- School of Health and Life Sciences, University of Health and Rehabilitation Sciences, Qingdao, China
The nucleus accumbens (NAc), a central component of the brain’s reward circuitry, has been implicated in a wide range of behaviors and emotional states. Emerging evidence, primarily drawing from recent rodent studies, suggests that the function of the NAc in reward and aversion processing is multifaceted. Prolonged stress or drug use induces maladaptive neuronal function in the NAc circuitry, which results in pathological conditions. This review aims to provide comprehensive and up-to-date insights on the role of the NAc in motivated behavior regulation and highlights areas that demand further in-depth analysis. It synthesizes the latest findings on how distinct NAc neuronal populations and pathways contribute to the processing of opposite valences. The review examines how a range of neuromodulators, especially monoamines, influence the NAc’s control over various motivational states. Furthermore, it delves into the complex underlying mechanisms of psychiatric disorders such as addiction and depression and evaluates prospective interventions to restore NAc functionality.
1 Introduction
Reward and aversion, two major components of motivation, are essential for learning and adaptation, emotional processes, and generating goal-directed behavior. The nucleus accumbens (NAc) has been historically acknowledged for its pivotal role in reward processing and the regulation of reward-seeking behaviors (Bakshi and Kelley, 1993; Kelley et al., 1997; Soares-Cunha et al., 2020). However, more recent studies have illuminated the NAc’s substantial involvement in aversive processing as well (Levita et al., 2012; Richard et al., 2013; Soares-Cunha et al., 2020; Nishioka et al., 2023). Dysfunction of the NAc induces deficits in both approach and avoidance behaviors (Hoebel et al., 2007; Schwartz et al., 2017).
The NAc’s function in the dichotomous processing of reward and aversion is intricate and dynamic (Lobo et al., 2010; Xiu et al., 2014; Hu, 2016), and its subregions appear to have distinct roles (Al-Hasani et al., 2015; Yang et al., 2018). Neurons within these subregions exhibit further heterogeneity in both the transcription and connectivity levels (Castro and Bruchas, 2019; Chen et al., 2021). Studies employing genetic engineering and optogenetic approaches have demonstrated that different NAc pathways might exert opposing functions over motivated behaviors (Yang et al., 2018; Soares-Cunha et al., 2022). Recently, the development of genetically encoded calcium indicators and fluorescent probes has enabled real-time tracking of neuronal activity and neurotransmitter dynamics in the NAc in freely moving animals (Sun et al., 2020; Chen et al., 2023; Zhuo et al., 2024). This review describes the latest advancements in comprehending the specific role of NAc neuronal ensembles or pathways in mediating reward and aversion and discusses the involvement of the NAc in psychiatric disorders such as addiction and depression (Russo and Nestler, 2013).
2 Behavioral processes modulated by the NAc
The NAc contributes to a variety of behavioral processes, including motivation, reward processing, reinforcement learning, and decision-making. Motivation, which involves the drive to pursue goals, is modulated by the NAc, influencing both the anticipation and consumption of rewarding stimuli (Berridge and Kringelbach, 2015). Reward processing, crucial for evaluating and encoding the hedonic value of outcomes, heavily relies on the NAc as a central hub for integrating information about rewarding stimuli (Luscher and Malenka, 2011). Reinforcement learning, the process of updating action–outcome associations based on experienced outcomes, is influenced by the NAc (Schultz, 2016). Moreover, decision-making, which entails selecting between available options, is modulated by the NAc through integrating information about the value, effort, and costs associated with different choices (Floresco, 2015).
3 NAc neurons mediate positive and negative reinforcement
The NAc is traditionally divided into two main subregions: the core and the shell (NAcC and NAcSh). NAc neurons can be classified into distinct subtypes based on their differential expression of genes, electrophysiological properties, and connectivity. Over 90% of NAc neurons are medium spiny neurons (MSNs) (Chen et al., 2021). Molecular heterogeneities in MSNs have been observed not only within the core and shell regions but also along the anterior–posterior and dorsal–ventral axes (Castro and Bruchas, 2019). Although certain genes are highly enriched in specific subregions (Voorn et al., 1989; Jongen-Relo et al., 1994), no definitive borders between these regions can be identified using marker genes (Chen et al., 2021).
MSNs are the major projection neurons in the NAc, while interneurons might exert their function by modulating the output of the MSNs (Figure 1). Typically, MSNs are not spontaneously active and can exhibit a bistable membrane potential (O'Donnell and Grace, 1995; O'Donnell et al., 1999; Belleau and Warren, 2000; Mahon et al., 2006). Activities of MSNs are primarily triggered by glutamatergic inputs induced depolarization (O'Donnell and Grace, 1995; O'Donnell et al., 1999). Glutamatergic inputs could also enhance spike discharge via metabotropic receptors (D'Ascenzo et al., 2009). GABAergic afferents from ventral arkypallidal neurons and local accumbens interneurons restrict MSN bursting and plasticity (Owen et al., 2018; Vachez et al., 2021). Neuromodulators also significantly shape the output of MSNs (O'Donnell et al., 1999; Brady and O'Donnell, 2004).
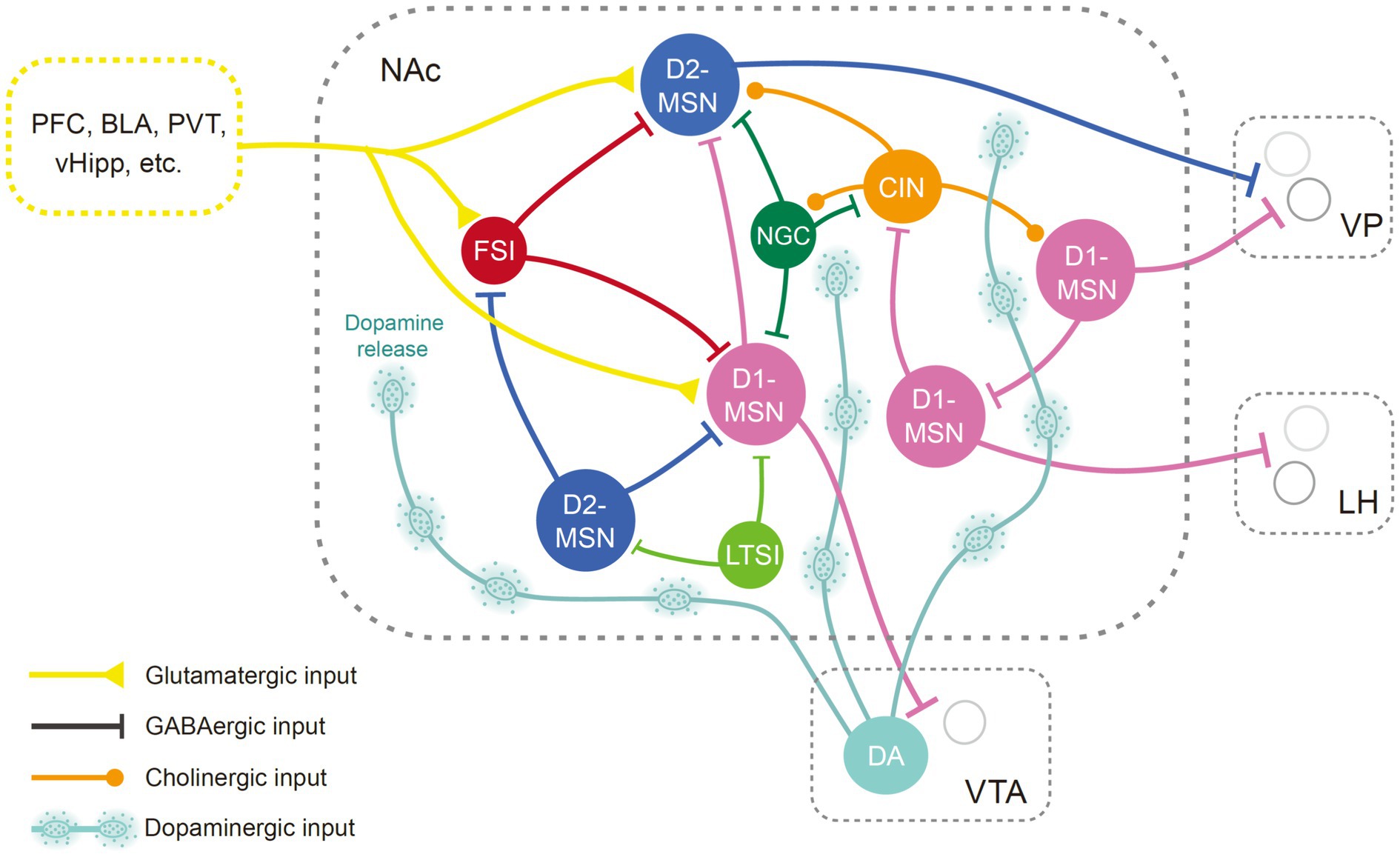
Figure 1. Anatomical diagram of NAc neuron types. Illustration of different NAc neuron types and their basic connectivity pattern. Neurons in the NAc receive glutamatergic inputs from the cortex, the hippocampus, the amygdala, and thalamic regions, as well as dopaminergic input from the midbrain. The MSNs, the principal neurons in the NAc, are innervated by neighboring GABAergic interneurons and cholinergic neurons. The NAc mainly targets the VP, LH, and VTA regions. BLA, basolateral amygdala; CIN, cholinergic interneuron; D1-MSN, D1 type dopamine receptor-expressing medium spiny neuron; D2-MSN, D2 type dopamine receptor-expressing medium spiny neuron; DA, dopaminergic neurons; FSI, fast-spiking interneurons; LH, lateral hypothalamus; LTSI, low-threshold-spiking interneuron; NGC, neurogliaform cells; NAc, nucleus accumbens; PFC, prefrontal cortex; PVT, paraventricular thalamus; vHipp, ventral hippocampus; VP, ventral pallidum; VTA, ventral tegmental area.
While traditional perspectives highlight a functional dichotomy in MSNs based on differential expression of dopamine receptor-1 (D1) and dopamine receptor-2 (D2) (Shippenberg et al., 1993; Hikida et al., 2010; Lobo et al., 2010; Kravitz et al., 2012), recent research challenges this notion (Soares-Cunha et al., 2016b; Cole et al., 2018; Soares-Cunha et al., 2020). In vivo recordings demonstrate that MSNs respond diversely to stimuli such as natural rewards, addictive drugs, and stress (Ghitza et al., 2003; Roitman et al., 2004; Nestler, 2015; Morrison et al., 2017). The response patterns of MSNs depend on their spatial localization, dopamine receptor type, and embedding within specific circuitry (Ray et al., 2022; Chen et al., 2023; Nishioka et al., 2023). It has been proposed that neurons in the core and shell regions process different information in reward-related behaviors (Carelli, 2004; West and Carelli, 2016). By examining immediate early gene c-Fos expression in the NAc, studies indicate that although rewarding stimuli predominantly activate D1-MSNs, aversive stimuli could activate both D1- and D2-MSNs (Xiu et al., 2014; Sheng et al., 2023). In vivo recordings offer enhanced insights into the behavioral correlations with neural activity. For example, fiber photometry recording has revealed that D1- and D2-MSNs are both recruited during hedonic feeding, but represent different aspects of the food approach (Guillaumin et al., 2023). Analysis of single-cell calcium activity has revealed both types of MSNs exhibit diverse responses toward sucrose reward (Pedersen et al., 2022). D1- and D2-MSNs receive similar excitatory inputs and can interact in complex ways (Burke et al., 2017; Li et al., 2018; Keyes et al., 2020). MSNs are interconnected via lateral inhibition (Tunstall et al., 2002; Taverna et al., 2004), which is modulated by dopamine and serotonin (Dobbs et al., 2016; Burke and Alvarez, 2022). In addition, the D1-MSNs express dynorphin and substance P, while the D2-MSNs express adenosine 2A receptors (A2A) and enkephalin (Anderson and Reiner, 1990; Besson et al., 1990). A recent study revealed that endogenous enkephalin could suppress MSN activity via mu opioid receptors, which is necessary for potentiating reward consumption (Castro et al., 2021). D1- and D2-MSNs can regulate the function of interneurons by releasing neuropeptides, which in turn modulate neighboring MSN activity (Francis et al., 2019). D1-MSN stimulations can lead to variable behavioral outcomes (Table 1). Moreover, optogenetic activation of D2-MSN subsets has been shown to increase motivation (Soares-Cunha et al., 2016a, 2018; Yao et al., 2021), while inhibition of D2-MSNs in the core region could slow associative learning (Zachry et al., 2024). Thus, the activity dynamics and function of D2-MSNs are not simply the inverse of those of D1-MSNs, instead, recent evidence suggests D1- and D2-MSNs work in tandem.
Cholinergic interneurons (ClNs) account for approximately 1–2% of NAc neurons (Zhou et al., 2002; Witten et al., 2010) and are more densely populated in the shell region than the core (Meredith et al., 1989). They receive inhibitory inputs from neighboring interneurons and MSNs, as well as GABAergic neurons in the VTA (Brown et al., 2012; Al-Hasani et al., 2021; Baimel et al., 2022). ClNs are tonically active, providing the main source of acetylcholine (ACh) in the NAc (Aosaki et al., 1995; Zhou et al., 2002). Neighboring CINs often show a synchronized cessation of firing in response to reward or associated cues (Goldberg and Reynolds, 2011; Marche et al., 2017), paralleled by a deflect in ACh release (Skirzewski et al., 2022), which might be regulated by dopaminergic and glutamatergic inputs (Chantranupong et al., 2023). On the contrary, an increase of ACh in the NAc is often associated with aversive stimuli or satiety (Helm et al., 2003; Rada et al., 2004; Hoebel et al., 2007; Avena and Rada, 2012). Recent studies have shown that activation of ClNs attenuates conditioning responses to rewards (Collins et al., 2019; Gallo et al., 2022), whereas their inhibition seems to enhance reward reinforcement (Al-Hasani et al., 2021). Although CINs predominantly target MSNs, they also form synapses with other interneurons (English et al., 2011; Mamaligas and Ford, 2016). ACh may modulate neuronal activity directly through muscarinic and nicotinic cholinergic receptors located postsynaptically (Collins et al., 2016; Mamaligas and Ford, 2016), and is also implicated in the regulation of neurotransmitter release (Abudukeyoumu et al., 2019; Reynolds et al., 2022), including dopamine (Cachope et al., 2012; Mohebi et al., 2023). Nonetheless, the influence of ACh on these processes can vary, influenced by the specific subregion of the NAc and receptor types involved (Collins et al., 2016; Shin et al., 2017; Klawonn et al., 2018; Mancini et al., 2022).
The GABAergic interneurons in the NAc are a heterogeneous assembly, comprising fast-spiking interneurons (FSIs), low-threshold-spiking interneurons (LTSI), and neurogliaform cells (NGC) (Tepper et al., 2018). These interneurons are recipients of the same excitatory inputs as the MSNs and contribute to the feedforward inhibition of the MSNs (Qi et al., 2016; Scudder et al., 2018; Trouche et al., 2019). FSIs, identified by their expression of the calcium-binding protein parvalbumin (Bennett and Bolam, 1994), are primarily responsible for fast inhibitory neurotransmission (Wright et al., 2017). Synchronized activation of FSIs promotes conditioned aversion (Schall et al., 2021; Xiao et al., 2021), while certain FSI subpopulations are essential for reward memory retrieval (Trouche et al., 2019), suggesting a nuanced functional diversity among these neurons. LTSIs and NGCs, characterized by their expression of the neuropeptide somatostatin (Kawaguchi et al., 1995) and neuropeptide Y (NPY) (Ibanez-Sandoval et al., 2011) respectively, modulate MSN activity in a more sustained manner (Tepper and Bolam, 2004). Recent studies have shown that LTSIs undergo contrasting changes in excitability following cocaine exposure and footshock experiences (Ribeiro et al., 2018; Kondev et al., 2023). However, how LTSIs participate in valence encoding is still not clear. The involvement of NGCs in reward-related behaviors warrants further investigation.
4 NAc pathways involved in reward and aversion processing
The NAc receives glutamatergic inputs from limbic and cortical areas like the prefrontal cortex (PFC), basolateral amygdala (BLA), ventral hippocampus (vHipp), thalamus (Li and Kirouac, 2008), and is reciprocally connected with the ventral tegmental area (VTA), ventral pallidum (VP), and lateral hypothalamus (LH) (Haber and Knutson, 2010). Studies using trans-synaptic viral strategy and optogenetics combined with patch-clamp electrophysiology have revealed diverse monosynaptic connectivity patterns of neurons in the NAc (Ma et al., 2020; Yao et al., 2021). In general, neurons in different subregions of the NAc exhibit distinct profiles of afferent and efferent connections (Groenewegen et al., 1999). For instance, projections from the vHipp mainly terminate within the medial NAcSh (Kelley and Domesick, 1982). On the other hand, projections from the dorsal prelimbic area predominantly terminate within the core region (Berendse et al., 1992a). Notwithstanding, within a given subregion, D1- and D2-MSNs tend to receive similar inputs (Barrientos et al., 2018; Li et al., 2018). The medial and lateral shell of the NAc targets the medial and lateral parts of the VTA, respectively (Lammel et al., 2012), while the NAcC innervates the substantia nigra (Berendse et al., 1992b). The projections from NAc subregions to the VP and LH are also topographically arranged (Heimer et al., 1991). Although both D1 and D2-MSNs project to the VP, the majority of neurons targeting the VTA and LH express the D1 receptor (Kupchik et al., 2015; Thoeni et al., 2020). Notably, subpopulations of D1-MSNs synapse on GABAergic interneurons in the downstream regions, which produce motivational drive via disinhibition (Watabe-Uchida et al., 2012; Yang et al., 2018; Thoeni et al., 2020; Yao et al., 2021).
Considering the diversity of inputs, MSNs could process and relay distinct pieces of information to specific downstream neurons, thereby orchestrating different motivational states (LeGates et al., 2018; Tong et al., 2024). The PFC inputs to the NAc convey cognitive and executive control signals, influencing decision-making processes and goal-directed behavior (Sun and Rebec, 2006; Domingo-Rodriguez et al., 2020). The input from the vHipp contributes spatial and contextual information to the NAc, influencing memory-related processes and spatial navigation, which may guide goal-directed behaviors in various environmental contexts (Pennartz et al., 2011). The amygdala relays emotional information to the NAc, modulating cue-triggered motivated behaviors (Ambroggi et al., 2008; Stuber et al., 2011). Manipulations of distinct inputs of the NAc might have opposing effects on reward-related behaviors. For instance, activations of the BLA ➔ NAc and PVT ➔ NAc pathways have been found to induce reward and aversion, respectively (Stuber et al., 2011; Zhu et al., 2016). It has been suggested that the BLA and PVT might target different MSN ensembles, which in turn exhibit distinct projection patterns. The MSNs receiving BLA input preferentially target GABAergic neurons in the VTA and glutamatergic neurons in the LH to mediate reward, whereas MSNs receiving PVT input are associated with aversive outcomes, primarily through the inhibition of GABAergic neurons in the LH (Zhou et al., 2022). Interestingly, it has been revealed that both the BLA and PVT encompass various neuron subtypes and functional subregions that might mediate opposing valence (Namburi et al., 2015; Shen et al., 2019; Christoffel et al., 2021a; Poggi et al., 2024). Both inputs innervate interneurons in the NAc (Yu et al., 2017; Paniccia et al., 2024), in addition to targeting directly on MSNs. Thus, convergence and segregation of heterogenous inputs might modulate MSN activity and contribute to the processing of reward and aversion in a more dynamic and complex way than previously expected. The recent discoveries utilizing optogenetic or pharmacogenetic approaches to dissect the functionality of NAc pathways are summarized in Table 1.
5 Monoamine neurotransmitters modulate NAc function
Multiple monoamine neurotransmitters modulate the activity of NAc neurons. These neurotransmitters transmit crucial information about rewards, punishment, and arousal, indispensable for adaptive motivation and learning (Castro and Bruchas, 2019). The following subsections will delve into the properties of dopamine, serotonin, and norepinephrine signals within the NAc, addressing the origin of their inputs, the stimuli or conditions that induce their release, the receptor types involved, and the behavioral relevance of these processes.
5.1 Dopamine (DA)
Dense dopaminergic projections from the ventral tegmental area (VTA) target the NAc, establishing a critical neurotransmitter for reward in this region (Schultz, 1998; Bjorklund and Dunnett, 2007; Haber and Knutson, 2010). DA neurons in the VTA exhibit irregular firing patterns with phasic high frequencies of action potentials (Marinelli and McCutcheon, 2014; Berke, 2018), potentially instigating transient surges in DA release (Floresco et al., 2003; Liu et al., 2018). Additionally, DA fluctuations in the NAc are subject to regulation by spike-independent processes regulating DA release and uptake (Mohebi et al., 2019; Morrens et al., 2020; Sabandal et al., 2021). For instance, presynaptic opioid and nicotinic receptors could modulate DA release in the NAc (Britt and McGehee, 2008; McGovern et al., 2023). DA concentration in the NAc is also regulated by glutamatergic inputs from the BLA and ACh release from local CINs (Floresco et al., 1998; Jones et al., 2010; Threlfell et al., 2012; de Jong et al., 2022). DA neurons display heterogeneity in their activity, co-released transmitters, and axon projections (Morales and Margolis, 2017). DA neurons in the VTA can be activated by both rewarding and aversive stimuli (Matsumoto and Hikosaka, 2009), yet it is believed that DA projections to the NAc primarily signal reward-related events (Day et al., 2007; Lammel et al., 2008, 2011; Gunaydin et al., 2014). Stimulation of DA terminals in the NAc is sufficient to support self-stimulation or induce place preference, implying that dopamine release per se can act as reinforcement (Tsai et al., 2009; Witten et al., 2011; Jing et al., 2022). Conversely, depletion of DA with 6-OHDA in the NAc impairs not only reward seeking but also punishment avoidance (Bergamini et al., 2016). Utilizing genetically encoded sensors, recent research has revealed the diversity of dopamine signaling throughout the NAc (Patriarchi et al., 2018; Sun et al., 2018). While reward stimuli increase DA release in most of the NAc, aversive stimuli specifically augment DA levels in the ventromedial shell of the NAc (vmNAcSh) (de Jong et al., 2019; Yuan et al., 2019). In addition, the cession of the punishment servers as a relief reward to increase DA release in the dorsomedial shell and the lateral shell of the NAc (dmNAcSh and lNAcSh) (Dong et al., 2023). Silencing the DA input to the NAc shell abolishes relief learning (Mayer et al., 2018; Dong et al., 2023). In NAcC, dopamine release denotes perceived saliency (Kutlu et al., 2021; Goedhoop et al., 2022; Jeong et al., 2022) rather than adhering to a prediction error model (Bayer and Glimcher, 2005; Schultz, 2016). A recent study indicates that optogenetic manipulation of the DA response to a novel stimulus in the NAcC, prior to training, can bidirectionally influence the stimulus’s capacity to act as a predictive cue for punishment (Kutlu et al., 2022), highlighting the significance of DA in novelty-based learning within this subregion.
Dopamine receptors are widely distributed across the NAc, existing in both pre-and postsynaptic locations (Goto and Grace, 2005). D1-like receptors (D1 and D5) and D2-like receptors (D2, D3, and D4) are coupled to Gs/olf and Gi/o signaling pathways, respectively, modulating neuronal excitability and synaptic plasticity in distinct manners (Soares-Cunha et al., 2016b; Lee et al., 2021; Tsuboi et al., 2022). For example, D1 receptors may promote the phosphorylation of AMPA receptors and increase spine density, thereby intensifying behavioral responses to drug rewards (Hobson et al., 2013; Nagai et al., 2016). The extinction of drug memory is associated with Rac1-dependent reduction in spine density in D1-MSNs (Tu et al., 2019; Zhao et al., 2019), which could be attenuated by D1 receptor stimulation (Kobrin et al., 2017). In addition, D1R in different NAc subregions might contribute to distinct aspects of reward processing (Gore and Zweifel, 2013; Hauser et al., 2015). Notably, evidence also points to D1 receptors playing a role in aversion processing (Acquas and Di Chiara, 1994; Chartoff et al., 2006; Kim et al., 2007), supporting the multifaceted role of DA transmission in the NAc (Verharen et al., 2020). D2-like receptors are also indispensable for reward and reward association (Caine et al., 2002; Tran et al., 2002). Knockdown of D2-like receptors in the NAc attenuates drug reward (Miyamoto et al., 2014), while their overexpression enhances motivation (Trifilieff et al., 2013), probably by suppressing inhibitory transmission to the VP (Gallo et al., 2018). It has thus been proposed that D1- and D2-like receptors play a cooperative role in regulating reward-related behaviors (Self and Stein, 1992; Ikemoto et al., 1997; Schmidt and Pierce, 2006; Steinberg et al., 2014). Compared with D1 receptors, D2 receptors have a higher affinity for DA (Richfield et al., 1989; Beaulieu and Gainetdinov, 2011). This characteristic potentially allows D2 receptors to detect dips in DA release (Iino et al., 2020), which might represent a negative prediction error (Chang et al., 2016), thereby mediating aversion (Danjo et al., 2014) and enabling reward discrimination (Iino et al., 2020; Nishioka et al., 2023). However, caution is warranted when interpreting data from studies involving drugs of abuse, where excessive DA release could lead to aberrant activation of receptors, which does not occur with natural rewards. In addition, hedonic states do not rely exclusively on dopamine receptors (Narayanan et al., 2004).
In summary, the role of D1 and D2 receptors in the NAc is not simply promoting reward and aversion, respectively. The information transmitted through the DA signal to the NAc may vary depending on behavioral context and should be analyzed with cell-type and pathway-specific evaluations in future studies. Additionally, a small proportion of MSNs co-express both D1 and D2 receptors, the functional significance of which remains to be elucidated.
5.2 Serotonin (5-hydroxytryptamine or 5-HT)
Serotoninergic innervation of the NAc originates primarily from the dorsal raphe nucleus (DRN) and, to a lesser extent, from the median raphe nucleus (MRN) (Vertes and Martin, 1988; Ren et al., 2018). 5-HT terminals establish synaptic contacts with both dendrites and axon terminals in the NAc (Van Bockstaele and Pickel, 1993), influencing NAc activity via a range of presynaptic and postsynaptic mechanisms (Hayes and Greenshaw, 2011; Virk et al., 2016; Christoffel et al., 2021a). 5-HT neurons in the DR show diverse response patterns toward a variety of rewarding and aversive stimuli (Liu et al., 2014; Li et al., 2016; Okaty et al., 2019). The 5-HT signal in the NAc has been associated with sociability and drug reward (Liu Z. et al., 2020). Optogenetic stimulation of the DR ➔ NAc pathway or inhibition of 5-HT re-uptake within the NAc enhances preferences for non-aggressive social interactions, without directly reinforcing behaviors (Walsh et al., 2018). The prosocial effect of 5-HT is dependent on the 5-HT1b receptors located predominantly at presynaptic sites in the NAc (Dolen et al., 2013; Pomrenze et al., 2022). It has been demonstrated that 5-HT depresses excitatory synaptic transmission to the NAc in an input-specific way (Christoffel et al., 2021a). Emphathogens such as MDMA are known to engender prosocial effects, partly through the inhibition of 5-HT re-uptake (Heifets et al., 2019). A recent study suggests that the 5-HT2a receptor is implicated in the reopening of the critical period for social reward learning, induced by lysergic acid diethylamide (LSD) and psilocybin (Nardou et al., 2023). However, ketamine and MDMA induce reinstatement of social reward learning in a 5-HT2a-independent way. There are conflicting reports regarding the role of 5-HT in mediating drug reinforcement effects (Roger-Sanchez et al., 2013; Matuskey et al., 2014; Heifets et al., 2019). Nevertheless, studies indicate that the 5-HT1b receptor modulates the rewarding effect of cocaine in a context-dependent manner (Barot et al., 2007; Pentkowski et al., 2012, 2014) and plays an important role in developing compulsive drug-seeking behavior (Li et al., 2021). Activation of 5-HT6 receptors in the NAc has been shown to promote both natural and drug reward (Ferguson et al., 2008; Pratt et al., 2012). Interestingly, despite both NAc and 5-HT signaling being associated with the suppression of premature reward-seeking behaviors (Bouwknecht et al., 2001; Basar et al., 2010; Miyazaki et al., 2014, 2018), direct stimulation of 5-HT terminals or pharmacological activation of the 5-HT2c receptor in the NAc does not promote patience for reward waiting (Miyazaki et al., 2020; Harmson et al., 2022).
5.3 Norepinephrine
Norepinephrine is another monoamine neurotransmitter that innervates the NAc, with primary projections arising from the locus coeruleus (LC) and the nucleus of the solitary tract (NST) (Delfs et al., 1998). The activity of catecholaminergic neurons in the LC and NST is typically suppressed during reward consumption (Roberts et al., 2017; Sciolino et al., 2022). NE signaling plays a key role in the regulation of arousal and the assignment of salience to stimuli (Ventura et al., 2007). NE release in the NAc is increased in response to aversive stimuli (Gomez-Milanes et al., 2012), and the direct administration of NE into the NAc produces a stimulatory effect (Svensson and Ahlenius, 1982; Plaznik et al., 1985). NE mediates its effect mainly through α- and β-adrenergic receptors, which are located in both dendrites and axons in the NAc (Mitrano et al., 2012). As a result, NE plays an important role in regulating glutamatergic and dopaminergic transmission in the NAc (Aono et al., 2007; Saigusa et al., 2012; Peng et al., 2018). Notably, NE signaling has been shown to selectively influence glutamatergic synapses onto FS interneurons, with minimal direct impact on MSNs (Manz et al., 2021). Although recording NE signals in freely moving animals has been challenging, there have been recent advances in the development of genetically encoded NE sensors (Feng et al., 2019; Kagiampaki et al., 2023; Mao et al., 2023). Monitoring and manipulating NE release in the NAc with high temporal and spatial precision during approach and avoidance behaviors presents a significant opportunity for future research.
The DA, 5-HT, and NE systems do not act in isolation but rather interact to finely tune the NAc’s response to environmental stimuli. In addition to the coordination of neuronal activity in regions upstream of the NAc, monoamines also locally interact within the NAc to modulate reward processing. On one hand, monoamine receptors, extensively located on presynaptic terminals within the NAc, modulate the release of various neurotransmitters. For instance, adrenoceptors may play an inhibitory role in DA efflux (Kochenborger et al., 2012), potentially contributing to an anhedonic state. On the other hand, DA and NE share structural similarities and can bind to each other’s receptors and transporters (Borgkvist et al., 2012). As a result, 6-OHDA can be taken up by both the dopamine transporter (DAT) and the norepinephrine transporter (NET), subsequently inducing damage to both DA and NE terminals (Braun et al., 2016). Additionally, 5-HT can be transported by the DAT and subsequently be released together with DA (Stamford et al., 1990; Zhou et al., 2005), and the 5-HT transporter is also capable of transporting DA (Kannari et al., 2006; Larsen et al., 2011). While the crosstalk between monoaminergic systems may not be evident under normal physiological conditions, it should not be overlooked in drug studies (Hall et al., 2004). Notably, the distribution and functionality of these neurotransmitters vary within different regions of the NAc (Pickel and Chan, 1999; Brown and Molliver, 2000; Chuhma et al., 2014), which could result in inconsistencies in the outcomes of pharmacological interventions. Addressing these nuances remains an important challenge for future research.
6 Role of the NAc in drug addiction
Drug addiction, a chronic and relapsing brain disorder, is characterized by compulsive drug seeking and use, loss of control over intake, and negative emotional states during withdrawal (Koob and Volkow, 2016). The NAc is a central hub in the neural circuitry of addiction. Studies have shown that animals will self-administer (SA) a variety of drugs directly into the NAc (McBride et al., 1999). Diverse genetically defined or pathway-specific neuron ensembles in the NAc contribute to distinct behavioral and emotional aspects of drug addiction (Luscher and Malenka, 2011; Zinsmaier et al., 2022). The NAc responds to both addictive drugs and environmental cues associated with drug use (Table 2). Drugs of abuse influence NAc neuronal activity in multiple ways, including alterations in gene expression, direct receptor activation, modulation of DA release, and changes in synaptic transmission (Dobbs et al., 2016; Christoffel et al., 2021b; Teague and Nestler, 2022; He Y. et al., 2023).
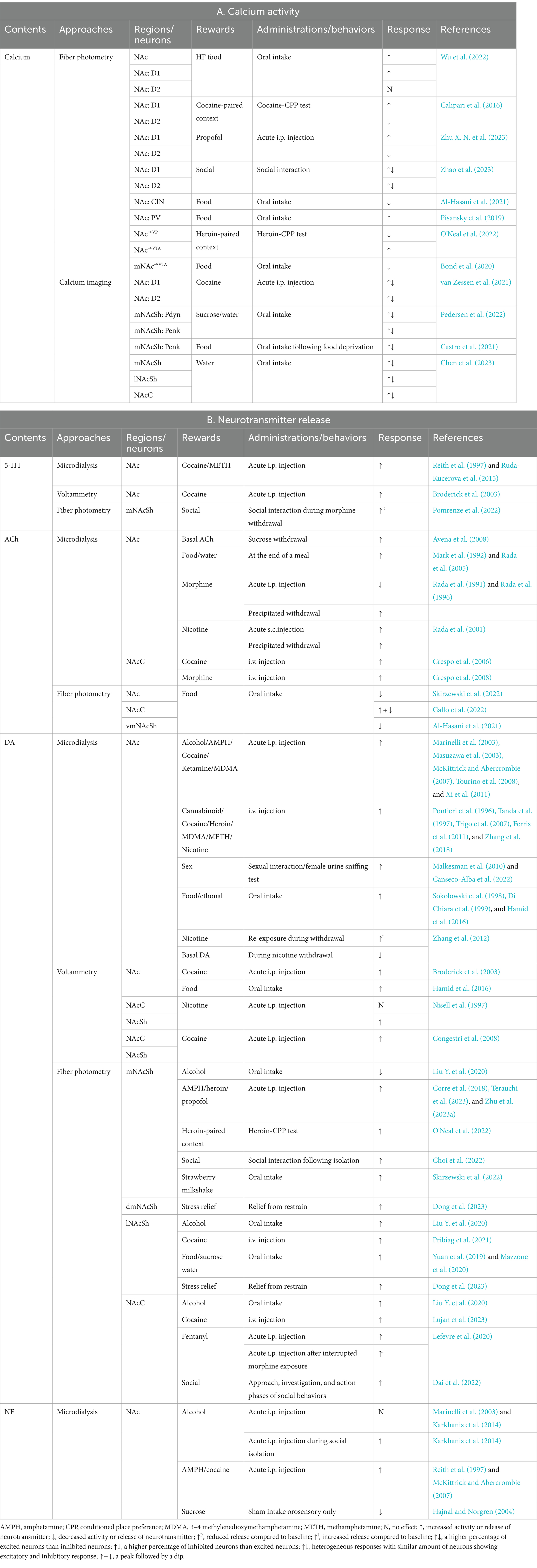
Table 2. In vivo monitoring of neuronal activity and neurotransmitter release in the NAc during reward-related behaviors.
The DA system plays a pivotal role in mediating the rewarding or reinforcing effects of addictive substances (Luscher and Pascoli, 2021; Samaha et al., 2021). Most drugs of abuse enhance DA release in the NAc (Di Chiara and Imperato, 1986; Johnson and North, 1992; Morel et al., 2019; Simmler et al., 2022) via a variety of mechanisms, such as stimulating or disinhibiting DA neurons, augmenting DA release, and blocking DA re-uptake (Chen et al., 2006; Venton et al., 2006; Sulzer, 2011; Bocklisch et al., 2013). The DA release in the NAc, particularly in the shell region, correlates with the initial rewarding effect of drug use (Di Chiara, 2002; Calabrese, 2008; Ito and Hayen, 2011; Floresco et al., 2018). Evidence suggests that the accumbal D1 receptor is essential for the positive reinforcing effects of drugs of abuse (Caine et al., 2007; Chellian et al., 2022). The DA responses to ongoing drug use vary with the drug intake regimen (Ferris et al., 2012; Calipari et al., 2013). With sporadic drug exposure, the DA response to drugs or associated cues may escalate over time (Addy et al., 2010), which could fortify drug memories and eventually promote drug- or cue-induced relapse (Bossert et al., 2013; Jing et al., 2022). Conversely, this process may hinder the learning and memory associated with natural rewards (Luscher and Malenka, 2011; Saddoris et al., 2017). During periods of drug abstinence, an anhedonia emotional state has been linked to reduced basal DA levels (Volkow et al., 2007). Interestingly, D1 and D2 receptor-expressing MSNs are both involved in withdrawal symptoms (Fox et al., 2023), likely through different downstream pathways. Apart from DA, a spectrum of neurotransmitter systems within the NAc are implicated in addiction. For example, the 5-HT release in the NAc increases following drug taking and decreases during withdrawal (Weiss et al., 1996; Broderick et al., 2004), contributing to the hedonic and anhedonic states experienced in addiction. Neuropeptide Y (NPY) in the NAc has been shown to potentiate drug reward (Tanaka et al., 2021). ACh, dynorphin, and norepinephrine signals in the NAc all play a part in the aversion associated with withdrawal (Mu et al., 2011; Almela et al., 2012; Gomez-Milanes et al., 2012).
Prolonged exposure to addictive substances induces enduring changes in neural plasticity within the NAc, which are crucial for the development of persistent drug-related memories and atypical behaviors (Parsegian and See, 2014). Following drug exposure, there is evidence of de novo spine formation in MSNs, which could be mediated by brain-derived neurotrophic factor (BDNF) signaling (Huang et al., 2009; Barrientos et al., 2018). The regulation of the amount and function of glutamatergic receptors and transporters has also been observed during the processes of drug-seeking and drug-memory formation (Lu et al., 2004; Conrad et al., 2008; Loweth et al., 2014; Niedzielska-Andres et al., 2021), whereas different drugs produce cell-specific effects on spines and synapses in the NAc (Graziane et al., 2016). Repeated drug use also alters GABA transmission in the NAc (Xi et al., 2003). Furthermore, changes in the intrinsic excitability of MSNs following drug use have been reported (Mu et al., 2010; He Y. et al., 2023). These structural and electrophysiological changes within the NAc may vary depending on the cell type and source of synaptic input (Thompson et al., 2021; Zhao et al., 2022; Zinsmaier et al., 2022). For instance, the BLA neurons, encoding emotional valence, might fine-tune the activities of MSNs for the acquisition of operant behavior and cue–reward association (Ambroggi et al., 2008; Yu et al., 2017). The maturation of silent synapses of the BLA ➔ NAc pathway during abstinence has been associated with cocaine craving (Lee et al., 2013). Inputs from the vHipp to the NAc, providing spatial information, play a role in drug-related contextual memories (Pascoli et al., 2014; Zhou et al., 2019, 2020), and the transmission is potentiated following cocaine exposure (Britt et al., 2012). Drug-induced adaptations in the PVT ➔ NAc pathway have also been revealed, which might differently contribute to withdrawal symptoms and the reinstatement of drug-seeking behaviors (Zhu et al., 2016; Giannotti et al., 2021; Paniccia et al., 2024). On the contrary, cocaine-seeking behavior correlates with decreased synaptic transmission from the PFC (Pascoli et al., 2014), which exerts executive control (Koob and Volkow, 2010; Chen et al., 2013). Targeted reversal of these synaptic alterations can significantly modify the likelihood of drug relapse (Gipson et al., 2013; Heinsbroek et al., 2021). Specifically, the maturation of silent synapses on infralimbic ➔ NAc and prelimbic ➔ NAc pathways inhibits and promotes drug-seeking, respectively (Ma et al., 2014).
Overall, these maladaptive changes in the NAc can undermine the normal systems for motivation control and cognitive flexibility, resulting in cravings and compulsive drug-seeking behavior (Everitt and Robbins, 2005). Restoring control over such behavior could be achieved by counteracting the pathological effects of drug exposure (Kalivas and O'Brien, 2008; Moussawi et al., 2011). Pharmacological approaches aiming to replace or neutralize the effect of aberrant receptor activations in the NAc induced by drug taking or withdrawal have been proven to be effective (Nestler, 2005; Koob et al., 2009). Methadone, a long-acting agonist of opioid receptors, is commonly prescribed as a substitution treatment for drug detoxification (Leri et al., 2006; Bell and Strang, 2020). D3/D2 receptor partial agonists have shown potential to decrease psychostimulant intake (Neisewander et al., 2014). Approaches such as normalizing NAc activity with deep brain stimulation (DBS) and transcranial-focused ultrasound (tFUS) have also shown promise (Legon et al., 2014; Niu et al., 2020; Chang et al., 2022). The genetic and epigenetic changes that underpin drug-induced neuroplasticity within the NAc present new targets for developing innovative treatments for drug addiction (Ribeiro et al., 2018; Gallegos et al., 2022). Moreover, immunotherapy represents a novel and potentially transformative approach to restoring NAc function in individuals with substance abuse, as highlighted by recent research from Zhu et al. (2023b). These strategies may offer hope for more effective treatments that could address the underlying neurobiological changes associated with addiction.
7 NAc and reward processing deficits in depression
Depression, a widely prevalent mental health disorder, involves a complex interplay of genetic, environmental, and neurobiological factors (Duman et al., 2019; Ormel et al., 2019). Despite the inability of animal models to fully replicate the spectrum of human depression symptoms, they allow for the assessment of key elements such as anhedonia, passive coping, and social avoidance. Both in patients and animal models, these behavioral abnormalities have been linked to dysfunctions of the NAc (Pizzagalli et al., 2009; Wacker et al., 2009).
Chronic stress is a significant risk factor for mood disorders. It is frequently employed to study stress-related reward processing deficits in psychiatric disorders in animal research. Chronic stress induces profound changes in the structure and function of the NAc (Wook Koo et al., 2016; Qi et al., 2022). Extensive studies have been conducted to examine the morphological changes in dendrites and dendritic spines in the NAc (Qiao et al., 2016; Fox et al., 2020). NAc neurons exhibit changes in activity and plasticity in response to stress that are specific to the type of cell and neural pathways (Heshmati et al., 2020; Pignatelli et al., 2021). Moreover, stress alters the release of transmitters and neuromodulators in the NAc (Lowes et al., 2021; Fontaine et al., 2022). Aberrant levels of dopamine and serotonin are recognized as significant contributors to the pathogenesis of depression (Cabib and Puglisi-Allegra, 2012). Furthermore, emerging research is investigating the potential role of stress-induced neuroinflammation in depression, suggesting it could be a contributing factor (Calcia et al., 2016). Pro-inflammatory cytokines can interfere with neurotransmission and synaptic plasticity (Song et al., 1999; Nguyen et al., 2020; Zipp et al., 2023) and heightened neuroinflammation in the NAc has been documented in both depressive patients and animal models (Menard et al., 2017; Wang J et al., 2022). In essence, structural and functional changes, impaired synaptic plasticity, neurotransmitter dysregulation, and neuroinflammation within the NAc could result in an imbalance between reward and aversion. Elevated sensitivity to aversive stimuli, coupled with diminished responses to rewards, may underlie core symptoms of depression such as anhedonia, increased negative affect, and heightened stress responses (Nestler and Carlezon, 2006; Treadway and Zald, 2011; Warner-Schmidt et al., 2012; Felger and Treadway, 2017).
Interestingly, not all animals subjected to environmental stressors develop depression-like behaviors (Wood et al., 2010; Nasca et al., 2015). This observation has propelled research into the mechanisms that dictate diverse behavioral profiles in ‘resilient’ versus ‘susceptible’ animals (Cathomas et al., 2019). Investigations have delved into the transcriptional and epigenetic modifications that may contribute to stress susceptibility (Chandra et al., 2017; Labonte et al., 2017; Wang Z. J. et al., 2022; Kim et al., 2024). For example, Shisa6, an AMPA receptor auxiliary protein, is more abundant in the D1-MSNs of susceptible mice compared to the resilient ones (Kim et al., 2021). Enzymes like Dot1l and Kdm2b, which selectively regulate the demethylation of histone H3 lysine 79 (H3K79me2) in D2-MSNs, have been implicated in early-life stress-induced susceptibility (Kronman et al., 2021). Studies interested in the circuitry mechanism of stress susceptibility have primarily concentrated on the neural pathways involving dopaminergic neurons (Koo et al., 2019; Zhang et al., 2019). It has been suggested that enhanced phasic firing of the VTA ➔ NAc pathway, as opposed to the VTA ➔ mPFC pathway, mediates vulnerability to chronic social defeat stress (CSDS) (Chaudhury et al., 2013; Friedman et al., 2014). It is worth pointing out that both behavioral and physiological differences might present as traits before stress exposure. Altered performance in novel environment exploration and passive avoidance tests has been reported to predict outcomes after chronic social defeats (Milic et al., 2021). In addition, mice that later become resilient might show increased baseline activity in D1-MSNs and enhanced calcium responses to social interaction (Muir et al., 2018), which is in accordance with the finding that enhancing activity in D1-MSNs results in resilient behaviors (Francis et al., 2015). Although the evidence is preliminary, it shows the potential for early preventive interventions.
Antidepressants, at least partially, work by normalizing NAc functionality to improve mood and motivation (Tan et al., 2020; Carboni and Carta, 2022). Agents like ketamine, known for their rapid action, have demonstrated efficacy in restoring synaptic plasticity and normalizing structural outgrowth in the NAc (Belujon and Grace, 2014; Abdallah et al., 2017; Nardou et al., 2023). Furthermore, deep brain stimulation targeting the NAc has shown promise as a treatment for depression (Bewernick et al., 2010; Scangos et al., 2021; Song et al., 2024). A recent study demonstrated that augmenting the rewarding effects of relief from stress by stimulating DA inputs to the NAc or providing animals with food rewards promotes resilience (Dong et al., 2023). Thus, a deeper understanding of reward processing in the NAc could pave the way for novel therapeutic strategies to treat depression (Nestler and Carlezon, 2006).
8 Discussion
Recent research underscores the complex and dynamic role of the NAc in mediating both reward and aversion. This review has scrutinized the heterogeneous patterns of neuronal activity within the NAc during motivated behaviors. A particular focus has been the innovative use of fiber photometry and genetically encoded fluorescent sensors in the NAc. These technologies have shed light on the roles of neurotransmitters such as dopamine, serotonin, and acetylcholine in orchestrating the delicate balance of reward and aversion. Such methods have enabled the real-time observation of neurotransmitter dynamics in active animals, which paves the way for detailed dissections of the signaling pathways that regulate NAc function and its dysfunctions with an unprecedented level of precision. While this review has not delved into the function of NAc glia cells, it is worth acknowledging the increasing interest in their roles in the regulation of emotion and behavior (Adeluyi et al., 2019; Corkrum et al., 2020; Reverte et al., 2024). Future research should not only probe deeper into neuronal activity but also the NAc’s microenvironment, which encompasses factors such as extracellular matrix changes, neurovascular interactions, and the impact of peripheral signals on central reward mechanisms (Menard et al., 2017; Zhang et al., 2020; Hazlett et al., 2024).
Contemporary advancements in human neuroimaging techniques, such as functional magnetic resonance imaging (fMRI) and positron emission tomography (PET), continue to emphasize the role of the NAc in addiction and depression (Huang et al., 2024). The burgeoning field of optogenetics and chemogenetics in animal research presents promising avenues for teasing apart the complex networks involved in psychiatric disorders. Bridging the gap between animal research and human clinical studies through translational research is crucial in validating findings and ensuring their relevance to clinical populations. This review has synthesized the present knowledge of the involvement of the NAc in addiction and depression, mainly drawing from rodent studies. The neuropathological features of these conditions should not be viewed as mere opposites, but as conditions that may involve common molecular and circuitry pathways (Zhu et al., 2023a). The comorbidity of these two diseases is often overlooked in research. For instance, individuals with substance abuse frequently experience depressive episodes during withdrawal that can trigger drug-seeking and relapse. The precise role that the NAc plays in the progression of these complex interactions remains to be fully elucidated. Additionally, there is emerging evidence for the use of psychostimulants as a treatment for depression (Willyard, 2022; Calder and Hasler, 2023), however, discerning their antidepressant benefits from their addictive risks is still not well-defined. The potential mechanisms of stress susceptibility in depression have been discussed in the review, but a research gap should be noted in understanding the mechanisms that may predispose individuals to addiction (Volkow et al., 2019).
The critical role of the NAc in processing aversion underscores its broader significance in psychiatric disorders. Functional imaging studies have revealed that patients with posttraumatic stress disorder (PTSD) exhibit reduced responses to rewards and increased activity in the left accumbens when exposed to trauma reminders (Liberzon et al., 1999; Sailer et al., 2008; Pessin et al., 2021; Ploski and Vaidya, 2021). NAc circuits are implicated in both the learning and extinction of fear (Correia et al., 2016; Smith et al., 2021). In anxiety disorders, dysfunction of the NAc is associated with heightened fear and stress responses (Kalin et al., 2005; Ray et al., 2020; Xiao et al., 2021). Additionally, the NAc plays a significant role in pain processing, with its activity correlating with variations in pain intensity and unpleasantness (Kaneko et al., 2017). The NAc integrates multiple neurotransmitter systems, including glutamate, GABA, dopamine, opioids, and substance P, each contributing to pain and fear modulation (Harris and Peng, 2020; Belilos et al., 2023). Beyond aversion perception dysregulation, the NAc is involved in the development of maladaptive avoidance behaviors, highlighting its integral role in the emotional and motivational aspects of mental health.
In conclusion, there is a pressing need for further research to unravel the nuances of reward and aversion processing in the NAc. Such understanding is critical for the development of targeted therapeutic approaches for psychiatric disorders.
Author contributions
YX: Writing – original draft, Writing – review & editing. YL: Writing – original draft, Writing – review & editing. MY: Funding acquisition, Writing – review & editing. KZ: Conceptualization, Funding acquisition, Writing – original draft, Writing – review & editing.
Funding
The author(s) declare financial support was received for the research, authorship, and/or publication of this article. This study was supported by the Science and Technology Innovation 2030 – Major Project (2022ZD0207100 and 2021ZD0203500), the National Natural Science Foundation of China (32371041), Key Project of the Basic and Applied Basic Research Foundation & Regional Joint Foundation of Guangdong Province (2021B1515120020), the Taishan Scholars Program of Shandong Province (tsqn202312243), the Natural Science Foundation of Qingdao (23-2-1-188-zyyd-jch) and the Natural Science Foundation of Shandong province (ZR2023MH051).
Conflict of interest
The authors declare that the research was conducted in the absence of any commercial or financial relationships that could be construed as a potential conflict of interest.
Publisher’s note
All claims expressed in this article are solely those of the authors and do not necessarily represent those of their affiliated organizations, or those of the publisher, the editors and the reviewers. Any product that may be evaluated in this article, or claim that may be made by its manufacturer, is not guaranteed or endorsed by the publisher.
References
Abdallah, C. G., Jackowski, A., Salas, R., Gupta, S., Sato, J. R., Mao, X., et al. (2017). The nucleus accumbens and ketamine treatment in major depressive disorder. Neuropsychopharmacology 42, 1739–1746. doi: 10.1038/npp.2017.49
Abudukeyoumu, N., Hernandez-Flores, T., Garcia-Munoz, M., and Arbuthnott, G. W. (2019). Cholinergic modulation of striatal microcircuits. Eur. J. Neurosci. 49, 604–622. doi: 10.1111/ejn.13949
Acquas, E., and Di Chiara, G. (1994). D1 receptor blockade stereospecifically impairs the acquisition of drug-conditioned place preference and place aversion. Behav. Pharmacol. 5, 555–569. doi: 10.1097/00008877-199410000-00001
Addy, N. A., Daberkow, D. P., Ford, J. N., Garris, P. A., and Wightman, R. M. (2010). Sensitization of rapid dopamine signaling in the nucleus accumbens core and shell after repeated cocaine in rats. J. Neurophysiol. 104, 922–931. doi: 10.1152/jn.00413.2010
Adeluyi, A., Guerin, L., Fisher, M. L., Galloway, A., Cole, R. D., Chan, S. S. L., et al. (2019). Microglia morphology and proinflammatory signaling in the nucleus accumbens during nicotine withdrawal. Sci. Adv. 5:eaax7031. doi: 10.1126/sciadv.aax7031
Al-Hasani, R., Gowrishankar, R., Schmitz, G. P., Pedersen, C. E., Marcus, D. J., Shirley, S. E., et al. (2021). Ventral tegmental area GABAergic inhibition of cholinergic interneurons in the ventral nucleus accumbens shell promotes reward reinforcement. Nat. Neurosci. 24, 1414–1428. doi: 10.1038/s41593-021-00898-2
Al-Hasani, R., McCall, J. G., Shin, G., Gomez, A. M., Schmitz, G. P., Bernardi, J. M., et al. (2015). Distinct subpopulations of nucleus accumbens dynorphin neurons drive aversion and reward. Neuron 87, 1063–1077. doi: 10.1016/j.neuron.2015.08.019
Almela, P., Navarro-Zaragoza, J., Garcia-Carmona, J. A., Mora, L., Hidalgo, J., Milanes, M. V., et al. (2012). Role of corticotropin-releasing factor (CRF) receptor-1 on the catecholaminergic response to morphine withdrawal in the nucleus accumbens (NAc). PLoS One 7:e47089. doi: 10.1371/journal.pone.0047089
Ambroggi, F., Ishikawa, A., Fields, H. L., and Nicola, S. M. (2008). Basolateral amygdala neurons facilitate reward-seeking behavior by exciting nucleus accumbens neurons. Neuron 59, 648–661. doi: 10.1016/j.neuron.2008.07.004
Anderson, K. D., and Reiner, A. (1990). Extensive co-occurrence of substance P and dynorphin in striatal projection neurons: an evolutionarily conserved feature of basal ganglia organization. J. Comp. Neurol. 295, 339–369. doi: 10.1002/cne.902950302
Aono, Y., Saigusa, T., Watanabe, S., Iwakami, T., Mizoguchi, N., Ikeda, H., et al. (2007). Role of alpha adrenoceptors in the nucleus accumbens in the control of accumbal noradrenaline efflux: a microdialysis study with freely moving rats. J. Neural Transm. (Vienna) 114, 1135–1142. doi: 10.1007/s00702-007-0745-1
Aosaki, T., Kimura, M., and Graybiel, A. M. (1995). Temporal and spatial characteristics of tonically active neurons of the primate's striatum. J. Neurophysiol. 73, 1234–1252. doi: 10.1152/jn.1995.73.3.1234
Avena, N. M., Bocarsly, M. E., Rada, P., Kim, A., and Hoebel, B. G. (2008). After daily bingeing on a sucrose solution, food deprivation induces anxiety and accumbens dopamine/acetylcholine imbalance. Physiol. Behav. 94, 309–315. doi: 10.1016/j.physbeh.2008.01.008
Avena, N. M., and Rada, P. V. (2012). Cholinergic modulation of food and drug satiety and withdrawal. Physiol. Behav. 106, 332–336. doi: 10.1016/j.physbeh.2012.03.020
Baimel, C., Jang, E., Scudder, S. L., Manoocheri, K., and Carter, A. G. (2022). Hippocampal-evoked inhibition of cholinergic interneurons in the nucleus accumbens. Cell Rep. 40:111042. doi: 10.1016/j.celrep.2022.111042
Bakshi, V. P., and Kelley, A. E. (1993). Feeding induced by opioid stimulation of the ventral striatum: role of opiate receptor subtypes. J. Pharmacol. Exp. Ther. 265, 1253–1260
Barnstedt, O., Mocellin, P., and Remy, S. (2024). A hippocampus-accumbens code guides goal-directed appetitive behavior. Nat. Commun. 15:3196. doi: 10.1038/s41467-024-47361-x
Barot, S. K., Ferguson, S. M., and Neumaier, J. F. (2007). 5-HT(1B) receptors in nucleus accumbens efferents enhance both rewarding and aversive effects of cocaine. Eur. J. Neurosci. 25, 3125–3131. doi: 10.1111/j.1460-9568.2007.05568.x
Barrientos, C., Knowland, D., Wu, M. M. J., Lilascharoen, V., Huang, K. W., Malenka, R. C., et al. (2018). Cocaine-induced structural plasticity in input regions to distinct cell types in nucleus Accumbens. Biol. Psychiatry 84, 893–904. doi: 10.1016/j.biopsych.2018.04.019
Basar, K., Sesia, T., Groenewegen, H., Steinbusch, H. W., Visser-Vandewalle, V., and Temel, Y. (2010). Nucleus accumbens and impulsivity. Prog. Neurobiol. 92, 533–557. doi: 10.1016/j.pneurobio.2010.08.007
Bayer, H. M., and Glimcher, P. W. (2005). Midbrain dopamine neurons encode a quantitative reward prediction error signal. Neuron 47, 129–141. doi: 10.1016/j.neuron.2005.05.020
Beas, B. S., Wright, B. J., Skirzewski, M., Leng, Y., Hyun, J. H., Koita, O., et al. (2018). The locus coeruleus drives disinhibition in the midline thalamus via a dopaminergic mechanism. Nat. Neurosci. 21, 963–973. doi: 10.1038/s41593-018-0167-4
Beaulieu, J. M., and Gainetdinov, R. R. (2011). The physiology, signaling, and pharmacology of dopamine receptors. Pharmacol. Rev. 63, 182–217. doi: 10.1124/pr.110.002642
Belilos, A., Gray, C., Sanders, C., Black, D., Mays, E., Richie, C., et al. (2023). Nucleus accumbens local circuit for cue-dependent aversive learning. Cell Rep. 42:113488. doi: 10.1016/j.celrep.2023.113488
Bell, J., and Strang, J. (2020). Medication treatment of opioid use disorder. Biol. Psychiatry 87, 82–88. doi: 10.1016/j.biopsych.2019.06.020
Belleau, M. L., and Warren, R. A. (2000). Postnatal development of electrophysiological properties of nucleus accumbens neurons. J. Neurophysiol. 84, 2204–2216. doi: 10.1152/jn.2000.84.5.2204
Belujon, P., and Grace, A. A. (2014). Restoring mood balance in depression: ketamine reverses deficit in dopamine-dependent synaptic plasticity. Biol. Psychiatry 76, 927–936. doi: 10.1016/j.biopsych.2014.04.014
Bennett, B. D., and Bolam, J. P. (1994). Synaptic input and output of parvalbumin-immunoreactive neurons in the neostriatum of the rat. Neuroscience 62, 707–719. doi: 10.1016/0306-4522(94)90471-5
Berendse, H. W., Galis-de Graaf, Y., and Groenewegen, H. J. (1992a). Topographical organization and relationship with ventral striatal compartments of prefrontal corticostriatal projections in the rat. J. Comp. Neurol. 316, 314–347. doi: 10.1002/cne.903160305
Berendse, H. W., Groenewegen, H. J., and Lohman, A. H. (1992b). Compartmental distribution of ventral striatal neurons projecting to the mesencephalon in the rat. J. Neurosci. 12, 2079–2103. doi: 10.1523/JNEUROSCI.12-06-02079.1992
Bergamini, G., Sigrist, H., Ferger, B., Singewald, N., Seifritz, E., and Pryce, C. R. (2016). Depletion of nucleus accumbens dopamine leads to impaired reward and aversion processing in mice: relevance to motivation pathologies. Neuropharmacology 109, 306–319. doi: 10.1016/j.neuropharm.2016.03.048
Berke, J. D. (2018). What does dopamine mean? Nat. Neurosci. 21, 787–793. doi: 10.1038/s41593-018-0152-y
Berridge, K. C., and Kringelbach, M. L. (2015). Pleasure systems in the brain. Neuron 86, 646–664. doi: 10.1016/j.neuron.2015.02.018
Besson, M. J., Graybiel, A. M., and Quinn, B. (1990). Co-expression of neuropeptides in the cat's striatum: an immunohistochemical study of substance P, dynorphin B and enkephalin. Neuroscience 39, 33–58. doi: 10.1016/0306-4522(90)90220-x
Bewernick, B. H., Hurlemann, R., Matusch, A., Kayser, S., Grubert, C., Hadrysiewicz, B., et al. (2010). Nucleus accumbens deep brain stimulation decreases ratings of depression and anxiety in treatment-resistant depression. Biol. Psychiatry 67, 110–116. doi: 10.1016/j.biopsych.2009.09.013
Bjorklund, A., and Dunnett, S. B. (2007). Dopamine neuron systems in the brain: an update. Trends Neurosci. 30, 194–202. doi: 10.1016/j.tins.2007.03.006
Bocklisch, C., Pascoli, V., Wong, J. C., House, D. R., Yvon, C., de Roo, M., et al. (2013). Cocaine disinhibits dopamine neurons by potentiation of GABA transmission in the ventral tegmental area. Science 341, 1521–1525. doi: 10.1126/science.1237059
Bond, C. W., Trinko, R., Foscue, E., Furman, K., Groman, S. M., Taylor, J. R., et al. (2020). Medial nucleus accumbens projections to the ventral tegmental area control food consumption. J. Neurosci. 40, 4727–4738. doi: 10.1523/JNEUROSCI.3054-18.2020
Borgkvist, A., Malmlof, T., Feltmann, K., Lindskog, M., and Schilstrom, B. (2012). Dopamine in the hippocampus is cleared by the norepinephrine transporter. Int. J. Neuropsychopharmacol. 15, 1–10. doi: 10.1017/S1461145711000812
Bossert, J. M., Marchant, N. J., Calu, D. J., and Shaham, Y. (2013). The reinstatement model of drug relapse: recent neurobiological findings, emerging research topics, and translational research. Psychopharmacology 229, 453–476. doi: 10.1007/s00213-013-3120-y
Bouwknecht, J. A., Hijzen, T. H., van der Gugten, J., Maes, R. A., Hen, R., and Olivier, B. (2001). Absence of 5-HT(1B) receptors is associated with impaired impulse control in male 5-HT(1B) knockout mice. Biol. Psychiatry 49, 557–568. doi: 10.1016/s0006-3223(00)01018-0
Brady, A. M., and O'Donnell, P. (2004). Dopaminergic modulation of prefrontal cortical input to nucleus accumbens neurons in vivo. J. Neurosci. 24, 1040–1049. doi: 10.1523/JNEUROSCI.4178-03.2004
Braun, A. A., Amos-Kroohs, R. M., Gutierrez, A., Lundgren, K. H., Seroogy, K. B., Vorhees, C. V., et al. (2016). 6-Hydroxydopamine-induced dopamine reductions in the nucleus accumbens, but not the medial prefrontal cortex, impair Cincinnati water maze egocentric and Morris water maze allocentric navigation in male Sprague-Dawley rats. Neurotox. Res. 30, 199–212. doi: 10.1007/s12640-016-9616-6
Britt, J. P., Benaliouad, F., McDevitt, R. A., Stuber, G. D., Wise, R. A., and Bonci, A. (2012). Synaptic and behavioral profile of multiple glutamatergic inputs to the nucleus accumbens. Neuron 76, 790–803. doi: 10.1016/j.neuron.2012.09.040
Britt, J. P., and McGehee, D. S. (2008). Presynaptic opioid and nicotinic receptor modulation of dopamine overflow in the nucleus accumbens. J. Neurosci. 28, 1672–1681. doi: 10.1523/JNEUROSCI.4275-07.2008
Broderick, P. A., Hope, O., Okonji, C., Rahni, D. N., and Zhou, Y. (2004). Clozapine and cocaine effects on dopamine and serotonin release in nucleus accumbens during psychostimulant behavior and withdrawal. Prog. Neuro Psychopharmacol. Biol. Psychiatry 28, 157–171. doi: 10.1016/j.pnpbp.2003.09.032
Broderick, P. A., Rahni, D. N., and Zhou, Y. (2003). Acute and subacute effects of risperidone and cocaine on accumbens dopamine and serotonin release using in vivo microvoltammetry on line with open-field behavior. Prog. Neuro Psychopharmacol. Biol. Psychiatry 27, 1037–1054. doi: 10.1016/S0278-5846(03)00176-3
Brown, P., and Molliver, M. E. (2000). Dual serotonin (5-HT) projections to the nucleus accumbens core and shell: relation of the 5-HT transporter to amphetamine-induced neurotoxicity. J. Neurosci. 20, 1952–1963. doi: 10.1523/JNEUROSCI.20-05-01952.2000
Brown, M. T., Tan, K. R., O'Connor, E. C., Nikonenko, I., Muller, D., and Luscher, C. (2012). Ventral tegmental area GABA projections pause accumbal cholinergic interneurons to enhance associative learning. Nature 492, 452–456. doi: 10.1038/nature11657
Browne, C. J., Abela, A. R., Chu, D., Li, Z., Ji, X., Lambe, E. K., et al. (2019). Dorsal raphe serotonin neurons inhibit operant responding for reward via inputs to the ventral tegmental area but not the nucleus accumbens: evidence from studies combining optogenetic stimulation and serotonin reuptake inhibition. Neuropsychopharmacology 44, 793–804. doi: 10.1038/s41386-018-0271-x
Burke, D. A., and Alvarez, V. A. (2022). Serotonin receptors contribute to dopamine depression of lateral inhibition in the nucleus accumbens. Cell Rep. 39:110795. doi: 10.1016/j.celrep.2022.110795
Burke, D. A., Rotstein, H. G., and Alvarez, V. A. (2017). Striatal local circuitry: a new framework for lateral inhibition. Neuron 96, 267–284. doi: 10.1016/j.neuron.2017.09.019
Cabib, S., and Puglisi-Allegra, S. (2012). The mesoaccumbens dopamine in coping with stress. Neurosci. Biobehav. Rev. 36, 79–89. doi: 10.1016/j.neubiorev.2011.04.012
Cachope, R., Mateo, Y., Mathur, B. N., Irving, J., Wang, H. L., Morales, M., et al. (2012). Selective activation of cholinergic interneurons enhances accumbal phasic dopamine release: setting the tone for reward processing. Cell Rep. 2, 33–41. doi: 10.1016/j.celrep.2012.05.011
Caine, S. B., Negus, S. S., Mello, N. K., Patel, S., Bristow, L., Kulagowski, J., et al. (2002). Role of dopamine D2-like receptors in cocaine self-administration: studies with D2 receptor mutant mice and novel D2 receptor antagonists. J. Neurosci. 22, 2977–2988. doi: 10.1523/JNEUROSCI.22-07-02977.2002
Caine, S. B., Thomsen, M., Gabriel, K. I., Berkowitz, J. S., Gold, L. H., Koob, G. F., et al. (2007). Lack of self-administration of cocaine in dopamine D1 receptor knock-out mice. J. Neurosci. 27, 13140–13150. doi: 10.1523/JNEUROSCI.2284-07.2007
Calabrese, E. J. (2008). Addiction and dose response: the psychomotor stimulant theory of addiction reveals that hormetic dose responses are dominant. Crit. Rev. Toxicol. 38, 599–617. doi: 10.1080/10408440802026315
Calcia, M. A., Bonsall, D. R., Bloomfield, P. S., Selvaraj, S., Barichello, T., and Howes, O. D. (2016). Stress and neuroinflammation: a systematic review of the effects of stress on microglia and the implications for mental illness. Psychopharmacology 233, 1637–1650. doi: 10.1007/s00213-016-4218-9
Calder, A. E., and Hasler, G. (2023). Towards an understanding of psychedelic-induced neuroplasticity. Neuropsychopharmacology 48, 104–112. doi: 10.1038/s41386-022-01389-z
Calipari, E. S., Bagot, R. C., Purushothaman, I., Davidson, T. J., Yorgason, J. T., Pena, C. J., et al. (2016). In vivo imaging identifies temporal signature of D1 and D2 medium spiny neurons in cocaine reward. Proc. Natl. Acad. Sci. USA 113, 2726–2731. doi: 10.1073/pnas.1521238113
Calipari, E. S., Ferris, M. J., Zimmer, B. A., Roberts, D. C., and Jones, S. R. (2013). Temporal pattern of cocaine intake determines tolerance vs sensitization of cocaine effects at the dopamine transporter. Neuropsychopharmacology 38, 2385–2392. doi: 10.1038/npp.2013.136
Canseco-Alba, A., Coffeen, U., Jaimes, O., Pellicer, F., and Rodriguez-Manzo, G. (2022). The nucleus accumbens dopamine increase, typically triggered by sexual stimuli in male rats, is no longer produced when animals are sexually inhibited due to sexual satiety. Psychopharmacology 239, 3679–3695. doi: 10.1007/s00213-022-06240-3
Carboni, E., and Carta, A. R. (2022). BDNF alterations in brain areas and the neurocircuitry involved in the antidepressant effects of ketamine in animal models, suggest the existence of a primary circuit of depression. J. Integr. Neurosci. 21:144. doi: 10.31083/j.jin2105144
Carelli, R. M. (2004). Nucleus accumbens cell firing and rapid dopamine signaling during goal-directed behaviors in rats. Neuropharmacology 47, 180–189. doi: 10.1016/j.neuropharm.2004.07.017
Castro, D. C., and Bruchas, M. R. (2019). A motivational and neuropeptidergic hub: anatomical and functional diversity within the nucleus accumbens shell. Neuron 102, 529–552. doi: 10.1016/j.neuron.2019.03.003
Castro, D. C., Oswell, C. S., Zhang, E. T., Pedersen, C. E., Piantadosi, S. C., Rossi, M. A., et al. (2021). An endogenous opioid circuit determines state-dependent reward consumption. Nature 598, 646–651. doi: 10.1038/s41586-021-04013-0
Cathomas, F., Murrough, J. W., Nestler, E. J., Han, M. H., and Russo, S. J. (2019). Neurobiology of resilience: Interface between mind and body. Biol. Psychiatry 86, 410–420. doi: 10.1016/j.biopsych.2019.04.011
Chandra, R., Francis, T. C., Nam, H., Riggs, L. M., Engeln, M., Rudzinskas, S., et al. (2017). Reduced Slc6a15 in nucleus accumbens D2-neurons underlies stress susceptibility. J. Neurosci. 37, 6527–6538. doi: 10.1523/JNEUROSCI.3250-16.2017
Chang, C. Y., Esber, G. R., Marrero-Garcia, Y., Yau, H. J., Bonci, A., and Schoenbaum, G. (2016). Brief optogenetic inhibition of dopamine neurons mimics endogenous negative reward prediction errors. Nat. Neurosci. 19, 111–116. doi: 10.1038/nn.4191
Chang, R., Peng, J., Chen, Y., Liao, H., Zhao, S., Zou, J., et al. (2022). Deep brain stimulation in drug addiction treatment: research Progress and perspective. Front. Psych. 13:858638. doi: 10.3389/fpsyt.2022.858638
Chantranupong, L., Beron, C. C., Zimmer, J. A., Wen, M. J., Wang, W., and Sabatini, B. L. (2023). Dopamine and glutamate regulate striatal acetylcholine in decision-making. Nature 621, 577–585. doi: 10.1038/s41586-023-06492-9
Chartoff, E. H., Mague, S. D., Barhight, M. F., Smith, A. M., and Carlezon, W. A. Jr. (2006). Behavioral and molecular effects of dopamine D1 receptor stimulation during naloxone-precipitated morphine withdrawal. J. Neurosci. 26, 6450–6457. doi: 10.1523/JNEUROSCI.0491-06.2006
Chaudhury, D., Walsh, J. J., Friedman, A. K., Juarez, B., Ku, S. M., Koo, J. W., et al. (2013). Rapid regulation of depression-related behaviours by control of midbrain dopamine neurons. Nature 493, 532–536. doi: 10.1038/nature11713
Chellian, R., Behnood-Rod, A., Wilson, R., Lin, K., King, G. W., Ruppert-Gomez, M., et al. (2022). Dopamine D1-like receptor blockade and stimulation decreases operant responding for nicotine and food in male and female rats. Sci. Rep. 12:14131. doi: 10.1038/s41598-022-18081-3
Chen, R., Blosser, T. R., Djekidel, M. N., Hao, J., Bhattacherjee, A., Chen, W., et al. (2021). Decoding molecular and cellular heterogeneity of mouse nucleus accumbens. Nat. Neurosci. 24, 1757–1771. doi: 10.1038/s41593-021-00938-x
Chen, G., Lai, S., Bao, G., Ke, J., Meng, X., Lu, S., et al. (2023). Distinct reward processing by subregions of the nucleus accumbens. Cell Rep. 42:112069. doi: 10.1016/j.celrep.2023.112069
Chen, R., Tilley, M. R., Wei, H., Zhou, F., Zhou, F. M., Ching, S., et al. (2006). Abolished cocaine reward in mice with a cocaine-insensitive dopamine transporter. Proc. Natl. Acad. Sci. U. S. A. 103, 9333–9338. doi: 10.1073/pnas.0600905103
Chen, B. T., Yau, H. J., Hatch, C., Kusumoto-Yoshida, I., Cho, S. L., Hopf, F. W., et al. (2013). Rescuing cocaine-induced prefrontal cortex hypoactivity prevents compulsive cocaine seeking. Nature 496, 359–362. doi: 10.1038/nature12024
Choi, J. E., Choi, D. I., Lee, J., Kim, J., Kim, M. J., Hong, I., et al. (2022). Synaptic ensembles between raphe and D(1)R-containing accumbens shell neurons underlie postisolation sociability in males. Sci. Adv. 8:eabo7527. doi: 10.1126/sciadv.abo7527
Christoffel, D. J., Walsh, J. J., Heifets, B. D., Hoerbelt, P., Neuner, S., Sun, G., et al. (2021a). Input-specific modulation of murine nucleus accumbens differentially regulates hedonic feeding. Nat. Commun. 12:2135. doi: 10.1038/s41467-021-22430-7
Christoffel, D. J., Walsh, J. J., Hoerbelt, P., Heifets, B. D., Llorach, P., Lopez, R. C., et al. (2021b). Selective filtering of excitatory inputs to nucleus accumbens by dopamine and serotonin. Proc. Natl. Acad. Sci. U. S. A. 118:e2106648118. doi: 10.1073/pnas.2106648118
Chuhma, N., Mingote, S., Moore, H., and Rayport, S. (2014). Dopamine neurons control striatal cholinergic neurons via regionally heterogeneous dopamine and glutamate signaling. Neuron 81, 901–912. doi: 10.1016/j.neuron.2013.12.027
Cole, S. L., Robinson, M. J. F., and Berridge, K. C. (2018). Optogenetic self-stimulation in the nucleus accumbens: D1 reward versus D2 ambivalence. PLoS One 13:e0207694. doi: 10.1371/journal.pone.0207694
Collins, A. L., Aitken, T. J., Greenfield, V. Y., Ostlund, S. B., and Wassum, K. M. (2016). Nucleus accumbens acetylcholine receptors modulate dopamine and motivation. Neuropsychopharmacology 41, 2830–2838. doi: 10.1038/npp.2016.81
Collins, A. L., Aitken, T. J., Huang, I. W., Shieh, C., Greenfield, V. Y., Monbouquette, H. G., et al. (2019). Nucleus accumbens cholinergic interneurons oppose cue-motivated behavior. Biol. Psychiatry 86, 388–396. doi: 10.1016/j.biopsych.2019.02.014
Congestri, F., Formenti, F., Sonntag, V., Hdou, G., and Crespi, F. (2008). Selective D3 receptor antagonist SB-277011-a potentiates the effect of cocaine on extracellular dopamine in the nucleus accumbens: a dual core-shell voltammetry study in anesthetized rats. Sensors 8, 6936–6951. doi: 10.3390/s8116936
Conrad, K. L., Tseng, K. Y., Uejima, J. L., Reimers, J. M., Heng, L. J., Shaham, Y., et al. (2008). Formation of accumbens GluR2-lacking AMPA receptors mediates incubation of cocaine craving. Nature 454, 118–121. doi: 10.1038/nature06995
Corkrum, M., Covelo, A., Lines, J., Bellocchio, L., Pisansky, M., Loke, K., et al. (2020). Dopamine-evoked synaptic regulation in the nucleus accumbens requires astrocyte activity. Neuron 105, 1036–1047.e5. doi: 10.1016/j.neuron.2019.12.026
Corre, J., van Zessen, R., Loureiro, M., Patriarchi, T., Tian, L., Pascoli, V., et al. (2018). Dopamine neurons projecting to medial shell of the nucleus accumbens drive heroin reinforcement. Elife 7:e39945. doi: 10.7554/eLife.39945
Correia, S. S., McGrath, A. G., Lee, A., Graybiel, A. M., and Goosens, K. A. (2016). Amygdala-ventral striatum circuit activation decreases long-term fear. Elife 5:e12669. doi: 10.7554/eLife.12669
Crespo, J. A., Stockl, P., Zorn, K., Saria, A., and Zernig, G. (2008). Nucleus accumbens core acetylcholine is preferentially activated during acquisition of drug- vs food-reinforced behavior. Neuropsychopharmacology 33, 3213–3220. doi: 10.1038/npp.2008.48
Crespo, J. A., Sturm, K., Saria, A., and Zernig, G. (2006). Activation of muscarinic and nicotinic acetylcholine receptors in the nucleus accumbens core is necessary for the acquisition of drug reinforcement. J. Neurosci. 26, 6004–6010. doi: 10.1523/JNEUROSCI.4494-05.2006
Dai, B., Sun, F., Tong, X., Ding, Y., Kuang, A., Osakada, T., et al. (2022). Responses and functions of dopamine in nucleus accumbens core during social behaviors. Cell Rep. 40:111246. doi: 10.1016/j.celrep.2022.111246
Danjo, T., Yoshimi, K., Funabiki, K., Yawata, S., and Nakanishi, S. (2014). Aversive behavior induced by optogenetic inactivation of ventral tegmental area dopamine neurons is mediated by dopamine D2 receptors in the nucleus accumbens. Proc. Natl. Acad. Sci. U. S. A. 111, 6455–6460. doi: 10.1073/pnas.1404323111
D'Ascenzo, M., Podda, M. V., Fellin, T., Azzena, G. B., Haydon, P., and Grassi, C. (2009). Activation of mGluR5 induces spike afterdepolarization and enhanced excitability in medium spiny neurons of the nucleus accumbens by modulating persistent Na+ currents. J. Physiol. 587, 3233–3250. doi: 10.1113/jphysiol.2009.172593
Day, J. J., Roitman, M. F., Wightman, R. M., and Carelli, R. M. (2007). Associative learning mediates dynamic shifts in dopamine signaling in the nucleus accumbens. Nat. Neurosci. 10, 1020–1028. doi: 10.1038/nn1923
de Jong, J. W., Afjei, S. A., Pollak Dorocic, I., Peck, J. R., Liu, C., Kim, C. K., et al. (2019). A neural circuit mechanism for encoding aversive stimuli in the mesolimbic dopamine system. Neuron 101, 133–151.e7. doi: 10.1016/j.neuron.2018.11.005
de Jong, J. W., Fraser, K. M., and Lammel, S. (2022). Mesoaccumbal dopamine heterogeneity: what do dopamine firing and release have to do with it? Annu. Rev. Neurosci. 45, 109–129. doi: 10.1146/annurev-neuro-110920-011929
Delfs, J. M., Zhu, Y., Druhan, J. P., and Aston-Jones, G. S. (1998). Origin of noradrenergic afferents to the shell subregion of the nucleus accumbens: anterograde and retrograde tract-tracing studies in the rat. Brain Res. 806, 127–140. doi: 10.1016/s0006-8993(98)00672-6
Di Chiara, G. (2002). Nucleus accumbens shell and core dopamine: differential role in behavior and addiction. Behav. Brain Res. 137, 75–114. doi: 10.1016/s0166-4328(02)00286-3
Di Chiara, G., and Imperato, A. (1986). Preferential stimulation of dopamine release in the nucleus accumbens by opiates, alcohol, and barbiturates: studies with transcerebral dialysis in freely moving rats. Ann. N. Y. Acad. Sci. 473, 367–381. doi: 10.1111/j.1749-6632.1986.tb23629.x
Di Chiara, G., Loddo, P., and Tanda, G. (1999). Reciprocal changes in prefrontal and limbic dopamine responsiveness to aversive and rewarding stimuli after chronic mild stress: implications for the psychobiology of depression. Biol. Psychiatry 46, 1624–1633. doi: 10.1016/s0006-3223(99)00236-x
Dobbs, L. K., Kaplan, A. R., Lemos, J. C., Matsui, A., Rubinstein, M., and Alvarez, V. A. (2016). Dopamine regulation of lateral inhibition between striatal neurons gates the stimulant actions of cocaine. Neuron 90, 1100–1113. doi: 10.1016/j.neuron.2016.04.031
Dolen, G., Darvishzadeh, A., Huang, K. W., and Malenka, R. C. (2013). Social reward requires coordinated activity of nucleus accumbens oxytocin and serotonin. Nature 501, 179–184. doi: 10.1038/nature12518
Domingo-Rodriguez, L., Ruiz de Azua, I., Dominguez, E., Senabre, E., Serra, I., Kummer, S., et al. (2020). A specific prelimbic-nucleus accumbens pathway controls resilience versus vulnerability to food addiction. Nat. Commun. 11:782. doi: 10.1038/s41467-020-14458-y
Dong, Y., Li, Y., Xiang, X., Xiao, Z. C., Hu, J., Li, Y., et al. (2023). Stress relief as a natural resilience mechanism against depression-like behaviors. Neuron 111, 3789–3801.e6. doi: 10.1016/j.neuron.2023.09.004
Duman, R. S., Sanacora, G., and Krystal, J. H. (2019). Altered connectivity in depression: GABA and glutamate neurotransmitter deficits and reversal by novel treatments. Neuron 102, 75–90. doi: 10.1016/j.neuron.2019.03.013
Edwards, N. J., Tejeda, H. A., Pignatelli, M., Zhang, S., McDevitt, R. A., Wu, J., et al. (2017). Circuit specificity in the inhibitory architecture of the VTA regulates cocaine-induced behavior. Nat. Neurosci. 20, 438–448. doi: 10.1038/nn.4482
Engelke, D. S., Zhang, X. O., O'Malley, J. J., Fernandez-Leon, J. A., Li, S., Kirouac, G. J., et al. (2021). A hypothalamic-thalamostriatal circuit that controls approach-avoidance conflict in rats. Nat. Commun. 12:2517. doi: 10.1038/s41467-021-22730-y
English, D. F., Ibanez-Sandoval, O., Stark, E., Tecuapetla, F., Buzsaki, G., Deisseroth, K., et al. (2011). GABAergic circuits mediate the reinforcement-related signals of striatal cholinergic interneurons. Nat. Neurosci. 15, 123–130. doi: 10.1038/nn.2984
Everitt, B. J., and Robbins, T. W. (2005). Neural systems of reinforcement for drug addiction: from actions to habits to compulsion. Nat. Neurosci. 8, 1481–1489. doi: 10.1038/nn1579
Felger, J. C., and Treadway, M. T. (2017). Inflammation effects on motivation and motor activity: role of dopamine. Neuropsychopharmacology 42, 216–241. doi: 10.1038/npp.2016.143
Feng, J., Zhang, C., Lischinsky, J. E., Jing, M., Zhou, J., Wang, H., et al. (2019). A genetically encoded fluorescent sensor for rapid and specific in vivo detection of norepinephrine. Neuron 102, 745–761.e8. doi: 10.1016/j.neuron.2019.02.037
Ferguson, S. M., Mitchell, E. S., and Neumaier, J. F. (2008). Increased expression of 5-HT6 receptors in the nucleus accumbens blocks the rewarding but not psychomotor activating properties of cocaine. Biol. Psychiatry 63, 207–213. doi: 10.1016/j.biopsych.2007.02.018
Ferris, M. J., Calipari, E. S., Mateo, Y., Melchior, J. R., Roberts, D. C., and Jones, S. R. (2012). Cocaine self-administration produces pharmacodynamic tolerance: differential effects on the potency of dopamine transporter blockers, releasers, and methylphenidate. Neuropsychopharmacology 37, 1708–1716. doi: 10.1038/npp.2012.17
Ferris, M. J., Mateo, Y., Roberts, D. C., and Jones, S. R. (2011). Cocaine-insensitive dopamine transporters with intact substrate transport produced by self-administration. Biol. Psychiatry 69, 201–207. doi: 10.1016/j.biopsych.2010.06.026
Floresco, S. B. (2015). The nucleus accumbens: an interface between cognition, emotion, and action. Annu. Rev. Psychol. 66, 25–52. doi: 10.1146/annurev-psych-010213-115159
Floresco, S. B., Montes, D. R., Tse, M. M. T., and van Holstein, M. (2018). Differential contributions of nucleus accumbens subregions to cue-guided risk/reward decision making and implementation of conditional rules. J. Neurosci. 38, 1901–1914. doi: 10.1523/JNEUROSCI.3191-17.2018
Floresco, S. B., West, A. R., Ash, B., Moore, H., and Grace, A. A. (2003). Afferent modulation of dopamine neuron firing differentially regulates tonic and phasic dopamine transmission. Nat. Neurosci. 6, 968–973. doi: 10.1038/nn1103
Floresco, S. B., Yang, C. R., Phillips, A. G., and Blaha, C. D. (1998). Basolateral amygdala stimulation evokes glutamate receptor-dependent dopamine efflux in the nucleus accumbens of the anaesthetized rat. Eur. J. Neurosci. 10, 1241–1251. doi: 10.1046/j.1460-9568.1998.00133.x
Fontaine, H. M., Silva, P. R., Neiswanger, C., Tran, R., Abraham, A. D., Land, B. B., et al. (2022). Stress decreases serotonin tone in the nucleus accumbens in male mice to promote aversion and potentiate cocaine preference via decreased stimulation of 5-HT(1B) receptors. Neuropsychopharmacology 47, 891–901. doi: 10.1038/s41386-021-01178-0
Fox, M. E., Figueiredo, A., Menken, M. S., and Lobo, M. K. (2020). Dendritic spine density is increased on nucleus accumbens D2 neurons after chronic social defeat. Sci. Rep. 10:12393. doi: 10.1038/s41598-020-69339-7
Fox, M. E., Wulff, A. B., Franco, D., Choi, E. Y., Calarco, C. A., Engeln, M., et al. (2023). Adaptations in nucleus accumbens neuron subtypes mediate negative affective behaviors in fentanyl abstinence. Biol. Psychiatry 93, 489–501. doi: 10.1016/j.biopsych.2022.08.023
Francis, T. C., Chandra, R., Friend, D. M., Finkel, E., Dayrit, G., Miranda, J., et al. (2015). Nucleus accumbens medium spiny neuron subtypes mediate depression-related outcomes to social defeat stress. Biol. Psychiatry 77, 212–222. doi: 10.1016/j.biopsych.2014.07.021
Francis, T. C., Yano, H., Demarest, T. G., Shen, H., and Bonci, A. (2019). High-frequency activation of nucleus accumbens D1-MSNs drives excitatory potentiation on D2-MSNs. Neuron 103, 432–444.e3. doi: 10.1016/j.neuron.2019.05.031
Friedman, A. K., Walsh, J. J., Juarez, B., Ku, S. M., Chaudhury, D., Wang, J., et al. (2014). Enhancing depression mechanisms in midbrain dopamine neurons achieves homeostatic resilience. Science 344, 313–319. doi: 10.1126/science.1249240
Gallegos, D. A., Minto, M., Liu, F., Hazlett, M. F., Aryana Yousefzadeh, S., Bartelt, L. C., et al. (2022). Cell-type specific transcriptional adaptations of nucleus accumbens interneurons to amphetamine. Mol. Psychiatry 28, 3414–3428. doi: 10.1038/s41380-022-01466-1
Gallo, E. F., Greenwald, J., Yeisley, J., Teboul, E., Martyniuk, K. M., Villarin, J. M., et al. (2022). Dopamine D2 receptors modulate the cholinergic pause and inhibitory learning. Mol. Psychiatry 27, 1502–1514. doi: 10.1038/s41380-021-01364-y
Gallo, E. F., Meszaros, J., Sherman, J. D., Chohan, M. O., Teboul, E., Choi, C. S., et al. (2018). Accumbens dopamine D2 receptors increase motivation by decreasing inhibitory transmission to the ventral pallidum. Nat. Commun. 9:1086. doi: 10.1038/s41467-018-03272-2
Ghitza, U. E., Fabbricatore, A. T., Prokopenko, V., Pawlak, A. P., and West, M. O. (2003). Persistent cue-evoked activity of accumbens neurons after prolonged abstinence from self-administered cocaine. J. Neurosci. 23, 7239–7245. doi: 10.1523/JNEUROSCI.23-19-07239.2003
Giannotti, G., Gong, S., Fayette, N., Heinsbroek, J. A., Orfila, J. E., Herson, P. S., et al. (2021). Extinction blunts paraventricular thalamic contributions to heroin relapse. Cell Rep. 36:109605. doi: 10.1016/j.celrep.2021.109605
Gipson, C. D., Kupchik, Y. M., Shen, H., Reissner, K. J., Thomas, C. A., and Kalivas, P. W. (2013). Relapse induced by cues predicting cocaine depends on rapid, transient synaptic potentiation. Neuron 77, 867–872. doi: 10.1016/j.neuron.2013.01.005
Goedhoop, J. N., van den Boom, B. J. G., Robke, R., Veen, F., Fellinger, L., van Elzelingen, W., et al. (2022). Nucleus accumbens dopamine tracks aversive stimulus duration and prediction but not value or prediction error. Elife 11:e82711. doi: 10.7554/eLife.82711
Goldberg, J. A., and Reynolds, J. N. (2011). Spontaneous firing and evoked pauses in the tonically active cholinergic interneurons of the striatum. Neuroscience 198, 27–43. doi: 10.1016/j.neuroscience.2011.08.067
Gomez-Milanes, I., Almela, P., Garcia-Carmona, J. A., Garcia-Gutierrez, M. S., Aracil-Fernandez, A., Manzanares, J., et al. (2012). Accumbal dopamine, noradrenaline and serotonin activity after naloxone-conditioned place aversion in morphine-dependent mice. Neurochem. Int. 61, 433–440. doi: 10.1016/j.neuint.2012.06.011
Gore, B. B., and Zweifel, L. S. (2013). Genetic reconstruction of dopamine D1 receptor signaling in the nucleus accumbens facilitates natural and drug reward responses. J. Neurosci. 33, 8640–8649. doi: 10.1523/JNEUROSCI.5532-12.2013
Goto, Y., and Grace, A. A. (2005). Dopaminergic modulation of limbic and cortical drive of nucleus accumbens in goal-directed behavior. Nat. Neurosci. 8, 805–812. doi: 10.1038/nn1471
Graziane, N. M., Sun, S., Wright, W. J., Jang, D., Liu, Z., Huang, Y. H., et al. (2016). Opposing mechanisms mediate morphine- and cocaine-induced generation of silent synapses. Nat. Neurosci. 19, 915–925. doi: 10.1038/nn.4313
Groenewegen, H. J., Wright, C. I., Beijer, A. V., and Voorn, P. (1999). Convergence and segregation of ventral striatal inputs and outputs. Ann. N. Y. Acad. Sci. 877, 49–63. doi: 10.1111/j.1749-6632.1999.tb09260.x
Guillaumin, M. C. C., Viskaitis, P., Bracey, E., Burdakov, D., and Peleg-Raibstein, D. (2023). Disentangling the role of NAc D1 and D2 cells in hedonic eating. Mol. Psychiatry 28, 3531–3547. doi: 10.1038/s41380-023-02131-x
Gunaydin, L. A., Grosenick, L., Finkelstein, J. C., Kauvar, I. V., Fenno, L. E., Adhikari, A., et al. (2014). Natural neural projection dynamics underlying social behavior. Cell 157, 1535–1551. doi: 10.1016/j.cell.2014.05.017
Haber, S. N., and Knutson, B. (2010). The reward circuit: linking primate anatomy and human imaging. Neuropsychopharmacology 35, 4–26. doi: 10.1038/npp.2009.129
Hajnal, A., and Norgren, R. (2004). Sucrose sham feeding decreases accumbens norepinephrine in the rat. Physiol. Behav. 82, 43–47. doi: 10.1016/j.physbeh.2004.04.024
Hall, F. S., Sora, I., Drgonova, J., Li, X. F., Goeb, M., and Uhl, G. R. (2004). Molecular mechanisms underlying the rewarding effects of cocaine. Ann. N. Y. Acad. Sci. 1025, 47–56. doi: 10.1196/annals.1316.006
Hamid, A. A., Pettibone, J. R., Mabrouk, O. S., Hetrick, V. L., Schmidt, R., Vander Weele, C. M., et al. (2016). Mesolimbic dopamine signals the value of work. Nat. Neurosci. 19, 117–126. doi: 10.1038/nn.4173
Harmson, O., Grima, L. L., Panayi, M. C., Husain, M., and Walton, M. E. (2022). 5-HT(2C) receptor perturbation has bidirectional influence over instrumental vigour and restraint. Psychopharmacology 239, 123–140. doi: 10.1007/s00213-021-05992-8
Harris, H. N., and Peng, Y. B. (2020). Evidence and explanation for the involvement of the nucleus accumbens in pain processing. Neural Regen. Res. 15, 597–605. doi: 10.4103/1673-5374.266909
Hauser, S. R., Deehan, G. A. Jr., Dhaher, R., Knight, C. P., Wilden, J. A., McBride, W. J., et al. (2015). D1 receptors in the nucleus accumbens-shell, but not the core, are involved in mediating ethanol-seeking behavior of alcohol-preferring (P) rats. Neuroscience 295, 243–251. doi: 10.1016/j.neuroscience.2015.03.030
Hayes, D. J., and Greenshaw, A. J. (2011). 5-HT receptors and reward-related behaviour: a review. Neurosci. Biobehav. Rev. 35, 1419–1449. doi: 10.1016/j.neubiorev.2011.03.005
Hazlett, M. F., Hall, V. L., Patel, E., Halvorsen, A., Calakos, N., and West, A. E. (2024). The perineuronal net protein brevican acts in nucleus accumbens parvalbumin-expressing interneurons of adult mice to regulate excitatory synaptic inputs and motivated behaviors. Biol. Psychiatry. doi: 10.1016/j.biopsych.2024.02.003
He, G., Huai, Z., Jiang, C., Huang, B., Tian, Z., Le, Q., et al. (2023). Persistent increase of accumbens cocaine ensemble excitability induced by IRK downregulation after withdrawal mediates the incubation of cocaine craving. Mol. Psychiatry 28, 448–462. doi: 10.1038/s41380-022-01884-1
He, Y., Wang, J., Li, K. L., Wang, Y. Q., Freyberg, Z., and Dong, Y. (2023). Membrane excitability of nucleus accumbens neurons gates the incubation of cocaine craving. Neuropsychopharmacology 48, 1318–1327. doi: 10.1038/s41386-023-01580-w
He, Z. X., Xi, K., Liu, K. J., Yue, M. H., Wang, Y., Yin, Y. Y., et al. (2023). A nucleus accumbens Tac1 neural circuit regulates avoidance responses to aversive stimuli. Int. J. Mol. Sci. 24:4346. doi: 10.3390/ijms24054346
He, Z. X., Yin, Y. Y., Xi, K., Xing, Z. K., Cao, J. B., Liu, T. Y., et al. (2020). Nucleus Accumbens Tac1-expressing neurons mediate stress-induced anhedonia-like behavior in mice. Cell Rep. 33:108343. doi: 10.1016/j.celrep.2020.108343
Heifets, B. D., Salgado, J. S., Taylor, M. D., Hoerbelt, P., Cardozo Pinto, D. F., Steinberg, E. E., et al. (2019). Distinct neural mechanisms for the prosocial and rewarding properties of MDMA. Sci. Transl. Med. 11:eaaw6435. doi: 10.1126/scitranslmed.aaw6435
Heimer, L., Zahm, D. S., Churchill, L., Kalivas, P. W., and Wohltmann, C. (1991). Specificity in the projection patterns of accumbal core and shell in the rat. Neuroscience 41, 89–125. doi: 10.1016/0306-4522(91)90202-y
Heinsbroek, J. A., Giannotti, G., Mandel, M. R., Josey, M., Aston-Jones, G., James, M. H., et al. (2021). A common limiter circuit for opioid choice and relapse identified in a rodent addiction model. Nat. Commun. 12:4788. doi: 10.1038/s41467-021-25080-x
Helm, K. A., Rada, P., and Hoebel, B. G. (2003). Cholecystokinin combined with serotonin in the hypothalamus limits accumbens dopamine release while increasing acetylcholine: a possible satiation mechanism. Brain Res. 963, 290–297. doi: 10.1016/s0006-8993(02)04051-9
Heshmati, M., Christoffel, D. J., LeClair, K., Cathomas, F., Golden, S. A., Aleyasin, H., et al. (2020). Depression and social defeat stress are associated with inhibitory synaptic changes in the nucleus accumbens. J. Neurosci. 40, 6228–6233. doi: 10.1523/JNEUROSCI.2568-19.2020
Hikida, T., Kimura, K., Wada, N., Funabiki, K., and Nakanishi, S. (2010). Distinct roles of synaptic transmission in direct and indirect striatal pathways to reward and aversive behavior. Neuron 66, 896–907. doi: 10.1016/j.neuron.2010.05.011
Hobson, B. D., O'Neill, C. E., Levis, S. C., Monteggia, L. M., Neve, R. L., Self, D. W., et al. (2013). Adenosine A1 and dopamine d1 receptor regulation of AMPA receptor phosphorylation and cocaine-seeking behavior. Neuropsychopharmacology 38, 1974–1983. doi: 10.1038/npp.2013.96
Hoebel, B. G., Avena, N. M., and Rada, P. (2007). Accumbens dopamine-acetylcholine balance in approach and avoidance. Curr. Opin. Pharmacol. 7, 617–627. doi: 10.1016/j.coph.2007.10.014
Hu, H. (2016). Reward and aversion. Annu. Rev. Neurosci. 39, 297–324. doi: 10.1146/annurev-neuro-070815-014106
Huang, Y., Ceceli, A. O., Kronberg, G., King, S., Malaker, P., Parvaz, M. A., et al. (2024). Association of cortico-striatal engagement during cue reactivity, reappraisal, and savoring of drug and non-drug stimuli with craving in heroin addiction. Am. J. Psychiatry 181, 153–165. doi: 10.1176/appi.ajp.20220759
Huang, Y. H., Lin, Y., Mu, P., Lee, B. R., Brown, T. E., Wayman, G., et al. (2009). In vivo cocaine experience generates silent synapses. Neuron 63, 40–47. doi: 10.1016/j.neuron.2009.06.007
Ibanez-Sandoval, O., Tecuapetla, F., Unal, B., Shah, F., Koos, T., and Tepper, J. M. (2011). A novel functionally distinct subtype of striatal neuropeptide Y interneuron. J. Neurosci. 31, 16757–16769. doi: 10.1523/JNEUROSCI.2628-11.2011
Ibrahim, K. M., Massaly, N., Yoon, H. J., Sandoval, R., Widman, A. J., Heuermann, R. J., et al. (2024). Dorsal hippocampus to nucleus accumbens projections drive reinforcement via activation of accumbal dynorphin neurons. Nat. Commun. 15:750. doi: 10.1038/s41467-024-44836-9
Iino, Y., Sawada, T., Yamaguchi, K., Tajiri, M., Ishii, S., Kasai, H., et al. (2020). Dopamine D2 receptors in discrimination learning and spine enlargement. Nature 579, 555–560. doi: 10.1038/s41586-020-2115-1
Ikemoto, S., Glazier, B. S., Murphy, J. M., and McBride, W. J. (1997). Role of dopamine D1 and D2 receptors in the nucleus accumbens in mediating reward. J. Neurosci. 17, 8580–8587. doi: 10.1523/JNEUROSCI.17-21-08580.1997
Ito, R., and Hayen, A. (2011). Opposing roles of nucleus accumbens core and shell dopamine in the modulation of limbic information processing. J. Neurosci. 31, 6001–6007. doi: 10.1523/JNEUROSCI.6588-10.2011
Jeong, H., Taylor, A., Floeder, J. R., Lohmann, M., Mihalas, S., Wu, B., et al. (2022). Mesolimbic dopamine release conveys causal associations. Science 378:eabq6740. doi: 10.1126/science.abq6740
Jing, M. Y., Ding, X. Y., Han, X., Zhao, T. Y., Luo, M. M., Wu, N., et al. (2022). Activation of mesocorticolimbic dopamine projections initiates cue-induced reinstatement of reward seeking in mice. Acta Pharmacol. Sin. 43, 2276–2288. doi: 10.1038/s41401-022-00866-x
Johnson, S. W., and North, R. A. (1992). Opioids excite dopamine neurons by hyperpolarization of local interneurons. J. Neurosci. 12, 483–488. doi: 10.1523/JNEUROSCI.12-02-00483.1992
Jones, J. L., Day, J. J., Aragona, B. J., Wheeler, R. A., Wightman, R. M., and Carelli, R. M. (2010). Basolateral amygdala modulates terminal dopamine release in the nucleus accumbens and conditioned responding. Biol. Psychiatry 67, 737–744. doi: 10.1016/j.biopsych.2009.11.006
Jongen-Relo, A. L., Voorn, P., and Groenewegen, H. J. (1994). Immunohistochemical characterization of the shell and core territories of the nucleus accumbens in the rat. Eur. J. Neurosci. 6, 1255–1264. doi: 10.1111/j.1460-9568.1994.tb00315.x
Kagiampaki, Z., Rohner, V., Kiss, C., Curreli, S., Dieter, A., Wilhelm, M., et al. (2023). Sensitive multicolor indicators for monitoring norepinephrine in vivo. Nat. Methods 20, 1426–1436. doi: 10.1038/s41592-023-01959-z
Kalin, N. H., Shelton, S. E., Fox, A. S., Oakes, T. R., and Davidson, R. J. (2005). Brain regions associated with the expression and contextual regulation of anxiety in primates. Biol. Psychiatry 58, 796–804. doi: 10.1016/j.biopsych.2005.05.021
Kalivas, P. W., and O'Brien, C. (2008). Drug addiction as a pathology of staged neuroplasticity. Neuropsychopharmacology 33, 166–180. doi: 10.1038/sj.npp.1301564
Kaneko, H., Zhang, S., Sekiguchi, M., Nikaido, T., Makita, K., Kurata, J., et al. (2017). Dysfunction of nucleus accumbens is associated with psychiatric problems in patients with chronic low back pain: a functional magnetic resonance imaging study. Spine 42, 844–853. doi: 10.1097/BRS.0000000000001930
Kannari, K., Shen, H., Arai, A., Tomiyama, M., and Baba, M. (2006). Reuptake of L-DOPA-derived extracellular dopamine in the striatum with dopaminergic denervation via serotonin transporters. Neurosci. Lett. 402, 62–65. doi: 10.1016/j.neulet.2006.03.059
Karkhanis, A. N., Locke, J. L., McCool, B. A., Weiner, J. L., and Jones, S. R. (2014). Social isolation rearing increases nucleus accumbens dopamine and norepinephrine responses to acute ethanol in adulthood. Alcohol. Clin. Exp. Res. 38, 2770–2779. doi: 10.1111/acer.12555
Kasper, J. M., McCue, D. L., Milton, A. J., Szwed, A., Sampson, C. M., Huang, M., et al. (2016). Gamma-aminobutyric acidergic projections from the dorsal raphe to the nucleus accumbens are regulated by neuromedin U. Biol. Psychiatry 80, 878–887. doi: 10.1016/j.biopsych.2016.02.031
Kawaguchi, Y., Wilson, C. J., Augood, S. J., and Emson, P. C. (1995). Striatal interneurones: chemical, physiological and morphological characterization. Trends Neurosci. 18, 527–535. doi: 10.1016/0166-2236(95)98374-8
Kelley, A. E., and Domesick, V. B. (1982). The distribution of the projection from the hippocampal formation to the nucleus accumbens in the rat: an anterograde- and retrograde-horseradish peroxidase study. Neuroscience 7, 2321–2335. doi: 10.1016/0306-4522(82)90198-1
Kelley, A. E., Smith-Roe, S. L., and Holahan, M. R. (1997). Response-reinforcement learning is dependent on N-methyl-D-aspartate receptor activation in the nucleus accumbens core. Proc. Natl. Acad. Sci. U. S. A. 94, 12174–12179. doi: 10.1073/pnas.94.22.12174
Keyes, P. C., Adams, E. L., Chen, Z., Bi, L., Nachtrab, G., Wang, V. J., et al. (2020). Orchestrating opiate-associated memories in thalamic circuits. Neuron 107, 1113–1123.e4. doi: 10.1016/j.neuron.2020.06.028
Kim, Y. C., Lee, H. G., and Han, K. A. (2007). D1 dopamine receptor dDA1 is required in the mushroom body neurons for aversive and appetitive learning in Drosophila. J. Neurosci. 27, 7640–7647. doi: 10.1523/JNEUROSCI.1167-07.2007
Kim, H. D., Wei, J., Call, T., Ma, X., Quintus, N. T., Summers, A. J., et al. (2024). SIRT1 coordinates transcriptional regulation of neural activity and modulates depression-like behaviors in the nucleus accumbens. Biol. Psychiatry. doi: 10.1016/j.biopsych.2024.03.017
Kim, H. D., Wei, J., Call, T., Quintus, N. T., Summers, A. J., Carotenuto, S., et al. (2021). Shisa6 mediates cell-type specific regulation of depression in the nucleus accumbens. Mol. Psychiatry 26, 7316–7327. doi: 10.1038/s41380-021-01217-8
Klawonn, A. M., Wilhelms, D. B., Lindstrom, S. H., Singh, A. K., Jaarola, M., Wess, J., et al. (2018). Muscarinic M4 receptors on cholinergic and dopamine D1 receptor-expressing neurons have opposing functionality for positive reinforcement and influence impulsivity. Front. Mol. Neurosci. 11:139. doi: 10.3389/fnmol.2018.00139
Kobrin, K. L., Arena, D. T., Heinrichs, S. C., Nguyen, O. H., and Kaplan, G. B. (2017). Dopamine D1 receptor agonist treatment attenuates extinction of morphine conditioned place preference while increasing dendritic complexity in the nucleus accumbens core. Behav. Brain Res. 322, 18–28. doi: 10.1016/j.bbr.2017.01.011
Kochenborger, L., Zanatta, D., Berretta, L. M., Lopes, A. P., Wunderlich, B. L., Januario, A. C., et al. (2012). Modulation of fear/anxiety responses, but not food intake, following alpha-adrenoceptor agonist microinjections in the nucleus accumbens shell of free-feeding rats. Neuropharmacology 62, 427–435. doi: 10.1016/j.neuropharm.2011.08.019
Kondev, V., Najeed, M., Loomba, N., Brown, J., Winder, D. G., Grueter, B. A., et al. (2023). Synaptic and cellular endocannabinoid signaling mechanisms regulate stress-induced plasticity of nucleus accumbens somatostatin neurons. Proc. Natl. Acad. Sci. U. S. A. 120:e2300585120. doi: 10.1073/pnas.2300585120
Koo, J. W., Chaudhury, D., Han, M. H., and Nestler, E. J. (2019). Role of mesolimbic brain-derived neurotrophic factor in depression. Biol. Psychiatry 86, 738–748. doi: 10.1016/j.biopsych.2019.05.020
Koob, G. F., Kenneth Lloyd, G., and Mason, B. J. (2009). Development of pharmacotherapies for drug addiction: a Rosetta stone approach. Nat. Rev. Drug Discov. 8, 500–515. doi: 10.1038/nrd2828
Koob, G. F., and Volkow, N. D. (2010). Neurocircuitry of addiction. Neuropsychopharmacology 35, 217–238. doi: 10.1038/npp.2009.110
Koob, G. F., and Volkow, N. D. (2016). Neurobiology of addiction: a neurocircuitry analysis. Lancet Psychiatry 3, 760–773. doi: 10.1016/S2215-0366(16)00104-8
Kravitz, A. V., Tye, L. D., and Kreitzer, A. C. (2012). Distinct roles for direct and indirect pathway striatal neurons in reinforcement. Nat. Neurosci. 15, 816–818. doi: 10.1038/nn.3100
Kronman, H., Torres-Berrio, A., Sidoli, S., Issler, O., Godino, A., Ramakrishnan, A., et al. (2021). Long-term behavioral and cell-type-specific molecular effects of early life stress are mediated by H3K79me2 dynamics in medium spiny neurons. Nat. Neurosci. 24, 667–676. doi: 10.1038/s41593-021-00814-8
Kupchik, Y. M., Brown, R. M., Heinsbroek, J. A., Lobo, M. K., Schwartz, D. J., and Kalivas, P. W. (2015). Coding the direct/indirect pathways by D1 and D2 receptors is not valid for accumbens projections. Nat. Neurosci. 18, 1230–1232. doi: 10.1038/nn.4068
Kutlu, M. G., Zachry, J. E., Melugin, P. R., Cajigas, S. A., Chevee, M. F., Kelly, S. J., et al. (2021). Dopamine release in the nucleus accumbens core signals perceived saliency. Curr. Biol. 31, 4748–4761.e8. doi: 10.1016/j.cub.2021.08.052
Kutlu, M. G., Zachry, J. E., Melugin, P. R., Tat, J., Cajigas, S., Isiktas, A. U., et al. (2022). Dopamine signaling in the nucleus accumbens core mediates latent inhibition. Nat. Neurosci. 25, 1071–1081. doi: 10.1038/s41593-022-01126-1
Labonte, B., Engmann, O., Purushothaman, I., Menard, C., Wang, J., Tan, C., et al. (2017). Sex-specific transcriptional signatures in human depression. Nat. Med. 23, 1102–1111. doi: 10.1038/nm.4386
Lammel, S., Hetzel, A., Hackel, O., Jones, I., Liss, B., and Roeper, J. (2008). Unique properties of mesoprefrontal neurons within a dual mesocorticolimbic dopamine system. Neuron 57, 760–773. doi: 10.1016/j.neuron.2008.01.022
Lammel, S., Ion, D. I., Roeper, J., and Malenka, R. C. (2011). Projection-specific modulation of dopamine neuron synapses by aversive and rewarding stimuli. Neuron 70, 855–862. doi: 10.1016/j.neuron.2011.03.025
Lammel, S., Lim, B. K., Ran, C., Huang, K. W., Betley, M. J., Tye, K. M., et al. (2012). Input-specific control of reward and aversion in the ventral tegmental area. Nature 491, 212–217. doi: 10.1038/nature11527
Larsen, M. B., Sonders, M. S., Mortensen, O. V., Larson, G. A., Zahniser, N. R., and Amara, S. G. (2011). Dopamine transport by the serotonin transporter: a mechanistically distinct mode of substrate translocation. J. Neurosci. 31, 6605–6615. doi: 10.1523/JNEUROSCI.0576-11.2011
Lee, S. J., Lodder, B., Chen, Y., Patriarchi, T., Tian, L., and Sabatini, B. L. (2021). Cell-type-specific asynchronous modulation of PKA by dopamine in learning. Nature 590, 451–456. doi: 10.1038/s41586-020-03050-5
Lee, B. R., Ma, Y. Y., Huang, Y. H., Wang, X., Otaka, M., Ishikawa, M., et al. (2013). Maturation of silent synapses in amygdala-accumbens projection contributes to incubation of cocaine craving. Nat. Neurosci. 16, 1644–1651. doi: 10.1038/nn.3533
Lefevre, E. M., Pisansky, M. T., Toddes, C., Baruffaldi, F., Pravetoni, M., Tian, L., et al. (2020). Interruption of continuous opioid exposure exacerbates drug-evoked adaptations in the mesolimbic dopamine system. Neuropsychopharmacology 45, 1781–1792. doi: 10.1038/s41386-020-0643-x
LeGates, T. A., Kvarta, M. D., Tooley, J. R., Francis, T. C., Lobo, M. K., Creed, M. C., et al. (2018). Reward behaviour is regulated by the strength of hippocampus-nucleus accumbens synapses. Nature 564, 258–262. doi: 10.1038/s41586-018-0740-8
Legon, W., Sato, T. F., Opitz, A., Mueller, J., Barbour, A., Williams, A., et al. (2014). Transcranial focused ultrasound modulates the activity of primary somatosensory cortex in humans. Nat. Neurosci. 17, 322–329. doi: 10.1038/nn.3620
Leri, F., Zhou, Y., Goddard, B., Cummins, E., and Kreek, M. J. (2006). Effects of high-dose methadone maintenance on cocaine place conditioning, cocaine self-administration, and mu-opioid receptor mRNA expression in the rat brain. Neuropsychopharmacology 31, 1462–1474. doi: 10.1038/sj.npp.1300927
Levita, L., Hoskin, R., and Champi, S. (2012). Avoidance of harm and anxiety: a role for the nucleus accumbens. Neuroimage 62, 189–198. doi: 10.1016/j.neuroimage.2012.04.059
Li, Z., Chen, Z., Fan, G., Li, A., Yuan, J., and Xu, T. (2018). Cell-type-specific afferent innervation of the nucleus accumbens core and shell. Front. Neuroanat. 12:84. doi: 10.3389/fnana.2018.00084
Li, S., and Kirouac, G. J. (2008). Projections from the paraventricular nucleus of the thalamus to the forebrain, with special emphasis on the extended amygdala. J. Comp. Neurol. 506, 263–287. doi: 10.1002/cne.21502
Li, Y., Simmler, L. D., Van Zessen, R., Flakowski, J., Wan, J. X., Deng, F., et al. (2021). Synaptic mechanism underlying serotonin modulation of transition to cocaine addiction. Science 373, 1252–1256. doi: 10.1126/science.abi9086
Li, Y., Zhong, W., Wang, D., Feng, Q., Liu, Z., Zhou, J., et al. (2016). Serotonin neurons in the dorsal raphe nucleus encode reward signals. Nat. Commun. 7:10503. doi: 10.1038/ncomms10503
Liberzon, I., Taylor, S. F., Amdur, R., Jung, T. D., Chamberlain, K. R., Minoshima, S., et al. (1999). Brain activation in PTSD in response to trauma-related stimuli. Biol. Psychiatry 45, 817–826. doi: 10.1016/s0006-3223(98)00246-7
Liu, Y., Jean-Richard-Dit-Bressel, P., Yau, J. O., Willing, A., Prasad, A. A., Power, J. M., et al. (2020). The mesolimbic dopamine activity signatures of relapse to alcohol-seeking. J. Neurosci. 40, 6409–6427. doi: 10.1523/JNEUROSCI.0724-20.2020
Liu, C., Kershberg, L., Wang, J., Schneeberger, S., and Kaeser, P. S. (2018). Dopamine secretion is mediated by sparse active zone-like release sites. Cell 172, 706–718.e15. doi: 10.1016/j.cell.2018.01.008
Liu, Z., Le, Q., Lv, Y., Chen, X., Cui, J., Zhou, Y., et al. (2022). A distinct D1-MSN subpopulation down-regulates dopamine to promote negative emotional state. Cell Res. 32, 139–156. doi: 10.1038/s41422-021-00588-5
Liu, H. M., Liao, M. L., Liu, G. X., Wang, L. J., Lian, D., Ren, J., et al. (2023). IPAC integrates rewarding and environmental memory during the acquisition of morphine CPP. Sci. Adv. 9:eadg5849. doi: 10.1126/sciadv.adg5849
Liu, Z., Lin, R., and Luo, M. (2020). Reward contributions to serotonergic functions. Annu. Rev. Neurosci. 43, 141–162. doi: 10.1146/annurev-neuro-093019-112252
Liu, Z., Wang, Y., Cai, L., Li, Y., Chen, B., Dong, Y., et al. (2016). Prefrontal cortex to accumbens projections in sleep regulation of reward. J. Neurosci. 36, 7897–7910. doi: 10.1523/JNEUROSCI.0347-16.2016
Liu, Z., Zhou, J., Li, Y., Hu, F., Lu, Y., Ma, M., et al. (2014). Dorsal raphe neurons signal reward through 5-HT and glutamate. Neuron 81, 1360–1374. doi: 10.1016/j.neuron.2014.02.010
Lobo, M. K., Covington, H. E. 3rd, Chaudhury, D., Friedman, A. K., Sun, H., Damez-Werno, D., et al. (2010). Cell type-specific loss of BDNF signaling mimics optogenetic control of cocaine reward. Science 330, 385–390. doi: 10.1126/science.1188472
Lowes, D. C., Chamberlin, L. A., Kretsge, L. N., Holt, E. S., Abbas, A. I., Park, A. J., et al. (2021). Ventral tegmental area GABA neurons mediate stress-induced blunted reward-seeking in mice. Nat. Commun. 12:3539. doi: 10.1038/s41467-021-23906-2
Loweth, J. A., Scheyer, A. F., Milovanovic, M., LaCrosse, A. L., Flores-Barrera, E., Werner, C. T., et al. (2014). Synaptic depression via mGluR1 positive allosteric modulation suppresses cue-induced cocaine craving. Nat. Neurosci. 17, 73–80. doi: 10.1038/nn.3590
Lu, L., Hope, B. T., and Shaham, Y. (2004). The cystine-glutamate transporter in the accumbens: a novel role in cocaine relapse. Trends Neurosci. 27, 74–76. doi: 10.1016/j.tins.2003.11.007
Lujan, M. A., Oliver, B. L., Young-Morrison, R., Engi, S. A., Zhang, L. Y., Wenzel, J. M., et al. (2023). A multivariate regressor of patterned dopamine release predicts relapse to cocaine. Cell Rep. 42:112553. doi: 10.1016/j.celrep.2023.112553
Luscher, C., and Malenka, R. C. (2011). Drug-evoked synaptic plasticity in addiction: from molecular changes to circuit remodeling. Neuron 69, 650–663. doi: 10.1016/j.neuron.2011.01.017
Luscher, C., and Pascoli, V. (2021). 'Ups, downs, and sideways' of dopamine in drug addiction. Trends Neurosci. 44, 593–594. doi: 10.1016/j.tins.2021.06.009
Ma, L., Chen, W., Yu, D., and Han, Y. (2020). Brain-wide mapping of afferent inputs to accumbens nucleus core subdomains and accumbens nucleus subnuclei. Front. Syst. Neurosci. 14:15. doi: 10.3389/fnsys.2020.00015
Ma, Y. Y., Lee, B. R., Wang, X., Guo, C., Liu, L., Cui, R., et al. (2014). Bidirectional modulation of incubation of cocaine craving by silent synapse-based remodeling of prefrontal cortex to accumbens projections. Neuron 83, 1453–1467. doi: 10.1016/j.neuron.2014.08.023
Mahon, S., Vautrelle, N., Pezard, L., Slaght, S. J., Deniau, J. M., Chouvet, G., et al. (2006). Distinct patterns of striatal medium spiny neuron activity during the natural sleep-wake cycle. J. Neurosci. 26, 12587–12595. doi: 10.1523/JNEUROSCI.3987-06.2006
Malkesman, O., Scattoni, M. L., Paredes, D., Tragon, T., Pearson, B., Shaltiel, G., et al. (2010). The female urine sniffing test: a novel approach for assessing reward-seeking behavior in rodents. Biol. Psychiatry 67, 864–871. doi: 10.1016/j.biopsych.2009.10.018
Mamaligas, A. A., and Ford, C. P. (2016). Spontaneous synaptic activation of muscarinic receptors by striatal cholinergic neuron firing. Neuron 91, 574–586. doi: 10.1016/j.neuron.2016.06.021
Mancini, M., Patel, J. C., Affinati, A. H., Witkovsky, P., and Rice, M. E. (2022). Leptin promotes striatal dopamine release via cholinergic interneurons and regionally distinct signaling pathways. J. Neurosci. 42, 6668–6679. doi: 10.1523/JNEUROSCI.0238-22.2022
Manz, K. M., Coleman, B. C., Grueter, C. A., Shields, B. C., Tadross, M. R., and Grueter, B. A. (2021). Noradrenergic signaling disengages feedforward transmission in the nucleus accumbens shell. J. Neurosci. 41, 3752–3763. doi: 10.1523/JNEUROSCI.2420-20.2021
Mao, L., Han, Y., Zhang, Q. W., and Tian, Y. (2023). Two-photon fluorescence imaging and specifically biosensing of norepinephrine on a 100-ms timescale. Nat. Commun. 14:1419. doi: 10.1038/s41467-023-36869-3
Marche, K., Martel, A. C., and Apicella, P. (2017). Differences between dorsal and ventral striatum in the sensitivity of tonically active neurons to rewarding events. Front. Syst. Neurosci. 11:52. doi: 10.3389/fnsys.2017.00052
Marinelli, M., and McCutcheon, J. E. (2014). Heterogeneity of dopamine neuron activity across traits and states. Neuroscience 282, 176–197. doi: 10.1016/j.neuroscience.2014.07.034
Marinelli, P. W., Quirion, R., and Gianoulakis, C. (2003). A microdialysis profile of beta-endorphin and catecholamines in the rat nucleus accumbens following alcohol administration. Psychopharmacology 169, 60–67. doi: 10.1007/s00213-003-1490-2
Mark, G. P., Rada, P., Pothos, E., and Hoebel, B. G. (1992). Effects of feeding and drinking on acetylcholine release in the nucleus accumbens, striatum, and hippocampus of freely behaving rats. J. Neurochem. 58, 2269–2274. doi: 10.1111/j.1471-4159.1992.tb10973.x
Masuzawa, M., Nakao, S., Miyamoto, E., Yamada, M., Murao, K., Nishi, K., et al. (2003). Pentobarbital inhibits ketamine-induced dopamine release in the rat nucleus accumbens: a microdialysis study. Anesth. Analg. 96, 148–152, table of contents. doi: 10.1097/00000539-200301000-00030
Matsumoto, M., and Hikosaka, O. (2009). Two types of dopamine neuron distinctly convey positive and negative motivational signals. Nature 459, 837–841. doi: 10.1038/nature08028
Matuskey, D., Bhagwagar, Z., Planeta, B., Pittman, B., Gallezot, J. D., Chen, J., et al. (2014). Reductions in brain 5-HT1B receptor availability in primarily cocaine-dependent humans. Biol. Psychiatry 76, 816–822. doi: 10.1016/j.biopsych.2013.11.022
Mayer, D., Kahl, E., Uzuneser, T. C., and Fendt, M. (2018). Role of the mesolimbic dopamine system in relief learning. Neuropsychopharmacology 43, 1651–1659. doi: 10.1038/s41386-018-0020-1
Mazzone, C. M., Liang-Guallpa, J., Li, C., Wolcott, N. S., Boone, M. H., Southern, M., et al. (2020). High-fat food biases hypothalamic and mesolimbic expression of consummatory drives. Nat. Neurosci. 23, 1253–1266. doi: 10.1038/s41593-020-0684-9
McBride, W. J., Murphy, J. M., and Ikemoto, S. (1999). Localization of brain reinforcement mechanisms: intracranial self-administration and intracranial place-conditioning studies. Behav. Brain Res. 101, 129–152. doi: 10.1016/s0166-4328(99)00022-4
McGovern, D. J., Polter, A. M., Prevost, E. D., Ly, A., McNulty, C. J., Rubinstein, B., et al. (2023). Ventral tegmental area glutamate neurons establish a mu-opioid receptor gated circuit to mesolimbic dopamine neurons and regulate opioid-seeking behavior. Neuropsychopharmacology 48, 1889–1900. doi: 10.1038/s41386-023-01637-w
McKittrick, C. R., and Abercrombie, E. D. (2007). Catecholamine mapping within nucleus accumbens: differences in basal and amphetamine-stimulated efflux of norepinephrine and dopamine in shell and core. J. Neurochem. 100, 1247–1256. doi: 10.1111/j.1471-4159.2006.04300.x
Menard, C., Pfau, M. L., Hodes, G. E., Kana, V., Wang, V. X., Bouchard, S., et al. (2017). Social stress induces neurovascular pathology promoting depression. Nat. Neurosci. 20, 1752–1760. doi: 10.1038/s41593-017-0010-3
Meredith, G. E., Blank, B., and Groenewegen, H. J. (1989). The distribution and compartmental organization of the cholinergic neurons in nucleus accumbens of the rat. Neuroscience 31, 327–345. doi: 10.1016/0306-4522(89)90377-1
Milic, M., Schmitt, U., Lutz, B., and Muller, M. B. (2021). Individual baseline behavioral traits predict the resilience phenotype after chronic social defeat. Neurobiol. Stress 14:100290. doi: 10.1016/j.ynstr.2020.100290
Mitrano, D. A., Schroeder, J. P., Smith, Y., Cortright, J. J., Bubula, N., Vezina, P., et al. (2012). Alpha-1 adrenergic receptors are localized on presynaptic elements in the nucleus accumbens and regulate mesolimbic dopamine transmission. Neuropsychopharmacology 37, 2161–2172. doi: 10.1038/npp.2012.68
Miyamoto, Y., Iida, A., Sato, K., Muramatsu, S., and Nitta, A. (2014). Knockdown of dopamine D(2) receptors in the nucleus accumbens core suppresses methamphetamine-induced behaviors and signal transduction in mice. Int. J. Neuropsychopharmacol. 18:pyu038. doi: 10.1093/ijnp/pyu038
Miyazaki, K., Miyazaki, K. W., Sivori, G., Yamanaka, A., Tanaka, K. F., and Doya, K. (2020). Serotonergic projections to the orbitofrontal and medial prefrontal cortices differentially modulate waiting for future rewards. Sci. Adv. 6:eabc7246. doi: 10.1126/sciadv.abc7246
Miyazaki, K. W., Miyazaki, K., Tanaka, K. F., Yamanaka, A., Takahashi, A., Tabuchi, S., et al. (2014). Optogenetic activation of dorsal raphe serotonin neurons enhances patience for future rewards. Curr. Biol. 24, 2033–2040. doi: 10.1016/j.cub.2014.07.041
Miyazaki, K., Miyazaki, K. W., Yamanaka, A., Tokuda, T., Tanaka, K. F., and Doya, K. (2018). Reward probability and timing uncertainty alter the effect of dorsal raphe serotonin neurons on patience. Nat. Commun. 9:2048. doi: 10.1038/s41467-018-04496-y
Mohebi, A., Collins, V. L., and Berke, J. D. (2023). Accumbens cholinergic interneurons dynamically promote dopamine release and enable motivation. Elife 12:e85011. doi: 10.7554/eLife.85011
Mohebi, A., Pettibone, J. R., Hamid, A. A., Wong, J. T., Vinson, L. T., Patriarchi, T., et al. (2019). Dissociable dopamine dynamics for learning and motivation. Nature 570, 65–70. doi: 10.1038/s41586-019-1235-y
Morales, M., and Margolis, E. B. (2017). Ventral tegmental area: cellular heterogeneity, connectivity and behaviour. Nat. Rev. Neurosci. 18, 73–85. doi: 10.1038/nrn.2016.165
Morel, C., Montgomery, S., and Han, M. H. (2019). Nicotine and alcohol: the role of midbrain dopaminergic neurons in drug reinforcement. Eur. J. Neurosci. 50, 2180–2200. doi: 10.1111/ejn.14160
Morrens, J., Aydin, C., Janse van Rensburg, A., Esquivelzeta Rabell, J., and Haesler, S. (2020). Cue-evoked dopamine promotes conditioned responding during learning. Neuron 106, 142–153.e7. doi: 10.1016/j.neuron.2020.01.012
Morrison, S. E., McGinty, V. B., du Hoffmann, J., and Nicola, S. M. (2017). Limbic-motor integration by neural excitations and inhibitions in the nucleus accumbens. J. Neurophysiol. 118, 2549–2567. doi: 10.1152/jn.00465.2017
Moussawi, K., Zhou, W., Shen, H., Reichel, C. M., See, R. E., Carr, D. B., et al. (2011). Reversing cocaine-induced synaptic potentiation provides enduring protection from relapse. Proc. Natl. Acad. Sci. U. S. A. 108, 385–390. doi: 10.1073/pnas.1011265108
Mu, P., Moyer, J. T., Ishikawa, M., Zhang, Y., Panksepp, J., Sorg, B. A., et al. (2010). Exposure to cocaine dynamically regulates the intrinsic membrane excitability of nucleus accumbens neurons. J. Neurosci. 30, 3689–3699. doi: 10.1523/JNEUROSCI.4063-09.2010
Mu, P., Neumann, P. A., Panksepp, J., Schluter, O. M., and Dong, Y. (2011). Exposure to cocaine alters dynorphin-mediated regulation of excitatory synaptic transmission in nucleus accumbens neurons. Biol. Psychiatry 69, 228–235. doi: 10.1016/j.biopsych.2010.09.014
Muir, J., Lorsch, Z. S., Ramakrishnan, C., Deisseroth, K., Nestler, E. J., Calipari, E. S., et al. (2018). In vivo fiber photometry reveals signature of future stress susceptibility in nucleus accumbens. Neuropsychopharmacology 43, 255–263. doi: 10.1038/npp.2017.122
Nagai, T., Nakamuta, S., Kuroda, K., Nakauchi, S., Nishioka, T., Takano, T., et al. (2016). Phosphoproteomics of the dopamine pathway enables discovery of Rap1 activation as a reward signal in vivo. Neuron 89, 550–565. doi: 10.1016/j.neuron.2015.12.019
Namburi, P., Beyeler, A., Yorozu, S., Calhoon, G. G., Halbert, S. A., Wichmann, R., et al. (2015). A circuit mechanism for differentiating positive and negative associations. Nature 520, 675–678. doi: 10.1038/nature14366
Narayanan, S., Lam, H., Christian, L., Levine, M. S., Grandy, D., Rubinstein, M., et al. (2004). Endogenous opioids mediate basal hedonic tone independent of dopamine D-1 or D-2 receptor activation. Neuroscience 124, 241–246. doi: 10.1016/j.neuroscience.2003.11.011
Nardou, R., Sawyer, E., Song, Y. J., Wilkinson, M., Padovan-Hernandez, Y., de Deus, J. L., et al. (2023). Psychedelics reopen the social reward learning critical period. Nature 618, 790–798. doi: 10.1038/s41586-023-06204-3
Nasca, C., Bigio, B., Zelli, D., Nicoletti, F., and McEwen, B. S. (2015). Mind the gap: glucocorticoids modulate hippocampal glutamate tone underlying individual differences in stress susceptibility. Mol. Psychiatry 20, 755–763. doi: 10.1038/mp.2014.96
Neisewander, J. L., Cheung, T. H., and Pentkowski, N. S. (2014). Dopamine D3 and 5-HT1B receptor dysregulation as a result of psychostimulant intake and forced abstinence: implications for medications development. Neuropharmacology 76, 301–319. doi: 10.1016/j.neuropharm.2013.08.014
Nestler, E. J. (2005). Is there a common molecular pathway for addiction? Nat. Neurosci. 8, 1445–1449. doi: 10.1038/nn1578
Nestler, E. J. (2015). ∆FosB: a transcriptional regulator of stress and antidepressant responses. Eur. J. Pharmacol. 753, 66–72. doi: 10.1016/j.ejphar.2014.10.034
Nestler, E. J., and Carlezon, W. A. Jr. (2006). The mesolimbic dopamine reward circuit in depression. Biol. Psychiatry 59, 1151–1159. doi: 10.1016/j.biopsych.2005.09.018
Nguyen, P. T., Dorman, L. C., Pan, S., Vainchtein, I. D., Han, R. T., Nakao-Inoue, H., et al. (2020). Microglial remodeling of the extracellular matrix promotes synapse plasticity. Cell 182, 388–403.e15. doi: 10.1016/j.cell.2020.05.050
Niedzielska-Andres, E., Pomierny-Chamiolo, L., Andres, M., Walczak, M., Knackstedt, L. A., Filip, M., et al. (2021). Cocaine use disorder: a look at metabotropic glutamate receptors and glutamate transporters. Pharmacol. Ther. 221:107797. doi: 10.1016/j.pharmthera.2020.107797
Nisell, M., Marcus, M., Nomikos, G. G., and Svensson, T. H. (1997). Differential effects of acute and chronic nicotine on dopamine output in the core and shell of the rat nucleus accumbens. J. Neural Transm. 104, 1–10. doi: 10.1007/BF01271290
Nishioka, T., Attachaipanich, S., Hamaguchi, K., Lazarus, M., de Kerchove d'Exaerde, A., Macpherson, T., et al. (2023). Error-related signaling in nucleus accumbens D2 receptor-expressing neurons guides inhibition-based choice behavior in mice. Nat. Commun. 14:2284. doi: 10.1038/s41467-023-38025-3
Niu, L., Guo, Y., Lin, Z., Shi, Z., Bian, T., Qi, L., et al. (2020). Noninvasive ultrasound deep brain stimulation of nucleus accumbens induces behavioral avoidance. Sci. China Life Sci. 63, 1328–1336. doi: 10.1007/s11427-019-1616-6
O'Donnell, P., and Grace, A. A. (1995). Synaptic interactions among excitatory afferents to nucleus accumbens neurons: hippocampal gating of prefrontal cortical input. J. Neurosci. 15, 3622–3639. doi: 10.1523/JNEUROSCI.15-05-03622.1995
O'Donnell, P., Greene, J., Pabello, N., Lewis, B. L., and Grace, A. A. (1999). Modulation of cell firing in the nucleus accumbens. Ann. N. Y. Acad. Sci. 877, 157–175. doi: 10.1111/j.1749-6632.1999.tb09267.x
Okaty, B. W., Commons, K. G., and Dymecki, S. M. (2019). Embracing diversity in the 5-HT neuronal system. Nat. Rev. Neurosci. 20, 397–424. doi: 10.1038/s41583-019-0151-3
O'Neal, T. J., Bernstein, M. X., MacDougall, D. J., and Ferguson, S. M. (2022). A conditioned place preference for heroin is signaled by increased dopamine and direct pathway activity and decreased indirect pathway activity in the nucleus accumbens. J. Neurosci. 42, 2011–2024. doi: 10.1523/JNEUROSCI.1451-21.2021
Ormel, J., Hartman, C. A., and Snieder, H. (2019). The genetics of depression: successful genome-wide association studies introduce new challenges. Transl. Psychiatry 9:114. doi: 10.1038/s41398-019-0450-5
Owen, S. F., Berke, J. D., and Kreitzer, A. C. (2018). Fast-spiking interneurons supply feedforward control of bursting, calcium, and plasticity for efficient learning. Cell 172, 683–695.e15. doi: 10.1016/j.cell.2018.01.005
Paniccia, J. E., Vollmer, K. M., Green, L. M., Grant, R. I., Winston, K. T., Buchmaier, S., et al. (2024). Restoration of a paraventricular thalamo-accumbal behavioral suppression circuit prevents reinstatement of heroin seeking. Neuron 112, 772–785.e9. doi: 10.1016/j.neuron.2023.11.024
Parsegian, A., and See, R. E. (2014). Dysregulation of dopamine and glutamate release in the prefrontal cortex and nucleus accumbens following methamphetamine self-administration and during reinstatement in rats. Neuropsychopharmacology 39, 811–822. doi: 10.1038/npp.2013.231
Pascoli, V., Terrier, J., Espallergues, J., Valjent, E., O'Connor, E. C., and Luscher, C. (2014). Contrasting forms of cocaine-evoked plasticity control components of relapse. Nature 509, 459–464. doi: 10.1038/nature13257
Patriarchi, T., Cho, J. R., Merten, K., Howe, M. W., Marley, A., Xiong, W. H., et al. (2018). Ultrafast neuronal imaging of dopamine dynamics with designed genetically encoded sensors. Science 360:eaat4422. doi: 10.1126/science.aat4422
Pedersen, C. E., Castro, D. C., Gray, M. M., Zhou, Z. C., Piantadosi, S. C., Gowrishankar, R., et al. (2022). Medial accumbens shell spiny projection neurons encode relative reward preference. bioRxiv. doi: 10.1101/2022.09.18.508426
Peng, S. Y., Li, B., Xi, K., Wang, J. J., and Zhu, J. N. (2018). Presynaptic alpha(2)-adrenoceptor modulates glutamatergic synaptic transmission in rat nucleus accumbens in vitro. Neurosci. Lett. 665, 117–122. doi: 10.1016/j.neulet.2017.11.060
Pennartz, C. M., Ito, R., Verschure, P. F., Battaglia, F. P., and Robbins, T. W. (2011). The hippocampal-striatal axis in learning, prediction and goal-directed behavior. Trends Neurosci. 34, 548–559. doi: 10.1016/j.tins.2011.08.001
Pentkowski, N. S., Cheung, T. H., Toy, W. A., Adams, M. D., Neumaier, J. F., and Neisewander, J. L. (2012). Protracted withdrawal from cocaine self-administration flips the switch on 5-HT(1B) receptor modulation of cocaine abuse-related behaviors. Biol. Psychiatry 72, 396–404. doi: 10.1016/j.biopsych.2012.03.024
Pentkowski, N. S., Harder, B. G., Brunwasser, S. J., Bastle, R. M., Peartree, N. A., Yanamandra, K., et al. (2014). Pharmacological evidence for an abstinence-induced switch in 5-HT1B receptor modulation of cocaine self-administration and cocaine-seeking behavior. ACS Chem. Neurosci. 5, 168–176. doi: 10.1021/cn400155t
Pessin, S., Philippi, C. L., Reyna, L., Buggar, N., and Bruce, S. E. (2021). Influence of anhedonic symptom severity on reward circuit connectivity in PTSD. Behav. Brain Res. 407:113258. doi: 10.1016/j.bbr.2021.113258
Pickel, V. M., and Chan, J. (1999). Ultrastructural localization of the serotonin transporter in limbic and motor compartments of the nucleus accumbens. J. Neurosci. 19, 7356–7366. doi: 10.1523/JNEUROSCI.19-17-07356.1999
Pignatelli, M., Tejeda, H. A., Barker, D. J., Bontempi, L., Wu, J., Lopez, A., et al. (2021). Cooperative synaptic and intrinsic plasticity in a disynaptic limbic circuit drive stress-induced anhedonia and passive coping in mice. Mol. Psychiatry 26, 1860–1879. doi: 10.1038/s41380-020-0686-8
Pisansky, M. T., Lefevre, E. M., Retzlaff, C. L., Trieu, B. H., Leipold, D. W., and Rothwell, P. E. (2019). Nucleus accumbens fast-spiking interneurons constrain impulsive action. Biol. Psychiatry 86, 836–847. doi: 10.1016/j.biopsych.2019.07.002
Pizzagalli, D. A., Holmes, A. J., Dillon, D. G., Goetz, E. L., Birk, J. L., Bogdan, R., et al. (2009). Reduced caudate and nucleus accumbens response to rewards in unmedicated individuals with major depressive disorder. Am. J. Psychiatry 166, 702–710. doi: 10.1176/appi.ajp.2008.08081201
Plaznik, A., Danysz, W., and Kostowski, W. (1985). A stimulatory effect of intraaccumbens injections of noradrenaline on the behavior of rats in the forced swim test. Psychopharmacology 87, 119–123. doi: 10.1007/BF00431791
Ploski, J. E., and Vaidya, V. A. (2021). The neurocircuitry of posttraumatic stress disorder and major depression: insights into overlapping and distinct circuit dysfunction-a tribute to Ron Duman. Biol. Psychiatry 90, 109–117. doi: 10.1016/j.biopsych.2021.04.009
Poggi, G., Bergamini, G., Dulinskas, R., Madur, L., Greter, A., Ineichen, C., et al. (2024). Engagement of basal amygdala-nucleus accumbens glutamate neurons in the processing of rewarding or aversive social stimuli. Eur. J. Neurosci. 59, 996–1015. doi: 10.1111/ejn.16272
Pomrenze, M. B., Cardozo Pinto, D. F., Neumann, P. A., Llorach, P., Tucciarone, J. M., Morishita, W., et al. (2022). Modulation of 5-HT release by dynorphin mediates social deficits during opioid withdrawal. Neuron 110, 4125–4143.e6. doi: 10.1016/j.neuron.2022.09.024
Pontieri, F. E., Tanda, G., Orzi, F., and Di Chiara, G. (1996). Effects of nicotine on the nucleus accumbens and similarity to those of addictive drugs. Nature 382, 255–257. doi: 10.1038/382255a0
Pratt, W. E., Schall, M. A., and Choi, E. (2012). Selective serotonin receptor stimulation of the medial nucleus accumbens differentially affects appetitive motivation for food on a progressive ratio schedule of reinforcement. Neurosci. Lett. 511, 84–88. doi: 10.1016/j.neulet.2012.01.038
Pribiag, H., Shin, S., Wang, E. H., Sun, F., Datta, P., Okamoto, A., et al. (2021). Ventral pallidum DRD3 potentiates a pallido-habenular circuit driving accumbal dopamine release and cocaine seeking. Neuron 109, 2165–2182.e10. doi: 10.1016/j.neuron.2021.05.002
Qi, G., Zhang, P., Li, T., Li, M., Zhang, Q., He, F., et al. (2022). NAc-VTA circuit underlies emotional stress-induced anxiety-like behavior in the three-chamber vicarious social defeat stress mouse model. Nat. Commun. 13:577. doi: 10.1038/s41467-022-28190-2
Qi, J., Zhang, S., Wang, H. L., Barker, D. J., Miranda-Barrientos, J., and Morales, M. (2016). VTA glutamatergic inputs to nucleus accumbens drive aversion by acting on GABAergic interneurons. Nat. Neurosci. 19, 725–733. doi: 10.1038/nn.4281
Qiao, H., Li, M. X., Xu, C., Chen, H. B., An, S. C., and Ma, X. M. (2016). Dendritic spines in depression: what we learned from animal models. Neural Plast. 2016, 8056370–8056326. doi: 10.1155/2016/8056370
Rada, P., Avena, N. M., and Hoebel, B. G. (2005). Daily bingeing on sugar repeatedly releases dopamine in the accumbens shell. Neuroscience 134, 737–744. doi: 10.1016/j.neuroscience.2005.04.043
Rada, P., Jensen, K., and Hoebel, B. G. (2001). Effects of nicotine and mecamylamine-induced withdrawal on extracellular dopamine and acetylcholine in the rat nucleus accumbens. Psychopharmacology 157, 105–110. doi: 10.1007/s002130100781
Rada, P., Johnson, D. F., Lewis, M. J., and Hoebel, B. G. (2004). In alcohol-treated rats, naloxone decreases extracellular dopamine and increases acetylcholine in the nucleus accumbens: evidence of opioid withdrawal. Pharmacol. Biochem. Behav. 79, 599–605. doi: 10.1016/j.pbb.2004.09.011
Rada, P. V., Mark, G. P., Taylor, K. M., and Hoebel, B. G. (1996). Morphine and naloxone, i.p. or locally, affect extracellular acetylcholine in the accumbens and prefrontal cortex. Pharmacol. Biochem. Behav. 53, 809–816. doi: 10.1016/0091-3057(95)02078-0
Rada, P., Pothos, E., Mark, G. P., and Hoebel, B. G. (1991). Microdialysis evidence that acetylcholine in the nucleus accumbens is involved in morphine withdrawal and its treatment with clonidine. Brain Res. 561, 354–356. doi: 10.1016/0006-8993(91)91616-9
Ray, M. H., Moaddab, M., and McDannald, M. A. (2022). Threat and bidirectional valence signaling in the nucleus accumbens core. J. Neurosci. 42, 817–833. doi: 10.1523/JNEUROSCI.1107-21.2021
Ray, M. H., Russ, A. N., Walker, R. A., and McDannald, M. A. (2020). The nucleus accumbens core is necessary to scale fear to degree of threat. J. Neurosci. 40, 4750–4760. doi: 10.1523/JNEUROSCI.0299-20.2020
Reith, M. E., Li, M. Y., and Yan, Q. S. (1997). Extracellular dopamine, norepinephrine, and serotonin in the ventral tegmental area and nucleus accumbens of freely moving rats during intracerebral dialysis following systemic administration of cocaine and other uptake blockers. Psychopharmacology 134, 309–317. doi: 10.1007/s002130050454
Ren, J., Friedmann, D., Xiong, J., Liu, C. D., Ferguson, B. R., Weerakkody, T., et al. (2018). Anatomically defined and functionally distinct dorsal raphe serotonin sub-systems. Cell 175, 472–487.e20. doi: 10.1016/j.cell.2018.07.043
Reverte, I., Marchetti, C., Pezza, S., Zenoni, S. F., Scaringi, G., Ferrucci, L., et al. (2024). Microglia-mediated calcium-permeable AMPAR accumulation in the nucleus accumbens drives hyperlocomotion during cocaine withdrawal. Brain Behav. Immun. 115, 535–542. doi: 10.1016/j.bbi.2023.11.007
Reynolds, J. N. J., Avvisati, R., Dodson, P. D., Fisher, S. D., Oswald, M. J., Wickens, J. R., et al. (2022). Coincidence of cholinergic pauses, dopaminergic activation and depolarisation of spiny projection neurons drives synaptic plasticity in the striatum. Nat. Commun. 13:1296. doi: 10.1038/s41467-022-28950-0
Ribeiro, E. A., Salery, M., Scarpa, J. R., Calipari, E. S., Hamilton, P. J., Ku, S. M., et al. (2018). Transcriptional and physiological adaptations in nucleus accumbens somatostatin interneurons that regulate behavioral responses to cocaine. Nat. Commun. 9:3149. doi: 10.1038/s41467-018-05657-9
Richard, J. M., Castro, D. C., Difeliceantonio, A. G., Robinson, M. J., and Berridge, K. C. (2013). Mapping brain circuits of reward and motivation: in the footsteps of Ann Kelley. Neurosci. Biobehav. Rev. 37, 1919–1931. doi: 10.1016/j.neubiorev.2012.12.008
Richfield, E. K., Penney, J. B., and Young, A. B. (1989). Anatomical and affinity state comparisons between dopamine D1 and D2 receptors in the rat central nervous system. Neuroscience 30, 767–777. doi: 10.1016/0306-4522(89)90168-1
Roberts, B. L., Zhu, M., Zhao, H., Dillon, C., and Appleyard, S. M. (2017). High glucose increases action potential firing of catecholamine neurons in the nucleus of the solitary tract by increasing spontaneous glutamate inputs. Am. J. Physiol. Regul. Integr. Comp. Physiol. 313, R229–R239. doi: 10.1152/ajpregu.00413.2016
Roger-Sanchez, C., Aguilar, M. A., Manzanedo, C., Minarro, J., and Rodriguez-Arias, M. (2013). Neurochemical substrates of MDMA reward: effects of the inhibition of serotonin reuptake on the acquisition and reinstatement of MDMA-induced CPP. Curr. Pharm. Des. 19, 7050–7064. doi: 10.2174/138161281940131209143632
Roitman, M. F., Stuber, G. D., Phillips, P. E., Wightman, R. M., and Carelli, R. M. (2004). Dopamine operates as a subsecond modulator of food seeking. J. Neurosci. 24, 1265–1271. doi: 10.1523/JNEUROSCI.3823-03.2004
Ruda-Kucerova, J., Amchova, P., Havlickova, T., Jerabek, P., Babinska, Z., Kacer, P., et al. (2015). Reward related neurotransmitter changes in a model of depression: an in vivo microdialysis study. World J. Biol. Psychiatry 16, 521–535. doi: 10.3109/15622975.2015.1077991
Russo, S. J., and Nestler, E. J. (2013). The brain reward circuitry in mood disorders. Nat. Rev. Neurosci. 14, 609–625. doi: 10.1038/nrn3381
Sabandal, J. M., Berry, J. A., and Davis, R. L. (2021). Dopamine-based mechanism for transient forgetting. Nature 591, 426–430. doi: 10.1038/s41586-020-03154-y
Saddoris, M. P., Sugam, J. A., and Carelli, R. M. (2017). Prior cocaine experience impairs normal phasic dopamine signals of reward value in accumbens shell. Neuropsychopharmacology 42, 766–773. doi: 10.1038/npp.2016.189
Saigusa, T., Aono, Y., Uchida, T., Takada, K., Verheij, M. M., Koshikawa, N., et al. (2012). The alpha(1)-, but not alpha(2)-, adrenoceptor in the nucleus accumbens plays an inhibitory role upon the accumbal noradrenaline and dopamine efflux of freely moving rats. Eur. J. Pharmacol. 688, 35–41. doi: 10.1016/j.ejphar.2012.05.005
Sailer, U., Robinson, S., Fischmeister, F. P., Konig, D., Oppenauer, C., Lueger-Schuster, B., et al. (2008). Altered reward processing in the nucleus accumbens and mesial prefrontal cortex of patients with posttraumatic stress disorder. Neuropsychologia 46, 2836–2844. doi: 10.1016/j.neuropsychologia.2008.05.022
Samaha, A. N., Khoo, S. Y., Ferrario, C. R., and Robinson, T. E. (2021). Dopamine 'ups and downs' in addiction revisited. Trends Neurosci. 44, 516–526. doi: 10.1016/j.tins.2021.03.003
Scangos, K. W., Makhoul, G. S., Sugrue, L. P., Chang, E. F., and Krystal, A. D. (2021). State-dependent responses to intracranial brain stimulation in a patient with depression. Nat. Med. 27, 229–231. doi: 10.1038/s41591-020-01175-8
Schall, T. A., Wright, W. J., and Dong, Y. (2021). Nucleus accumbens fast-spiking interneurons in motivational and addictive behaviors. Mol. Psychiatry 26, 234–246. doi: 10.1038/s41380-020-0683-y
Schmidt, H. D., and Pierce, R. C. (2006). Cooperative activation of D1-like and D2-like dopamine receptors in the nucleus accumbens shell is required for the reinstatement of cocaine-seeking behavior in the rat. Neuroscience 142, 451–461. doi: 10.1016/j.neuroscience.2006.06.004
Schultz, W. (1998). Predictive reward signal of dopamine neurons. J. Neurophysiol. 80, 1–27. doi: 10.1152/jn.1998.80.1.1
Schultz, W. (2016). Dopamine reward prediction-error signalling: a two-component response. Nat. Rev. Neurosci. 17, 183–195. doi: 10.1038/nrn.2015.26
Schwartz, N., Miller, C., and Fields, H. L. (2017). Cortico-accumbens regulation of approach-avoidance behavior is modified by experience and chronic pain. Cell Rep. 19, 1522–1531. doi: 10.1016/j.celrep.2017.04.073
Sciolino, N. R., Hsiang, M., Mazzone, C. M., Wilson, L. R., Plummer, N. W., Amin, J., et al. (2022). Natural locus coeruleus dynamics during feeding. Sci. Adv. 8:eabn9134. doi: 10.1126/sciadv.abn9134
Scudder, S. L., Baimel, C., Macdonald, E. E., and Carter, A. G. (2018). Hippocampal-evoked feedforward inhibition in the nucleus accumbens. J. Neurosci. 38, 9091–9104. doi: 10.1523/JNEUROSCI.1971-18.2018
Self, D. W., and Stein, L. (1992). Receptor subtypes in opioid and stimulant reward. Pharmacol. Toxicol. 70, 87–94. doi: 10.1111/j.1600-0773.1992.tb00435.x
Shen, C. J., Zheng, D., Li, K. X., Yang, J. M., Pan, H. Q., Yu, X. D., et al. (2019). Cannabinoid CB(1) receptors in the amygdalar cholecystokinin glutamatergic afferents to nucleus accumbens modulate depressive-like behavior. Nat. Med. 25, 337–349. doi: 10.1038/s41591-018-0299-9
Sheng, H., Lei, C., Yuan, Y., Fu, Y., Cui, D., Yang, L., et al. (2023). Nucleus accumbens circuit disinhibits lateral hypothalamus glutamatergic neurons contributing to morphine withdrawal memory in male mice. Nat. Commun. 14:71. doi: 10.1038/s41467-022-35758-5
Shin, J. H., Adrover, M. F., and Alvarez, V. A. (2017). Distinctive modulation of dopamine release in the nucleus accumbens shell mediated by dopamine and acetylcholine receptors. J. Neurosci. 37, 11166–11180. doi: 10.1523/JNEUROSCI.0596-17.2017
Shippenberg, T. S., Bals-Kubik, R., and Herz, A. (1993). Examination of the neurochemical substrates mediating the motivational effects of opioids: role of the mesolimbic dopamine system and D-1 vs. D-2 dopamine receptors. J. Pharmacol. Exp. Ther. 265, 53–59
Simmler, L. D., Li, Y., Hadjas, L. C., Hiver, A., van Zessen, R., and Luscher, C. (2022). Dual action of ketamine confines addiction liability. Nature 608, 368–373. doi: 10.1038/s41586-022-04993-7
Skirzewski, M., Princz-Lebel, O., German-Castelan, L., Crooks, A. M., Kim, G. K., Tarnow, S. H., et al. (2022). Continuous cholinergic-dopaminergic updating in the nucleus accumbens underlies approaches to reward-predicting cues. Nat. Commun. 13:7924. doi: 10.1038/s41467-022-35601-x
Smith, M. L., Asada, N., and Malenka, R. C. (2021). Anterior cingulate inputs to nucleus accumbens control the social transfer of pain and analgesia. Science 371, 153–159. doi: 10.1126/science.abe3040
Soares-Cunha, C., Coimbra, B., David-Pereira, A., Borges, S., Pinto, L., Costa, P., et al. (2016a). Activation of D2 dopamine receptor-expressing neurons in the nucleus accumbens increases motivation. Nat. Commun. 7:11829. doi: 10.1038/ncomms11829
Soares-Cunha, C., Coimbra, B., Domingues, A. V., Vasconcelos, N., Sousa, N., and Rodrigues, A. J. (2018). Nucleus accumbens microcircuit underlying D2-MSN-driven increase in motivation. eNeuro 5:EENEURO.0386-18.2018. doi: 10.1523/ENEURO.0386-18.2018
Soares-Cunha, C., Coimbra, B., Sousa, N., and Rodrigues, A. J. (2016b). Reappraising striatal D1- and D2-neurons in reward and aversion. Neurosci. Biobehav. Rev. 68, 370–386. doi: 10.1016/j.neubiorev.2016.05.021
Soares-Cunha, C., de Vasconcelos, N. A. P., Coimbra, B., Domingues, A. V., Silva, J. M., Loureiro-Campos, E., et al. (2020). Nucleus accumbens medium spiny neurons subtypes signal both reward and aversion. Mol. Psychiatry 25, 3241–3255. doi: 10.1038/s41380-019-0484-3
Soares-Cunha, C., Domingues, A. V., Correia, R., Coimbra, B., Vieitas-Gaspar, N., de Vasconcelos, N. A. P., et al. (2022). Distinct role of nucleus accumbens D2-MSN projections to ventral pallidum in different phases of motivated behavior. Cell Rep. 38:110380. doi: 10.1016/j.celrep.2022.110380
Sokolowski, J. D., Conlan, A. N., and Salamone, J. D. (1998). A microdialysis study of nucleus accumbens core and shell dopamine during operant responding in the rat. Neuroscience 86, 1001–1009. doi: 10.1016/s0306-4522(98)00066-9
Song, N., Liu, Z., Gao, Y., Lu, S., Yang, S., and Yuan, C. (2024). NAc-DBS corrects depression-like behaviors in CUMS mouse model via disinhibition of DA neurons in the VTA. Mol. Psychiatry 29, 1550–1566. doi: 10.1038/s41380-024-02476-x
Song, C., Merali, Z., and Anisman, H. (1999). Variations of nucleus accumbens dopamine and serotonin following systemic interleukin-1, interleukin-2 or interleukin-6 treatment. Neuroscience 88, 823–836. doi: 10.1016/s0306-4522(98)00271-1
Stamford, J. A., Kruk, Z. L., and Millar, J. (1990). Striatal dopamine terminals release serotonin after 5-HTP pretreatment: in vivo voltammetric data. Brain Res. 515, 173–180. doi: 10.1016/0006-8993(90)90593-z
Steinberg, E. E., Boivin, J. R., Saunders, B. T., Witten, I. B., Deisseroth, K., and Janak, P. H. (2014). Positive reinforcement mediated by midbrain dopamine neurons requires D1 and D2 receptor activation in the nucleus accumbens. PLoS One 9:e94771. doi: 10.1371/journal.pone.0094771
Stuber, G. D., Sparta, D. R., Stamatakis, A. M., van Leeuwen, W. A., Hardjoprajitno, J. E., Cho, S., et al. (2011). Excitatory transmission from the amygdala to nucleus accumbens facilitates reward seeking. Nature 475, 377–380. doi: 10.1038/nature10194
Sulzer, D. (2011). How addictive drugs disrupt presynaptic dopamine neurotransmission. Neuron 69, 628–649. doi: 10.1016/j.neuron.2011.02.010
Sun, W., and Rebec, G. V. (2006). Repeated cocaine self-administration alters processing of cocaine-related information in rat prefrontal cortex. J. Neurosci. 26, 8004–8008. doi: 10.1523/JNEUROSCI.1413-06.2006
Sun, F., Zeng, J., Jing, M., Zhou, J., Feng, J., Owen, S. F., et al. (2018). A genetically encoded fluorescent sensor enables rapid and specific detection of dopamine in flies, fish, and mice. Cell 174, 481–496.e19. doi: 10.1016/j.cell.2018.06.042
Sun, F., Zhou, J., Dai, B., Qian, T., Zeng, J., Li, X., et al. (2020). Next-generation GRAB sensors for monitoring dopaminergic activity in vivo. Nat. Methods 17, 1156–1166. doi: 10.1038/s41592-020-00981-9
Svensson, L., and Ahlenius, S. (1982). Functional importance of nucleus accumbens noradrenaline in the rat. Acta Pharmacol Toxicol 50, 22–24. doi: 10.1111/j.1600-0773.1982.tb00934.x
Tan, A., Costi, S., Morris, L. S., Van Dam, N. T., Kautz, M., Whitton, A. E., et al. (2020). Effects of the KCNQ channel opener ezogabine on functional connectivity of the ventral striatum and clinical symptoms in patients with major depressive disorder. Mol. Psychiatry 25, 1323–1333. doi: 10.1038/s41380-018-0283-2
Tanaka, M., Yamada, S., and Watanabe, Y. (2021). The role of neuropeptide Y in the nucleus accumbens. Int. J. Mol. Sci. 22:7287. doi: 10.3390/ijms22147287
Tanda, G., Pontieri, F. E., and Di Chiara, G. (1997). Cannabinoid and heroin activation of mesolimbic dopamine transmission by a common mu1 opioid receptor mechanism. Science 276, 2048–2050. doi: 10.1126/science.276.5321.2048
Taverna, S., van Dongen, Y. C., Groenewegen, H. J., and Pennartz, C. M. (2004). Direct physiological evidence for synaptic connectivity between medium-sized spiny neurons in rat nucleus accumbens in situ. J. Neurophysiol. 91, 1111–1121. doi: 10.1152/jn.00892.2003
Teague, C. D., and Nestler, E. J. (2022). Key transcription factors mediating cocaine-induced plasticity in the nucleus accumbens. Mol. Psychiatry 27, 687–709. doi: 10.1038/s41380-021-01163-5
Tepper, J. M., and Bolam, J. P. (2004). Functional diversity and specificity of neostriatal interneurons. Curr. Opin. Neurobiol. 14, 685–692. doi: 10.1016/j.conb.2004.10.003
Tepper, J. M., Koos, T., Ibanez-Sandoval, O., Tecuapetla, F., Faust, T. W., and Assous, M. (2018). Heterogeneity and diversity of striatal GABAergic interneurons: update 2018. Front. Neuroanat. 12:91. doi: 10.3389/fnana.2018.00091
Terauchi, A., Yee, P., Johnson-Venkatesh, E. M., Seiglie, M. P., Kim, L., Pitino, J. C., et al. (2023). The projection-specific signals that establish functionally segregated dopaminergic synapses. Cell 186, 3845–3861.e24. doi: 10.1016/j.cell.2023.07.023
Thoeni, S., Loureiro, M., O'Connor, E. C., and Luscher, C. (2020). Depression of accumbal to lateral hypothalamic synapses gates overeating. Neuron 107, 158–172.e4. doi: 10.1016/j.neuron.2020.03.029
Thompson, B. L., Oscar-Berman, M., and Kaplan, G. B. (2021). Opioid-induced structural and functional plasticity of medium-spiny neurons in the nucleus accumbens. Neurosci. Biobehav. Rev. 120, 417–430. doi: 10.1016/j.neubiorev.2020.10.015
Threlfell, S., Lalic, T., Platt, N. J., Jennings, K. A., Deisseroth, K., and Cragg, S. J. (2012). Striatal dopamine release is triggered by synchronized activity in cholinergic interneurons. Neuron 75, 58–64. doi: 10.1016/j.neuron.2012.04.038
Tong, Y., Cho, S., Coenen, V. A., and Dobrossy, M. D. (2024). Input-output relation of midbrain connectomics in a rodent model of depression. J. Affect. Disord. 345, 443–454. doi: 10.1016/j.jad.2023.10.124
Tourino, C., Ledent, C., Maldonado, R., and Valverde, O. (2008). CB1 cannabinoid receptor modulates 3,4-methylenedioxymethamphetamine acute responses and reinforcement. Biol. Psychiatry 63, 1030–1038. doi: 10.1016/j.biopsych.2007.09.003
Tran, A. H., Tamura, R., Uwano, T., Kobayashi, T., Katsuki, M., Matsumoto, G., et al. (2002). Altered accumbens neural response to prediction of reward associated with place in dopamine D2 receptor knockout mice. Proc. Natl. Acad. Sci. U. S. A. 99, 8986–8991. doi: 10.1073/pnas.132284599
Treadway, M. T., and Zald, D. H. (2011). Reconsidering anhedonia in depression: lessons from translational neuroscience. Neurosci. Biobehav. Rev. 35, 537–555. doi: 10.1016/j.neubiorev.2010.06.006
Trifilieff, P., Feng, B., Urizar, E., Winiger, V., Ward, R. D., Taylor, K. M., et al. (2013). Increasing dopamine D2 receptor expression in the adult nucleus accumbens enhances motivation. Mol. Psychiatry 18, 1025–1033. doi: 10.1038/mp.2013.57
Trigo, J. M., Renoir, T., Lanfumey, L., Hamon, M., Lesch, K. P., Robledo, P., et al. (2007). 3,4-methylenedioxymethamphetamine self-administration is abolished in serotonin transporter knockout mice. Biol. Psychiatry 62, 669–679. doi: 10.1016/j.biopsych.2006.11.005
Trouche, S., Koren, V., Doig, N. M., Ellender, T. J., El-Gaby, M., Lopes-Dos-Santos, V., et al. (2019). A hippocampus-accumbens tripartite neuronal motif guides appetitive memory in space. Cell 176, 1393–1406.e16. doi: 10.1016/j.cell.2018.12.037
Tsai, H. C., Zhang, F., Adamantidis, A., Stuber, G. D., Bonci, A., de Lecea, L., et al. (2009). Phasic firing in dopaminergic neurons is sufficient for behavioral conditioning. Science 324, 1080–1084. doi: 10.1126/science.1168878
Tsuboi, D., Otsuka, T., Shimomura, T., Faruk, M. O., Yamahashi, Y., Amano, M., et al. (2022). Dopamine drives neuronal excitability via KCNQ channel phosphorylation for reward behavior. Cell Rep. 40:111309. doi: 10.1016/j.celrep.2022.111309
Tu, G., Ying, L., Ye, L., Zhao, J., Liu, N., Li, J., et al. (2019). Dopamine D(1) and D(2) receptors differentially regulate Rac1 and Cdc42 signaling in the nucleus accumbens to modulate behavioral and structural plasticity after repeated methamphetamine treatment. Biol. Psychiatry 86, 820–835. doi: 10.1016/j.biopsych.2019.03.966
Tunstall, M. J., Oorschot, D. E., Kean, A., and Wickens, J. R. (2002). Inhibitory interactions between spiny projection neurons in the rat striatum. J. Neurophysiol. 88, 1263–1269. doi: 10.1152/jn.2002.88.3.1263
Vachez, Y. M., Tooley, J. R., Abiraman, K., Matikainen-Ankney, B., Casey, E., Earnest, T., et al. (2021). Ventral arkypallidal neurons inhibit accumbal firing to promote reward consumption. Nat. Neurosci. 24, 379–390. doi: 10.1038/s41593-020-00772-7
Van Bockstaele, E. J., and Pickel, V. M. (1993). Ultrastructure of serotonin-immunoreactive terminals in the core and shell of the rat nucleus accumbens: cellular substrates for interactions with catecholamine afferents. J. Comp. Neurol. 334, 603–617. doi: 10.1002/cne.903340408
van Zessen, R., Li, Y., Marion-Poll, L., Hulo, N., Flakowski, J., and Luscher, C. (2021). Dynamic dichotomy of accumbal population activity underlies cocaine sensitization. Elife 10:e66048. doi: 10.7554/eLife.66048
van Zessen, R., Phillips, J. L., Budygin, E. A., and Stuber, G. D. (2012). Activation of VTA GABA neurons disrupts reward consumption. Neuron 73, 1184–1194. doi: 10.1016/j.neuron.2012.02.016
Venton, B. J., Seipel, A. T., Phillips, P. E., Wetsel, W. C., Gitler, D., Greengard, P., et al. (2006). Cocaine increases dopamine release by mobilization of a synapsin-dependent reserve pool. J. Neurosci. 26, 3206–3209. doi: 10.1523/JNEUROSCI.4901-04.2006
Ventura, R., Morrone, C., and Puglisi-Allegra, S. (2007). Prefrontal/accumbal catecholamine system determines motivational salience attribution to both reward- and aversion-related stimuli. Proc. Natl. Acad. Sci. U. S. A. 104, 5181–5186. doi: 10.1073/pnas.0610178104
Verharen, J. P. H., Zhu, Y., and Lammel, S. (2020). Aversion hot spots in the dopamine system. Curr. Opin. Neurobiol. 64, 46–52. doi: 10.1016/j.conb.2020.02.002
Vertes, R. P., and Martin, G. F. (1988). Autoradiographic analysis of ascending projections from the pontine and mesencephalic reticular formation and the median raphe nucleus in the rat. J. Comp. Neurol. 275, 511–541. doi: 10.1002/cne.902750404
Virk, M. S., Sagi, Y., Medrihan, L., Leung, J., Kaplitt, M. G., and Greengard, P. (2016). Opposing roles for serotonin in cholinergic neurons of the ventral and dorsal striatum. Proc. Natl. Acad. Sci. U. S. A. 113, 734–739. doi: 10.1073/pnas.1524183113
Volkow, N. D., Fowler, J. S., Wang, G. J., Swanson, J. M., and Telang, F. (2007). Dopamine in drug abuse and addiction: results of imaging studies and treatment implications. Arch. Neurol. 64, 1575–1579. doi: 10.1001/archneur.64.11.1575
Volkow, N. D., Michaelides, M., and Baler, R. (2019). The neuroscience of drug reward and addiction. Physiol. Rev. 99, 2115–2140. doi: 10.1152/physrev.00014.2018
Voorn, P., Gerfen, C. R., and Groenewegen, H. J. (1989). Compartmental organization of the ventral striatum of the rat: immunohistochemical distribution of enkephalin, substance P, dopamine, and calcium-binding protein. J. Comp. Neurol. 289, 189–201. doi: 10.1002/cne.902890202
Wacker, J., Dillon, D. G., and Pizzagalli, D. A. (2009). The role of the nucleus accumbens and rostral anterior cingulate cortex in anhedonia: integration of resting EEG, fMRI, and volumetric techniques. Neuroimage 46, 327–337. doi: 10.1016/j.neuroimage.2009.01.058
Wakabayashi, K. T., Feja, M., Baindur, A. N., Bruno, M. J., Bhimani, R. V., Park, J., et al. (2019). Chemogenetic activation of ventral tegmental area GABA neurons, but not mesoaccumbal GABA terminals, disrupts responding to reward-predictive cues. Neuropsychopharmacology 44, 372–380. doi: 10.1038/s41386-018-0097-6
Walsh, J. J., Christoffel, D. J., Heifets, B. D., Ben-Dor, G. A., Selimbeyoglu, A., Hung, L. W., et al. (2018). 5-HT release in nucleus accumbens rescues social deficits in mouse autism model. Nature 560, 589–594. doi: 10.1038/s41586-018-0416-4
Wang, J., Lai, S., Wang, R., Zhou, T., Dong, N., Zhu, L., et al. (2022). Dopamine D3 receptor in the nucleus accumbens alleviates neuroinflammation in a mouse model of depressive-like behavior. Brain Behav. Immun. 101, 165–179. doi: 10.1016/j.bbi.2021.12.019
Wang, Y., Liu, Z., Cai, L., Guo, R., Dong, Y., and Huang, Y. H. (2020). A critical role of basolateral amygdala-to-nucleus accumbens projection in sleep regulation of reward seeking. Biol. Psychiatry 87, 954–966. doi: 10.1016/j.biopsych.2019.10.027
Wang, Z. J., Shwani, T., Liu, J., Zhong, P., Yang, F., Schatz, K., et al. (2022). Molecular and cellular mechanisms for differential effects of chronic social isolation stress in males and females. Mol. Psychiatry 27, 3056–3068. doi: 10.1038/s41380-022-01574-y
Warlow, S. M., Singhal, S. M., Hollon, N. G., Faget, L., Dowlat, D. S., Zell, V., et al. (2024). Mesoaccumbal glutamate neurons drive reward via glutamate release but aversion via dopamine co-release. Neuron 112, 488–499.e5. doi: 10.1016/j.neuron.2023.11.002
Warner-Schmidt, J. L., Schmidt, E. F., Marshall, J. J., Rubin, A. J., Arango-Lievano, M., Kaplitt, M. G., et al. (2012). Cholinergic interneurons in the nucleus accumbens regulate depression-like behavior. Proc. Natl. Acad. Sci. USA 109, 11360–11365. doi: 10.1073/pnas.1209293109
Watabe-Uchida, M., Zhu, L., Ogawa, S. K., Vamanrao, A., and Uchida, N. (2012). Whole-brain mapping of direct inputs to midbrain dopamine neurons. Neuron 74, 858–873. doi: 10.1016/j.neuron.2012.03.017
Weiss, F., Parsons, L. H., Schulteis, G., Hyytia, P., Lorang, M. T., Bloom, F. E., et al. (1996). Ethanol self-administration restores withdrawal-associated deficiencies in accumbal dopamine and 5-hydroxytryptamine release in dependent rats. J. Neurosci. 16, 3474–3485. doi: 10.1523/JNEUROSCI.16-10-03474.1996
West, E. A., and Carelli, R. M. (2016). Nucleus accumbens core and shell differentially encode reward-associated cues after reinforcer devaluation. J. Neurosci. 36, 1128–1139. doi: 10.1523/JNEUROSCI.2976-15.2016
Willyard, C. (2022). Psychedelic drugs take on depression. Nature 608, S36–S38. doi: 10.1038/d41586-022-02205-w
Witten, I. B., Lin, S. C., Brodsky, M., Prakash, R., Diester, I., Anikeeva, P., et al. (2010). Cholinergic interneurons control local circuit activity and cocaine conditioning. Science 330, 1677–1681. doi: 10.1126/science.1193771
Witten, I. B., Steinberg, E. E., Lee, S. Y., Davidson, T. J., Zalocusky, K. A., Brodsky, M., et al. (2011). Recombinase-driver rat lines: tools, techniques, and optogenetic application to dopamine-mediated reinforcement. Neuron 72, 721–733. doi: 10.1016/j.neuron.2011.10.028
Wood, S. K., Walker, H. E., Valentino, R. J., and Bhatnagar, S. (2010). Individual differences in reactivity to social stress predict susceptibility and resilience to a depressive phenotype: role of corticotropin-releasing factor. Endocrinology 151, 1795–1805. doi: 10.1210/en.2009-1026
Wook Koo, J., Labonte, B., Engmann, O., Calipari, E. S., Juarez, B., Lorsch, Z., et al. (2016). Essential role of mesolimbic brain-derived neurotrophic factor in chronic social stress-induced depressive behaviors. Biol. Psychiatry 80, 469–478. doi: 10.1016/j.biopsych.2015.12.009
Wright, W. J., Schluter, O. M., and Dong, Y. (2017). A feedforward inhibitory circuit mediated by CB1-expressing fast-spiking interneurons in the nucleus accumbens. Neuropsychopharmacology 42, 1146–1156. doi: 10.1038/npp.2016.275
Wu, H., Kakusa, B., Neuner, S., Christoffel, D. J., Heifets, B. D., Malenka, R. C., et al. (2022). Local accumbens in vivo imaging during deep brain stimulation reveals a strategy-dependent amelioration of hedonic feeding. Proc. Natl. Acad. Sci. U. S. A. 119:e2109269118. doi: 10.1073/pnas.2109269118
Xi, Z. X., Peng, X. Q., Li, X., Song, R., Zhang, H. Y., Liu, Q. R., et al. (2011). Brain cannabinoid CB(2) receptors modulate cocaine's actions in mice. Nat. Neurosci. 14, 1160–1166. doi: 10.1038/nn.2874
Xi, Z. X., Ramamoorthy, S., Shen, H., Lake, R., Samuvel, D. J., and Kalivas, P. W. (2003). GABA transmission in the nucleus accumbens is altered after withdrawal from repeated cocaine. J. Neurosci. 23, 3498–3505. doi: 10.1523/JNEUROSCI.23-08-03498.2003
Xiao, Q., Zhou, X., Wei, P., Xie, L., Han, Y., Wang, J., et al. (2021). A new GABAergic somatostatin projection from the BNST onto accumbal parvalbumin neurons controls anxiety. Mol. Psychiatry 26, 4719–4741. doi: 10.1038/s41380-020-0816-3
Xiu, J., Zhang, Q., Zhou, T., Zhou, T. T., Chen, Y., and Hu, H. (2014). Visualizing an emotional valence map in the limbic forebrain by TAI-FISH. Nat. Neurosci. 17, 1552–1559. doi: 10.1038/nn.3813
Yang, H., de Jong, J. W., Tak, Y., Peck, J., Bateup, H. S., and Lammel, S. (2018). Nucleus accumbens subnuclei regulate motivated behavior via direct inhibition and disinhibition of VTA dopamine subpopulations. Neuron 97, 434–449.e4. doi: 10.1016/j.neuron.2017.12.022
Yao, Y., Gao, G., Liu, K., Shi, X., Cheng, M., Xiong, Y., et al. (2021). Projections from D2 neurons in different subregions of nucleus accumbens shell to ventral pallidum play distinct roles in reward and aversion. Neurosci. Bull. 37, 623–640. doi: 10.1007/s12264-021-00632-9
Yu, J., Yan, Y., Li, K. L., Wang, Y., Huang, Y. H., Urban, N. N., et al. (2017). Nucleus accumbens feedforward inhibition circuit promotes cocaine self-administration. Proc. Natl. Acad. Sci. U. S. A. 114, E8750–E8759. doi: 10.1073/pnas.1707822114
Yuan, L., Dou, Y. N., and Sun, Y. G. (2019). Topography of reward and aversion encoding in the mesolimbic dopaminergic system. J. Neurosci. 39, 6472–6481. doi: 10.1523/JNEUROSCI.0271-19.2019
Zachry, J. E., Kutlu, M. G., Yoon, H. J., Leonard, M. Z., Chevee, M., Patel, D. D., et al. (2024). D1 and D2 medium spiny neurons in the nucleus accumbens core have distinct and valence-independent roles in learning. Neuron 112, 835–849.e7. doi: 10.1016/j.neuron.2023.11.023
Zhang, H., Chaudhury, D., Nectow, A. R., Friedman, A. K., Zhang, S., Juarez, B., et al. (2019). Alpha(1)- and beta(3)-adrenergic receptor-mediated mesolimbic homeostatic plasticity confers resilience to social stress in susceptible mice. Biol. Psychiatry 85, 226–236. doi: 10.1016/j.biopsych.2018.08.020
Zhang, L., Dong, Y., Doyon, W. M., and Dani, J. A. (2012). Withdrawal from chronic nicotine exposure alters dopamine signaling dynamics in the nucleus accumbens. Biol. Psychiatry 71, 184–191. doi: 10.1016/j.biopsych.2011.07.024
Zhang, X., Guan, W., Yang, T., Furlan, A., Xiao, X., Yu, K., et al. (2021). Genetically identified amygdala-striatal circuits for valence-specific behaviors. Nat. Neurosci. 24, 1586–1600. doi: 10.1038/s41593-021-00927-0
Zhang, M., Harrison, E., Biswas, L., Tran, T., and Liu, X. (2018). Menthol facilitates dopamine-releasing effect of nicotine in rat nucleus accumbens. Pharmacol. Biochem. Behav. 175, 47–52. doi: 10.1016/j.pbb.2018.09.004
Zhang, Y., Lu, W., Wang, Z., Zhang, R., Xie, Y., Guo, S., et al. (2020). Reduced neuronal cAMP in the nucleus accumbens damages blood-brain barrier integrity and promotes stress vulnerability. Biol. Psychiatry 87, 526–537. doi: 10.1016/j.biopsych.2019.09.027
Zhao, P., Chen, X., Bellafard, A., Murugesan, A., Quan, J., Aharoni, D., et al. (2023). Accelerated social representational drift in the nucleus accumbens in a model of autism. bioRxiv. doi: 10.1101/2023.08.05.552133
Zhao, Z. D., Han, X., Chen, R., Liu, Y., Bhattacherjee, A., Chen, W., et al. (2022). A molecularly defined D1 medium spiny neuron subtype negatively regulates cocaine addiction. Sci. Adv. 8:eabn3552. doi: 10.1126/sciadv.abn3552
Zhao, J., Ying, L., Liu, Y., Liu, N., Tu, G., Zhu, M., et al. (2019). Different roles of Rac1 in the acquisition and extinction of methamphetamine-associated contextual memory in the nucleus accumbens. Theranostics 9, 7051–7071. doi: 10.7150/thno.34655
Zhou, F. M., Liang, Y., Salas, R., Zhang, L., De Biasi, M., and Dani, J. A. (2005). Corelease of dopamine and serotonin from striatal dopamine terminals. Neuron 46, 65–74. doi: 10.1016/j.neuron.2005.02.010
Zhou, F. M., Wilson, C. J., and Dani, J. A. (2002). Cholinergic interneuron characteristics and nicotinic properties in the striatum. J. Neurobiol. 53, 590–605. doi: 10.1002/neu.10150
Zhou, K., Xu, H., Lu, S., Jiang, S., Hou, G., Deng, X., et al. (2022). Reward and aversion processing by input-defined parallel nucleus accumbens circuits in mice. Nat. Commun. 13:6244. doi: 10.1038/s41467-022-33843-3
Zhou, Y., Yan, E., Cheng, D., Zhu, H., Liu, Z., Chen, X., et al. (2020). The projection from ventral CA1, not prefrontal cortex, to nucleus accumbens core mediates recent memory retrieval of cocaine-conditioned place preference. Front. Behav. Neurosci. 14:558074. doi: 10.3389/fnbeh.2020.558074
Zhou, Y., Zhu, H., Liu, Z., Chen, X., Su, X., Ma, C., et al. (2019). A ventral CA1 to nucleus accumbens core engram circuit mediates conditioned place preference for cocaine. Nat. Neurosci. 22, 1986–1999. doi: 10.1038/s41593-019-0524-y
Zhu, X. N., Li, J., Qiu, G. L., Wang, L., Lu, C., Guo, Y. G., et al. (2023). Propofol exerts anti-anhedonia effects via inhibiting the dopamine transporter. Neuron 111, 1626–1636.e6. doi: 10.1016/j.neuron.2023.02.017
Zhu, Y., Wang, K., Ma, T., Ji, Y., Lou, Y., Fu, X., et al. (2023a). Nucleus accumbens D1/D2 circuits control opioid withdrawal symptoms in mice. J. Clin. Invest. 133:e163266. doi: 10.1172/JCI163266
Zhu, Y., Wienecke, C. F., Nachtrab, G., and Chen, X. (2016). A thalamic input to the nucleus accumbens mediates opiate dependence. Nature 530, 219–222. doi: 10.1038/nature16954
Zhu, Y., Yan, P., Wang, R., Lai, J., Tang, H., Xiao, X., et al. (2023b). Opioid-induced fragile-like regulatory T cells contribute to withdrawal. Cell 186, 591–606.e23. doi: 10.1016/j.cell.2022.12.030
Zhuo, Y., Luo, B., Yi, X., Dong, H., Miao, X., Wan, J., et al. (2024). Improved green and red GRAB sensors for monitoring dopaminergic activity in vivo. Nat. Methods 21, 680–691. doi: 10.1038/s41592-023-02100-w
Zinsmaier, A. K., Dong, Y., and Huang, Y. H. (2022). Cocaine-induced projection-specific and cell type-specific adaptations in the nucleus accumbens. Mol. Psychiatry 27, 669–686. doi: 10.1038/s41380-021-01112-2
Keywords: addiction, aversion, depression, nucleus accumbens, reward
Citation: Xu Y, Lin Y, Yu M and Zhou K (2024) The nucleus accumbens in reward and aversion processing: insights and implications. Front. Behav. Neurosci. 18:1420028. doi: 10.3389/fnbeh.2024.1420028
Edited by:
Keith B. J. Franklin, McGill University, CanadaReviewed by:
Christopher Robert Pryce, University of Zurich, SwitzerlandJasper Heinsbroek, University of Alabama at Birmingham, United States
Kabirullah Lutfy, Western University of Health Sciences, United States
Copyright © 2024 Xu, Lin, Yu and Zhou. This is an open-access article distributed under the terms of the Creative Commons Attribution License (CC BY). The use, distribution or reproduction in other forums is permitted, provided the original author(s) and the copyright owner(s) are credited and that the original publication in this journal is cited, in accordance with accepted academic practice. No use, distribution or reproduction is permitted which does not comply with these terms.
*Correspondence: Kuikui Zhou, emhvdWt1aWt1aUB1b3IuZWR1LmNu
†These authors have contributed equally to this work