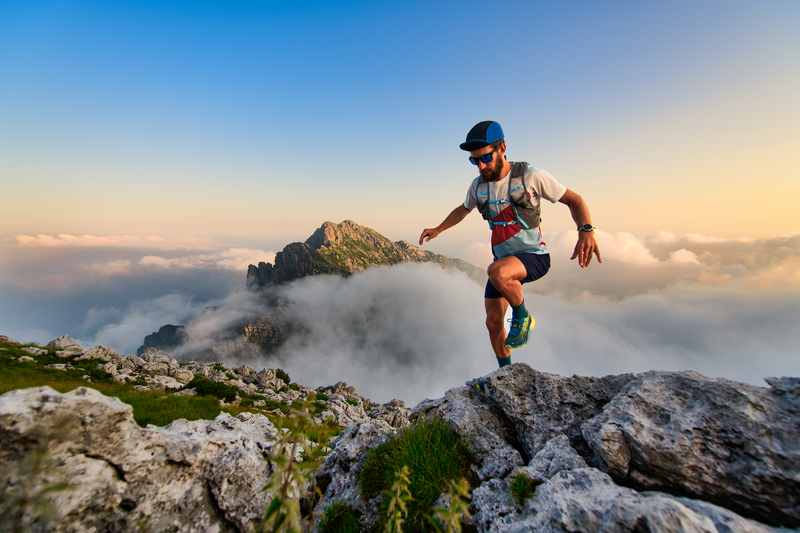
95% of researchers rate our articles as excellent or good
Learn more about the work of our research integrity team to safeguard the quality of each article we publish.
Find out more
ORIGINAL RESEARCH article
Front. Behav. Neurosci. , 06 September 2024
Sec. Motivation and Reward
Volume 18 - 2024 | https://doi.org/10.3389/fnbeh.2024.1458502
Introduction: Fragile X syndrome is an inherited X-linked disorder associated with intellectual disabilities that begin in childhood and last a lifetime. The symptoms overlap with autism spectrum disorder, and the syndrome predominantly affects males. Consequently, FXS research tends to favor analysis of social behaviors in males, leaving a gap in our understanding of other behavioral traits, especially in females.
Methods: We used a mouse model of FXS to analyze developmental, behavioral, neurochemical, and transcriptomic profiles in males and females.
Results: Our behavioral assays demonstrated locomotor hyperactivity, motor impulsivity, increased “approach” behavior in an approach-avoidance assay, and deficits in nest building behavior. Analysis of brain neurotransmitter content revealed deficits in striatal GABA, glutamate, and serotonin content. RNA sequencing of the ventral striatum unveiled expression changes associated with neurotransmission as well as motivation and substance use pathways. Sex differences were identified in nest building behavior, striatal neurotransmitter content, and ventral striatal gene expression.
Discussion: In summary, our study identified sex differences in specific behavioral, neurotransmitter, and gene expression phenotypes and gene set enrichment analysis identified significant enrichment of pathways associated with motivation and drug reward.
Fragile X syndrome (FXS) is an inherited pediatric rare disorder that causes a range of developmental disabilities, including delayed speech and language development, intellectual disability, anxiety, attention deficit, hyperactivity, as well as communication and social deficits (Hagerman et al., 2017). Physical features associated with FXS include hypotonia, flat feet, hyperextensible joints, and macroorchidism. The prevalence of FXS is higher in males than in females. It affects approximately 1:4,000 males and 1:8,000 females in the general population (Hunter et al., 2014).
FXS is the result of a mutation in the fragile x messenger ribonucleoprotein 1 (FMR1) gene. The mutation expands a DNA segment, known as the CGG triplet repeat in the 5′ untranslated region of FMR1 beyond its normal range of 5 to 40 repeats. The full mutation (>200 repeats) results in hypermethylation and silencing of the FMR1 gene and a complete loss or a significant downregulation of the fragile x messenger ribonucleoprotein (FMRP). FMRP is an RNA-binding protein that regulates translation of messenger RNA and serves critical functions in the developing and mature nervous system (Hagerman et al., 2017).
The Fmr1 knockout (KO) mouse was developed and characterized by Bakker et al. (1994). It shows changes in biochemical (Dahlhaus and El-Husseini, 2010), electrophysiological (Rais et al., 2018; Lovelace et al., 2018), neuropathological (Greco et al., 2011), and dendritic spine morphology (Irwin et al., 2000) features, many of which are consistent with FXS. Despite considerable variability in phenotypes (Paradee et al., 1999; Spencer et al., 2011) the Fmr1 KO mouse has contributed to major advances in our understanding of the behavioral, molecular, and synaptic changes associated with the loss of Fmr1 (Kazdoba et al., 2014; Yan et al., 2005; Hebert et al., 2014; Spencer et al., 2005; Yuskaitis et al., 2010; Rotschafer et al., 2012; Schaefer et al., 2017; Gurney et al., 2017; Gantois et al., 2017; Gomis-Gonzalez et al., 2016; Dolan et al., 2013; Jiang et al., 2022).
Traditionally FXS research has focused on men and boys because of the higher rates of prevalence and greater severity of symptoms in males (Hartley et al., 2011). However, FXS in females presents with a variety of challenges including a high frequency of avoidant behavior, shyness, mood disorders, and learning disabilities (Hartley et al., 2011; Murphy and Abbeduto, 2007). Moreover, FXS research tends to focus on cognitive and social behaviors because of symptom overlap between FXS and autism spectrum disorder (Smith et al., 2012; Kaufmann et al., 2004; Roberts et al., 2009; McDuffie et al., 2012; Niu et al., 2017). However, FXS is associated with changes in motivated behaviors including drug reward (Fish et al., 2013; Smith et al., 2014), although these behaviors traditionally have not been the focus of FXS research.
Since a side-by-side analysis of male and female Fmr1 KO mice could offer further insights into sex differences in the neurobiology of FXS, we examined behavioral, neurotransmitter, and changes in gene expression in both sexes of Fmr1 KO mice. For the gene expression analysis, we focused on the ventral striatum because it is at the crossroads of multiple behaviors associated with the limbic system, and ventral striatal transcriptome had not been analyzed previously in the Fmr1 KO mouse. Our data demonstrate significant sex-specific changes in behavioral and neurotransmitter phenotypes as well as gene expression in the ventral striatum.
Eight-week-old male and female C57BL/6J1 and Fmr1 KO2 mice were purchased from Jackson Laboratories (Bar Harbor, ME). The line was maintained by breeding hemizygous male Fmr1 mice with homozygous female Fmr1 mice. Wild-type (WT) mice of the same strain were used as controls, consistent with the design of experiments using this mouse model published previously (Kat et al., 2022). Five cohorts of mice were generated to provide sufficient numbers for all the analyses. Mice were maintained in a temperature-controlled environment with a 12 h/12 h light/dark cycle with food and water available ad libitum. The day of birth was designated P0. Litter size was recorded on P0, and entire litters were weighed on P0, P7, P14, and P21 for offspring body weight measurements. Mice were weaned on P21 per standard husbandry procedures. All the experimental procedures were approved by the Animal Care and Use Committee at Florida State University and were in full compliance with the NIH Guide for the Care and Use of Laboratory Animals.
Mice were monitored throughout the pre-weaning period for body weight, bilateral external ear detachment, body fur appearance, and eye-opening and tested for neuromotor skills such as righting reflex, cliff avoidance, and negative geotaxis. Analysis of body weight and physical features did not discriminate between males and females. For the assessment of neuromotor skills, male and female mice were analyzed separately, and sex was an independent variable. In all cases, 4–5 litters were used, and litter was used as “n” for statistical analyses.
Offspring were tested for righting reflex beginning at P2 by placing them in a supine position on a flat surface and measuring the time taken to “right” onto all four paws. This test was repeated daily, with one trial per day, until the mice met the criterion of righting within 3 s on two consecutive days.
Cliff avoidance reflex was tested beginning on P6 by placing the mouse on a flat Plexiglass surface raised to a height of 18 cm above the workbench. The pup was placed with its front paws and snout over the edge of the Plexiglass, and the time taken to turn 180° away from the cliff (edge) was recorded. The test was repeated daily until a latency of 6 s or less (criterion) was met on two consecutive days.
Negative geotaxis was assessed beginning on P6 by placing the pups head-downward on a 30° mesh incline and the time to turn 180° and reverse orientation to face upward was measured. Mice were tested once daily until a latency of 6 s or less (criterion) was reached for two consecutive days.
If the mice did not complete any of the neuromotor skill assessments within 30 s, or if they fell off the test apparatus at any time the tests were terminated, and the mice were assigned the full score of 30 s and returned to their home cages. The order of testing on each day was: Cliff avoidance, negative geotaxis, and righting reflex. A rest period of at least 15 min was used between tests (Cole et al., 2012).
Behavioral analyses were performed in adult (2–3-month-old) male and female WT and Fmr1 KO mice during the lights-off period when mice are naturally more active. A dim red light was used for ambient illumination. Mice from each genotype and sex were tested on each test day in parallel sessions. The experimenter was blinded to the identity of the mice. Each maze or test apparatus was cleaned with 1.6% Quatracide before testing and between trials to eliminate odor cues.
Mice were handled by the experimenter for at least 2 days, 2 min per day, for 1 week before the beginning of behavioral analyses. Prior to the commencement of each behavioral test, 30 min of habituation was used to permit acclimation to the testing room environment. The n used was 6–9 per genotype per sex for all behaviors except for nest building where the n was 7–15. When a mouse completed more than one behavioral test, we waited 1 week before administering the next behavioral test. Given the extensive battery of behavioral analyses performed, 3 cohorts of mice were used in total.
Locomotor activity was measured in individual testing chambers equipped with photobeam motion sensors (Photobeam Activity System; San Diego Instruments), which create a 3-dimensional grid (5.4 cm spacing) of infrared beams enveloping the entire cage (Zhu et al., 2017; McCarthy et al., 2018; McCarthy et al., 2020). As the mouse moved along the x- and y-axes, the number of breaks in the infrared beams was recorded. Each consecutive beam break was scored as an ambulatory event. The total ambulatory activity was analyzed over a period of 14 h which included an initial 2 h “lights-on” period (exploratory activity) and a subsequent 12 h “lights-off” period (spontaneous locomotor activity).
An elevated plus maze (EPM) with two open and two closed arms (50 cm × 10 cm each) with 40 cm high walls was used (Med Associates, Inc., St. Albans, VT). The time spent in the open and closed arms as well as the number of entries into the open and closed arms were recorded over a 5 min test period (Martin et al., 2020).
The test apparatus consisted of an exploration chamber and a test chamber separated by a sliding door. The test consisted of 3 sessions: habituation (day 1), training (day 2) and test (day 3). During the habituation session, each mouse was placed in the empty exploration and test chambers for 5 min each. During the training session, each mouse explored 5 uniquely shaped objects for 5 min in the exploration chamber. During the test session, each mouse interacted with one previously explored familiar object and one previously unexplored novel object in the test chamber for 3 min. A recognition index for the test session was calculated using the formula: TN ÷ (TF + TN) × 100, where TF = Time spent exploring the Familiar objects, and TN = Time spent exploring the Novel objects (McCarthy et al., 2018; McCarthy et al., 2020). We included in the analysis only those mice that spent at least 20 s with both objects during the test session.
A custom-built clear Plexiglass maze with 3 equal-sized arms arranged in the shape of the letter Y (Y-maze; each arm = 35 cm long × 6 cm wide × 10 cm high; McCarthy et al., 2018; McCarthy et al., 2020) was used. Unique visual cues were placed on the outer walls of each arm. A letter code (A, B, or C) was assigned to each arm. The mouse was placed at the end of a randomly chosen arm. During a 6 min test period, the sequence of arm entries, and the total number of arm entries were recorded. A “spontaneous alternation” is a set of 3 nonrepeating consecutive arm choices (e.g., ABC, BCA, CBA but not ABB, CCB, BAA, etc.). An alternation index (a measure of spatial working memory) was calculated as follows: number of alternations ÷ (number of entries − 2) × 100.
A cliff avoidance reflex (CAR) test was used (McCarthy et al., 2020; Zhang et al., 2018). Mice were placed at the center of a raised platform (20 cm in diameter) supported on a plastic rod (50 cm in height) resembling a barstool. The number of falls/jumps from the platform over 30 min was recorded for each mouse. If a mouse fell/jumped off the platform, it was gently picked up and returned to the center of the platform. A mouse that remained on the platform received a CAR score of 1 whereas a mouse that fell from the platform (regardless of the number of falls) received a score of 0.
The apparatus consisted of a Plexiglass tube approximately 17 inches long and 1 inch internal diameter (Maze Engineers, Skokie, IL; Spencer et al., 2005). The test included a 3-day training period during which the mice were habituated daily to the tube for 2 min. On test day one WT mouse and one Fmr1 KO mouse (age- and sex-matched) were placed headfirst at opposite ends of the tube and simultaneously released into the tube. Once both mice arrived at the center of the tube, the divider separating the mice was raised. The “match” lasted 2 min and ended when one mouse completely retreated from the tube. The mouse that remained in the tube is designated the winner (score = 1), and the mouse that retreated is the loser (score = 0). A match that lasted greater than 2 min was not scored, as 2 min was the cut-off time for this test. Each mouse competed in three matches in total.
Group-housed mice were removed from their cages and housed individually in new cages (home cage) for a week and then nest building was examined in the home cage (7.25″ W × 11.5″ D × 5″ H). Four days later, the test was repeated in a novel environment represented by a new, larger mouse breeder cage (10.5″ W × 19″ D × 6.25″ H) placed in a new room (Carreno-Munoz et al., 2018). During the tests, mice were provided with a single 5 cm2 condensed cotton nestlet, which the mice tore up to build nests. The quality and condition of the nests were scored on a 5-point scale at 30 min intervals for 5 h, as follows. Score 1: The nestlet material was essentially untouched (>95% intact) and no nest was built. Score 2: The nestlet was partially torn up (50–95% remaining intact) and no nest was built. Score 3: The majority of the nestlet (but <90%) was shredded and evidence of attempts at nest building could be seen. Score 4: >90% of the nestlet was torn up and the material was used to construct a nest, which was flat and not dome-shaped. Score 5: Most of the nestlet (>90%) was torn up and a perfect dome-shaped nest with a crater and a wall could be seen. The nest wall was taller than the mouse along >50% of the nest circumference. The test period of 5 h was chosen for home cage nesting because WT mice achieved the maximum nest score by 5 h. In the novel environment, WT mice required more time to achieve the maximum nest score, therefore, we monitored the mice for an additional 3 h (a total of 8 h).
Following the behavioral analyses, the mice were weighed, euthanized with isoflurane anesthetic overdose, decapitated, and the brains dissected from the skull. The dorsal and ventral striatum, prefrontal cortex, medial prefrontal cortex, and hippocampus were micro-dissected based on anatomical landmarks from both hemispheres (McCarthy et al., 2018). Samples from the 2 hemispheres were pooled, weighed, and immediately frozen with liquid nitrogen. Testes and adrenal glands were removed and weighed, and the weights were converted to percentages of body weight for each mouse. The n used was 4–7 per genotype per sex.
Tissue samples were shipped to the Neurochemistry Core at Vanderbilt University (Nashville, TN), where they were homogenized, and the protein concentration was estimated in each sample as previously described (McCarthy et al., 2018). Tissue concentrations (ng/mg protein) of dopamine, norepinephrine (NE), gamma-aminobutyric acid (GABA), glutamate, and serotonin were analyzed.
Samples of the ventral striatum were processed for RNA extraction as previously described (Qiagen RNeasy mini kit McCarthy et al., 2018). A next-generation sequencing library was prepared for each sample using the Illumina TruSeq stranded mRNA library kit. Libraries were barcoded for multiplexing with IDT for Illumina unique dual indexes. Each multiplexed library was sequenced on an Illumina NovaSeq 6000 as a 150 base pair paired-end sequencing run. Adapter trimming was performed as part of individual library demultiplexing. Quality Control analysis of each library was performed using fastQC.3 Illumina RNA-Seq Alignment (Version 2.0.2) was used, including STAR Aligner (Dobin et al., 2013) to align and map sequencing reads to the mouse genome (genome release UCSC mm10) and to generate read counts, which were used as a measure of abundance for differential gene expression analysis. DESeq2 (Love et al., 2014) was used to determine statistically significant differentially expressed genes (a false discovery rate, FDR, of <0.05 was used) and to generate a principal component analysis (PCA) plot. The analysis yielded a list of mRNAs present at significantly different levels between the WT and Fmr1 KO mice and provided a measure of confidence in each difference. Genes with a statistically significant differential expression were further analyzed by Kyoto Encyclopedia of Genes and Genomes (KEGG) pathway analysis and phenotype set enrichment analyses using Webgestalt (Zhang et al., 2005; Wang et al., 2013) to establish possible functional roles (Brown et al., 2015; Darkazalli et al., 2017; Jones et al., 2022; Vied et al., 2016; Vied et al., 2014). Benjamini–Hochberg procedure was used as the multiple test adjustment parameter against the mouse genome as a reference for enrichment and a p-value of <0.05 was considered significant. The heat map was generated using Morpheus (Broad Institute; https://software.broadinstitute.org/morpheus). All data are available in the NCBI Gene Expression Omnibus (Accession number GSE275170).4 Data was collected from four male and female WT and Fmr1 KO mice per genotype per sex. One outlier (Fmr1 KO Male 2) was excluded from the analysis based on the PCA generated during the DESeq2 analysis.
Tissue samples were processed for RNA extraction as previously described (Qiagen; RNeasy mini kit McCarthy et al., 2018). Reverse transcription reactions were performed using the SuperScript III cDNA synthesis kit (Thermo Fisher Scientific; 18080-044). Primer sequences for 18 s (Thermo Fischer Scientific, Hs Hs99999901_s1) and Fmr1 (Thermo Fischer Scientific, Mm01339582_m1) were chosen based on previously published data (McCarthy et al., 2018; Zhao and Usdin, 2014). Quantitative real-time PCR was performed in a StepOne Plus Thermocycler (Thermo Fisher Scientific) using Taqman PCR Master Mix (Thermo Fisher Scientific; 4369016) through 50 PCR cycles (95°C for 30 s, 57°C for 60 s, 72°C for 90 s). Fmr1 mRNA expression was normalized to 18 s using the Delta- Delta Ct method. Fold change was calculated for each sex separately using the WT values. The n used was 3 mice per genotype per sex.
From each homogenized striatal tissue sample, 30 μg protein was loaded on a 10% SDS-PAGE gel and the separated proteins were transferred to the PVDF membrane. The membranes were incubated with rabbit anti-FMRP monoclonal antibody (Cell Signaling Technology, #7104; 1:1,000; 80 kDa), and mouse β-tubulin antibody (loading control; Sigma Aldrich, #T8328; 1:5,000; 50 kDa). Immunoreactive bands were detected using goat anti-mouse IRDye 800 (Li-Cor, #926-32210; 1:20,000) and anti-rabbit IRDye 680 (Li-Cor, #926-68071; 1:20,000). The signals were captured using Odyssey CLx Imaging System (Li-Cor). The n used was 2 mice per genotype per sex.
A series of mixed model ANOVA followed by post hoc contrasts, where appropriate, using the Benjamini–Hochberg linear step up procedure to control for type I error were used to analyze developmental milestones, exploratory behavior (EPM and home cage activity) locomotor activity, nest building behavior, spatial working memory, and object-based attention (OBA). A binary logistic regression model was used to analyze the cliff avoidance reflex score. A negative binomial distribution was used to analyze the number of falls from the platform in the cliff avoidance reflex assay. Binomial logistic regression was used to analyze the tube test scores to determine whether scores were significantly different from an outcome expected by chance (50:50 win-lose outcome). A two-tailed student’s t-test was used to analyze testes weight where differences between only two groups were analyzed. Statistical analyses were performed using SAS/STAT 9.4 (SAS, Cary, NC). When a significant effect of genotype was observed without a significant effect of sex, data combined from male and female mice for each genotype (WT and KO) are shown in the Figures. Post-contrast analyses were performed only when a statistically significant interaction was found. Graphs were prepared using Prism 10.1.2 (GraphPad Prism, San Diego, CA).
Bodyweights at P0, P7, P14, and P21 were recorded and analyzed for entire litters without separating male and female offspring. The data did not reveal significant effects of genotype (F (1,27) = 0.64; p > 0.05), or genotype x age interaction (F (3,27) = 1.29; p > 0.05). However, there was a significant effect of age (F (4,27) = 159.62; p < 0.001) reflecting bodyweight gain during development in both Fmr1 KO and WT mice. The analyses of postnatal days on which the external ears detached, body fur appeared, or eyes opened did not show significant effects of genotype (student’s t-test, p > 0.05; Table 1).
There was no significant effect of genotype on righting reflex (F (1,12) = 0.53; p > 0.05), cliff avoidance (F (1,12) = 0.87, p > 0.05), or negative geotaxis (F (1,12) = 3.98, p > 0.05; Table 1 and Supplementary Figure S1). There was no significant effect of sex or sex x genotype interaction on any of the neuromotor skills (p > 0.05).
Locomotor activity was analyzed over a period of 14 h which included an initial 2 h “lights-on” period (exploratory activity) and a subsequent 12 h “lights-off” period (McCarthy et al., 2018; McCarthy et al., 2020). There was a significant effect of genotype during the initial 2 h lights-on period (i.e., exploratory activity; Figure 1A: asterisks; Fmr1 > WT; F (1,25) = 10.26; p < 0.01) and during the 12 h lights off period (spontaneous locomotor activity; Figure 1B; asterisks; Fmr1 > WT; F (1,25) = 27.56; p < 0.001). There was no significant effect of sex (exploratory activity: F (1,25) = 3.87; p > 0.05; spontaneous locomotor activity: F (1,25) = 1.24; p > 0.05) or sex x genotype interaction (exploratory activity: F (1,25) = 0.01; p > 0.05; spontaneous locomotor activity: F (1,25) = 0.27; p > 0.05). Thus, both male and female Fmr1 KO mice were more exploratory upon placement in the testing environment and were hyperactive in the familiar environment (Figures 1A,B).
Figure 1. Exploratory activity, spontaneous locomotor activity, and approach behavior in WT and Fmr1 KO mice. Fmr1 KO mice showed significant increases in locomotor activity during the lights-on period (A; exploratory activity in a novel environment) and the lights-off period (B; spontaneous locomotor activity) compared to WT mice. Fmr1 KO mice spent significantly more time in the open arms of the elevated plus maze (C; approach behavior) and made significantly greater number of entries into the open arms of the maze (D; exploration) compared to WT mice. A mixed model ANOVA showed a significant effect of genotype (asterisks) and no significant effect of sex or genotype x sex interaction Therefore, data from male and female mice from each genotype were combined to illustrate the differences between genotypes. **p < 0.01 and ****p < 0.0001, n = 6–9 per genotype per sex.
The elevated plus maze was used to test approach-avoidance behavior (Martin et al., 2020). Time spent in the open arms of the maze was taken to represent approach whereas time spent in the closed arms to represent avoidance. There was a significant effect of genotype on the time spent in the open arms of the maze (Figure 1C: asterisks; Fmr1 > WT; F (1,25) = 14.45; p < 0.001) and on the number of open arm entries (Figure 1D: asterisks; Fmr1 > WT; F (1,25) = 30.45; p < 0.001). There was no significant effect of sex (Time in open: F (1,25) = 0.08; p > 0.05; entries: F (1,25) = 2.14; p > 0.05) or genotype x sex interaction (Time in open: F (1,25) = 1.66; p > 0.05; entries: F (1,25) = 0.19; p > 0.05), suggesting impaired approach-avoidance balance in favor of approach and novelty seeking in both male and female Fmr1 KO mice.
A cliff avoidance reflex test was used to test motor impulsivity. There was a significant effect of genotype on cliff avoidance reflex score (Figure 2A; Fmr1 > WT; χ2 (1,37) = 7.03; p < 0.01). The Fmr1 KO mice were 10.7 times more likely to fall off the platform than WT mice. There was also a significant effect of genotype on the number of falls from the platform (Figure 2B; Fmr1 > WT; χ2 (1,37) = 7.41; p < 0.01). The effect of sex was not significant for either measure (score: χ2 (1,37) = 0.002; p > 0.05; falls: χ2 (1,37) = 0.05; p > 0.05) suggesting that motor impulsivity was observed in both male and female Fmr1 K0 mice.
Figure 2. Motor-impulsivity, social dominance, spatial working memory and object based attention in WT and Fmr1 KO mice. Fmr1 KO mice had a significantly lower cliff avoidance reflex score (A; binary logistic regression) and a significantly greater number of falls from the platform (B; negative binomial distribution) compared to WT mice. Fmr1 KO mice had significantly fewer wins compared to WT mice in the tube test of social dominance (C; binomial logistic regression). Significant effects of genotype (asterisks) were found for all three measures (A–C), but the effect of sex was not significant. Spontaneous alternation index, which is a measure of spatial working memory (D); the number of arm entries in the Y-maze (measure of maze exploration; E), or recognition index (unit of measure for attention in the object-based attention task; F) did not show significant effects of genotype, sex of genotype x sex interaction. Since genotype produced significant effects on some behaviors and sex did not produce significant effects on any measures, data from male and female mice from each genotype were combined to illustrate the differences between genotypes. **p < 0.01; n = 6–9 per genotype per sex.
A tube test was used to examine social dominance. Fmr1 KO mice won significantly fewer matches against sex and age-matched WT mice (Figure 2C; Fmr1 < WT; χ2 (1,78) = 3.02; p < 0.01). The Fmr1 KO mice were 14.3 times more likely to lose a match than WT mice. The effect of sex was not significant (χ2 (1,78) = 0.80; p > 0.05) indicating that Fmr1 KO mice, regardless of sex had a deficit in social dominance behavior.
Nest building was examined in the home environment and 4 days later, the test was repeated in a novel environment. We found a significant effect of genotype on the nest building score in the home cage (Figure 3B; Fmr1 KO < WT: F (1,20) = 7.54; p < 0.05). There was no significant effect of sex (F (1,18) = 1.93; p > 0.05) or sex x genotype interaction (F (1,18) = 0.77; p > 0.05) in the home environment. In the novel environment, there was a significant effect of genotype (Figure 3C; Fmr1 KO < WT: F (1,20) = 25.07; p < 0.0001), sex (female < male: F (1,18) = 61.65; p < 0.0001) and genotype x sex interaction (F (1,18) = 17.73; p < 0.001) on nest building score.
Figure 3. Nest building deficit in male and female Fmr1 KO mice in the home and novel environments. Representative images of nests built by WT and Fmr1 KO mice (A; novel environment). The Fmr1 KO mice received significantly lower nest scores compared to the WT mice in the home (B) and the novel environment (C). A mixed model ANOVA showed a significant effect of genotype under both conditions. Sex differences were present only in the novel environment. Since genotype, sex, and sex x genotype showed significant effects, data from male and female mice are shown separately for each genotype. n = 7–15 per genotype per sex.
Thus, there were significant effects of genotype on the nest building score in the home and the novel environments (Fmr1 KO < WT). Sex differences (female < male) and sex x genotype interaction were present only in the novel environment.
An example of a fully formed nest, which was observed typically in WT mice in the novel environment at the end of the 5 h. observation period is shown alongside a flat, poorly formed nest observed typically in Fmr1 KO mice housed in the novel environment at the end of the 5 h. period (Figure 3A).
A Y-maze was used to test spatial working memory, and the spontaneous alternation index was the test parameter (McCarthy et al., 2018; McCarthy et al., 2020). There was no significant effect of genotype (F (1,25) = 0.31; p > 0.05), sex (F (1,25) = 3.48; p > 0.05), or genotype x sex interaction (F (1,25) = 0.02; p > 0.05) on spontaneous alternations, suggesting that neither male nor female mice showed significant deficits in spatial working memory (Figure 2D). Similarly, the total number of arm entries did not show significant effects of genotype (F (1,25) = 3.17; p > 0.05), sex (F (1,25) = 1.42; p > 0.05) or genotype x sex interaction (F (1,25) = 0.01; p > 0.05; Figure 2E), suggesting that mice in all groups showed comparable maze exploration.
We used the object-based attention test (OBA) to evaluate attention (McCarthy et al., 2018; McCarthy et al., 2020). There was no significant effect of genotype (F (1,24) = 0.39; p > 0.05), sex (F (1,24) = 0.02; p > 0.05), or genotype x sex interaction (F (1,24) = 3.32; p > 0.05) on recognition index, a measure of attention, suggesting lack of differences in object-based attention among the different groups (Figure 2F).
Following the behavioral analyses, neurotransmitter content in the prefrontal cortex, medial prefrontal cortex, hippocampus, and dorsal striatum was analyzed. Striatal GABA tissue concentration showed a significant effect of sex (Table 2; male < female) and significant genotype x sex interaction (Table 2). Genotype did not produce significant effects (Table 2). Post hoc contrast analysis showed a significant decrease in striatal GABA tissue concentration in Fmr1 KO males compared to WT males (t(1,11) = −2.97, p < 0.05; Table 2). Tissue concentrations of GABA in the other brain regions did not show significant effects of genotype, sex, or genotype x sex interaction (Table 2).
Changes in striatal glutamate tissue concentration showed the same pattern as that of GABA. There was a significant effect of sex (male < female) and a significant genotype x sex interaction (Table 2). Genotype did not produce significant effects. Post hoc contrast analysis showed a significant decrease in striatal glutamate tissue concentration in Fmr1 KO males compared to WT males (t(1,11) = −3.81, p < 0.01; Table 2). Tissue concentrations of glutamate in the other brain regions did not produce significant effects of genotype, sex, or genotype x sex interaction (Table 2).
Striatal serotonin tissue concentration showed significant effects of genotype (Table 2; Fmr1 KO < WT), and no significant effect of sex or genotype x sex interaction (Table 2). Tissue concentrations of serotonin in the other brain regions did not show significant effects of genotype, sex, or genotype x sex interaction (Table 2).
Dopamine tissue concentration showed significant effects of sex in the striatum and the hippocampus (male < female; Table 2). The effects of genotype or genotype x sex interaction were not significant in the striatum or the hippocampus. The prefrontal cortex or the medial prefrontal cortex did not show significant effects of genotype, sex, or genotype x sex interaction (Table 2).
Noradrenaline tissue concentration showed a significant effect of sex in the medial prefrontal cortex and the hippocampus (male < female; Table 2). The effects of sex or genotype x sex interaction were not significant in the medial prefrontal cortex and the hippocampus. The striatum or the prefrontal cortex did not show significant effects of genotype, sex, or genotype x sex interaction (Table 2).
In summary, striatal GABA and glutamate content was reduced significantly in male Fmr1 KO mice compare to wild type males. Striatal serotonin showed a significant effect of genotype, with lower concentrations in Fmr1 KO mice. Dopamine tissue concentration showed significant effects of sex in the striatum and hippocampus, with lower concentrations in males compared to females regardless of genotype. Similarly, noradrenaline tissue concentration showed a significant effect of sex in the prefrontal cortex and the hippocampus, with lower concentrations in males compared to females.
Since males with fragile X show macroorchidism and increases in adrenal weights (Hagerman et al., 2017; Bhattacharya and Klann, 2012; Qin et al., 2011), we analyzed both these parameters in male and female mice. There was a significant increase in testes weight in Fmr1 KO mice compared to WT counterparts (t = 3.245, df = 10; p < 0.01). Adrenal weights showed a significant effect of genotype (Fmr1 KO > WT: F (1,12) = 13.14; p < 0.001;) and sex (female > male: F (1,12) = 1.42; p < 0.05;) but no significant genotype x sex interaction (F (1,12) = 3.88, p > 0.05).
Our behavioral and neurotransmitter analyses covered a broad range of functional (behavioral) and structural (brain regions for neurotransmitter tissue content analysis) domains. Since the nucleus accumbens of the ventral striatum is at the crossroads of multiple limbic circuits, we reasoned that gene expression in the ventral striatum could offer valuable insights into changes in a broad array of functional domains. Moreover, analysis of gene expression in the brain’s limbic centers such as the ventral striatum had not been undertaken in the Fmr1 KO mouse model, despite reports of changes in motivation and reward mechanisms in FXS (Smith et al., 2014; Huebschman et al., 2021).
We identified 1,234 differentially expressed genes (DEGs) in the Fmr1 KO mice relative to WT, with 576 genes upregulated and 658 genes downregulated. When we analyzed the data separately for male and female mice, 809 DEGs were found in males, with 375 genes upregulated and 434 genes downregulated. In female mice, we found 347 DEGs, with 119 genes upregulated and 228 genes downregulated (Table 3).
As anticipated, Fmr1 was the top downregulated DEG in both male and female mice. These findings were validated by qPCR analysis of Fmr1 mRNA expression in the striatum, which showed very low but detectable levels in the Fmr1 KO mice compared to WT mice (Supplementary Figure S2A). Western blot showed that FMRP was not detectable at all in either male or female Fmr1 KO mouse striatum (Supplementary Figure S2B). WT male and female mice showed robust Fmr1 mRNA and FMRP expression in the striatum.
The KEGG enrichment analysis of the 1,234 DEGs (Figure 4A) revealed 40 significantly enriched pathways. The vast majority (75%) of the total number of DEGs contributed to only 4 categories namely, nervous system, substance dependence, signal transduction, and endocrine system. Among the nervous system associated pathways, neurotransmission (e.g., glutamatergic synapse, GABAergic synapse, cholinergic synapse, dopaminergic synapse, serotonergic synapse, norepinephrine signaling) was prominently represented.
Figure 4. Transcriptome profiling of the ventral striatum by RNA sequencing in WT and Fmr1 KO mice. KEGG gene set enrichment analysis of 1,243 differentially expressed genes (DEGs) in WT and Fmr1 KO mice (data from males and females were combined) identified 40 significantly enriched pathways (A). The pathways fall into 4 main categories (unique symbol for each pathway in A) namely, the nervous system (*); substance dependence (bold font) signal transduction (#), and the endocrine system (α). Heat map of normalized expression values of DEGs for male and female WT (male n = 4, female n = 4) and Fmr1 KO (male n = 3, female n = 4) (columns) in each of the 5 substance dependence categories namely, cocaine addiction (B), alcoholism (C), amphetamine addiction (D), morphine addiction (E), and nicotine addiction (F). The dark blue to dark red gradient represents gradient of minimum to maximum expression, respectively (B–F).
Consistent with the KEGG pathway analysis, phenotype set enrichment analysis of the 1,234 DEGs identified drug reward pathways as being significantly enriched. Among the enriched reward pathways, conditioned place preference and behavioral response to morphine were at the very top of the list. In addition, multiple pathways associated with neurotransmission (e.g., synaptic transmission, and increased susceptibility to neuronal excitotoxicity, abnormal glutamate-mediated receptor currents, and abnormal excitatory postsynaptic currents pathways) were identified as well (Supplementary Figure S3).
Evaluation of gene expression in the cocaine addiction, alcoholism, and amphetamine addiction pathways for male and female WT and Fmr1 KO mice revealed that approximately 30% of the DEGs were upregulated and 70% were downregulated in the Fmr1 KO mice (Figures 4B–D). Among the downregulated DEGs Pdyn (prodynorphin), Adyc5 (adenylate cyclase 5), Ppp1r1b (protein phosphatase 1 regulatory inhibitor subunit 1B) and Drd1 (dopamine receptor D1) were common to all 3 pathways. Among the upregulated DEGs, Creb5 (cAMP responsive element binding protein 5) and Creb3l3 (cAMP responsive element binding protein 3 like 3) were common to all 3 pathways (Figures 4B–D). Evaluation of gene expression in the nicotine addiction pathway revealed gene expression changes that were opposite to those in the other 3 pathways (Figure 4F). Specifically, approximately 30% of the DEGS were downregulated and 70% were upregulated in the nicotine addiction pathway. In the morphine addiction pathway, approximately equal proportion (50%) of the DEGs were upregulated and downregulated (Figure 4E).
Our data show that Fmr1 KO mice show significant changes in locomotor activity, approach-avoidance behavior, motor impulsivity, and nest building behavior. Striatal GABA, glutamate and serotonin tissue concentrations are significantly decreased in the Fmr1 KO mice. RNA sequencing of the ventral striatum identified 1,234 DEGs in the Fmr1 KO mice relative to WT mice. Sex differences were evident in nest building behavior, striatal neurotransmitter content, and the number of differentially expressed genes in the ventral striatum.
There are significant genotypic differences between humans with FXS and the Fmr1 KO mouse model used here, especially between males and females. In FXS, the affected males carry the CGG expansion in the mutant FMR1 gene on the X chromosome and lack a “normal” copy of FMR1. FXS carrier females have a mutant and a normal FMR1 allele each on their X chromosomes. Although rare, women may carry two copies of the mutant FMR1 gene (one on each X chromosome) (Vafaeie et al., 2021). In the Fmr1 KO mouse model used here, the Fmr1 gene is deleted in both males and females. We show that Fmr1 mRNA expression (Supplementary Figure S2A) was virtually absent (only low levels were expressed) (Yan et al., 2004) and FMRP expression was undetectable in the striatum (Supplementary Figure S2B) in both sexes of the Fmr1 KO mouse. Thus, genotype differences between humans with FXS and the Fmr1 KO mouse should be considered while extrapolating data from the Fmr1 KO mouse to humans with FXS.
Our findings from the behavioral assays are largely consistent with previous reports that used the Fmr1 KO mouse model used here. For example, our findings of increased spontaneous exploratory activity and locomotor activity in the Fmr1 KO mice are consistent with findings from multiple previous studies, although the methods used for the analysis differed among the studies (Bakker et al., 1994; Dahlhaus and El-Husseini, 2010; Spencer et al., 2005; Peier et al., 2000; Pietropaolo et al., 2011; Ding et al., 2014; Gholizadeh et al., 2014; Uutela et al., 2014; Mineur et al., 2002; Liu et al., 2011; Longo et al., 2023).
The elevated plus maze assay revealed significant increases in time spent in the open arms and the number of entries into the open arms in the Fmr1 KO mice, consistent with findings from previous reports (Arsenault et al., 2016; Dansie et al., 2013; Sare et al., 2016). We interpret this outcome as evidence of increased approach behavior (Buck et al., 2019), suggestive of novelty-seeking and risk-taking (Cloninger, 1986; Hansson et al., 2012; Kelley et al., 2004; Wang et al., 2015). Others interpreted these outcomes as evidence of reduced anxiety (Hagerman et al., 2017; Spencer et al., 2005; Schaefer et al., 2017). Further studies would be necessary to discriminate between approach-avoidance and anxiety-like behaviors in the Fmr1 KO mice.
The cliff avoidance reflex assay revealed motor impulsivity in the Fmr1 KO mice consistent with previous reports in the literature (Moon et al., 2006). The Fmr1 KO mice were 10 times more likely to fall from the platform compared to the WT mice, as indicated by their poor cliff avoidance reflex score. It is possible that the increased number of falls are the result of the increased spontaneous locomotor activity, exploratory activity or approach behavior in the Fmr1 KO mice rather than the result of motor impulsivity per se. Our data do not discriminate between these possibilities fully. However, the Fmr1 KO mice did not show increased arm entries in the Y-maze (Figure 2E) nor did they show increased locomotor activity during nest building in the novel environment (Supplementary Figure S4). In other words, the increased locomotor activity, exploratory activity, or approach behavior did not transfer to these behavioral assays. Therefore, we suggest that the outcome from the cliff avoidance reflex assay reflects motor impulsivity in the Fmr1 KO mice.
Individuals with FXS are often diagnosed with social phobia and social interaction deficits and some of these behaviors have been recapitulated in the Fmr1 KO mouse model, although findings from the different studies are variable (Dahlhaus and El-Husseini, 2010; Spencer et al., 2005; Pietropaolo et al., 2011; Mineur et al., 2002; Liu et al., 2011; McNaughton et al., 2008). We used the tube test of social dominance, which has been used to study social hierarchy in mice (Fan et al., 2019). We found that Fmr1 KO mice lost significantly more matches when paired against age- and sex-matched WT partners in the tube test and were 14 times more likely to lose a match regardless of sex, suggesting a significant impairment of social dominance. These findings are consistent with previous reports of impaired social dominance in the Fmr1 KO mice (Spencer et al., 2005; Pacey et al., 2011).
We found significant deficits in nest building behavior in the Fmr1 KO mice in the home as well as novel environments, consistent with findings from previous studies (Gurney et al., 2017; Carreno-Munoz et al., 2018; Gross et al., 2019; Gross et al., 2015). Nest building is a motivated behavior displayed by both sexes of multiple species. Poor quality of the nest or delay in building a fully formed nest reflect deficits in motivated behaviors and arousal-anxiety disequilibrium (Jacobson et al., 2020). The environment of the novel cage introduces an element of anxiety, which the mice must overcome to build the nest. Limbic circuits involving the hippocampus and ventral striatum, which influence motivated behaviors as well as emotional behaviors including anxiety, play a role in the regulation of nest building behavior (Deacon et al., 2002; Luo et al., 2018). The specific contributions of motivation, reward, and anxiety-arousal to nest building are difficult to distinguish and the contribution of each to the overall deficit in nest building in the Fmr1 KO mouse is difficult to establish using the nest building assay alone. Additional studies will be necessary to address these issues.
The poor nest building and the increased “approach” behavior (or reduced anxiety-like behavior) exhibited by the Fmr1 KO mice in the elevated plus maze assay could have common origins. The nest building behavior likely reflects intrinsic motivation to create a place of safety, warmth and protection. If one conceded the speculative interpretation that the poor nest building by the Fmr1 KO mice reflects “disregard” for safety, then such behavior would be consistent with the increased “approach” behavior in the elevated plus maze. We emphasize that additional research would be needed to test the validity of this suggestion.
We did not find significant differences between Fmr1 KO and WT mice in spatial working memory or object-based attention. There are reports of significant deficits in working memory and attention in the Fmr1 KO mice, although a consensus is yet to emerge (review in Kazdoba et al., 2014). Methodological differences may contribute to the variability in outcomes.
A principal goal of the present study was an investigation of sex differences in the behavioral traits in the Fmr1 KO mouse model. Selective analysis of male mice is frequent in FXS research (Spencer et al., 2005; Arsenault et al., 2016; Dansie et al., 2013; Sare et al., 2016; Pacey et al., 2011). However, when both sexes of mice are examined, as in the present study, lack of sex differences in behavioral parameters is a frequent finding (Bakker et al., 1994; Ding et al., 2014; Gholizadeh et al., 2014; Gauducheau et al., 2017; Schmitt et al., 2022). Consistent with this observation, a comparison between male and female mice showed that most of the behavioral traits analyzed here did not show a sex difference. Nest building in the novel environment was the only behavior that showed significant effects of sex x genotype. The nest building deficits in the novel environment were more pronounced and emerged earlier in the task in male Fmr1 KO mice compared to females. Nest building deficits in male Fmr1 KO mice were reported by others, although these reports did not study female mice (Gurney et al., 2017; Carreno-Munoz et al., 2018; Gross et al., 2019; Gross et al., 2015).
Analysis of neurotransmitter content showed significant deficits in striatal GABA and glutamate content in male Fmr1 KO mice, and deficits in serotonin content in male and female Fmr1 KO mice demonstrating significant sex differences. Previous studies reported an overall reduction in GABA tone in FXS patients as well as Fmr1 KO mice based on analysis of GABA synthesis, release, and GABA receptor signaling mechanism (for review see Paluszkiewicz et al., 2011; Dionne and Corbin, 2021). Reduced glutamatergic neurotransmission has not been reported in FXS patients. However, metabotropic glutamate receptor antagonists produce behavioral improvements in Fmr1 KO mouse models (Yan et al., 2005; Xu et al., 2012; Osterweil et al., 2010), which implies increased rather than decreased glutamatergic tone in the Fmr1 KO mouse brain. In some patients with FXS, lack of FMRP may affect serotonin-mediated pathways (Hanson and Hagerman, 2014; Protic et al., 2019) and serotonin receptor agonists and selective serotonin reuptake inhibitors have been shown to ameliorate behavioral deficits in the Fmr1 KO mouse (Uutela et al., 2014; Costa et al., 2018; Tao et al., 2023; Kim et al., 2022). The deficits in striatal glutamate, GABA and serotonin likely contribute to the behavioral changes observed in the Fmr1 KO mice, although a correlation with each behavioral trait is difficult to establish. Further study to characterize potential changes in pharmacological targets of neurotransmission such as receptor signaling, neurotransmitter synthesis and re-uptake could offer direct translational implications for FXS.
KEGG and phenotype gene set enrichment analyses identified signal transduction, nervous system, and endocrine system categories were significantly enriched in the Fmr1 KO mouse ventral striatum (Figure 4). Consistent with data from the tissue neurotransmitter content assays, neurotransmission (e.g., glutamatergic synapse, GABAergic synapse, cholinergic synapse, dopaminergic synapse, serotonergic synapse, norepinephrine signaling) category was prominently represented among the enriched pathways.
Gene set enrichment analysis further highlighted substance dependence and drug reinforcement as significantly enriched pathways in the Fmr1 KO ventral striatum. Cocaine, amphetamine, nicotine, morphine, and alcohol addiction pathways were among the significantly enriched. Downregulated DEGs such as Pdyn, Ppp1r1b, Drd1, and Adcy5, which are known to be associated with reward and addiction (Berman et al., 2014; Kim et al., 2006; Dichter et al., 2012; Baik, 2013; McLaughlin et al., 2003; Galeote et al., 2009) were found in the cocaine, alcohol, amphetamine, and morphine addiction pathways in the Fmr1 KO ventral striatum. Previous reports showed that Adcy5 KO mice have reduced reward response to morphine, whereas Ppp1r1b (commonly referred to as Darpp32) KO mice have reduced responses to cocaine in conditioned place preference test (Kim et al., 2006; Zachariou et al., 2002).
Other reports in the literature demonstrate reduced cocaine reward, behavioral sensitization, and cocaine-seeking behavior in Fmr1 KO mice (Smith et al., 2014; Huebschman et al., 2021). These behavioral changes are associated with increased dendritic spine density and synaptic strength in the nucleus accumbens (Smith et al., 2014) a major constituent of the ventral striatum. A recent study showed that Fmr1 mRNA is reduced in leukocytes of patients diagnosed with alcohol or drug dependence in comparison to healthy controls (Krasteva et al., 2020), further demonstrating a potential link between Fmr1 and drug dependence. The present study offers evidence from gene set enrichment analysis that compromised motivation and drug reward mechanisms are likely an intrinsic property in the Fmr1 KO mouse. We did not seek direct evidence of compromised motivation or drug reinforcement in the Fmr1 KO mice.
Finally, we did not observe significant differences in litter size or the age of acquisition of developmental milestones in the Fmr1 KO offspring (Table 1). There were no significant differences in body weight at any time during development (Table 1) or in adulthood between the Fmr1 KO and WT mice. Thus, in the present study, the mouse model did not recapitulate findings of developmental delays in FXS nor did they support the observation that children and adolescents with FXS are heavier than age-matched controls (McLennan et al., 2011). We found that the testes and adrenal gland weights (as a percentage of total body weight) were increased in the Fmr1 KO mice, consistent with previous reports (Hagerman et al., 2017; Bhattacharya and Klann, 2012; Qin et al., 2011). Both male and female Fmr1 KO mice had increased adrenal gland weights compared to WT mice. Incidentally, females had greater adrenal gland weights than males regardless of genotype (Bielohuby et al., 2007).
In summary, our findings on behavioral traits in the Fmr1 KO mouse agree with those from earlier studies. We identified significant deficits in striatal GABA, glutamate and serotonin content in the Fmr1 KO mouse and significant changes in gene expression in the Fmr1 KO mouse ventral striatum. Our methodologies do not permit us to draw direct relationships among the changes observed in the different parameters, although a general relationship between deficits in striatal neurotransmitter content, enrichment of the neurotransmission category in gene set enrichment pathways and behaviors such as locomotor hyperactivity, approach behavior, motor impulsivity, social dominance and nest building appears plausible. The enrichment of pathways of motivation and drug reward in the ventral striatal gene set enrichment analysis offers novel perspectives on compromised motivation and reward mechanisms in the Fmr1 KO mouse. Although these findings do not offer insights into the direction of the change (up- or down-regulation), previous reports (Smith et al., 2014; Huebschman et al., 2021) suggest downregulation of motivation and reward processing in FXS, consistent with current ideas of dysfunction in these mechanisms in autism spectrum disorder (Keifer et al., 2021). Finally, sex differences in nest building behavior in novel environments, striatal neurotransmitter content, and gene expression in the ventral striatum highlight the significance of including male and female Fmr1 KO mice in FXS research.
The data presented in the study are deposited in the NCBI Gene Expression Omnibus repository, https://www.ncbi.nlm.nih.gov/geo/query/acc.cgi?acc=GSE275170; accession number GSE275170.
The animal study was approved by Florida State University Institutional Animal Care and Use Committee. The study was conducted in accordance with the local legislation and institutional requirements.
DM: Conceptualization, Data curation, Formal analysis, Funding acquisition, Investigation, Methodology, Project administration, Resources, Software, Supervision, Validation, Visualization, Writing – original draft, Writing – review & editing. CV: Conceptualization, Data curation, Formal analysis, Investigation, Methodology, Software, Validation, Writing – original draft, Writing – review & editing. MT: Writing – review & editing, Conceptualization, Data curation, Investigation, Methodology. AC: Conceptualization, Data curation, Formal analysis, Investigation, Methodology, Writing – review & editing. YW: Conceptualization, Investigation, Methodology, Writing – review & editing. CS: Data curation, Formal analysis, Investigation, Software, Validation, Writing – review & editing. PB: Conceptualization, Data curation, Formal analysis, Funding acquisition, Investigation, Methodology, Project administration, Resources, Software, Supervision, Validation, Visualization, Writing – original draft, Writing – review & editing.
The author(s) declare that financial support was received for the research, authorship, and/or publication of this article. This work was supported by The Jim and Betty Ann Rodgers Chair Fund (F00662) and The Council on Research and Creativity at Florida State University (046227).
The authors declare that the research was conducted in the absence of any commercial or financial relationships that could be construed as a potential conflict of interest.
All claims expressed in this article are solely those of the authors and do not necessarily represent those of their affiliated organizations, or those of the publisher, the editors and the reviewers. Any product that may be evaluated in this article, or claim that may be made by its manufacturer, is not guaranteed or endorsed by the publisher.
The Supplementary material for this article can be found online at: https://www.frontiersin.org/articles/10.3389/fnbeh.2024.1458502/full#supplementary-material
1. ^https://www.jax.org/strain/000664
2. ^https://www.jax.org/strain/003025
3. ^http://www.bioinformatics.babraham.ac.uk/projects/fastqc/
Arsenault, J., Gholizadeh, S., Niibori, Y., Pacey, L. K., Halder, S. K., Koxhioni, E., et al. (2016). FMRP expression levels in mouse central nervous system neurons determine behavioral phenotype. Hum. Gene Ther. 27, 982–996. doi: 10.1089/hum.2016.090
Baik, J. H. (2013). Dopamine signaling in reward-related behaviors. Front. Neural Circuits 7:152. doi: 10.3389/fncir.2013.00152
Bakker, C., Verheij, C., Willemsen, R., Vanderhelm, R., Oerlemans, F., Vermey, M., et al. (1994). Fmr1 knockout mice: a model to study fragile X mental retardation. Cell 78, 23–33. doi: 10.1016/0092-8674(94)90569-X
Berman, R. F., Buijsen, R. A., Usdin, K., Pintado, E., Kooy, F., Pretto, D., et al. (2014). Mouse models of the fragile X premutation and fragile X-associated tremor/ataxia syndrome. J. Neurodev. Disord. 6:25. doi: 10.1186/1866-1955-6-25
Bhattacharya, A., and Klann, E. (2012). Fragile X syndrome therapeutics S(C)TEP through the developmental window. Neuron 74, 1–3. doi: 10.1016/j.neuron.2012.03.014
Bielohuby, M., Herbach, N., Wanke, R., Maser-Gluth, C., Beuschlein, F., Wolf, E., et al. (2007). Growth analysis of the mouse adrenal gland from weaning to adulthood: time- and gender-dependent alterations of cell size and number in the cortical compartment. Am. J. Physiol. Endocrinol. Metab. 293, E139–E146. doi: 10.1152/ajpendo.00705.2006
Brown, A. N., Vied, C., Dennis, J. H., and Bhide, P. G. (2015). Nucleosome repositioning: a novel mechanism for nicotine- and cocaine-induced epigenetic changes. PLoS One 10:e0139103. doi: 10.1371/journal.pone.0139103
Buck, J. M., Sanders, K. N., Wageman, C. R., Knopik, V. S., Stitzel, J. A., and O’Neill, H. C. (2019). Developmental nicotine exposure precipitates multigenerational maternal transmission of nicotine preference and ADHD-like behavioral, rhythmometric, neuropharmacological, and epigenetic anomalies in adolescent mice. Neuropharmacology 149, 66–82. doi: 10.1016/j.neuropharm.2019.02.006
Carreno-Munoz, M. I., Martins, F., Medrano, M. C., Aloisi, E., Pietropaolo, S., Dechaud, C., et al. (2018). Potential involvement of impaired BKCa channel function in sensory defensiveness and some behavioral disturbances induced by unfamiliar environment in a mouse model of fragile X syndrome. Neuropsychopharmacology 43, 492–502. doi: 10.1038/npp.2017.149
Cloninger, C. R. (1986). A unified biosocial theory of personality and its role in the development of anxiety states. Psychiatr. Dev. 4, 167–226.
Cole, T. B., Fisher, J. C., Burbacher, T. M., Costa, L. G., and Furlong, C. E. (2012). Neurobehavioral assessment of mice following repeated postnatal exposure to chlorpyrifos-oxon. Neurotoxicol. Teratol. 34, 311–322. doi: 10.1016/j.ntt.2012.02.003
Costa, L., Sardone, L. M., Bonaccorso, C. M., D’Antoni, S., Spatuzza, M., Gulisano, W., et al. (2018). Activation of serotonin 5-HT7 receptors modulates hippocampal synaptic plasticity by stimulation of adenylate cyclases and rescues learning and behavior in a mouse model of fragile X syndrome. Front. Mol. Neurosci. 11:353. doi: 10.3389/fnmol.2018.00353
Dahlhaus, R., and El-Husseini, A. (2010). Altered neuroligin expression is involved in social deficits in a mouse model of the fragile X syndrome. Behav. Brain Res. 208, 96–105. doi: 10.1016/j.bbr.2009.11.019
Dansie, L. E., Phommahaxay, K., Okusanya, A. G., Uwadia, J., Huang, M., Rotschafer, S. E., et al. (2013). Long-lasting effects of minocycline on behavior in young but not adult fragile X mice. Neuroscience 246, 186–198. doi: 10.1016/j.neuroscience.2013.04.058
Darkazalli, A., Vied, C., Badger, C. D., and Levenson, C. W. (2017). Human mesenchymal stem cell treatment normalizes cortical gene expression after traumatic brain injury. J. Neurotrauma 34, 204–212. doi: 10.1089/neu.2015.4322
Deacon, R. M., Croucher, A., and Rawlins, J. N. (2002). Hippocampal cytotoxic lesion effects on species-typical behaviours in mice. Behav. Brain Res. 132, 203–213. doi: 10.1016/S0166-4328(01)00401-6
Dichter, G. S., Damiano, C. A., and Allen, J. A. (2012). Reward circuitry dysfunction in psychiatric and neurodevelopmental disorders and genetic syndromes: animal models and clinical findings. J. Neurodev. Disord. 4:19. doi: 10.1186/1866-1955-4-19
Ding, Q., Sethna, F., and Wang, H. (2014). Behavioral analysis of male and female Fmr1 knockout mice on C57BL/6 background. Behav. Brain Res. 271, 72–78. doi: 10.1016/j.bbr.2014.05.046
Dionne, O., and Corbin, F. (2021). An “Omic” overview of fragile X syndrome. Biology 10:433. doi: 10.3390/biology10050433
Dobin, A., Davis, C. A., Schlesinger, F., Drenkow, J., Zaleski, C., Jha, S., et al. (2013). STAR: ultrafast universal RNA-seq aligner. Bioinformatics 29, 15–21. doi: 10.1093/bioinformatics/bts635
Dolan, B. M., Duron, S. G., Campbell, D. A., Vollrath, B., Shankaranarayana Rao, B. S., Ko, H. Y., et al. (2013). Rescue of fragile X syndrome phenotypes in Fmr1 KO mice by the small-molecule PAK inhibitor FRAX486. Proc. Natl. Acad. Sci. U.S.A. 110, 5671–5676. doi: 10.1073/pnas.1219383110
Fan, Z., Zhu, H., Zhou, T., Wang, S., Wu, Y., and Hu, H. (2019). Using the tube test to measure social hierarchy in mice. Nat. Protoc. 14, 819–831. doi: 10.1038/s41596-018-0116-4
Fish, E. W., Krouse, M. C., Stringfield, S. J., Diberto, J. F., Robinson, J. E., and Malanga, C. J. (2013). Changes in sensitivity of reward and motor behavior to dopaminergic, glutamatergic, and cholinergic drugs in a mouse model of fragile X syndrome. PLoS One 8:e77896. doi: 10.1371/journal.pone.0077896
Galeote, L., Berrendero, F., Bura, S. A., Zimmer, A., and Maldonado, R. (2009). Prodynorphin gene disruption increases the sensitivity to nicotine self-administration in mice. Int. J. Neuropsychopharmacol. 12, 615–625. doi: 10.1017/S1461145708009450
Gantois, I., Khoutorsky, A., Popic, J., Aguilar-Valles, A., Freemantle, E., Cao, R., et al. (2017). Metformin ameliorates core deficits in a mouse model of fragile X syndrome. Nat. Med. 23, 674–677. doi: 10.1038/nm.4335
Gauducheau, M., Lemaire-Mayo, V., D’Amato, F. R., Oddi, D., Crusio, W. E., and Pietropaolo, S. (2017). Age-specific autistic-like behaviors in heterozygous Fmr1-KO female mice. Autism Res. 10, 1067–1078. doi: 10.1002/aur.1743
Gholizadeh, S., Arsenault, J., Xuan, I. C., Pacey, L. K., and Hampson, D. R. (2014). Reduced phenotypic severity following adeno-associated virus-mediated Fmr1 gene delivery in fragile X mice. Neuropsychopharmacology 39, 3100–3111. doi: 10.1038/npp.2014.167
Gomis-Gonzalez, M., Busquets-Garcia, A., Matute, C., Maldonado, R., Mato, S., and Ozaita, A. (2016). Possible therapeutic doses of cannabinoid type 1 receptor antagonist reverses key alterations in fragile X syndrome mouse model. Genes 7:56. doi: 10.3390/genes7090056
Greco, C. M., Navarro, C. S., Hunsaker, M. R., Maezawa, I., Shuler, J. F., Tassone, F., et al. (2011). Neuropathologic features in the hippocampus and cerebellum of three older men with fragile X syndrome. Mol. Autism. 2:2. doi: 10.1186/2040-2392-2-2
Gross, C., Banerjee, A., Tiwari, D., Longo, F., White, A. R., Allen, A. G., et al. (2019). Isoform-selective phosphoinositide 3-kinase inhibition ameliorates a broad range of fragile X syndrome-associated deficits in a mouse model. Neuropsychopharmacology 44, 324–333. doi: 10.1038/s41386-018-0150-5
Gross, C., Chang, C. W., Kelly, S. M., Bhattacharya, A., McBride, S. M., Danielson, S. W., et al. (2015). Increased expression of the PI3K enhancer PIKE mediates deficits in synaptic plasticity and behavior in fragile X syndrome. Cell Rep. 11, 727–736. doi: 10.1016/j.celrep.2015.03.060
Gurney, M. E., Cogram, P., Deacon, R. M., Rex, C., and Tranfaglia, M. (2017). Multiple behavior phenotypes of the fragile-X syndrome mouse model respond to chronic inhibition of phosphodiesterase-4D (PDE4D). Sci. Rep. 7:14653. doi: 10.1038/s41598-017-15028-x
Hagerman, R. J., Berry-Kravis, E., Hazlett, H. C., Bailey, D. B. Jr., Moine, H., Kooy, R. F., et al. (2017). Fragile X syndrome. Nat. Rev. Dis. Primers 3:17065. doi: 10.1038/nrdp.2017.65
Hanson, A. C., and Hagerman, R. J. (2014). Serotonin dysregulation in fragile X syndrome: implications for treatment. Intractable Rare Dis. Res. 3, 110–117. doi: 10.5582/irdr.2014.01027
Hansson, C., Shirazi, R. H., Naslund, J., Vogel, H., Neuber, C., Holm, G., et al. (2012). Ghrelin influences novelty seeking behavior in rodents and men. PLoS One 7:e50409. doi: 10.1371/journal.pone.0050409
Hartley, S. L., Seltzer, M. M., Raspa, M., Olmstead, M., Bishop, E., and Bailey, D. B. (2011). Exploring the adult life of men and women with fragile X syndrome: results from a national survey. Am. J. Intellect. Dev. Disabil. 116, 16–35. doi: 10.1352/1944-7558-116.1.16
Hebert, B., Pietropaolo, S., Meme, S., Laudier, B., Laugeray, A., Doisne, N., et al. (2014). Rescue of fragile X syndrome phenotypes in Fmr1 KO mice by a BKCa channel opener molecule. Orphanet J. Rare Dis. 9:124. doi: 10.1186/s13023-014-0124-6
Huebschman, J. L., Davis, M. C., Tovar Pensa, C., Guo, Y., and Smith, L. N. (2021). The fragile X mental retardation protein promotes adjustments in cocaine self-administration that preserve reinforcement level. Eur. J. Neurosci. 54, 4920–4933. doi: 10.1111/ejn.15356
Hunter, J., Rivero-Arias, O., Angelov, A., Kim, E., Fotheringham, I., and Leal, J. (2014). Epidemiology of fragile X syndrome: a systematic review and meta-analysis. Am. J. Med. Genet. A 164, 1648–1658. doi: 10.1002/ajmg.a.36511
Irwin, S. A., Galvez, R., and Greenough, W. T. (2000). Dendritic spine structural anomalies in fragile-X mental retardation syndrome. Cereb. Cortex 10, 1038–1044. doi: 10.1093/cercor/10.10.1038
Jacobson, M. L., Wulf, H. A., Tsuda, M. C., Browne, C. A., and Lucki, I. (2020). Sex differences in the modulation of mouse nest building behavior by kappa opioid receptor signaling. Neuropharmacology 177:108254. doi: 10.1016/j.neuropharm.2020.108254
Jiang, Y., Han, L., Meng, J., Wang, Z., Zhou, Y., Yuan, H., et al. (2022). Gene therapy using human FMRP isoforms driven by the human FMR1 promoter rescues fragile X syndrome mouse deficits. Mol. Ther. Methods Clin. Dev. 27, 246–258. doi: 10.1016/j.omtm.2022.10.002
Jones, S. K., McCarthy, D. M., Vied, C., Stanwood, G. D., Schatschneider, C., and Bhide, P. G. (2022). Transgenerational transmission of aspartame-induced anxiety and changes in glutamate-GABA signaling and gene expression in the amygdala. Proc. Natl. Acad. Sci. U.S.A. 119:e2213120119. doi: 10.1073/pnas.2213120119
Kat, R., Arroyo-Araujo, M., de Vries, R. B. M., Koopmans, M. A., de Boer, S. F., and Kas, M. J. H. (2022). Translational validity and methodological underreporting in animal research: a systematic review and meta-analysis of the fragile X syndrome (Fmr1 KO) rodent model. Neurosci. Biobehav. Rev. 139:104722. doi: 10.1016/j.neubiorev.2022.104722
Kaufmann, W. E., Cortell, R., Kau, A. S., Bukelis, I., Tierney, E., Gray, R. M., et al. (2004). Autism spectrum disorder in fragile X syndrome: communication, social interaction, and specific behaviors. Am. J. Med. Genet. A 129A, 225–234. doi: 10.1002/ajmg.a.30229
Kazdoba, T. M., Leach, P. T., Silverman, J. L., and Crawley, J. N. (2014). Modeling fragile X syndrome in the Fmr1 knockout mouse. Intractable Rare Dis. Res. 3, 118–133. doi: 10.5582/irdr.2014.01024
Keifer, C. M., Day, T. C., Hauschild, K. M., and Lerner, M. D. (2021). Social and nonsocial reward anticipation in typical development and autism spectrum disorders: current status and future directions. Curr. Psychiatry Rep. 23:32. doi: 10.1007/s11920-021-01247-7
Kelley, A. E., Schochet, T., and Landry, C. F. (2004). Risk taking and novelty seeking in adolescence: introduction to part I. Ann. N. Y. Acad. Sci. 1021, 27–32. doi: 10.1196/annals.1308.003
Kim, Y., Jeon, S. J., Gonzales, E. L., Shin, D., Remonde, C. G., Ahn, T., et al. (2022). Pirenperone relieves the symptoms of fragile X syndrome in Fmr1 knockout mice. Sci. Rep. 12:20966. doi: 10.1038/s41598-022-25582-8
Kim, K. S., Lee, K. W., Lee, K. W., Im, J. Y., Yoo, J. Y., Kim, S. W., et al. (2006). Adenylyl cyclase type 5 (AC5) is an essential mediator of morphine action. Proc. Natl. Acad. Sci. U.S.A. 103, 3908–3913. doi: 10.1073/pnas.0508812103
Krasteva, M., Koycheva, Y., Racheva, R., Taseva, T., Raycheva, T., Simeonova, S., et al. (2020). Decreased FMR1 mRNA levels found in men with substance use disorders. Heliyon 6:e05270. doi: 10.1016/j.heliyon.2020.e05270
Liu, Z. H., Chuang, D. M., and Smith, C. B. (2011). Lithium ameliorates phenotypic deficits in a mouse model of fragile X syndrome. Int. J. Neuropsychopharmacol. 14, 618–630. doi: 10.1017/S1461145710000520
Longo, F., Aryal, S., Anastasiades, P. G., Maltese, M., Baimel, C., Albanese, F., et al. (2023). Cell-type-specific disruption of cortico-striatal circuitry drives repetitive patterns of behavior in fragile X syndrome model mice. Cell Rep. 42:112901. doi: 10.1016/j.celrep.2023.112901
Love, M. I., Huber, W., and Anders, S. (2014). Moderated estimation of fold change and dispersion for RNA-seq data with DESeq2. Genome Biol. 15:550. doi: 10.1186/s13059-014-0550-8
Lovelace, J. W., Ethell, I. M., Binder, D. K., and Razak, K. A. (2018). Translation-relevant EEG phenotypes in a mouse model of fragile X syndrome. Neurobiol. Dis. 115, 39–48. doi: 10.1016/j.nbd.2018.03.012
Luo, Y. J., Li, Y. D., Wang, L., Yang, S. R., Yuan, X. S., Wang, J., et al. (2018). Nucleus accumbens controls wakefulness by a subpopulation of neurons expressing dopamine D1 receptors. Nat. Commun. 9:1576. doi: 10.1038/s41467-018-03889-3
Martin, M. M., McCarthy, D. M., Schatschneider, C., Trupiano, M. X., Jones, S. K., Kalluri, A., et al. (2020). Effects of developmental nicotine exposure on frontal cortical GABA-to-non-GABA neuron ratio and novelty-seeking behavior. Cereb. Cortex 30, 1830–1842. doi: 10.1093/cercor/bhz207
McCarthy, D. M., Lowe, S. E., Morgan, T. J., Cannon, E. N., Biederman, J., Spencer, T. J., et al. (2020). Transgenerational transmission of behavioral phenotypes produced by exposure of male mice to saccharin and nicotine. Sci. Rep. 10:11974. doi: 10.1038/s41598-020-68883-6
McCarthy, D. M., Morgan, T. J. Jr., Lowe, S. E., Williamson, M. J., Spencer, T. J., Biederman, J., et al. (2018). Nicotine exposure of male mice produces behavioral impairment in multiple generations of descendants. PLoS Biol. 16:e2006497. doi: 10.1371/journal.pbio.2006497
McDuffie, A., Kover, S., Abbeduto, L., Lewis, P., and Brown, T. (2012). Profiles of receptive and expressive language abilities in boys with comorbid fragile X syndrome and autism. Am. J. Intellect. Dev. Disabil. 117, 18–32. doi: 10.1352/1944-7558-117.1.18
McLaughlin, J. P., Marton-Popovici, M., and Chavkin, C. (2003). Kappa opioid receptor antagonism and prodynorphin gene disruption block stress-induced behavioral responses. J. Neurosci. 23, 5674–5683. doi: 10.1523/JNEUROSCI.23-13-05674.2003
McLennan, Y., Polussa, J., Tassone, F., and Hagerman, R. (2011). Fragile x syndrome. Curr. Genomics 12, 216–224. doi: 10.2174/138920211795677886
McNaughton, C. H., Moon, J., Strawderman, M. S., Maclean, K. N., Evans, J., and Strupp, B. J. (2008). Evidence for social anxiety and impaired social cognition in a mouse model of fragile X syndrome. Behav. Neurosci. 122, 293–300. doi: 10.1037/0735-7044.122.2.293
Mineur, Y. S., Sluyter, F., de Wit, S., Oostra, B. A., and Crusio, W. E. (2002). Behavioral and neuroanatomical characterization of the Fmr1 knockout mouse. Hippocampus 12, 39–46. doi: 10.1002/hipo.10005
Moon, J., Beaudin, A. E., Verosky, S., Driscoll, L. L., Weiskopf, M., Levitsky, D. A., et al. (2006). Attentional dysfunction, impulsivity, and resistance to change in a mouse model of fragile X syndrome. Behav. Neurosci. 120, 1367–1379. doi: 10.1037/0735-7044.120.6.1367
Murphy, M. M., and Abbeduto, L. (2007). Gender differences in repetitive language in fragile X syndrome. J. Intellect. Disabil. Res. 51, 387–400. doi: 10.1111/j.1365-2788.2006.00888.x
Niu, M., Han, Y., Dy, A. B. C., Du, J., Jin, H., Qin, J., et al. (2017). Autism symptoms in fragile X syndrome. J. Child Neurol. 32, 903–909. doi: 10.1177/0883073817712875
Osterweil, E. K., Krueger, D. D., Reinhold, K., and Bear, M. F. (2010). Hypersensitivity to mGluR5 and ERK1/2 leads to excessive protein synthesis in the hippocampus of a mouse model of fragile X syndrome. J. Neurosci. 30, 15616–15627. doi: 10.1523/JNEUROSCI.3888-10.2010
Pacey, L. K., Doss, L., Cifelli, C., van der Kooy, D., Heximer, S. P., and Hampson, D. R. (2011). Genetic deletion of regulator of G-protein signaling 4 (RGS4) rescues a subset of fragile X related phenotypes in the FMR1 knockout mouse. Mol. Cell. Neurosci. 46, 563–572. doi: 10.1016/j.mcn.2010.12.005
Paluszkiewicz, S. M., Martin, B. S., and Huntsman, M. M. (2011). Fragile X syndrome: the GABAergic system and circuit dysfunction. Dev. Neurosci. 33, 349–364. doi: 10.1159/000329420
Paradee, W., Melikian, H. E., Rasmussen, D. L., Kenneson, A., Conn, P. J., and Warren, S. T. (1999). Fragile X mouse: strain effects of knockout phenotype and evidence suggesting deficient amygdala function. Neuroscience 94, 185–192. doi: 10.1016/S0306-4522(99)00285-7
Peier, A. M., McIlwain, K. L., Kenneson, A., Warren, S. T., Paylor, R., and Nelson, D. L. (2000). (Over)correction of FMR1 deficiency with YAC transgenics: behavioral and physical features. Hum. Mol. Genet. 9, 1145–1159. doi: 10.1093/hmg/9.8.1145
Pietropaolo, S., Guilleminot, A., Martin, B., D’Amato, F. R., and Crusio, W. E. (2011). Genetic-background modulation of core and variable autistic-like symptoms in Fmr1 knock-out mice. PLoS One 6:e17073. doi: 10.1371/journal.pone.0017073
Protic, D., Salcedo-Arellano, M. J., Dy, J. B., Potter, L. A., and Hagerman, R. J. (2019). New targeted treatments for fragile X syndrome. Curr. Pediatr. Rev. 15, 251–258. doi: 10.2174/1573396315666190625110748
Qin, M., Xia, Z., Huang, T., and Smith, C. B. (2011). Effects of chronic immobilization stress on anxiety-like behavior and basolateral amygdala morphology in Fmr1 knockout mice. Neuroscience 194, 282–290. doi: 10.1016/j.neuroscience.2011.06.047
Rais, M., Binder, D. K., Razak, K. A., and Ethell, I. M. (2018). Sensory processing phenotypes in fragile X syndrome. ASN Neuro 10:175909141880109. doi: 10.1177/1759091418801092
Roberts, J. E., Clarke, M. A., Alcorn, K., Carter, J. C., Long, A. C., and Kaufmann, W. E. (2009). Autistic behavior in boys with fragile X syndrome: social approach and HPA-axis dysfunction. J. Neurodev. Disord. 1, 283–291. doi: 10.1007/s11689-009-9028-5
Rotschafer, S. E., Trujillo, M. S., Dansie, L. E., Ethell, I. M., and Razak, K. A. (2012). Minocycline treatment reverses ultrasonic vocalization production deficit in a mouse model of fragile X syndrome. Brain Res. 1439, 7–14. doi: 10.1016/j.brainres.2011.12.041
Sare, R. M., Levine, M., and Smith, C. B. (2016). Behavioral phenotype of Fmr1 knock-out mice during active phase in an altered light/dark cycle. eNeuro 3, ENEURO.0035–ENEU16.2016. doi: 10.1523/ENEURO.0035-16.2016
Schaefer, T. L., Davenport, M. H., Grainger, L. M., Robinson, C. K., Earnheart, A. T., Stegman, M. S., et al. (2017). Acamprosate in a mouse model of fragile X syndrome: modulation of spontaneous cortical activity, ERK1/2 activation, locomotor behavior, and anxiety. J. Neurodev. Disord. 9:6. doi: 10.1186/s11689-017-9184-y
Schmitt, L. M., Arzuaga, A. L., Dapore, A., Duncan, J., Patel, M., Larson, J. R., et al. (2022). Parallel learning and cognitive flexibility impairments between Fmr1 knockout mice and individuals with fragile X syndrome. Front. Behav. Neurosci. 16:1074682. doi: 10.3389/fnbeh.2022.1074682
Smith, L. E., Barker, E. T., Seltzer, M. M., Abbeduto, L., and Greenberg, J. S. (2012). Behavioral phenotype of fragile X syndrome in adolescence and adulthood. Am. J. Intellect. Dev. Disabil. 117, 1–17. doi: 10.1352/1944-7558-117.1.1
Smith, L. N., Jedynak, J. P., Fontenot, M. R., Hale, C. F., Dietz, K. C., Taniguchi, M., et al. (2014). Fragile X mental retardation protein regulates synaptic and behavioral plasticity to repeated cocaine administration. Neuron 82, 645–658. doi: 10.1016/j.neuron.2014.03.028
Spencer, C. M., Alekseyenko, O., Hamilton, S. M., Thomas, A. M., Serysheva, E., Yuva-Paylor, L. A., et al. (2011). Modifying behavioral phenotypes in Fmr1 KO mice: genetic background differences reveal autistic-like responses. Autism Res. 4, 40–56. doi: 10.1002/aur.168
Spencer, C. M., Alekseyenko, O., Serysheva, E., Yuva-Paylor, L. A., and Paylor, R. (2005). Altered anxiety-related and social behaviors in the Fmr1 knockout mouse model of fragile X syndrome. Genes Brain Behav. 4, 420–430. doi: 10.1111/j.1601-183X.2005.00123.x
Tao, X., Newman-Tancredi, A., Varney, M. A., and Razak, K. A. (2023). Acute and repeated administration of NLX-101, a selective serotonin-1A receptor biased agonist, reduces audiogenic seizures in developing Fmr1 knockout mice. Neuroscience 509, 113–124. doi: 10.1016/j.neuroscience.2022.11.014
Uutela, M., Lindholm, J., Rantamaki, T., Umemori, J., Hunter, K., Voikar, V., et al. (2014). Distinctive behavioral and cellular responses to fluoxetine in the mouse model for fragile X syndrome. Front. Cell. Neurosci. 8:150. doi: 10.3389/fncel.2014.00150
Vafaeie, F., Alerasool, M., Kaseb Mojaver, N., and Mojarrad, M. (2021). Fragile X syndrome in a female with homozygous full-mutation alleles of the FMR1 gene. Cureus 13:e16340. doi: 10.7759/cureus.16340
Vied, C. M., Freudenberg, F., Wang, Y., Raposo, A. A., Feng, D., and Nowakowski, R. S. (2014). A multi-resource data integration approach: identification of candidate genes regulating cell proliferation during neocortical development. Front. Neurosci. 8:257. doi: 10.3389/fnins.2014.00257
Vied, C., Ray, S., Badger, C. D., Bundy, J. L., Arbeitman, M. N., and Nowakowski, R. S. (2016). Transcriptomic analysis of the hippocampus from six inbred strains of mice suggests a basis for sex-specific susceptibility and severity of neurological disorders. J. Comp. Neurol. 524, 2696–2710. doi: 10.1002/cne.23989
Wang, J., Duncan, D., Shi, Z., and Zhang, B. (2013). WEB-based GEne SeT AnaLysis Toolkit (WebGestalt): update 2013. Nucleic Acids Res. 41, W77–W83. doi: 10.1093/nar/gkt439
Wang, Y., Liu, Y., Yang, L., Gu, F., Li, X., Zha, R., et al. (2015). Novelty seeking is related to individual risk preference and brain activation associated with risk prediction during decision making. Sci. Rep. 5:10534. doi: 10.1038/srep10534
Xu, Z. H., Yang, Q., Feng, B., Liu, S. B., Zhang, N., Xing, J. H., et al. (2012). Group I mGluR antagonist rescues the deficit of D1-induced LTP in a mouse model of fragile X syndrome. Mol. Neurodegener. 7:24. doi: 10.1186/1750-1326-7-24
Yan, Q. J., Asafo-Adjei, P. K., Arnold, H. M., Brown, R. E., and Bauchwitz, R. P. (2004). A phenotypic and molecular characterization of the fmr1-tm1Cgr fragile X mouse. Genes Brain Behav. 3, 337–359. doi: 10.1111/j.1601-183X.2004.00087.x
Yan, Q. J., Rammal, M., Tranfaglia, M., and Bauchwitz, R. P. (2005). Suppression of two major fragile X syndrome mouse model phenotypes by the mGluR5 antagonist MPEP. Neuropharmacology 49, 1053–1066. doi: 10.1016/j.neuropharm.2005.06.004
Yuskaitis, C. J., Mines, M. A., King, M. K., Sweatt, J. D., Miller, C. A., and Jope, R. S. (2010). Lithium ameliorates altered glycogen synthase kinase-3 and behavior in a mouse model of fragile X syndrome. Biochem. Pharmacol. 79, 632–646. doi: 10.1016/j.bcp.2009.09.023
Zachariou, V., Benoit-Marand, M., Allen, P. B., Ingrassia, P., Fienberg, A. A., Gonon, F., et al. (2002). Reduction of cocaine place preference in mice lacking the protein phosphatase 1 inhibitors DARPP 32 or inhibitor 1. Biol. Psychiatry 51, 612–620. doi: 10.1016/S0006-3223(01)01318-X
Zhang, B., Kirov, S., and Snoddy, J. (2005). WebGestalt: an integrated system for exploring gene sets in various biological contexts. Nucleic Acids Res. 33, W741–W748. doi: 10.1093/nar/gki475
Zhang, L., Spencer, T. J., Biederman, J., and Bhide, P. G. (2018). Attention and working memory deficits in a perinatal nicotine exposure mouse model. PLoS One 13:e0198064. doi: 10.1371/journal.pone.0198064
Zhao, X. N., and Usdin, K. (2014). Gender and cell-type-specific effects of the transcription-coupled repair protein, ERCC6/CSB, on repeat expansion in a mouse model of the fragile X-related disorders. Hum. Mutat. 35, 341–349. doi: 10.1002/humu.22495
Keywords: hyperactivity, nest building, impulsivity, GABA, glutamate, substance dependence
Citation: McCarthy DM, Vied C, Trupiano MX, Canekeratne AJ, Wang Y, Schatschneider C and Bhide PG (2024) Behavioral, neurotransmitter and transcriptomic analyses in male and female Fmr1 KO mice. Front. Behav. Neurosci. 18:1458502. doi: 10.3389/fnbeh.2024.1458502
Received: 02 July 2024; Accepted: 22 August 2024;
Published: 06 September 2024.
Edited by:
Emmanuel Darcq, Institut National de la Santé et de la Recherche Médicale (INSERM), FranceReviewed by:
Tiffany Danielle Rogers, Middle Tennessee State University, United StatesCopyright © 2024 McCarthy, Vied, Trupiano, Canekeratne, Wang, Schatschneider and Bhide. This is an open-access article distributed under the terms of the Creative Commons Attribution License (CC BY). The use, distribution or reproduction in other forums is permitted, provided the original author(s) and the copyright owner(s) are credited and that the original publication in this journal is cited, in accordance with accepted academic practice. No use, distribution or reproduction is permitted which does not comply with these terms.
*Correspondence: Deirdre M. McCarthy, RGVpcmRyZS5tY2NhcnRoeUBtZWQuZnN1LmVkdQ==
Disclaimer: All claims expressed in this article are solely those of the authors and do not necessarily represent those of their affiliated organizations, or those of the publisher, the editors and the reviewers. Any product that may be evaluated in this article or claim that may be made by its manufacturer is not guaranteed or endorsed by the publisher.
Research integrity at Frontiers
Learn more about the work of our research integrity team to safeguard the quality of each article we publish.