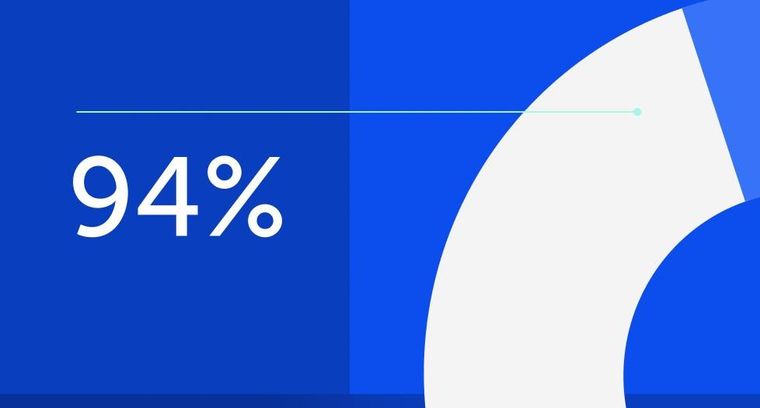
94% of researchers rate our articles as excellent or good
Learn more about the work of our research integrity team to safeguard the quality of each article we publish.
Find out more
ORIGINAL RESEARCH article
Front. Behav. Neurosci., 26 November 2024
Sec. Individual and Social Behaviors
Volume 18 - 2024 | https://doi.org/10.3389/fnbeh.2024.1477984
This article is part of the Research TopicEthological neuroscienceView all 10 articles
Social stress can increase reactive oxygen species and derail antioxidant function in the brain, which may contribute to the onset and progression of mental health disorders. In hierarchical species, repeated social defeat can raise oxidative stress in the brain. However, how oxidative balance in the brain is regulated across different levels in a social hierarchy is unknown. Here, we study the effect of social status on patterns of oxidative stress across several brain divisions in a highly social cichlid fish, Astatotilapia burtoni. In this species, dominant males are territorial, brightly colored, and reproductively active while subordinate males are not. We measured several markers of oxidative stress in macrodissected brain divisions in dominant and subordinate males. We found that dominant individuals had lower oxidative DNA damage (8-OhdG) in the midbrain while also having increased total antioxidant capacity in the midbrain and hypothalamus. However, in dominant males, oxidative DNA damage tended to be higher in the hypothalamus while total glutathione levels were lower in the telencephalon compared to subordinate males. Finally, we found that indicators of reproductive activity (gonadosomatic index and social behavior) were co-regulated with antioxidant function or oxidative damage in the telencephalon. Combined, our results suggest that social status and activation of the reproductive system regulate oxidative balance in the brain in a highly brain division specific manner.
Oxidative stress occurs when reactive oxygen species (ROS) overwhelm the antioxidant and repair systems, resulting in oxidative damage and cellular dysfunction (Janicki-Deverts et al., 2009; Beaulieu et al., 2014; Georgiev et al., 2015; Border et al., 2019). The brain is particularly vulnerable to oxidative stress due to its high oxygen consumption, lipid-rich environment, and relatively low antioxidant defense mechanisms (Kandlur et al., 2020). Accordingly, cumulative oxidative damage in the brain has been implicated in the onset and progression of depression and neurodegenerative disease (Black et al., 2015; Blesa et al., 2015; Cobb and Cole, 2015; Do et al., 2015).
It is well recognized that environmental stressors can increase ROS and derail antioxidant function (Ghezzi et al., 2018; Münzel et al., 2018; Rao et al., 2018). In modern human societies, environmental stressors are mostly social in nature, such as conflict at home, social marginalization, or social isolation (Almeida, 2005; Koenen et al., 2010; Park et al., 2015; Neblett, 2019). A certain degree of social stress is physiologically tolerated but when social stress is persistent (such as prolonged social marginalization or daily social conflict) it can contribute to the development of disease (Picard and McEwen, 2018). Therefore, there is a critical need to understand how social stress influences oxidative stress in the brain and how social stress-induced oxidative insults are regulated by innate defense mechanisms (Walton et al., 2012; Schiavone et al., 2013).
In social animals, competition for access to mates and resources may lead to the emergence of dominance hierarchies (Tibbetts et al., 2022). Individuals with high social status are more aggressive and monopolize mates and resources while those with low social status behave submissively and have fewer reproductive opportunities (Snyder-Mackler et al., 2020). Past studies have shown that social stress arising from social defeat (which leads to low social status) leads to increases in oxidative stress in specific brain regions that are relevant to mood disorders (Schiavone et al., 2013; Salim, 2014; Fedoce et al., 2018). For example, repeated social defeat stress resulted in increased oxidative stress in the hippocampus but not the amygdala and cortex in rats (Patki et al., 2013). However, past studies considered a small subset of brain regions relevant to mood disorders (Patki et al., 2013), used only one marker of oxidative stress (Ibi et al., 2017; Lehmann et al., 2019), and only examined individuals with low social status using brief dyadic encounters in resident-intruder tests (Bouvier et al., 2017; Lim et al., 2020). However, hierarchy establishment in an experimental setting featuring a steady-state group leads to a more realistic and ethologically relevant social structure (Larrieu and Sandi, 2018). Finally, while low social status is clearly stressful, in these group settings high social status can also be challenging since maintaining high social status is psychologically and metabolically demanding due to intense competition for rank and increased investment in reproduction (Archie et al., 2012; Creel et al., 2013; Anderson et al., 2021). However, how social stress alters redox state across the brain of both low and high ranked individuals in stable social hierarchies is unknown (Gonçalves et al., 2008).
Social status is often linked to distinct behavioral and neuroendocrine profiles. Low-ranked individuals typically exhibit increased activation of the hypothalamic–pituitary adrenal (HPA) axis as indicated by high levels of circulating glucocorticoids (Abbott et al., 2003; Goymann and Wingfield, 2004). By contrast, in highly ranked individuals the hypothalamic–pituitary-gonadal (HPG) axis, which controls androgen release and activates the reproductive system, is upregulated (Goymann, 2009; Maruska and Fernald, 2011). Most attention has been given to social stress-induced hyperactivity of the HPA axis and glucocorticoid-mediated effects of oxidative stress in the brain (Patki et al., 2013; Ibi et al., 2017). However, the HPG axis also responds to social stress, with androgen levels increasing when individuals are challenged by others in the hierarchy (Zilioli and Watson, 2014). Less attention has been given to the role of the HPG axis in modulating brain oxidative stress, despite reproduction and androgens having direct effects on oxidative balance in a range of tissue types, including the brain (Bueno et al., 2017; Snyder et al., 2018; Chunchai et al., 2019; Duong et al., 2020; Muthu and Seppan, 2020). Given that rank regulates activation of the HPG axis in many social species, dominance hierarchies are ideally suited to explore the link between HPG axis activity and brain oxidative stress within a social context.
The highly social cichlid fish Astatotilapia burtoni, forms social hierarchies in the laboratory mimicking those found in the wild (Maruska and Fernald, 2018). Male A. burtoni exist as two reversible phenotypes (Figure 1a) dominant and subordinate males (O’Connell and Hofmann, 2012; Maguire et al., 2021). Dominant males have a highly active HPG axis (as indicated by high androgen levels and large gonads), are brightly colored, aggressive, and establish territories where they court females (Border et al., 2021). Subordinate males have a downregulated HPG axis, are non-territorial, and drab in color. Previous studies have indicated that in A. burtoni, dominant males have higher circulating levels of reactive oxygen metabolites, a marker of oxidative damage, than subordinate males (Border et al., 2019, 2021; Fialkowski et al., 2021, 2022), and a similar pattern was found in two other haplochromine cichlid species (Dijkstra et al., 2011). Our model species A. burtoni has unique properties that make it ideal to study the effect of the social environment and competition on brain oxidative stress: (a) the social dominance hierarchy is highly tractable since there are clear phenotypic signatures of social status (Fernald and Hirata, 1979; Alward et al., 2020), and (b) social status mostly results from direct competitive interactions (Dijkstra et al., 2022) and is not significantly complicated by other factors such as affiliative interactions, coalitionary behavior, or hereditary determinants of social status (Snyder-Mackler et al., 2020).
Figure 1. (a) Picture of a dominant and subordinate Astatotilapia burtoni male. (b) Experimental timeline and gonadosomatic index in dominant and subordinate males. (c) Behavior in dominant (D, n = 20) and subordinate (S, n = 20) males. Shown are the number of behaviors per 5 min (chases, aggressive displays, cave visits or flowerpot entries, courtship), time spent shoaling amount during the 5-min observation period, and the dominance index (aggressive behavior and courtship minus flees) averaged across the four weekly filming sessions prior to tissue sampling. Bold lines indicate medians. Boxes enclose 25th to 75th percentiles. Error bars enclose data range, excluding outliers.
In the current study, we assigned social status and quantified social dominance behavior in experimental mixed-sex groups of A. burtoni. We then analyzed markers of oxidative stress (oxidative damage and antioxidant function) in macrodissected brain divisions in dominant and subordinate males. We hypothesize that oxidative stress varies by social status as well as by indicators of HPG axis activity, such as relative gonad size and social dominance behavior.
All animals used in this experiment were adult A. burtoni (approx. 15–18 months; standard length 57–81 mm; weight 5.5–15.5 g; total males n = 40) from a laboratory-bred population that was derived from wild-caught fish collected from the Burundi coast of Lake Tanganyika (Fernald and Hirata, 1977). Fish were housed in aquaria containing gravel substrate and kept at 28°C with a 12-h light/dark cycle containing a 10-min dusk and dawn period to mimic natural settings. Fish were fed cichlid flakes (Omega Sea LLC, Painesville, Ohio) each morning between 8:00 and 10:00 am. All males were individually tagged with colored beads through the dorsal musculature using a stainless-steel tagging gun. All animal care procedures were approved by Central Michigan University Institutional Animal Care and Use Committee (IACUC protocol 15–22) and were in compliance with the US National Research Council’s Guide for the Care and Use of Laboratory Animals, the US Public Health Service’s Policy on Humane Care and Use of Laboratory Animals, and Guide for the Care and Use of Laboratory Animals.
A visual of the experimental timeline is shown in Figure 1b. We divided experimental 110-liter tanks in half widthwise with clear, perforated acrylic barriers into two compartments as described previously (Fialkowski et al., 2021). To assign social status based on size difference, we placed one larger male and one smaller male (weight difference: 2.385 ± 0.930 g; standard length difference: 6.95 ± 1.76 mm) along with 6 females in each experimental compartment. Assignment of status by size was successful as each larger male was dominant while the smaller male was subordinate throughout the duration of the experiment (the hierarchy was typically established in 1 day, with the dominant males defending a flowerpot, expressing a dark eye bar while subordinate males were more cryptically colored and shoaled with females). We set up a total of 30 groups (each containing 2 males and 6 females). Of these 30 groups, 8 groups were lost during the experiment either due to a male that died (in 6 groups the smaller male died) or because the group was adjacent to a group where a male died (2 cases). We further excluded two groups where there was no clear hierarchy with both males assuming dominance status. Our final sample size was 20 groups where the largest male became dominant (n = 20) and the smaller subordinate (n = 20). Males were weighed and their standard length was measured prior to being added to experimental compartments. The initial body mass of dominant males was 10.75 ± 2.098 g (range: 7.7–15.5 g) with a standard length (SL) of 71.75 ± 4.84 mm (range: 63–81 mm). The initial body mass of subordinate males was 8.705 ± 1.868 g (range: 5.5–11.7 g) with a standard length of 65.5 ± 4.68 mm (range: 57–74 mm). In each compartment, one halved terracotta flowerpot was added to promote territoriality in the dominant male. Fish were able to interact physically with members in their own group and visually with those in the adjacent compartment. This allowed for the full expression of social behaviors including aggressive interactions between dominant males between compartments. Fish were allowed to settle in their experimental tank for 2 weeks. We then started weekly filming of each group for behavioral quantification (5 min) between 8 and 10 a.m. for 4–5 weeks. The final recording was made on the morning of tissue collection. During the 4–5 weeks experiment, we recorded the social status of all experimental fish three times per week. Social status was determined by characterizing males as dominant or subordinate, and all males retained the same social status throughout the experiment.
From each experimental group (n = 20), we collected tissue and blood from the dominant male and subordinate male at the end of the experiment (i.e., after the final recording in week 4 or 5). Fish were weighed and measured for standard length before blood and tissue collection. Blood was drawn through the caudal vein using heparinized 26-gauge butterfly needles (Terumo) and plasma was saved for another project. Immediately after blood draw, males were euthanized by spinal cord dislocation. Brains were harvested and rapidly macrodissected using a razor blade into four brain divisions: telencephalon (telencephalon and olfactory bulbs), hypothalamus, midbrain (tectum and thalamus), and hindbrain (cerebellum and hindbrain). When separating the telencephalon from the rest of the brain we made a transverse cut at the anterior commissure. The telencephalon cut likely contained a significant part of the parvocellular portion of the preoptic area. The hypothalamic cut included the magno−/gigantocellular portion of the preoptic area, and it likely also contained a small part of the parvocellular portion. Each brain division was flash frozen in liquid nitrogen then immediately placed in a 1.5 mL tube on a Coolrack block (Biocision, Larkspur, CA, USA) on dry ice. Gonads were weighed for gonadosomatic index data. The experiment was carried out between October 2020 through December 2020. All experimental procedures were done blind with respect to social status.
Using the videos from the previous 4 weeks before tissue collection we quantified frequencies and/or duration of behavior using Behavioral Observation Research Interactive Software [BORIS] (Friard and Gamba, 2016) as described previously (Fialkowski et al., 2022). As indicators of social dominance behaviors, we quantified the number of chases, aggressive display behaviors (border threat and lateral display), cave visits, and courtship displays. We also scored the frequency of fleeing and duration of shoaling (both are indicators of subordinate behavior) and the frequency of foraging behavior. Behavioral recording was done by a single observer.
In each brain division sample, we measured two markers of antioxidant capacity and one marker of oxidative damage. Total antioxidant capacity (TAC) is a measure of both enzymatic and non-enzymatic antioxidant capacity. We also measured glutathione, an important antioxidant (detailed below). In each brain division sample, we evaluated oxidative DNA damage by measuring levels of 8-hydroxy-2′ -deoxyguanosine (8-OhDG). Below is a description of all the assays. All samples were run in duplicate, and we included a pooled tissue sample to evaluate interplate variability for all assays. For all assays, the intra-assay CV (coefficient of variation) and the interassay CV were typically below 5 and 13%, respectively unless indicated otherwise. For all assays we used clear flat-bottom 96-well plates unless indicated otherwise.
We homogenized each brain division sample and prepared different aliquots for total antioxidant capacity (TAC), glutathione, and oxidative DNA damage assay using a generalized homogenization buffer. Each brain division sample was homogenized in tissue homogenization buffer using a motorized pestle mixer (VWR) in a 1.5 mL centrifuge tube. The homogenization buffer consisted of potassium phosphate buffer (50 mM PBS (pH 7.4) and 0.5 mM EDTA) with a Protease Inhibitor Cocktail (Sigma-Aldrich P2714) added at a 1:10 dilution. We used ~10 μL buffer per 1 mg tissue. Once homogenized, the tissue homogenates were aliquoted into 3 separate 0.5 mL centrifuge tubes for further samples preparations for TAC, glutathione, and DNA damage (volumes of tissue homogenates for each assay are indicated below).
For TAC, 40 μL of tissue homogenate was mixed with 40 μL lysis buffer (20 mM Tris–HCl, 137 mM NaCl, 1% NP-40, 10% glycerol, 2 mM EDTA). This mixture was then centrifuged at 4°C at 10,000 g for 10 min as described elsewhere (Fialkowski et al., 2021). The supernatant was removed and stored at −80°C in separate tubes for TAC and protein quantification.
For glutathione, 60 μL tissue homogenate were centrifuged at 10,000 g for 10 min. Following centrifugation, 45 μL supernatant was transferred to a new tube and mixed with 45 μL 5% 5-sulfosalicylic acid dihydrate (SSA) solution and incubated at 4°C for 10 min. Samples were then centrifuged at 10,000 g for another 10 min at 4°C. The supernatant was removed and stored at −80°C in separate tubes for glutathione and protein quantification.
For oxidative DNA damage (8-OHdG), 150 μL of tissue homogenate was centrifuged at 4°C at 10,000 g for 10 min. The supernatant was then discarded, and the remaining pelleted tissue homogenate was stored at −80°C for quantifying DNA damage.
Protein concentration of each prepared brain division sample for glutathione and TAC was measured with a bicinchoninic acid (BCA) Protein Assay kit (Pierce, Rockford IL) following the manufacturer’s protocol. Frozen supernatant was thawed on ice and diluted 1:4 with buffer used in their respective sample preparation. We used 10 μL of this diluted supernatant in the assay. Absorbance was read by a plate reader (Epoch2T, Biotech Instruments, Winooski, VT, USA).
Total antioxidant capacity (TAC) was measured via an Oxygen Radical Absorbance Capacity (ORAC) assay as previously described (Border et al., 2019; Fialkowski et al., 2021). For each sample, the brain division supernatant was standardized to 150 ug protein/mL using the 50:50 lysis-homogenization buffer. We took 50 μL of this 150 ug protein/mL sample and diluted it again 1:2 using PBS to a total volume of 100 μL. We used 20 μL of diluted sample for the ORAC assay. For each brain division sample, ORAC was reported as μmol TE/μg protein. Absorbance was read by a plate reader (Spectramax M3, Molecular Devices, Sunnyvale, CA, USA).
We measured glutathione (GSH) in homogenized brain division samples using a glutathione fluorescence kit (Arbor Assays, Ann Arbor, MI, USA) per manufacturer’s protocol. Samples were first diluted 1:2.5 with kit assay buffer to reduce Aqueous 5-sulfo-salicylic acid dihydrate (SSA) concentration to 1%. Using 50 μL of diluted sample, samples and standards were then added to the plate (Corning 3,686 black half-area microtitre plate), mixed with 25 μL ThioStar detection reagent, and incubated at room temperature for 15 min. Endpoint fluorescence was read using a plate reader (Spectramax M3, Molecular Devices, Sunnyvale, CA, USA) to obtain reduced GSH. We then mixed each sample with 25 μL kit reaction mixture and incubated it for 15 min at room temperature to convert all oxidized GSH dimers (GSSG) in sample to reduced GSH. Following incubation, samples were again measured via endpoint fluorescence reading using the same plate reader mentioned above to obtain total GSH. Due to technical limitations, we were only able to measure total GSH (which is reduced GSH as well as oxidized GSH measured as reduced). All reported values were standardized to the protein concentration in each sample (total GSH/ μg protein).
For each brain division sample, we measured oxidative DNA damage (8-OHdG) following a previously described protocol (Fialkowski et al., 2021) which we modified to allow for measurements of small tissue samples. DNA was extracted from homogenized brain tissue samples using a DNA extraction kit (Zymo quick-DNA miniprep plus kit, Irvine, CA, USA) per manufacturer’s protocol. Purified DNA samples were extracted by adding 200 μL of DNA elution buffer and incubating at room temperature for 5 min before centrifuging at 17,000 g for 1 min to obtain optimal DNA concentrations. We then used ethanol precipitation to increase DNA concentration in each sample through addition of 19 μL 3 M Sodium acetate (pH 5.2) and 475 μL cold ethanol. Samples were then stored at −20°C overnight before undergoing 30-min centrifugation at top speed at 4°C. All ethanol was then removed, and the DNA was reconstituted in 15 μL elution buffer. After ethanol precipitation, the total DNA concentrations were determined using a BioTek take3 reader in an Epoch2T microplate reader using the Nucleic Acid Quantification software provided. We standardized all DNA samples to 200 ng/μL and stored sample at 4°C until digestion.
DNA samples were digested following a modified protocol from Quinlivan and Gregory (2008) and as described previously (Fialkowski et al., 2021). Following digestion, samples were stored at −20°C until use in DNA damage assay.
DNA damage (8-OHdG) was measured with a DNA damage ELISA kit (StressMarq, Biosciences Inc., Victoria, BC, Canada) following manufacturer’s protocol. We diluted each digested sample in sample and standard diluent to ensure samples were put on the plate at a standardized concentration (midbrain and hindbrain: plate concentration 8.33 ng/μL; telencephalon and hypothalamus: plate concentration 7.14 ng/ μL) using the DNA concentrations measured after ethanol precipitation. Results are reported as ng damage (8-OHdG) / ng DNA.
As a measure of investment in the reproductive (HPG) axis, we calculated the gonadosomatic index as GSI = [gonad mass/body mass] * 100 (Bolger and Connolly, 1989). Upregulation of the reproductive axis is also associated with increased territorial behavior whereas downregulation of this axis is linked to fleeing behavior. To quantify this link between investment of the reproductive axis and behavior, we calculated a dominance index for each fish through subtracting the number of flees from the sum of the aggressive behaviors, courtship, and cave visits (Maruska et al., 2013). All analyses were conducted in RStudio using the R packages lme4, lmerTest, MASS (Bates et al., 2015), glmmTMB (Brooks et al., 2017), and emmeans. We identified and excluded outliers in our oxidative stress data based on Tukey’s rule (between 0 and 3 values were excluded per measurement with most measurements having no outliers).
Most behaviors were (almost) exclusively exhibited by either subordinate or dominant males. We therefore compared total aggression (sum of chases and aggressive display behaviors), cave visits, courtship, fleeing events, and shoaling duration, averaged for each male across the 4-week observation period, using Wilcoxon Signed-Rank (WSR) on paired samples (comparing dominant and subordinate males in the same group).
We used linear mixed models (LMMs) with a maximum-likelihood protocol with social status and/or TAC as fixed effects and pair as a random effect. We compared GSI between dominant and subordinate males using LMMs with social status as fixed effect. We ran similar models to compare levels of TAC, DNA damage, and total GSH between dominant and subordinate males for each brain division separately. To test for social status specific regulation of oxidative balance, we tested, for each brain division separately, whether variation in oxidative DNA damage was explained by TAC in a social status-specific manner using LMM with social status and TAC as interaction effect. In all analyses we also included body weight but this effect was only retained if significant. To evaluate the validity of our models, we examined the residuals, qqplots, and plots of predicted values versus residuals.
To examine whether HPG axis regulation is related to patterns of oxidative stress in the brain, we examined covariance patterns of the different measures of oxidative stress with two markers of HPG axis activity, the dominance index and gonadosomatic index (GSI). We carried out this analysis in dominant and subordinate males separately and created heatmaps using the R package gplots. In dominant males, we also included aggressive display (sum of lateral display and border threat) and chases in the analysis. The behavioral data used in the covariance analysis was based on the final video recorded immediately before tissue collection, closest to the physiological sampling period. We carried out hierarchical cluster analysis to determine clusters that were co-regulated. p-values for each cluster were obtained through multiscale bootstrap resampling (10,000 steps) using the pvclust package with the Ward clustering method (Suzuki and Shimodaira, 2006). A significance level (α) of 0.05 was used for all tests. We report mean ± SE for our model estimates.
Males did not change social status during the entire duration of the experiment. We calculated the average rate or duration of different behaviors across the four weekly five-minute observations (Figure 1c). Compared to subordinate males, dominant males exhibited more chases (WSR test, V = 210, p < 0.0001), more aggressive display behaviors (V = 210, p < 0.0001), more cave visits (V = 210, p < 0.0001), and more courtship behavior than subordinate males (V = 190, p < 0.0001). Conversely, subordinate males performed more flee behavior (WSR test, V = 0, p = 0.0009) and showed a greater duration of shoaling behavior (V = 0, p = 0.0009) than dominant males. The dominant index (DI, sum of aggressive and courtship behavior minus flees) was significantly higher in dominant than subordinate males (WSR test, V = 210, p < 0.0001). There was no difference in foraging behavior between dominant and subordinate males (WSR test, V = 46.5, p = 0.57). These results confirm that dominant males direct territorial aggression towards subordinate males. Dominant males had greater GSI than subordinate males (LMM, −0.20474 ± 0.04457, t20 = −4.594, p = 0.000176, Figure 1b), confirming that the former had an activated reproductive system.
We evaluated the effect of social status on multiple markers of oxidative stress (TAC, total glutathione, oxidative DNA damage) in the telencephalon, midbrain, hindbrain, and hypothalamus (Figure 2a). Here we report p values in the text only (for complete statistical results, see Table 1). We found that dominant males had higher levels of TAC than subordinate males in both the midbrain (p < 0.002) and hypothalamic region (p < 0.005). However, total glutathione levels were significantly higher in the telencephalon in subordinate males compared to dominant males (p < 0.02). We further found that dominant males had lower levels of oxidative DNA damage in the midbrain (p < 0.006). However, in the hypothalamus the average level of oxidative DNA damage was higher in dominant males than in subordinate males, but we note that this effect was not significant (p < 0.07). A schematic summary of patterns of oxidative stress in the brain relative to social status is shown in Figure 2b.
Figure 2. (a) Measurements of oxidative stress in dominant (D) and subordinate (S) males in each of the four brain divisions. Shown is data for total antioxidant capacity, total glutathione (GSH), and oxidative DNA damage. We collected samples from 20 dominant and 20 subordinate males but sample sizes ranged across assays (final sample sizes are shown in Table 1). Boxes enclose 25th to 75th percentiles. Error bars enclose data range, excluding outliers. Dots are data points. TE: Trolox equivalents. (b) Summary schematic of social status specific patterns of oxidative stress in the brain as shown in panel ‘a’. Arrows represent a significant effect (dashed arrow indicates a marginally non-significant effect).
Table 1. Statistical results comparing measurements of oxidative stress between dominant and subordinate males.
To test for social status specific regulation of oxidative balance, we tested whether variation in oxidative DNA damage was explained by total antioxidant capacity in a social status-specific manner (Figure 3). We found evidence for an interaction effect of social status x TAC on oxidative DNA damage in three brain divisions (Table 2). This effect was significant in the telencephalon (p < 0.02) and borderline non-significant in the hypothalamus (p < 0.07) and hindbrain (p < 0.08). When split by social status, TAC had a significant effect on oxidative DNA damage in several tests (Figure 3; solid lines indicate significant effects), and in all cases this effect was positive.
Figure 3. Status-dependent relationship between levels of oxidative DNA damage and total antioxidant capacity in specific brain divisions. Circles are dominant males and triangles are subordinate males. The relationships for dominant males (solid lines) and subordinate males (dashed lines) are shown when the significance level of the interaction effect between total antioxidant function and social status on oxidative DNA damage was p < 0.1.
Table 2. Statistical results testing the effect of total antioxidant capacity (TAC) and social status (dominant and subordinate males) on oxidative DNA damage (8-OHdG).
We performed a hierarchical cluster analysis involving all oxidative stress measurements and two indicators of HPG axis activation: gonadosomatic index (GSI) and dominance index (DI). In dominant males, we also included chases and aggressive display behaviors in the analysis. We found two significant clusters where a marker of oxidative stress co-varied with either GSI, DI, or behavior in both dominant (Figure 4a) and subordinate males (Figure 4b). In dominant males we found that within the telencephalon aggressive display frequency was co-regulated with oxidative DNA damage (hierarchical cluster analysis, p = 0.01), and GSI was co-regulated with glutathione levels (p = 0.04). Other significant clusters were DI and chase frequency (p = 0.02) and TAC and glutathione levels within the hindbrain (p = 0.04). In subordinate males, DI (which in subordinate males shows more negative values corresponding to more fleeing behavior) was co-regulated with glutathione levels in the telencephalon (p = 0.04). We also found that within subordinate males, GSI was co-regulated with four measurements of oxidative stress in the brain, including TAC and oxidative DNA damage in the telencephalon (p < 0.05). Finally, there was a significant cluster containing TAC and glutathione levels within the hindbrain of subordinate males (p = 0.01).
Figure 4. Covariances patterns across markers of oxidative stress combined with indicators of reproduction and social dominance (gonadosomatic index and behavior) for dominant (a) and subordinate males (b). For dominant males, we used both the dominance index and measures of overt (chases) and covert aggression (display behaviors). The dominance index is calculated as aggressive behavior + courtship minus flees, and hence in subordinate males lower, more negative dominance index values correspond to more fleeing behavior. For subordinate males, we only used the dominance index due to the lack of aggressive behaviors. Hierarchical clustering revealed significant (indicated by solid box) clusters in both dominant (n = 20) and subordinate males (n = 20). Each cell represents the correlation value, with red indicating positive correlations and blue indicating negative correlations. Circles are dominant males and triangles are subordinate males. TAC: Total antioxidant capacity; GSH: glutathione; DNA: oxidative DNA damage (8-OHdG).
We found support for the hypothesis that social status and the extent of HPG axis regulation impacts oxidative stress in the brain. Although circulating oxidative damage is higher in dominant males in our study species (Border et al., 2019), they generally exhibited higher total antioxidant capacity and lower oxidative DNA damage in the brain. However, these patterns were brain division specific, with dominant males showing lower glutathione levels in the telencephalon and a higher levels of oxidative DNA damage in the hypothalamus (this latter effect was marginally non-significant) relative to subordinate males. Social dominance index and gonadosomatic index, which are indicators of HPG axis activity, were co-regulated with some oxidative stress measurements in the brain. Below we will discuss these findings in more detail.
Our findings show that both antioxidant capacity and oxidative DNA damage are impacted by social status but these effects varied by brain division and were marker specific. These general findings are consistent with the idea that distinct social challenges experienced by dominant and subordinate males could impact patterns of oxidative balance in the brain. Social status has been linked to processes in the brain that can alter redox homeostasis, such as differences in synaptic plasticity (Behrens et al., 2007; Hasam-Henderson et al., 2018) as well as rank-specific activation of neural circuits that regulate social behavior (Fernald and Maruska, 2012; Weitekamp et al., 2017). Here we focus on the possibility that social status effects on oxidative stress in the brain are mediated by social status-specific activation of neuroendocrine systems, such as the HPA axis and the HPG axis.
The reduced antioxidant capacity in subordinate males in two brain divisions is consistent with the idea that social stress may impair redox balance in the brain. Chronic psychosocial stress experienced by socially subordinate individuals increases basal levels of glucocorticoids (Creel, 2001; Abbott et al., 2003; Goymann and Wingfield, 2004; Sapolsky, 2004; Creel et al., 2013; Larrieu and Sandi, 2018), which is also the case in A. burtoni with subordinate males typically exhibiting higher levels of cortisol than dominant males (Fox et al., 1997). High levels of glucocorticoids make neurons across the brain more susceptible to oxidative insults via well-described actions of glucocorticoids, including downregulating several antioxidant enzymes and increasing baseline levels of ROS production (Şahin and Gümüşlü, 2004; Spiers et al., 2015; Torres et al., 2021). The increased oxidative DNA damage in the midbrain in subordinate males compared to dominant males is consistent with social subordination impairing the ability to maintain proper redox homeostasis in specific parts of the brain.
However, there was also some support for an oxidative cost of social dominance: dominant males tended to have higher levels of oxidative DNA damage (but note that this effect was non-significant) in the hypothalamus and had reduced glutathione levels in the telencephalon. As stated earlier, dominant A. burtoni males have an upregulated reproductive HPG axis and increased androgen levels to facilitate reproduction (mate searching, courtship, gonadal growth). Androgens can directly modulate oxidative balance since androgens can have both neuroprotective and neurotoxic effects (Bueno et al., 2017; Snyder et al., 2018; Chunchai et al., 2019) depending on the oxidative state of specific neurons (Duong et al., 2020). It is possible that social status differences in circulating androgens at least in part mediate differences in oxidative stress between dominant and subordinate males.
To further examine how activation of the HPG axis regulates patterns of oxidative stress in the brain, we took advantage of the fact that both dominant and subordinate males exhibit individual variation in indicators of HPG axis activity, the dominance index (DI, essentially a measure of the strength of territoriality), aggressive behavior (chases and aggressive displays) and gonadosomatic index (GSI). Both DI and GSI were co-regulated with markers of oxidative stress in specific brain divisions in both dominant and subordinate males. In dominant males, GSI was co-regulated with glutathione levels in the telencephalon and aggressive displays were co-regulated with oxidative damage in the telencephalon. In subordinate males, DI was co-regulated with glutathione levels in the telencephalon. The fact that these associations occurred in the telencephalon supports our suggestion that HPG axis regulation and androgens modulate oxidative balance, because the telencephalon of A. burtoni expresses a high density of androgen and estrogen receptors (Burmeister et al., 2007), and hence it would be easy to expect a potentially stronger androgenic regulation of oxidative stress in this part of the brain (possibly via estrogenic signaling after the local conversion of androgens into estrogens). It should be noted however, that glucocorticoid signaling may also explain some of these associations. For example, in subordinate males we found that more negative DI scores, which reflect more fleeing behavior, were linked to reduced glutathione levels in the telencephalon. This pattern is consistent with the high density of glucocorticoid receptors in the teleost telencephalon (Natsaridis et al., 2023) and the well-described negative effects of glucocorticoids on brain antioxidant function (McIntosh et al., 1998). Our findings are consistent with a previous study where the rate of social dominance behavior, which is influenced by the HPG axis, was positively related to total antioxidant function in the brain of the cichlid fish Neolamprologus pulcher (Culbert et al., 2022). An effect of HPG axis regulation on oxidative balance in the brain is also supported by brain transcriptomics data showing the pathways involved in metabolism and redox homeostasis are coregulated with indicators of HPG axis activation in A. burtoni (Peter Dijkstra and Ryan Wong, unpublished data).
To specifically test whether HPG axis activation influences oxidative stress in the brain, future studies should directly manipulate HPG axis using pharmacology (O’Connell and Hofmann, 2012) or genetically altered A. burtoni where specific androgen receptors have been disrupted (Alward et al., 2020). We note that in the current study, circulating androgen levels were not measured. Although gonadosomatic index and dominance index are correlated with androgen levels (Dijkstra, unpublished data), directly linking androgen levels to patterns of oxidative stress in the brain will be an important future direction. Furthermore, while our approach provides important insight into broad-scale patterns of brain oxidative stress, we may have missed regional differences in oxidative stress. Future studies should therefore define the effect of social status and HPG axis regulation on oxidative damage in specific brain regions that regulate adaptive responses to social challenges. Ideally, these brain region specific patterns of oxidative stress should be linked to variation in androgen or estrogen receptor density in specific cells of the nervous system (e.g., neurons vs. glial cells) as well as circulating levels of androgens.
There are additional mechanisms that could induce higher levels of oxidative stress in specific parts of the brain of dominant males. Dominant males face greater cognitive demands than subordinate males because they need to be more attentive and responsive to changes in the social landscape while maintaining their position in the hierarchy (Sapolsky, 2005). Accordingly, adult neurogenesis is typically higher in dominant males than subordinate males across vertebrates (Maruska et al., 2012; Holmes, 2016; Tea et al., 2019), including A. burtoni. Since adult neurogenesis can increase ROS generation (Walton et al., 2012), dominant males may be susceptible to localized oxidative stress in regions of the brain where neurogenesis occurs (e.g., the hippocampus).
Although our study was largely framed within the context of social stress and redox balance in the brain, it would be interesting to investigate how patterns of oxidative stress in the brain are relevant to Darwinian fitness and behavioral decision making in a natural context. The cichlid fish A. burtoni inhabits Lake Tanganyika and associated river systems (Fernald and Hirata, 1977). It lives in shallow shore pools and rivers where males compete aggressively for social dominance and territory ownership which is linked to mating success. The patterns of oxidative stress in the brain could influence the relative cost and benefits of high and low social status in cichlid dominance hierarchies. Both dominant (territorial) and subordinate (non-territorial) status have a distinct cost (Hofmann et al., 1999; Dijkstra et al., 2013). For example, in A. burtoni dominant males grow slower than subordinate males (Hofmann et al., 1999), dominant males were found to display higher levels of circulating oxidative damage than subordinate males (Border et al., 2019; Fialkowski et al., 2021), and the level of territorial defense was linked to circulating oxidative damage in a color phenotype dependent manner (Border et al., 2019; Dijkstra et al., 2024). Our study suggests that we may need to consider oxidative stress in the brain when investigating the costs of high and low rank in group-living organisms. We also note that the oxidative cost of social dominance and reproduction has received considerable interest in the field of evolution and life history theory aimed at understanding the trade-off between competing life history traits, such as reproduction and lifespan (Beaulieu et al., 2014; Sharick et al., 2015; Marasco et al., 2017). Given the importance of the brain for making optimal behavioral decisions and the impact of redox balance in the brain on behavior (Vicente-Gutierrez et al., 2019), improving our understanding of how demanding activities impact oxidative balance in the brain is a fruitful avenue for future studies to those who are interested in the social determinants of Darwinian fitness and health.
We have previously shown that social status and reproduction affect patterns of oxidative stress in the peripheral tissue of cichlid fish (Border et al., 2019; Sawecki et al., 2019; Fialkowski et al., 2021; Culbert et al., 2023). In the current study, we expand on these findings to show that social status also influences patterns of oxidative stress in the central nervous system. Cumulative oxidative damage is one of the main mechanisms determining vulnerability to depression and driving age-related changes in neurodegenerative disease (Kandlur et al., 2020). A deeper understanding of the sources of oxidative stress during social challenges, and how innate defensive mechanisms are activated across the entire brain is critical for expanding our understanding of how brain health is maintained in the face of social stress and aging (Liu et al., 2017). Our study lays a foundation for future research on the effect of social competition on oxidative stress in the brain in a tractable model organism, amenable to social manipulation (Rodriguez-Santiago et al., 2020; Piefke et al., 2021).
The raw data supporting the conclusions of this article will be made available by the authors upon request.
The animal study was approved by Central Michigan University Institutional Animal Care and Use Committee. The study was conducted in accordance with the local legislation and institutional requirements.
PD: Conceptualization, Data curation, Formal analysis, Funding acquisition, Investigation, Methodology, Project administration, Resources, Supervision, Validation, Visualization, Writing – original draft, Writing – review & editing. RF: Conceptualization, Data curation, Investigation, Methodology, Project administration, Writing – review & editing. BB: Investigation, Writing – review & editing. RW: Funding acquisition, Writing – review & editing, Conceptualization. TM: Investigation, Writing – review & editing. AH: Formal analysis, Visualization, Writing – review & editing.
The author(s) declare that financial support was received for the research, authorship, and/or publication of this article. This research was supported by a grant from Central Michigan University’s Neuroscience Program and a grant from the National Institute of General Medical Sciences (NIGMS, R15GM150286).
We thank Andrew Drumheller for providing helpful comments to earlier drafts of the manuscript. Deric Learman, Benjamin Swarts, and Hans Hofmann provided technical support.
The authors declare that the research was conducted in the absence of any commercial or financial relationships that could be construed as a potential conflict of interest.
All claims expressed in this article are solely those of the authors and do not necessarily represent those of their affiliated organizations, or those of the publisher, the editors and the reviewers. Any product that may be evaluated in this article, or claim that may be made by its manufacturer, is not guaranteed or endorsed by the publisher.
Abbott, D. H., Keverne, E. B., Bercovitch, F. B., Shively, C. A., Mendoza, S. P., Saltzman, W., et al. (2003). Are subordinates always stressed? A comparative analysis of rank differences in cortisol levels among primates. Horm. Behav. 43, 67–82. doi: 10.1016/S0018-506X(02)00037-5
Almeida, D. M. (2005). Resilience and vulnerability to daily stressors assessed via diary methods. Curr. Dir. Psychol. Sci. 14, 64–68. doi: 10.1111/j.0963-7214.2005.00336.x
Alward, B. A., Laud, V. A., Skalnik, C. J., York, R. A., Juntti, S. A., and Fernald, R. D. (2020). Modular genetic control of social status in a cichlid fish. Proc. Natl. Acad. Sci. USA 117, 28167–28174. doi: 10.1073/pnas.2008925117
Anderson, J. A., Johnston, R. A., Lea, A. J., Campos, F. A., Voyles, T. N., Akinyi, M. Y., et al. (2021). High social status males experience accelerated epigenetic aging in wild baboons. eLife 10:e66128. doi: 10.7554/ELIFE.66128
Archie, E. A., Altmann, J., and Alberts, S. C. (2012). Social status predicts wound healing in wild baboons. Proc. Natl. Acad. Sci. USA 109, 9017–9022. doi: 10.1073/pnas.1206391109
Bates, D., Mächler, M., Bolker, B. M., and Walker, S. C. (2015). Fitting linear mixed-effects models using lme4. J. Stat. Softw. 67, 1–48.
Beaulieu, M., Mboumba, S., Willaume, E., Kappeler, P. M., and Charpentier, M. J. E. (2014). The oxidative cost of unstable social dominance. J. Exp. Biol. 217, 2629–2632. doi: 10.1242/jeb.104851
Behrens, M. M., Ali, S. S., Dao, D. N., Lucero, J., Shekhtman, G., Quick, K. L., et al. (2007). Ketamine-induced loss of phenotype of fast-spiking interneurons is mediated by NADPH-oxidase. Science 318, 1645–1647. doi: 10.1126/SCIENCE.1148045
Black, C. N., Bot, M., Scheffer, P. G., Cuijpers, P., and Penninx, B. W. J. H. (2015). Is depression associated with increased oxidative stress? A systematic review and meta-analysis. Psychoneuroendocrinology 51, 164–175. doi: 10.1016/J.PSYNEUEN.2014.09.025
Blesa, J., Trigo-Damas, I., Quiroga-Varela, A., and Jackson-Lewis, V. R. (2015). Oxidative stress and Parkinson’s disease. Front. Neuroanat. 9, 1–9. doi: 10.3389/fnana.2015.00091
Bolger, T., and Connolly, P. L. (1989). The selection of suitable indices for the measurement and analysis of fish condition. J. Fish Biol. 34, 171–182. doi: 10.1111/J.1095-8649.1989.TB03300.X
Border, S. E., Deoliveira, G. M., Janeski, H. M., Piefke, T. J., Brown, T. J., and Dijkstra, P. D. (2019). Social rank, color morph, and social network metrics predict oxidative stress in a cichlid fish. Behav. Ecol. 30, 490–499. doi: 10.1093/beheco/ary189
Border, S. E., Piefke, T. J., Funnell, T. R., Fialkowski, R. F., Sawecki, J., and Dijkstra, P. D. (2021). Social instability influences rank-specific patterns of oxidative stress in a cichlid fish. J. Exp. Biol. 224:jeb237172. doi: 10.1242/jeb.237172
Bouvier, E., Brouillard, F., Molet, J., Claverie, D., Cabungcal, J. H., Cresto, N., et al. (2017). Nrf2-dependent persistent oxidative stress results in stress-induced vulnerability to depression. Mol. Psychiatry 22, 1701–1713. doi: 10.1038/mp.2016.144
Brooks, M. E., Kristensen, K., van Benthem, K. J., Magnusson, A., Berg, C. W., Nielsen, A., et al. (2017). glmmTMB balances speed and flexibility among packages for zero-inflated generalized linear mixed modeling. R J. 9, 378–400. doi: 10.32614/rj-2017-066
Bueno, A., Carvalho, F. B., Gutierres, J. M., Lhamas, C., and Andrade, C. M. (2017). A comparative study of the effect of the dose and exposure duration of anabolic androgenic steroids on behavior, cholinergic regulation, and oxidative stress in rats. PLoS One 12:e0177623. doi: 10.1371/JOURNAL.PONE.0177623
Burmeister, S. S., Kailasanath, V., and Fernald, R. D. (2007). Social dominance regulates androgen and estrogen receptor gene expression. Horm. Behav. 51, 164–170. doi: 10.1016/j.yhbeh.2006.09.008
Chunchai, T., Apaijai, N., Keawtep, P., Mantor, D., Arinno, A., Pratchayasakul, W., et al. (2019). Testosterone deprivation intensifies cognitive decline in obese male rats via glial hyperactivity, increased oxidative stress, and apoptosis in both hippocampus and cortex. Acta Physiol. 226:e13229. doi: 10.1111/APHA.13229
Cobb, C. A., and Cole, M. P. (2015). Oxidative and nitrative stress in neurodegeneration. Exp. Neurol. 84, 4–21. doi: 10.1016/j.nbd.2015.04.020
Creel, S. (2001). Social dominance and stress hormones. Trends Ecol. Evol. 16, 491–497. doi: 10.1016/S0169-5347(01)02227-3
Creel, S., Dantzer, B., Goymann, W., and Rubenstein, D. R. (2013). The ecology of stress: effects of the social environment. Funct. Ecol. 27, 66–80. doi: 10.1111/j.1365-2435.2012.02029.x
Culbert, B. M., Border, S. E., Fialkowski, R. J., Bolitho, I., and Dijkstra, P. D. (2023). Social rank influences relationships between hormones and oxidative stress in a cichlid fish. Horm. Behav. 152:105365. doi: 10.1101/2022.10.13.512121
Culbert, B. M., Dijkstra, P. D., Gilmour, K. M., and Balshine, S. (2022). Antioxidant capacity differs across social ranks and with ascension in males of a group-living fish. Comp. Biochem. Physiol. -Part A Mol. Integr. Physiol. 265:111126. doi: 10.1016/j.cbpa.2021.111126
Dijkstra, P. D., Funnell, T. R., Fialkowski, R. J., Piefke, T. J., Border, S. E., Aufdemberge, P. M., et al. (2024). Sexual selection may support phenotypic plasticity in male coloration of an African cichlid fish. Proc. R. Soc. B Biol. Sci. 291:20241127. doi: 10.1098/rspb.2024.1127
Dijkstra, P. D., Piefke, T. J., and Bonnell, T. R. (2022). Behavioral changes during social ascent and descent in replicate social networks of an African cichlid fish. Hydrobiologia 850, 1–19. doi: 10.1007/S10750-022-04980-Z/FIGURES/6
Dijkstra, P. D., Seehausen, O., and Metcalfe, N. B. (2013). Metabolic divergence between sibling species of cichlids Pundamilia nyererei and Pundamilia pundamilia. J. Fish Biol. 82, 1975–1989. doi: 10.1111/jfb.12125
Dijkstra, P. D., Wiegertjes, G. F., Forlenza, M., van der Sluijs, I., Hofmann, H. A., Metcalfe, N. B., et al. (2011). The role of physiology in the divergence of two incipient cichlid species. J. Evol. Biol. 24, 2639–2652. doi: 10.1111/j.1420-9101.2011.02389.x
Do, K. Q., Cuenod, M., and Hensch, T. K. (2015). Targeting oxidative stress and aberrant critical period plasticity in the developmental trajectory to schizophrenia. Schizophr. Bull. 41, 835–846. doi: 10.1093/schbul/sbv065
Duong, P., Tenkorang, M. A. A., Trieu, J., McCuiston, C., Rybalchenko, N., and Cunningham, R. L. (2020). Neuroprotective and neurotoxic outcomes of androgens and estrogens in an oxidative stress environment. Biol. Sex Differ. 11, 12–18. doi: 10.1186/s13293-020-0283-1
Fedoce, A., Ferreira, F., Bota, R. G., Bonet-Costa, V., Sun, P. Y., and Davies, K. J. A. (2018). The role of oxidative stress in anxiety disorder: cause or consequence? Free Radic. Res. Commun. 52, 737–750. doi: 10.1080/10715762.2018.1475733
Fernald, R. D., and Hirata, N. R. (1977). Field study of Haplochromis burtoni: quantitative behavioural observations. Anim. Behav. 25, 964–975. doi: 10.1016/0003-3472(77)90048-3
Fernald, R. D., and Hirata, N. R. (1979). The ontogeny of social behavior and body coloration in the african cichlid fish haplochromis burtoni. Zeitschrif Tierpsychologie 50, 180–187. doi: 10.1111/j.1439-0310.1979.tb01025.x
Fernald, R. D., and Maruska, K. P. (2012). Social information changes the brain. Proc. Natl. Acad. Sci. USA 109, 17194–17199. doi: 10.1073/pnas.1202552109
Fialkowski, R., Aufdemberge, P., Wright, V., and Dijkstra, P. (2021). Radical change: temporal patterns of oxidative stress during social ascent in a dominance hierarchy. Behav. Ecol. Sociobiol. 75:43. doi: 10.1007/s00265-021-02981-x
Fialkowski, R. J., Border, S. E., Bolitho, I., and Dijkstra, P. D. (2022). Social dominance and reproduction result in increased integration of oxidative state in males of an African cichlid fish. Comp. Biochem. Physiol. Part A Mol. Integr. Physiol. 269:111216. doi: 10.1016/j.cbpa.2022.111216
Fox, H. E., White, S., Kao, M. H. F., and Fernald, R. D. (1997). Stress and dominance in a social fish. J. Neurosci. 17, 6463–6469. doi: 10.1523/JNEUROSCI.17-16-06463.1997
Friard, O., and Gamba, M. (2016). BORIS: a free, versatile open-source event-logging software for video/audio coding and live observations. Methods Ecol. Evol. 7, 1325–1330. doi: 10.1111/2041-210X.12584
Georgiev, A. V., Thompson, M. E., Mandalaywala, T. M., and Maestripieri, D. (2015). Oxidative stress as an indicator of the costs of reproduction among free-ranging rhesus macaques. J. Exp. Biol. 218, 1981–1985. doi: 10.1242/jeb.121947
Ghezzi, P., Floridi, L., Boraschi, D., Cuadrado, A., Manda, G., Levic, S., et al. (2018). Oxidative stress and inflammation induced by environmental and psychological stressors: a biomarker perspective. Antioxidants Redox Signal. 28, 852–872. doi: 10.1089/ARS.2017.7147
Gonçalves, L., Dafre, A. L., Carobrez, S. G., and Gasparotto, O. C. (2008). A temporal analysis of the relationships between social stress, humoral immune response and glutathione-related antioxidant defenses. Behav. Brain Res. 192, 226–231. doi: 10.1016/j.bbr.2008.04.010
Goymann, W. (2009). Social modulation of androgens in male birds. Gen. Comp. Endocrinol. 163, 149–157. doi: 10.1016/j.ygcen.2008.11.027
Goymann, W., and Wingfield, J. C. (2004). Allostatic load, social status and stress hormones: the costs of social status matter. Anim. Behav. 67, 591–602. doi: 10.1016/j.anbehav.2003.08.007
Hasam-Henderson, L., Gotti, G., Mishto, M., Klisch, C., Gerevich, Z., Geiger, J., et al. (2018). NMDA-receptor inhibition and oxidative stress during hippocampal maturation differentially alter parvalbumin expression and gamma-band activity. Sci. Rep. 8:9545. doi: 10.1038/S41598-018-27830-2
Hofmann, H. A., Benson, M. E., and Fernald, R. D. (1999). Social status regulates growth rate: consequences for life-history strategies. Proc. Natl. Acad. Sci. USA 96, 14171–14176. doi: 10.1073/pnas.96.24.14171
Holmes, M. M. (2016). Social regulation of adult neurogenesis: a comparative approach. Front. Neuroendocrinol. 41, 59–70. doi: 10.1016/j.yfrne.2016.02.001
Ibi, M., Liu, J., Arakawa, N., Kitaoka, S., Kawaji, A., Matsuda, K., et al. (2017). Depressive-like behaviors are regulated by NOX1/NADPH oxidase by redox modification of NMDA receptor 1. J. Neurosci. 37, 4200–4212. doi: 10.1523/JNEUROSCI.2988-16.2017
Janicki-Deverts, D., Cohen, S., Matthews, K., Gross, M., and Jacobs, D. (2009). Socioeconomic status, antioxidant micronutrients, and correlates of oxidative damage: the coronary artery risk development in young adults (CARDIA) study. Psychosom. Med. 71, 541–548. doi: 10.1097/PSY.0B013E31819E7526
Kandlur, A., Satyamoorthy, K., and Gangadharan, G. (2020). Oxidative stress in cognitive and epigenetic aging: a retrospective glance. Front. Mol. Neurosci. 13:41. doi: 10.3389/fnmol.2020.00041
Koenen, K. C., Uddin, M., Amstadter, A. B., and Galea, S. (2010). Incorporating the social environment in genotype environment interaction studies of mental disorders. Int. J. Clin. Pract. 64, 1489–1492. doi: 10.1111/J.1742-1241.2010.02359.X
Larrieu, T., and Sandi, C. (2018). Stress-induced depression: is social rank a predictive risk factor? BioEssays 40, 1–10. doi: 10.1002/bies.201800012
Lehmann, M. L., Weigel, T. K., Poffenberger, C. N., and Herkenham, M. (2019). The behavioral sequelae of social defeat require microglia and are driven by oxidative stress in mice. J. Neurosci. 39, 5594–5605. doi: 10.1523/JNEUROSCI.0184-19.2019
Lim, C. H., Soga, T., Levavi-Sivan, B., and Parhar, I. S. (2020). Chronic social defeat stress up-regulates Spexin in the brain of Nile Tilapia (Oreochromis niloticus). Sci. Rep. 10, 7666–7668. doi: 10.1038/s41598-020-64639-4
Liu, Z., Zhou, T., Ziegler, A. C., Dimitrion, P., and Zuo, L. (2017). Oxidative stress in neurodegenerative diseases: from molecular mechanisms to clinical applications. Oxidative Med. Cell. Longev. 2017:2525967. doi: 10.1155/2017/2525967
Maguire, S. M., DeAngelis, R., Dijkstra, P. D., Jordan, A., and Hofmann, H. A. (2021). Social network dynamics predict hormone levels and behavior in a highly social cichlid fish. Horm. Behav. 132:104994. doi: 10.1016/j.yhbeh.2021.104994
Marasco, V., Stier, A., Boner, W., Griffiths, K., Heidinger, B., and Monaghan, P. (2017). Environmental conditions can modulate the links among oxidative stress, age, and longevity. Mech. Ageing Dev. 164, 100–107. doi: 10.1016/J.MAD.2017.04.012
Maruska, K. P., Carpenter, R. E., and Fernald, R. D. (2012). Characterization of cell proliferation throughout the brain of the African cichlid fish Astatotilapia burtoni and its regulation by social status. J. Comp. Neurol. 520, 3471–3491. doi: 10.1002/cne.23100
Maruska, K. P., and Fernald, R. D. (2011). Social regulation of gene expression in the hypothalamic-pituitary-gonadal axis. Physiology 26, 412–423. doi: 10.1152/physiol.00032.2011
Maruska, K. P., Becker, L., Neboori, A., and Fernald, R. D. (2013). Social descent with territory loss causes rapid behavioral, endocrine and transcriptional changes in the brain. J. Exp. Biol. 216, 3656–3666. doi: 10.1242/jeb.088617
Maruska, K. P., and Fernald, R. D. (2018). Astatotilapia burtoni: a model system for analyzing the neurobiology of behavior. ACS Chem. Neurosci. 9, 1951–1962. doi: 10.1021/acschemneuro.7b00496
McIntosh, L. J., Hong, K. E., and Sapolsky, R. M. (1998). Glucocorticoids may alter antioxidant enzyme capacity in the brain: baseline studies. Brain Res. 791, 209–214. doi: 10.1016/S0006-8993(98)00115-2
Münzel, T., Sørensen, M., Schmidt, F., Schmidt, E., Steven, S., Kröller-Schön, S., et al. (2018). The adverse effects of environmental noise exposure on oxidative stress and cardiovascular risk. Antioxidants Redox Signal. 28, 873–908. doi: 10.1089/ARS.2017.7118
Muthu, S. J., and Seppan, P. (2020). Apoptosis in hippocampal tissue induced by oxidative stress in testosterone deprived male rats. Aging Male 23, 1598–1610. doi: 10.1080/13685538.2021.1892625
Natsaridis, E., Perdikaris, P., Fokos, S., and Dermon, C. R. (2023). Neuronal and astroglial localization of glucocorticoid receptor GRα in adult zebrafish brain (Danio rerio). Brain Sci. 13:861. doi: 10.3390/brainsci13060861
Neblett, E. W. (2019). Racism and health: challenges and future directions in behavioral and psychological research. Cultur. Divers. Ethnic Minor. Psychol. 25, 12–20. doi: 10.1037/CDP0000253
O’Connell, L. A., and Hofmann, H. A. (2012). Social status predicts how sex steroid receptors regulate complex behavior across levels of biological organization. Endocrinology 153, 1341–1351. doi: 10.1210/en.2011-1663
Park, M., Verhoeven, J. E., Cuijpers, P., Reynolds, C. F., and Penninx, B. W. J. H. (2015). Where you live may make you old: the association between perceived poor neighborhood quality and leukocyte telomere length. PLoS One 10:e0128460. doi: 10.1371/JOURNAL.PONE.0128460
Patki, G., Solanki, N., Atrooz, F., Allam, F., and Salim, S. (2013). Depression, anxiety-like behavior and memory impairment are associated with increased oxidative stress and inflammation in a rat model of social stress. Brain Res. 1539, 73–86. doi: 10.1016/j.brainres.2013.09.033
Picard, M., and McEwen, B. S. (2018). Psychological stress and mitochondria: a conceptual framework. Psychosom. Med. 80, 126–140. doi: 10.1097/PSY.0000000000000544
Piefke, T. J., Bonnell, T. R., DeOliveira, G. M., Border, S. E., and Dijkstra, P. D. (2021). Social network stability is impacted by removing a dominant male in replicate dominance hierarchies of a cichlid fish. Anim. Behav. 175, 7–20. doi: 10.1016/j.anbehav.2021.02.012
Quinlivan, E. P., and Gregory, J. F. (2008). DNA digestion to deoxyribonucleoside: a simplified one-step procedure. Anal. Biochem. 373, 383–385. doi: 10.1016/j.ab.2007.09.031
Rao, X., Zhong, J., Brook, R. D., and Rajagopalan, S. (2018). Effect of particulate matter air pollution on cardiovascular oxidative stress pathways. Antioxidants Redox Signal. 28, 797–818. doi: 10.1089/ARS.2017.7394
Rodriguez-Santiago, M., Nührenberg, P., Derry, J., Deussen, O., Francisco, F. A., Garrison, L. K., et al. (2020). Behavioral traits that define social dominance are the same that reduce social influence in a consensus task. Proc. Natl. Acad. Sci. USA 117, 18566–18573. doi: 10.1073/pnas.2000158117
Şahin, E., and Gümüşlü, S. (2004). Alterations in brain antioxidant status, protein oxidation and lipid peroxidation in response to different stress models. Behav. Brain Res. 155, 241–248. doi: 10.1016/j.bbr.2004.04.022
Salim, S. (2014). Oxidative stress and psychological disorders. Curr. Neuropharmacol. 12, 140–147. doi: 10.2174/1570159X11666131120230309
Sapolsky, R. M. (2004). Social status and health in humans and other animals. Annu. Rev. Anthropol. 33, 393–418. doi: 10.1146/annurev.anthro.33.070203.144000
Sapolsky, R. M. (2005). The influence of social hierarchy on primate health. Science 308, 648–652. doi: 10.1126/science.1106477
Sawecki, J., Miros, E., Border, S. E., and Dijkstra, P. D. (2019). Reproduction and maternal care increase oxidative stress in a mouthbrooding cichlid fish. Behav. Ecol. 30, 1662–1671. doi: 10.1093/beheco/arz133
Schiavone, S., Jaquet, V., Trabace, L., and Krause, K.-H. (2013). Severe life stress and oxidative stress in the brain: from animal models to human pathology. Antioxid. Redox Signal. 18, 1475–1490. doi: 10.1089/ars.2012.4720
Sharick, J. T., Vazquez-Medina, J. P., Ortiz, R. M., and Crocker, D. E. (2015). Oxidative stress is a potential cost of breeding in male and female northern elephant seals. Funct. Ecol. 29, 367–376. doi: 10.1111/1365-2435.12330
Snyder, B., Duong, P., Trieu, J., and Cunningham, R. L. (2018). Androgens modulate chronic intermittent hypoxia effects on brain and behavior. Horm. Behav. 106, 62–73. doi: 10.1016/j.yhbeh.2018.09.005
Snyder-Mackler, N., Burger, J. R., Gaydosh, L., Belsky, D. W., Noppert, G. A., Campos, F. A., et al. (2020). Social determinants of health and survival in humans and other animals. Science 368:eaax9553. doi: 10.1126/SCIENCE.AAX9553/ASSET/95FF5807-2989-42D4-A136-3EDB42814959/ASSETS/GRAPHIC/368_AAX9553_F4.JPEG
Spiers, J. G., Chen, H. J. C., Sernia, C., and Lavidis, N. A. (2015). Activation of the hypothalamic-pituitary-adrenal stress axis induces cellular oxidative stress. Front. Neurosci. 8, 1–6. doi: 10.3389/fnins.2014.00456
Suzuki, R., and Shimodaira, H. (2006). Pvclust: an R package for assessing the uncertainty in hierarchical clustering. Bioinformatics 22, 1540–1542. doi: 10.1093/bioinformatics/btl117
Tea, J., Alderman, S. L., and Gilmour, K. M. (2019). Social stress increases plasma cortisol and reduces forebrain cell proliferation in subordinate male zebrafish (Danio rerio). J. Exp. Biol. 222, 1–10. doi: 10.1242/jeb.194894
Tibbetts, E. A., Pardo-Sanchez, J., and Weise, C. (2022). The establishment and maintenance of dominance hierarchies. Philos. Trans. R. Soc. B Biol. Sci. 377:20200450. doi: 10.1098/rstb.2020.0450
Torres, D. J., Alfulaij, N., and Berry, M. J. (2021). Stress and the brain: an emerging role for selenium. Front. Neurosci. 15, 1–8. doi: 10.3389/fnins.2021.666601
Vicente-Gutierrez, C., Bonora, N., Bobo-Jimenez, V., Jimenez-Blasco, D., Lopez-Fabuel, I., Fernandez, E., et al. (2019). Astrocytic mitochondrial ROS modulate brain metabolism and mouse behaviour. Nat. Metab. 1, 201–211. doi: 10.1038/s42255-018-0031-6
Walton, N. M., Shin, R., Tajinda, K., Heusner, C. L., Kogan, J. H., Miyake, S., et al. (2012). Adult neurogenesis transiently generates oxidative stress. PLoS One 7:35264. doi: 10.1371/journal.pone.0035264
Weitekamp, C. A., Nguyen, J., and Hofmann, H. A. (2017). Neuromolecular regulation of aggression differs by social role during joint territory defense. Integr. Comp. Biol. 57, 631–639. doi: 10.1093/icb/icx0009
Keywords: oxidative stress, cichlid, social stress, reproduction, dominance hierarchies, antioxidant capacity, territoriality
Citation: Dijkstra PD, Fialkowski RJ, Bush B, Wong RY, Moore TI and Harvey AR (2024) Oxidative stress in the brain is regulated by social status in a highly social cichlid fish. Front. Behav. Neurosci. 18:1477984. doi: 10.3389/fnbeh.2024.1477984
Received: 08 August 2024; Accepted: 07 October 2024;
Published: 26 November 2024.
Edited by:
Raffaele d’Isa, San Raffaele Scientific Institute (IRCCS), ItalyReviewed by:
Eric Schuppe, University of California, San Francisco, United StatesCopyright © 2024 Dijkstra, Fialkowski, Bush, Wong, Moore and Harvey. This is an open-access article distributed under the terms of the Creative Commons Attribution License (CC BY). The use, distribution or reproduction in other forums is permitted, provided the original author(s) and the copyright owner(s) are credited and that the original publication in this journal is cited, in accordance with accepted academic practice. No use, distribution or reproduction is permitted which does not comply with these terms.
*Correspondence: Peter D. Dijkstra, ZGlqa3MxcEBjbWljaC5lZHU=
Disclaimer: All claims expressed in this article are solely those of the authors and do not necessarily represent those of their affiliated organizations, or those of the publisher, the editors and the reviewers. Any product that may be evaluated in this article or claim that may be made by its manufacturer is not guaranteed or endorsed by the publisher.
Research integrity at Frontiers
Learn more about the work of our research integrity team to safeguard the quality of each article we publish.