- 1Department of Psychology, The University of Texas at Austin, Austin, TX, United States
- 2Department of Neurology, Dell Medical School, The University of Texas at Austin, Austin, TX, United States
- 3Institute of Mental Health Research, The University of Texas at Austin, Austin, TX, United States
There is a strong association between traumatic brain injuries (TBIs) and the development of psychiatric disorders, including post-traumatic stress disorder (PTSD). Exposure-based therapy is a first-line intervention for individuals who suffer from PTSD and other anxiety-related disorders; however, up to 50% of individuals with PTSD do not respond well to this approach. Fear extinction, a core mechanism underlying exposure-based therapy, is a procedure in which a repeated presentation of a conditioned stimulus in the absence of an unconditioned stimulus leads to a decrease in fear expression, and is a useful tool to better understand exposure-based therapy. Identifying predictors of extinction would be useful in developing alternative treatments for the non-responders. We recently found that CO2 reactivity predicts extinction phenotypes in rats, likely through the activation of orexin receptors in the lateral hypothalamus. While studies have reported mixed results in extinction of fear after TBI, none have examined the long-term durability of this phenotype in the more chronically injured brain. Here we tested the hypothesis that TBI results in a long-term deficit in fear extinction, and that CO2 reactivity would be predictive of this extinction phenotype. Isoflurane-anesthetized adult male rats received TBI (n = 59) (produced by a controlled cortical impactor) or sham surgery (n = 29). One month post-injury or sham surgery, rats underwent a CO2 or air challenge, followed by fear conditioning, extinction, and fear expression testing. TBI rats exposed to CO2 (TBI-CO2) showed no difference during extinction or fear expression relative to shams exposed to CO2 (sham-CO2). However, TBI-CO2 rats, showed significantly better fear expression than TBI rats exposed to air (TBI-air). In contrast to previous findings, we observed no relationship between CO2 reactivity and post-extinction fear expression in either the sham or TBI rats. However, compared to the previously observed naïve sample, we observed more variability in post-extinction fear expression but a very similar distribution of CO2 reactivity in the current sample. Isoflurane anesthesia may lead to interoceptive threat habituation, possibly via action on orexin receptors in the lateral hypothalamus, and may interact with CO2 exposure, resulting in enhanced extinction. Future work will directly test this possibility.
1. Introduction
According to recent data from the Centers for Disease Control and Prevention (CDC), there were approximately 223,135 traumatic brain injury (TBI)-related hospitalizations and 64,362 deaths in 2019 alone (CDC, 2022). Males were twice as likely as females to be hospitalized, with three times the risk of mortality, spanning early life to the aged population (Center for Disease Control and Prevention, 2018-2022). TBIs are well-known for their heterogeneity (Saatman et al., 2008), which is, in part, attributed to the variable nature and severity of the insult and brain regions involved. Regardless of this heterogeneity, there is a strong association between TBIs and the subsequent development of psychiatric disorders, including altered mood, psychoses, anxiety, stress, depression, substance abuse and posttraumatic stress disorders (PTSD) (Whelan-Goodinson et al., 2009; Gould et al., 2011; Koponen et al., 2011; Alway et al., 2016; Ponsford et al., 2018). PTSD frequently presents as a comorbid condition among brain-injured patients (Ponsford et al., 2018); however, several factors likely influence their association, including a history of mental illness prior to a TBI, gender, level of education, severity and type of injury, and time post-injury (Whelan-Goodinson et al., 2010; Perry et al., 2016; Ponsford et al., 2018).
Traumatic brain injury (TBI)-related PTSD has been extensively studied in the military population (Vasterling et al., 2018). As a signature of the conflicts in Iraq and Afghanistan (Okie, 2005), 43.9% of brain-injured soldiers who experienced loss of consciousness met criteria for a PTSD diagnosis (Hoge et al., 2008). Brenner et al. (2010) reported that 26% of troops, returning from Iraq with a diagnosed mild TBI, screened positive for PTSD, compared to 7% without a brain injury (Brenner et al., 2010; Wojcik et al., 2010). Furthermore, a meta-analysis of military and civilian populations found military personnel are nearly 3 times more likely to develop PTSD following a TBI than civilians (Loignon et al., 2020). The target population for these analysis are mostly male dominated as they are, in general, 40% more likely to experience a TBI (Gupte et al., 2019) and are more prominent in the military population.
Trauma-focused therapy such as prolonged exposure therapy (PE) and cognitive processing therapy (CPT) are first-line interventions for individuals who suffer from PTSD (Berg, 2008). However, meta-analyses of randomized-controlled trials (RCTs) (Lewis et al., 2020) and practice-based studies (Herzog et al., 2021) suggest that non-responder rates may be as high as 50%. Fear extinction, a procedure in which the repeated presentation of a conditioned stimulus in the absence of the unconditioned stimulus leads to a decrease in fear expression, is a core mechanism underlying exposure-based therapy, and evidence suggests that PTSD is associated with extinction deficits (Maren, 2001).
Studies have used fear conditioning to examine the impact of TBIs on fear learning in rodents, but far fewer have assessed the effects of TBIs on extinction (Meyer et al., 2012; Genovese et al., 2013; Sierra-Mercado et al., 2015; Davies et al., 2016; Schneider et al., 2016; Hoffman et al., 2019; Corne et al., 2019; Nonaka et al., 2021; Zhao et al., 2021). Within the subset of studies that did examine extinction post-injury, there is variability in outcomes, ranging from no difference in extinction (Sierra-Mercado et al., 2015), to impaired extinction (Zhao et al., 2018) or a resurgence in fear after extinction learning (Corne et al., 2019). There have also been reports of both an increase (Meyer et al., 2012; Schneider et al., 2016; Hoffman et al., 2019) or decrease in freezing during fear acquisition following injury (Genovese et al., 2013; Hoffman et al., 2019; Corne et al., 2019; Nonaka et al., 2021; see Table 1). The lack of consistency in these findings is likely due to several factors including the nature of the brain injury (focal versus diffuse), variations in fear conditioning, extinction, or both, as well as the timepoint after injury at which extinction is assessed. Because TBIs may elicit progressive neurodegeneration throughout the neuroaxis (DeKosky and Asken, 2017), the emergence of extinction deficits (and possibly PTSD) may be critically linked to time post-injury.
Although results vary as to the effect of TBI on extinction, there are substantial individual differences in the response to extinction, even among healthy subjects (Bush et al., 2007; Shumake et al., 2014, 2018). Identifying predictors of extinction would be useful in developing alternative treatments for the non-responders. There is evidence to suggest that individual differences in extinction phenotype are, in part, due to increased orexin neuronal activity in the hypothalamus (Sears et al., 2013; Sharko et al., 2017). Interestingly, these same orexin neurons are activated in the presence of CO2 inhalation (Johnson et al., 2011). Indeed, Monfils et al. (2019) found that CO2 reactivity predicts extinction phenotypes in rats, likely through the activation of orexin receptors in the lateral hypothalamus. Since CO2-exposure is associated with increased activity of orexin neurons in the lateral hypothalamus (Monfils et al., 2019), reactivity to elevated CO2 levels may serve as prognostic marker for poor extinction learning. Similarly, those with anxiety disorders display heightened emotional reactivity to a single inhalation of 35% CO2 (Telch et al., 2010). In soldiers, CO2 reactivity pre-deployment predicted the emergence of PTSD and symptoms of anxiety (but not depression) while deployed in Iraq (Telch et al., 2012). Individuals with PTSD show extinction deficits (Rothbaum and Davis, 2003), reinforcing the potential for CO2 reactivity to be a good predictor of extinction phenotypes.
In the present study, we examined brain-injured rats beginning 1 month post-injury (N = ∼29/group), a time point at which extinction deficits become evident in rats that received a TBI (Zhao et al., 2018; Corne et al., 2019). We hypothesized that TBI would result in a disruption in extinction, and that CO2 reactivity would predict variability in extinction phenotypes.
2. Materials and methods
2.1. Animals
Adult male Sprague-Dawley rats 60–70 days old (n = 88, 300–350 g, Charles River, Raleigh, NC, USA) were tri-housed in transparent polyethylene cages (27 cm × 48 cm × 20 cm) and provided with ad libitum food and water. Housing was temperature and humidity-controlled (70°F, 44% humidity) with a 12 h/12 h light/dark cycle. All procedures were approved by the University of Texas at Austin Institutional Animal Care and Use Committee. They were also in accordance with the National Institutes of Health Guide for the Care and Use of Laboratory Animals.
2.2. Experiential timeline
Rats (n = 88) underwent either a TBI or sham surgery. At 1 month post-injury (PI), animals that received TBIs were screened for reactivity to CO2 (TBI-CO2) (n = 30) or normoxic air (TBI-air) (n = 29), while all sham animals (n = 29) were screened for CO2 reactivity (sham-CO2). Then 6 days later, all groups of rats were fear conditioned using 3 tone shock (US) pairings with conditioned stimulus (CS). The next day, they received an extinction session (19 CSs without US). The day after extinction, rats were tested for fear expression using 4 CSs without US. Either 3 or 4 days later, all animals received a CO2 challenge and were sacrificed 1 h later. Brains were removed and prepared for immunohistochemistry (see Figure 1).
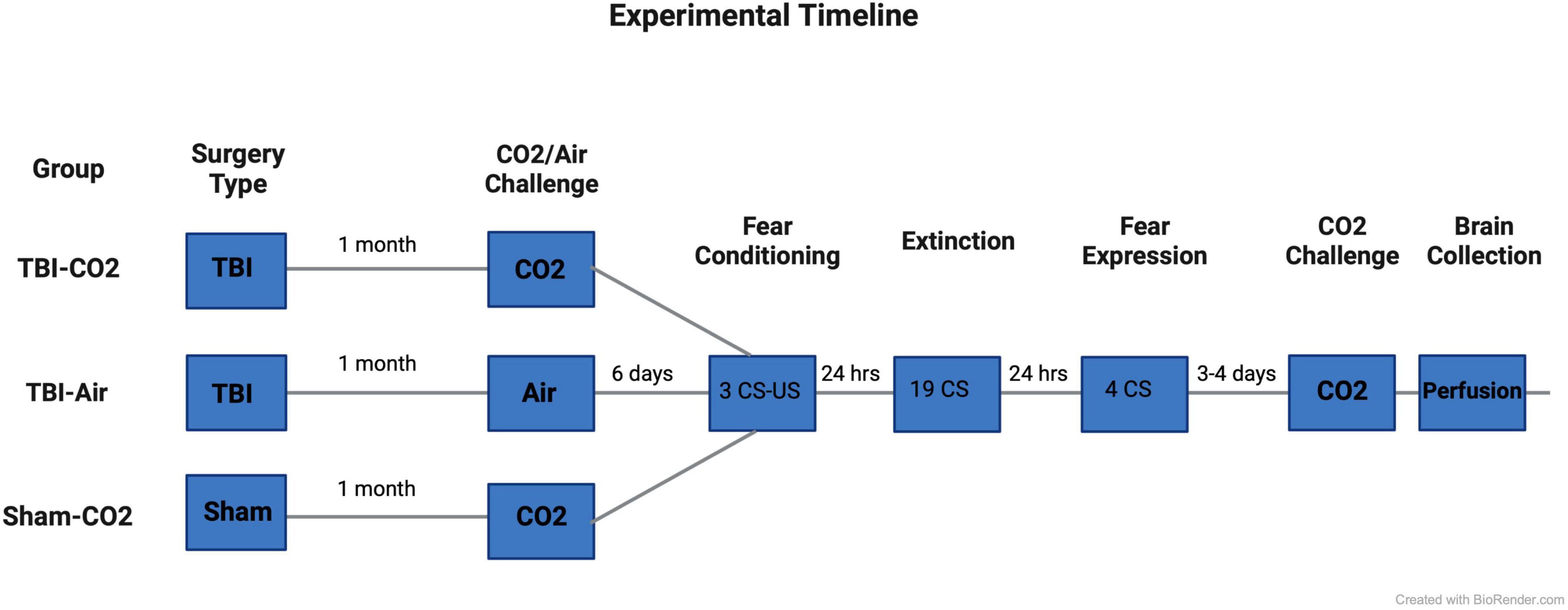
Figure 1. Experimental timeline. Rats first received either a TBI (n = 59) or sham (n = 29) surgery. 30 days post-injury, half of the TBI (TBI-CO2) animals (n = 30) and all sham (sham-CO2) controls were screened for CO2 reactivity. The remaining TBI (TBI-air) animals were screened for normoxic air (n = 29). 6 days later, all animals went through fear conditioning, extinction and fear expression separated by 24 h. 3–4 days following fear expression, all animals underwent a final CO2 screening and were then sacrificed 1 h later. Created with BioRender.com.
2.3. Controlled cortical impact
Each rat received a focal brain injury (TBI), produced by a controlled cortical impactor (CCI), as previously described (Igarashi et al., 2007; Semple et al., 2015). Briefly, the rat was anesthetized in a 4% isoflurane chamber and then positioned in a stereotaxic frame with an anesthetic mask delivering 2.5% isoflurane throughout the surgery. A midline incision was made to expose the skull followed by a circular craniectomy midway between bregma and lambda. Each animal was randomly assigned to receive either a TBI (n = 59) or sham surgery (n = 29). Injury parameters were set at 4.0 m/s velocity and a 2.0 mm depth of penetration using a 6.0 mm convex impactor tip. Sham surgery consisted of the same surgical procedures, including craniectomy, but without CCI. All rats received bupivacaine (0.25%, < 8 mg/kg, subcutaneous) locally at the incision site before craniectomy and buprenorphine (0.05 mg/kg, subcutaneous) immediately following surgery and again 6–8 h later.
2.4. Screening for CO2 reactivity
Gas was delivered through a custom built plexi-glass flow chamber (12″ width × 12″ height × 24″ length). Flow was controlled using a two-stage regulator (Praxair, Inc., Danbury, CT, USA) that delivered gas to the chamber. Ambient air entered the chamber for the first 30 s after the rat was introduced to the chamber. This was followed by a 2 min induction phase, during which 25% CO2 was infused into the chamber causing the CO2 percentage to slowly rise. CO2 was held at 25% for an additional 2 min, after which the chamber was flushed with atmospheric air allowing the CO2 percentage to return to normal levels. After 4 min of flushing with atmospheric air, the rat was transferred to its home cage (Figure 2).
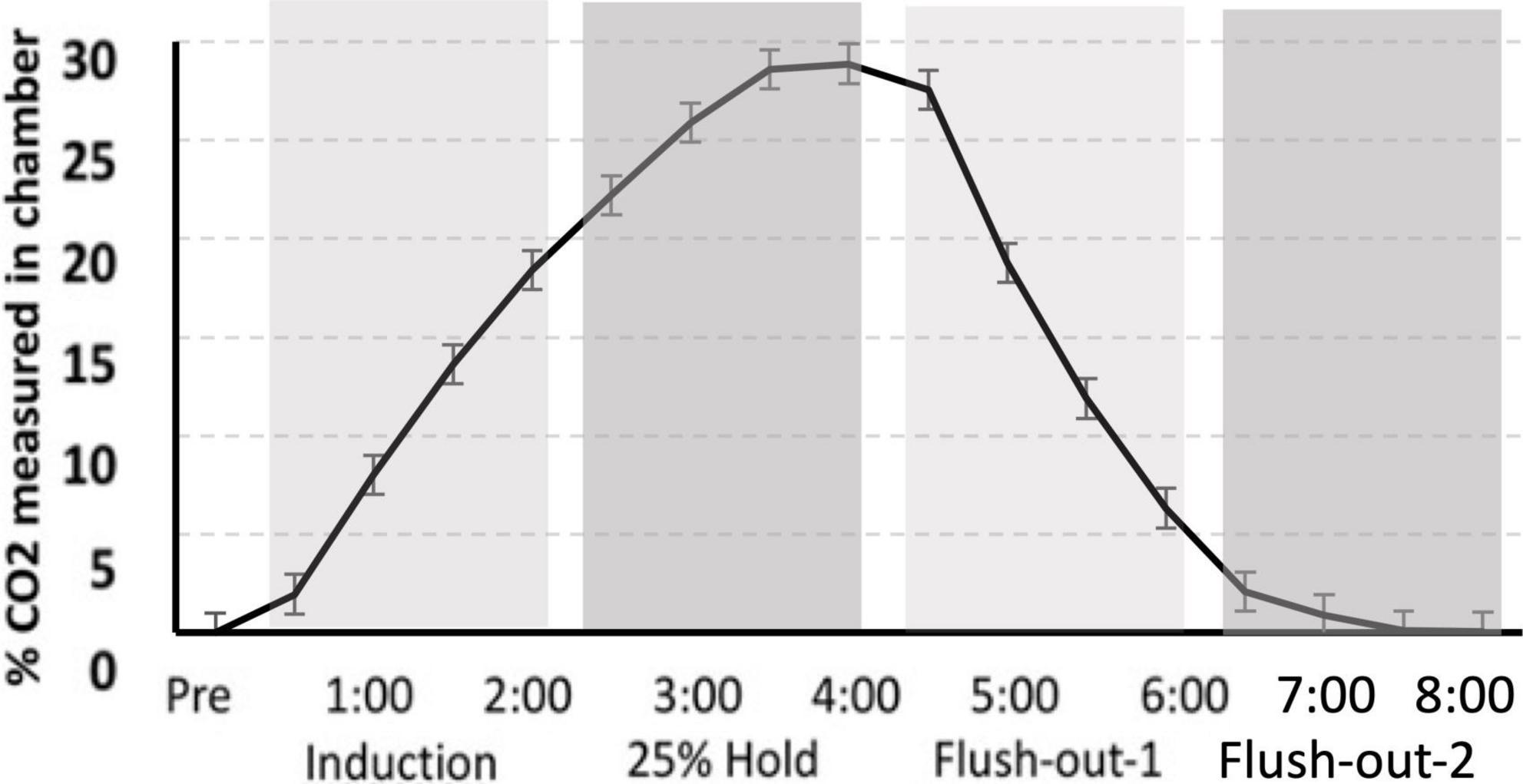
Figure 2. CO2 calibration curve. The measurement of CO2 in the chamber during “induction,” “hold,” and “flush-out” phases. Over time, CO2 was administer into the chamber, held constant around 25% and flushed out with normoxic air. Data is expressed as mean ± standard error (SE).
2.5. CO2 behavioral analyses
The scoring system for CO2 reactivity was adapted from Monfils et al. (2019). Briefly, each behavior was quantified at baseline (30 s), during CO2 induction (2 min), hold period (2 min) and during flush-out period (4 min). Behaviors were monitored through a video camera and were hand scored by an observer, blinded to the experimental condition. The following behaviors were quantified: ambulation (A), grooming (G), rearing (R), and labored breathing (L). For coding purposes, induction was referred to as phase 1, 25% hold phase 2, and the first and second half of flush-out as phases 3 and 4.
2.6. CO2 challenge and brain collection
At the end of the experiment, all rats received a CO2 challenge (as previously described above under CO2 screening). One hour post CO2 challenge, rats received a lethal dose of Euthasol (Vibric, 1 ml/200 g) and were intracardially perfused with phosphate buffered saline followed by 4% paraformaldehyde (PFA). The brains were extracted and stored in 4% PFA overnight, then transferred into 30% sucrose solution.
2.7. Apparatus
All experimental manipulations (fear conditioning, extinction, fear expression) were administered in the same context (operant conditioning chambers; Coulbourn Instruments, Whitehall, PA, USA). Chambers were equipped with stainless-steel rod floor bottoms connected to a shock generator (Model H10-11R-TC-SF; Coulbourn Instruments). All chambers were illuminated under red light. Behavior was recorded by infrared digital cameras (Panasonic, model wvBP344, Osaka, Japan), mounted on the ceiling of each unit. An automated stimulus presentation was elicited using Freezeframe2 software (Coulbourn Instruments, Whitehall, PA, USA). Between each session, chambers were cleaned with Windex (SC Johnson, Racine, WI, USA).
2.8. Fear conditioning
Rats were placed in the conditioning chambers for a 3 min habituation period followed by fear conditioning with three 20 s 5 kHz, 80 dB tones conditioned stimulus (CS). Each CS was co-terminated with a 500 ms, 0.7 mA footshock (US). The interval between each CS was on average 120 s in duration. After conditioning, rats remained in the chamber for 3 min and then were returned to the home cage.
2.9. Extinction
The day after conditioning, subjects were returned to the same conditioning chambers where they reacclimated for 3 min. This was followed by 19 CS presentations without the US, with variable intervals with a mean of 180 s. After the extinction trial, animals remained in the chamber for 3 min before returning to the homecage.
2.10. Fear expression test
The day after extinction, rats were returned to the conditioning chamber, acclimated for 3 min, then presented with 4 CSs without US. The interval between each CS was on average, 120 s in duration. Rats reminded in the chamber for 3 min before returning to the homecage.
2.11. Behavioral scoring: freezing
Freezing was defined as the absence of movement aside from breathing, scanning and ear twitching, and excluded sleeping or resting. All behaviors were scored manually by an individual who was blinded to the experimental conditions.
2.12. Quantification of lesion volume
Lesion volume, determined at 1 month post-injury, was based upon 40 μm coronal sections stained with hematoxylin and eosin. Measurements of the cortical mantel were taken from both the contralateral and ipsilateral hemispheres using a Nikon Ni-E microscope (Nikon Instruments Inc., NY, USA) spanning Bregma 1.5 to −3.8 mm. This yielded 8–10 sections per brain, using a sampling interval of 12, a 2× objective and a grid size of 400 μm. Cortical measurements were performed by an individual who was blinded to the experimental conditions. Cortical volume was estimated as the product of summed areas of sections and the distance between sections. Lesion volume was then calculated as the difference between volumes of the contralateral and ipsilateral cortices (Tennant et al., 2015; Clark et al., 2019).
2.13. Statistical analyses
R (R Development Core Team, 2018, Vienna, Austria), together with the packages beset (Shumake et al., 2018) and nlme (Pinheiro et al., 2017), were used to perform all statistical analyses. Fear acquisition, extinction and fear expression were compared between TBI-CO2 and TBI-air rats as well as TBI-CO2 and sham-CO2 groups using a repeated measures ANOVA, this data included the pre-CS. Data is expressed as mean ± standard error. Exclusion from analysis occurred if video footage was not captured completely (n = 7 Extinction).
A modified version of the “best subset” approach to linear regression was used to determine which of the CO2-reactivity behaviors accounted for the greatest portion of variance in post-extinction fear expression, the first 2 CS of fear expression, freezing. This approach fits a different linear model for every possible combination of predictor variables. We then used resampling (k-fold cross validation where k = 10) to estimate how well each model would predict new samples in terms of mean squared error (MSE). Each model was repeatedly refitted to random subsamples of data and then tested for how well it predicted the remainder of the data. The “best” model was then chosen as the one with the fewest predictors and was within one standard error of the model with the smallest MSE, i.e., the best at predicting new data. Nested cross-validation was used to avoid overly optimistic estimates of prediction error when selecting the best model.
3. Results
3.1. No differential effects between TBI and sham groups that received CO2
Rats received either a TBI (TBI-CO2) or sham (sham-CO2) surgery followed by a brief exposure to CO2 (n = 30) or a TBI (TBI-air) with an exposure to normoxic air (n = 29), one-month post-surgery, followed by fear conditioning, extinction and fear expression. TBI-air rats served as a control group to ensure there were no interacting effects of surgery and CO2 on behavior. We compared groups over the course of fear acquisition, extinction and fear expression (Figure 3). Our primary hypothesis was that TBI would result in a disruption in extinction. We first determined if there was an effect of TBI alone on the measured behaviors. We found no significant differences between TBI-CO2 and sham-CO2 groups during extinction [F(1, 51) = 0.22, p = 0.637] or fear expression [F(1, 57) = 0.114, p = 0.736]. However, a significant interaction was found between groups during fear acquisition [F(2, 114) = 6.82, p = 0.001] with a main effect between groups [F(1, 57) = 5.30, p = 0.02]. This difference seen in fear acquisition is driven by the 2nd conditioned stimulus (CS2) alone and did not persist throughout the remainder of fear conditioning nor did this difference hold up at the beginning of extinction.
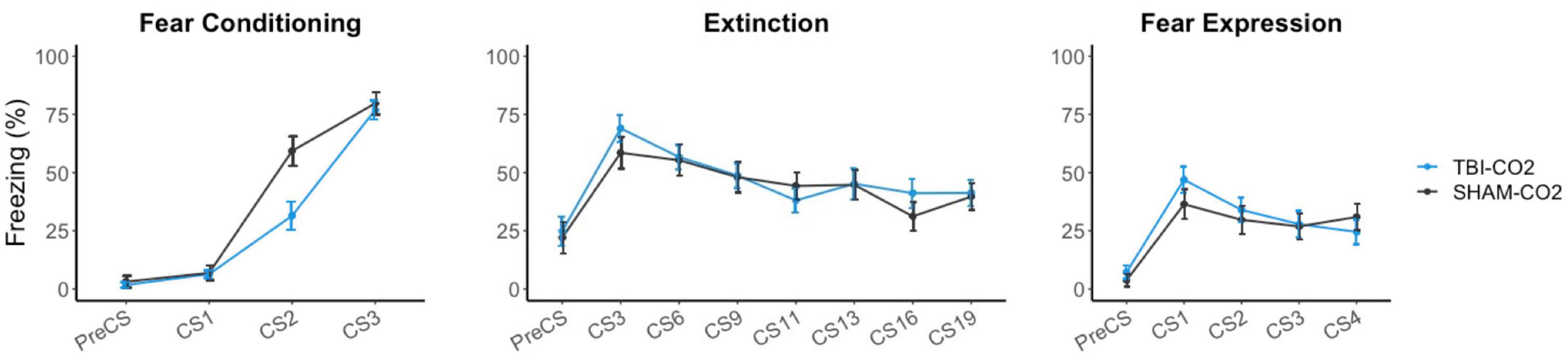
Figure 3. Effects of TBI on fear conditioning, extinction and fear expression 1-month post-injury. There was no difference between TBI-CO2 and sham-CO2 rats in percent freezing during extinction [F(1, 51) = 0.22, p = 0.637] or fear expression [F(1, 56) = 0.16, p = 0.68]. TBI-CO2 froze less during CS2 during fear acquisition [F(1, 57) = 9.31, p = 0.003] but returned to similar freezing rates as sham-CO2 rats at the end of fear acquisition and at the beginning of extinction. Data is expressed as mean ± standard error (SE).
3.2. Within TBI groups, CO2 exposure results in a decreased freezing 24 h post-extinction
A control group was used to ensure there were no interacting effects of TBI surgery and CO2 on preceding behaviors (TBI-air). We compared both groups, TBI-CO2 and TBI-air, throughout fear acquisition, extinction and fear expression (Figure 4). There was no significant difference between TBI-CO2 and TBI-air groups during both fear acquisition [F(1, 57) = 2.20, p = 0.14] and extinction [F(1, 52) = 1.22, p = 0.27]. However, TBI-CO2 rats showed a decrease in freezing during fear expression compared to TBI-air group [F(1, 57) = 4.01, p = 0.05].
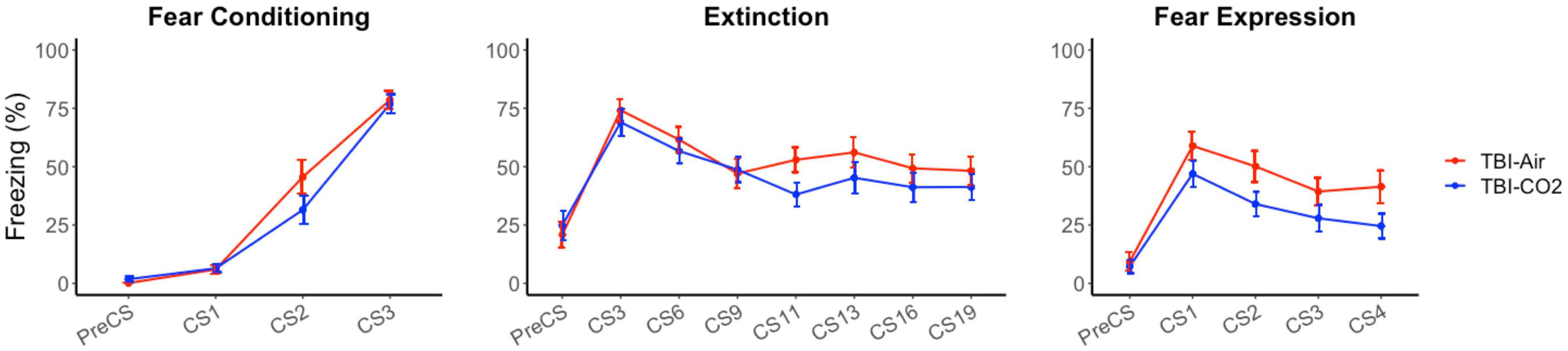
Figure 4. Effect of CO2 exposure on fear conditioning, extinction and fear expression 1-month post-injury in rats that received a TBI (TBI-CO2). The control group (TBI-air), showed no difference in percent freezing during fear conditioning or extinction than the TBI-CO2 group. However, TBI-CO2 rats froze less than the TBI-air rats during fear expression [F(1, 57) = 4.01, p = 0.05]. Data is expressed as mean ± standard error.
3.3. CO2 reactivity does not predict post-extinction fear expression in rats receiving TBI or sham surgery
We previously showed that CO2 reactivity was predictive of post-extinction fear expression behavior in naïve rats (Monfils et al., 2019). Here we tested whether CO2 reactivity can predict post-extinction fear expression in injured and sham rats. Post-extinction fear expression was defined as the mean freezing of the first two trials of fear expression. In order to determine if CO2 reactivity was a good predictor of post-extinction fear expression, we first ran a regression analysis using the previous a priori predictor separately (A3) for the TBI-CO2 and sham-CO2 groups together and separately. In Monfils et al. (2019), A3 (ambulation during the flush-out phase) had a cross-validation R2 estimate of 0.085 meaning it was assessed to be reliably good at predicting 8.5% of fear expression variance. Thus, we considered this an a priori predictor. When combining TBI-CO2 and sham-CO2 rats, A3 did not predict post-extinction fear expression (t = 0.076, p = 0.939). TBI (t = 0.909, p = 0.372) and sham groups (t = −0.609, p = 0.547) alone also showed A3 was also not a significant predictor for post-extinction fear expression. Overall, A3 alone was not a significant predictor of post-extinction fear expression.
In order to examine all of the behaviors measured during the CO2 challenge, we analyzed each group (TBI-CO2 and sham-CO2) separately and together with the best-subset approach. With these parameters, the TBI-CO2 and sham-CO2 group combined, the null (intercept-only) model was the best model selected for 97% of random subsamples. In the sham-CO2 group alone, the best model was also a null model. So, when examining the two groups combined or the sham-CO2 group alone, CO2 reactivity did not predict post-extinction fear expression.
This same approach was then used for the TBI-CO2 group to determine the best predictive effect of CO2 reactivity. The null model was selected 50% of the time. Labored breathing during flush-out-1 (L3) also was selected about 30% of the time, and explained 9.3% of the variance in the full sample, but this fell to approximately 0% of the variance in the hold-out samples. Therefore, it seems likely that this predictor is detecting something that is sample specific and is not likely to replicate.
3.4. No difference in lesion volume between groups that received a TBI
Brain injured animals were randomly assigned to 2 groups; namely, those screened for reactivity to CO2 (TBI-CO2) or normoxic air (TBI-air). Due to differences in freezing behavior, we compared lesion volumes in each of these groups (Figure 5). As this was not part of the original hypothesis, we only chose a subset of each group that upon evaluation had no artifact from brain removal or mounting. There were no significant differences in lesion volume between the group that received CO2 and the control group [t(9) = 0.88, p = 0.39].
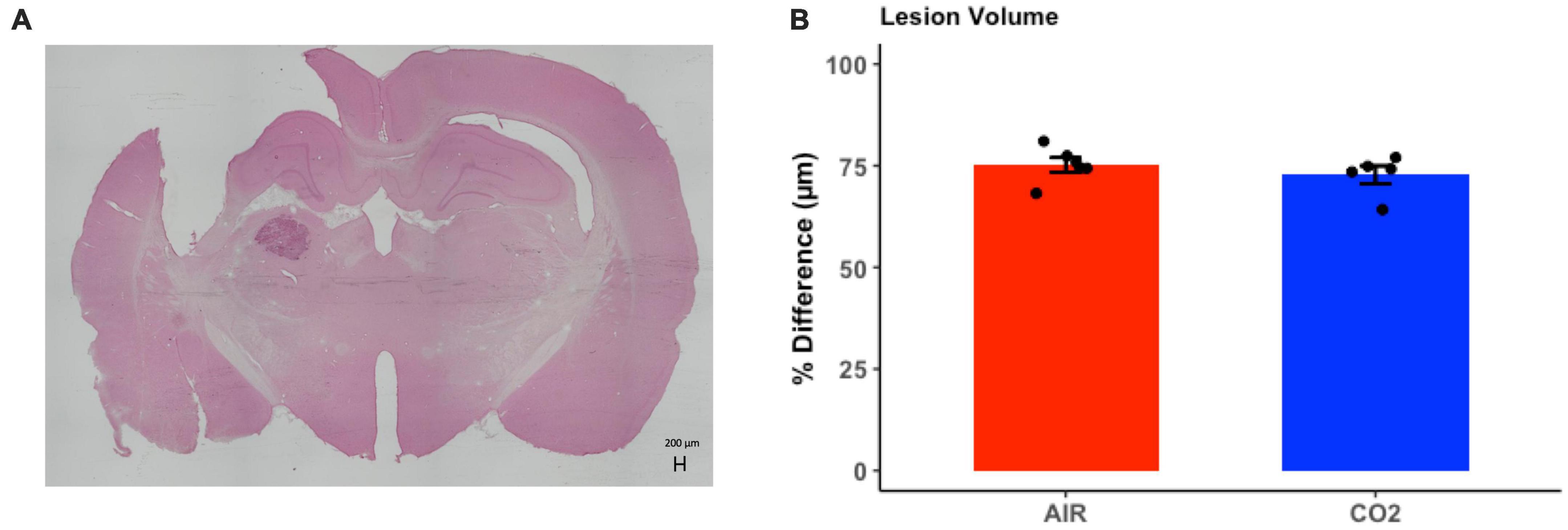
Figure 5. Quantitative assessment of lesion volume at 1-month post-injury in TBI rats. (A) Representative H&E stained coronal section, illustrating the site of maximal damage and partial loss of the cortical mantle. (B) There were no differences in in lesion volume between groups [t(9) = 0.88, p = 0.39]. Data are expressed as mean ± standard error.
3.5. Exploratory analyses
Since this study did not replicate our previous findings, which showed that CO2 was a good predictor of post-extinction fear expression in naive rats (Monfils et al., 2019), we next examined what may have been different between the 2 populations. Our aim was to use the naive rats from our 2019 study to compare the distribution of CO2 reactivity and freezing during post-extinction fear expression, and the CO2 curves between studies.
3.5.1. Shifted distribution in post-extinction fear expression and A3 compared to original naive sample
Using previous data from Monfils et al. (2019), we compared the original data distributions of the a priori predictor (A3) and post-extinction fear expression to the new distributions of sham-CO2 rats. In order for a predictive model to successfully generalize from one sample to another, a minimum requirement would be no large shifts in the observed distributions of either the covariates or the response variables. The observed measurements in this study failed to meet this basic assumption. Compared to the naïve rats in the previous study, post-extinction fear expression freezing was far more variable (SD = 32.0 vs. 14.8) and skewed more toward 0 (M = 33.1 vs. 50.7), while the measurement of A3 ambulation was skewed toward higher values (Figure 6).
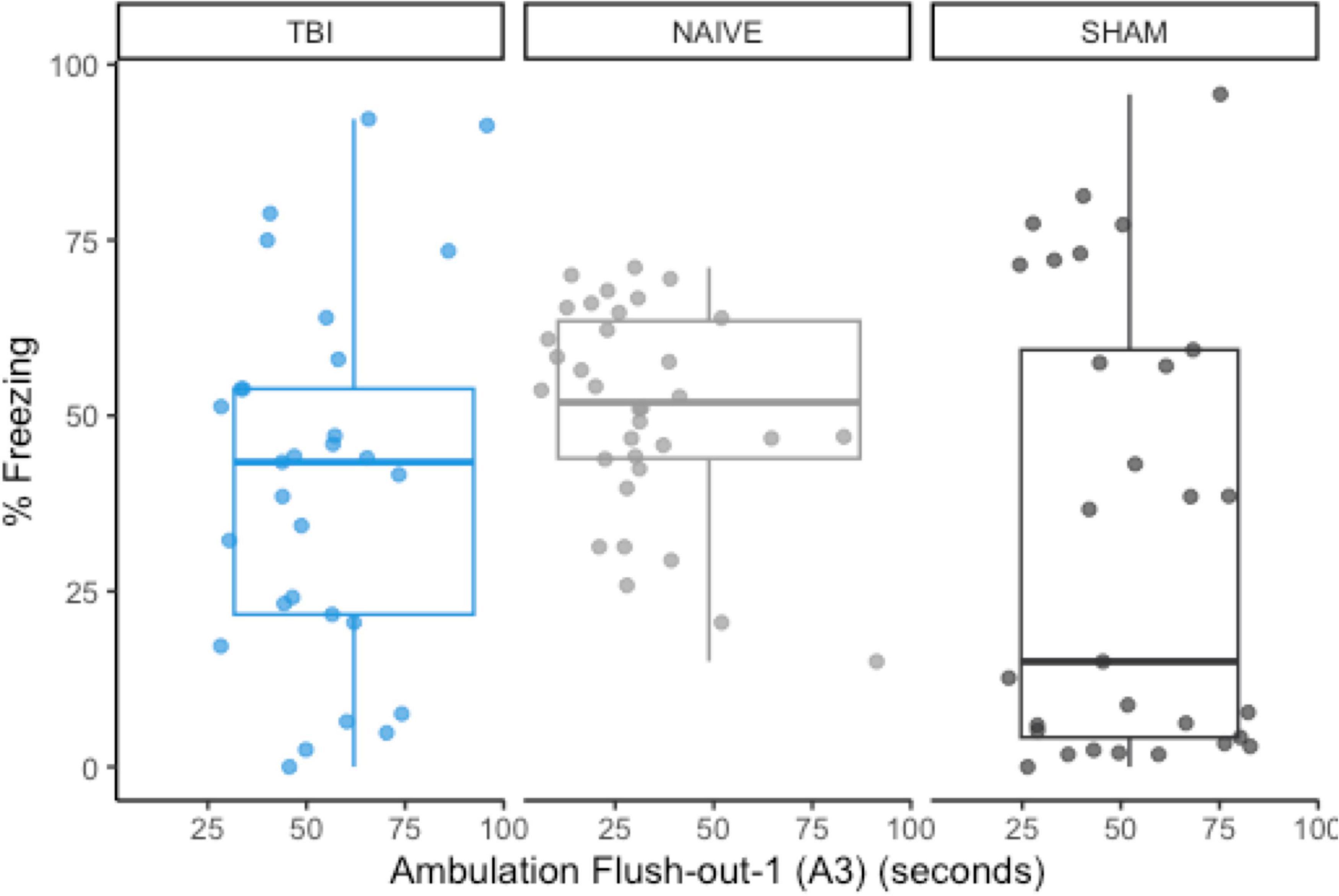
Figure 6. The group distributions for a priori predictor A3 and freezing during post-extinction fear expression using data from naïve rats (Monfils et al., 2019). There are noticeable shifts in the distribution of post-extinction fear expression, but A3 remains similarly distributed between groups.
3.5.2. CO2 reactivity is greater in current study during intro and flush out phases
We then compared the distributions of CO2 reactivity between TBI-CO2 and sham-CO2 groups, along with the naive sample previously found in Monfils et al. (2019) (Figure 7). An examination of the TBI-CO2 and sham-CO2 groups, revealed very similar findings for the measured behaviors. This is consistent in both the first CO2 challenge and at euthanasia (Figures 7, 8). The naive group, however, showed visibly lower CO2 reactivity during some behaviors, specifically during the induction and flush-out phases of ambulation, rearing and labored breathing. All of these groups showed similar deviation or spread of CO2 reactivity, meaning CO2 reactivity is defining individual variability similarly but the current study on average visibly displays more CO2 reactivity overall.
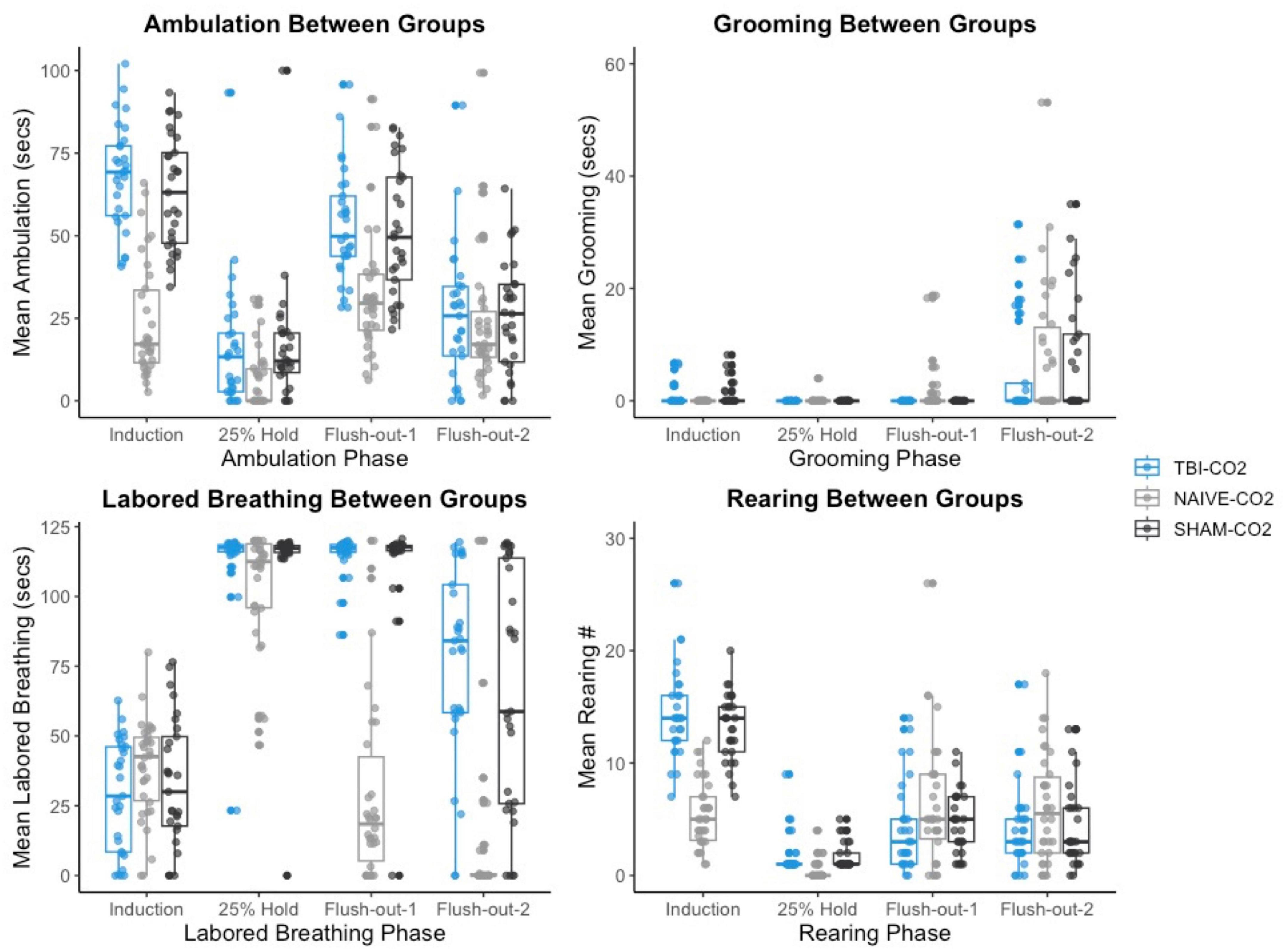
Figure 7. The comparison of measured CO2 reactivity between TBI-CO2, sham-CO2 and naive groups (from Monfils et al., 2019). The TBI-CO2 and sham-CO2 group both have very similar distributions for all of the behaviors measured. The naive group, during intro and flush-out phases within some behaviors, has on average less measured CO2 reactivity.
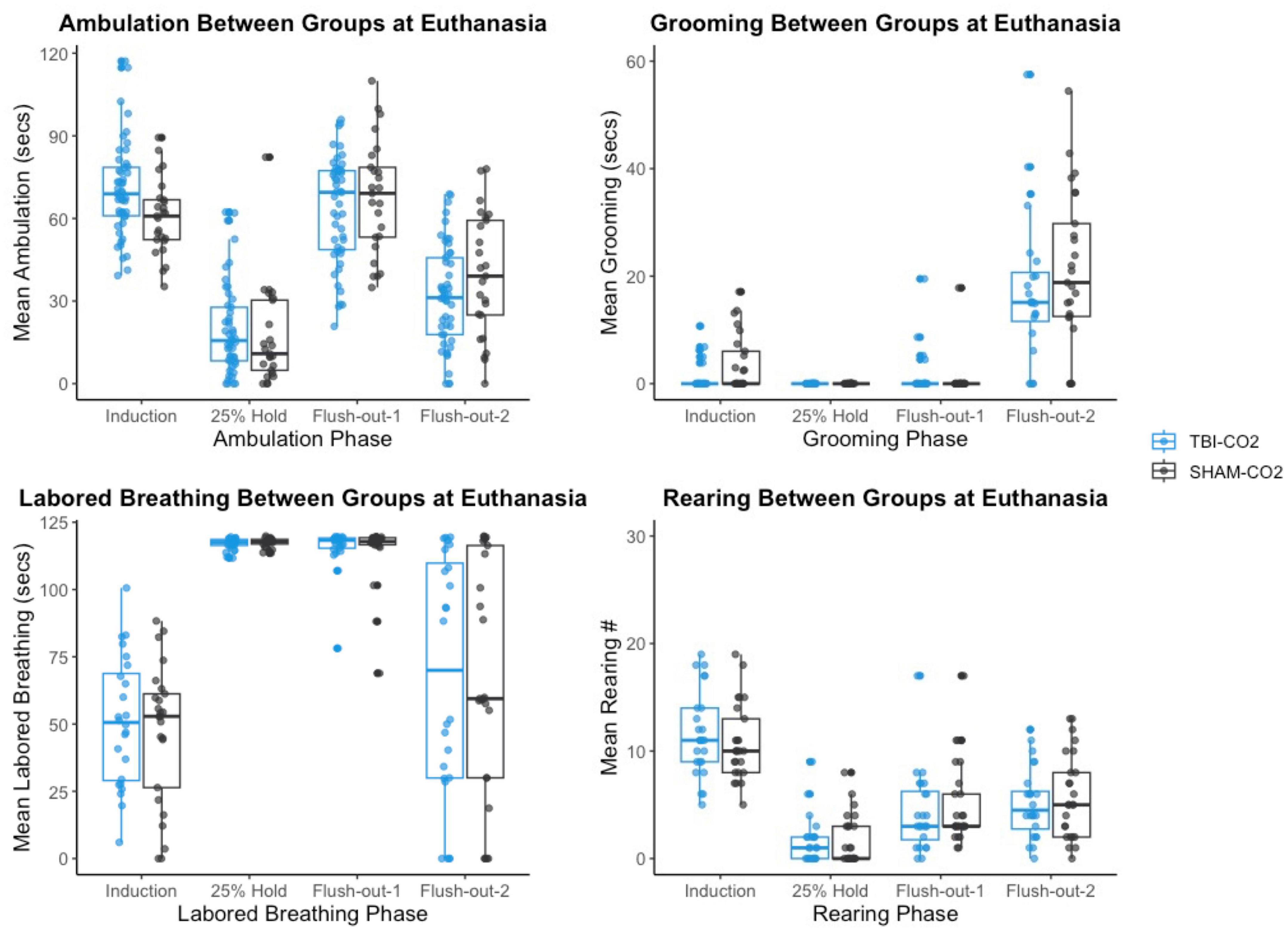
Figure 8. The comparison of measured CO2 reactivity between TBI-CO2, sham-CO2 at euthanasia. The TBI-CO2 and sham-CO2 group both have very similar distributions for all of the behaviors measured.
3.5.3. Compared to the original CO2 curve, the induction of CO2 is greater and the speed of flush out is slower
The CO2 challenge in this study was meant to replicate that seen in Monfils et al. (2019). However, there is variation in CO2 tank flow between the two studies. Despite using the same delivery protocol as we had previously described, there are differences in the actual level of CO2 measured in chamber. The hold period in this study peaks at approximately 30% max CO2 in the chamber, whereas in our previous study, the hold period peaked around 25%. Thus, CO2 in the latter is more rapidly removed during the flush-out phase. These distinctions may have led to differences in CO2 reactivity between the two studies (Figure 9).
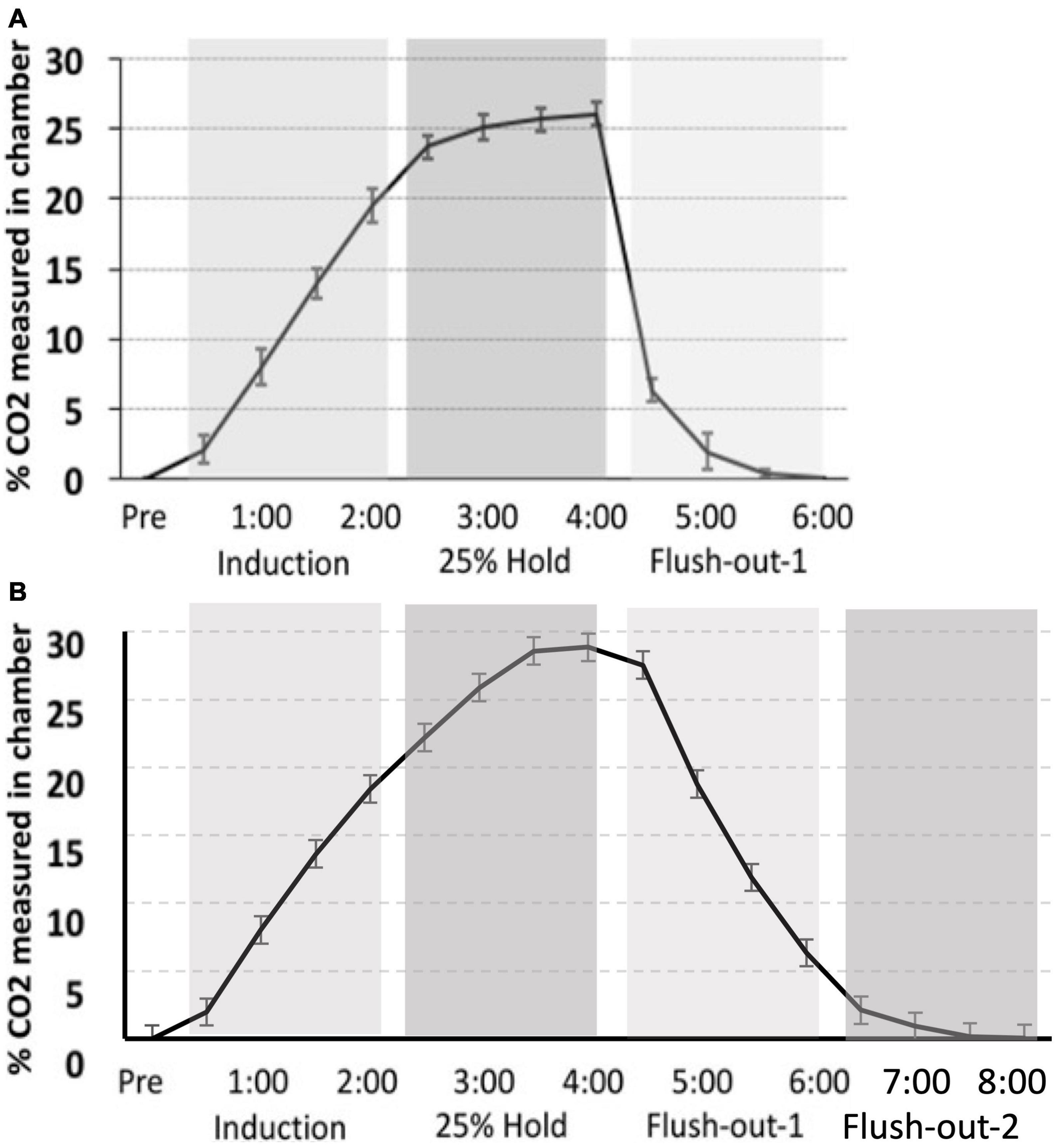
Figure 9. (A) Monfils et al. (2019) percent level of CO2 in the chamber (CO2 curve) as compared to (B) the CO2 calibration curve for the current study. Although a similar protocol was used, the overall maximum level of CO2 is higher in the current study, as well as the slower flush-out time period.
4. Discussion
This study examined the effects of TBI on the extinction of fear and determined if CO2 reactivity is a predictor of extinction variability following TBI. Contrary to our a priori hypothesis, we found that TBI alone did not have an effect on extinction, but rather the combination of CO2 and prior TBI resulted in a decrease in freezing behavior during post-extinction fear expression. We did see a significant decrease in freezing during fear conditioning in TBI rats compared to sham. CO2 reactivity did not predict variability seen in post-extinction fear expression in sham or TBI rats. These findings are at odds with our prior hypothesis, but in the context of previous literature, these results have validity.
There are a number of preclinical models of TBI that generate focal and diffuse injuries and are characterized by temporal patterns of neurodegeneration that reflect the type of injury, magnitude, and location of the initial insult (Xiong et al., 2013). As such, it is often difficult to compare behavioral findings across studies where there is inherent variability in behavioral protocols, as well as differences in sample size, the preclinical models employed including when the assays are conducted post-injury. Despite these differences, a few patterns can be extracted from the relevant studies (see Table 1). For example, studies that utilized delayed timepoints (15–28 days) reported extinction deficits in rodents after using either a lateral fluid percussion insult (Zhao et al., 2018) that induces diffuse axonal injury or a CCI (Schneider et al., 2016) that generates a focal cortical injury. These prior studies served as the basis for conducting behavioral analyses at a chronic timepoint where there would be opportunity to compare findings. We chose a CCI model of TBI for this study, because of its well established, reproducible, injury that results in a predictable pattern of neurodegenerative throughout the neuroaxis (Chen et al., 2003; Osier and Dixon, 2016). This model resulted in a decrease in freezing behavior during fear conditioning, which is consistent with another study that used a similar model of TBI (Corne et al., 2019). However, unique to our study, this effect was not sustained for the entire duration of fear conditioning, suggesting a possible delay in fear learning that diminishes over time. Our study likewise examined extinction at a chronic timepoint with a CCI model, however, our findings did not replicate previous work. This may be, in part, attributable to variability in fear conditioning and extinction across protocols.
Previous studies that have examined the effect of TBI on fear acquisition and extinction have all employed somewhat different protocols. Our approach was in line with that used in Monfils et al., 2019. Most previous studies have reported either an increase in freezing or no difference in the injured group relative to sham animals, during extinction and fear expression. However, the present study, as well as others (Hoffman et al., 2019; Nonaka et al., 2021) showed the opposite—a decrease in freezing. One common aspect of the few studies (including our own) that have shown a decrease in freezing after TBI is the repeated use of inhalants throughout the study—in the present case, isoflurane and CO2. A repeat blast model of TBI resulted in a decrease in freezing (Nonaka et al., 2021). This model of TBI requires isoflurane exposure up to four times throughout the paradigm. Although groups were not compared directly, sham animals following repeated exposure to isoflurane had overall lower freezing at three days following their last exposure than shams that received only one exposure. Our current study provides a direct comparison between brain-injured rats that have received either isoflurane and CO2 or isoflurane and air. The group that had received repeated anesthesia type inhalants also showed a decrease in freezing during fear expression.
The mechanisms that underlie fear conditioning and extinction are well established and are dependent on brain regions that are compromised in individuals suffering from TBI and PTSD. Alterations in the amygdala, hippocampus, thalamus and prefrontal cortext (PFC) result in moderation of fear conditioning and extinction (Maren, 2001). These brain regions are also vulnerable to damage following a TBI (Sato et al., 2001). Reports of fear enhancement during fear conditioning, following injury also reportedly involve increased regulation in N-methyl-D-aspartate (NMDA) receptors in the basolateral amygdala (BLA), along with an overall increase of neurons in the amygdala and a decrease of neurons in the dorsal hippocampus (Meyer et al., 2012; Reger et al., 2012). Extinction impairments following fluid percussion injury coincide with reduced spine density in layers II and III of pyramidal neurons in the hippocampus (Zhao et al., 2018). Although CCI produces focal cortical damage, subcortical regions, including the hippocampus, thalamus and amygdala, also undergo degeneration. Following a CCI, there are decreases in amygdala volume as well as white matter density in the corpus callosum, hippocampus, thalamus and amygdala, which coincide with a resurgence in extinguished fear after successful extinction (Corne et al., 2019). There are also fewer neurons within sub-regions of the hippocampus and changes in volume (Chen et al., 2003; Huang et al., 2021; Knott et al., 2021), as well as reduced GABAergic inhibition in the BLA which overlaps with the development of anxiety-like behavior (Almeida-Suhett et al., 2014). Due to the complex interaction between neurodegeneration and behavior, it is conceivable that a focal cortical injury does is not sufficient to cause the behavioral disruptions reported in diffuse models. However, our results may also have been confounded by the interacting effects of anesthesia (isoflurane) used during surgery and CO2.
There is strong evidence that that CO2 reactivity may serve as a diagnostic tool in predicting the emergence of fear related disorders. In humans, anxiety disorders display heightened reactivity to a single inhalation of 35% CO2 (Telch et al., 2010; Kellner et al., 2018). Similarly, emotional reactivity to 35% CO2 is predictive of PTSD and anxiety disorder development following deployment to Iraq in military individuals (Telch et al., 2012). In order to understand possible biological underpinnings, this was modeled in rodents. Similar to humans, rodent studies demonstrate that exposure to moderate concentrations of CO2 increase sympathetic activity (Elam et al., 1981), amplify anxiety-like behaviors (Johnson et al., 2011, 2012) and is predictive of extinction phenotypes (Monfils et al., 2019). CO2 reactivity accounts for variability found in extinction in healthy adult rats (Monfils et al., 2019). In the present study we did not replicate this finding, even in our sham animals. It bears highlighting that our only sham group for the present study received CO2 exposure. A decrease in freezing in rats that received both TBI surgery and CO2, suggests that interactions between TBI, CO2, and isoflurane interfered with the predictability of CO2-reactivity for extinction phenotype.
Indeed, the underlying mechanisms, hypothesized to explain the relationship between CO2 and extinction, are known to be affected by isoflurane exposure. Exposure to CO2 activates orexin neurons in the lateral hypothalamus (Johnson et al., 2011), which are the same neurons that account for individual differences in extinction (Sharko et al., 2017; Monfils et al., 2019). Isoflurane also inhibits these orexin neurons in the lateral hypothalamus (Kelz et al., 2008). Isoflurane has lingering effects that can induce inflammation and learning impairments up to a month after its use (Cao et al., 2012). Although the exposure to CO2 and isoflurane were a month apart, it is possible that cumulative impacts on the same neurons could have affected extinction behavior. A major confound of the repeated blast model of TBI is its repeated use of isoflurane, which may impair fear memory acquisition (Long et al., 2016). CO2, which also has the capability to act as a form of anesthesia, may create a confound in interpreting our data. It is possible that together, the repeated exposure of these inhalants could have caused a form of habituation to interoceptive threat that acted via orexinergic neurons in the lateral hypothalamus.
In summary, this study is the first to utilize a chronic, focal model of TBI to examine CO2 as a diagnostic tool to explain variability in extinction in the degenerating neuroaxis in male rats. However, the interacting effects of prior TBI surgery, including isoflurane exposure, and CO2 have made it difficult to reach definitive conclusions regarding the impacts of TBI on the predictive relationship between CO2 reactivity and fear extinction. Recognizing the limitation of studying male rats only, future work should consider comparative studies of both sexes to determine if this interaction between CO2 and isoflurane may yield similar interoceptive threat habituation, resulting in better extinction when exposed to both inhalants.
Data availability statement
The datasets presented in this study can be found in online repositories. All raw data files are available in the Monfils Lab repository, housed in the Texas Data Repository in Dataverse https://dataverse.tdl.org/dataverse/monfilsfearmemorylab. Data is also publicly available at Open Data Commons for Traumatic Brain Injury (ODC-TBI) https://odc-tbi.org.
Ethics statement
The animal study was reviewed and approved by the University of Texas at Austin Institutional Animal Care and Use Committee and were in accordance with the National Institutes of Health Guide for the Care and Use of Laboratory Animals, and are in line with the ARRIVE guidelines.
Author contributions
MM, LN-H, and KS designed the study. KS carried out the study, SM, VR, MR, and MD provided technical assistance. JS provided statistical assistance. All authors contributed to the article and approved the submitted version.
Conflict of interest
The authors declare that the research was conducted in the absence of any commercial or financial relationships that could be construed as a potential conflict of interest.
Publisher’s note
All claims expressed in this article are solely those of the authors and do not necessarily represent those of their affiliated organizations, or those of the publisher, the editors and the reviewers. Any product that may be evaluated in this article, or claim that may be made by its manufacturer, is not guaranteed or endorsed by the publisher.
References
Almeida-Suhett, C. P., Prager, E. M., Pidoplichko, V., Figueiredo, T. H., Marini, A. M., Li, Z., et al. (2014). Reduced GABAergic inhibition in the basolateral amygdala and the development of anxiety-like behaviors after mild traumatic brain injury. PLoS One 9:e102627. doi: 10.1371/journal.pone.0102627
Alway, Y., Gould, K. R., Johnston, L., McKenzie, D., and Ponsford, J. (2016). A prospective examination of Axis I psychiatric disorders in the first 5 years following moderate to severe traumatic brain injury. Psychol. Med. 46, 1331–1341. doi: 10.1017/s0033291715002986
Berg, A. O. (2008). Treatment of posttraumatic stress disorder: an assessment of the evidence, Institute of Medicine. Washington, DC: The National Academies Press.
Brenner, L. A., Ivins, B. J., Schwab, K., Warden, D., Nelson, L. A., Jaffee, M., et al. (2010). Traumatic brain injury, posttraumatic stress disorder, and postconcussive symptom reporting among troops returning from iraq. J. Head Trauma Rehabil. 25, 307–312. doi: 10.1097/HTR.0b013e3181cada03
Bush, D. E., Sotres-Bayon, F., and LeDoux, J. E. (2007). Individual differences in fear: isolating fear reactivity and fear recovery phenotypes. J. Trauma Stress 20, 413–422. doi: 10.1002/jts.20261
Cao, L., Li, L., Lin, D., and Zuo, Z. (2012). Isoflurane induces learning impairment that is mediated by interleukin 1β in rodents. PLoS One 7:e51431. doi: 10.1371/journal.pone.0051431
Center for Disease Control and Prevention (2018-2022). QuickStats: Rate of unintentional traumatic brain injury–related deaths among persons aged ≤19 years, by age group and sex — National Vital statistics system, United States, 2018-2020. MMWR Morb. Mortal. Wkly. Rep. 71:437. doi: 10.15585/mmwr.mm7111a5
Chen, S., Pickard, J. D., and Harris, N. G. (2003). Time course of cellular pathology after controlled cortical impact injury. Exp. Neurol. 182, 87–102. doi: 10.1016/s0014-4886(03)00002-5
Clark, T. A., Sullender, C., Kazmi, S. M., Speetles, B. L., Williamson, M. R., Palmberg, D. M., et al. (2019). Artery targeted photothrombosis widens the vascular penumbra, instigates peri-infarct neovascularization and models forelimb impairments. Sci. Rep. 9:2323. doi: 10.1038/s41598-019-39092-7
Corne, R., Leconte, C., Ouradou, M., Fassina, V., Zhu, Y., Déou, E., et al. (2019). Spontaneous resurgence of conditioned fear weeks after successful extinction in brain injured mice. Prog. Neuropsychopharmacol. Biol. Psychiatry 88, 276–286. doi: 10.1016/j.pnpbp.2018.07.023
Davies, D. R., Olson, D., Meyer, D. L., Scholl, J. L., Watt, M. J., Manzerra, P., et al. (2016). Mild traumatic brain injury with social defeat stress alters anxiety, contextual fear extinction, and limbic monoamines in adult rats. Front. Behav. Neurosci. 10:71. doi: 10.3389/fnbeh.2016.00071
DeKosky, S. T., and Asken, B. M. (2017). Injury cascades in TBI-related neurodegeneration. Brain Injury 31, 1177–1182.
Elam, M., Yao, T., Thorén, P., and Svensson, T. H. (1981). Hypercapnia and hypoxia: chemoreceptor-mediated control of locus coeruleus neurons and splanchnic, sympathetic nerves. Brain Res. 222, 373–381. doi: 10.1016/0006-8993(81)91040-4
Genovese, R. F., Simmons, L. P., Ahlers, S. T., Maudlin-Jeronimo, E., Dave, J. R., and Boutte, A. M. (2013). Effects of mild TBI from repeated blast overpressure on the expression and extinction of conditioned fear in rats. Neuroscience 254, 120–129. doi: 10.1016/j.neuroscience.2013.09.021
Gould, K. R., Ponsford, J. L., Johnston, L., and Schönberger, M. (2011). The nature, frequency and course of psychiatric disorders in the first year after traumatic brain injury: a prospective study. Psychol. Med. 41, 2099–2109. doi: 10.1017/s003329171100033x
Gupte, R., Brooks, W., Vukas, R., Pierce, J., and Harris, J. (2019). Sex differences in traumatic brain injury: what we know and what we should know. J. Neurotrauma 36, 3063–3091. doi: 10.1089/neu.2018.6171
Herzog, P., Voderholzer, U., Gärtner, T., Osen, B., Svitak, M., Doerr, R., et al. (2021). Predictors of outcome during inpatient psychotherapy for posttraumatic stress disorder: a single-treatment, multi-site, practice-based study. Psychother. Res. 31, 468–482. doi: 10.1080/10503307.2020.1802081
Hoffman, A. N., Lam, J., Hovda, D. A., Giza, C. C., and Fanselow, M. S. (2019). Sensory sensitivity as a link between concussive traumatic brain injury and PTSD. Sci. Rep. 9, 1–11.
Hoge, C. W., McGurk, D., Thomas, J. L., Cox, A. L., Engel, C. C., and Castro, C. A. (2008). Mild traumatic brain injury in U.S. Soldiers returning from Iraq. N. Engl. J. Med. 358, 453–463. doi: 10.1056/NEJMoa072972
Huang, P. S., Tsai, P. Y., Yang, L. Y., Lecca, D., Luo, W., Kim, D. S., et al. (2021). 3,6’-Dithiopomalidomide ameliorates hippocampal neurodegeneration, microgliosis and astrogliosis and improves cognitive behaviors in rats with a moderate traumatic brain injury. Int. J. Mol. Sci. 22:8276. doi: 10.3390/ijms22158276
Igarashi, T., Potts, M. B., and Noble-Haeusslein, L. J. (2007). Injury severity determines Purkinje cell loss and microglial activation in the cerebellum after cortical contusion injury. Exp. Neurol. 203, 258–268. doi: 10.1016/j.expneurol.2006.08.030
Johnson, P. L., Fitz, S. D., Hollis, J. H., Moratalla, R., Lightman, S. L., Shekhar, A., et al. (2011). Induction of c-Fos in ‘panic/defence’-related brain circuits following brief hypercarbic gas exposure. J. Psychopharmacol. 25, 26–36. doi: 10.1177/0269881109353464
Johnson, P. L., Samuels, B. C., Fitz, S. D., Lightman, S. L., Lowry, C. A., and Shekhar, A. (2012). Activation of the orexin 1 receptor is a critical component of CO2-mediated anxiety and hypertension but not bradycardia. Neuropsychopharmacology 37, 1911–1922. doi: 10.1038/npp.2012.38
Kellner, M., Muhtz, C., Nowack, S., Leichsenring, I., Wiedemann, K., and Yassouridis, A. (2018). Effects of 35% carbon dioxide (CO(2)) inhalation in patients with post-traumatic stress disorder (PTSD): a double-blind, randomized, placebo-controlled, cross-over trial. J. Psychiatr. Res. 96, 260–264. doi: 10.1016/j.jpsychires.2017.10.019
Kelz, M. B., Sun, Y., Chen, J., Cheng Meng, Q., Moore, J. T., Veasey, S. C., et al. (2008). An essential role for orexins in emergence from general anesthesia. Proc. Natl. Acad. Sci. U.S.A. 105, 1309–1314. doi: 10.1073/pnas.0707146105
Knott, M. V., Ngwenya, L. B., Correll, E. A., Bohnert, J., Ziemba, N. J., Allgire, E., et al. (2021). Lack of glutamate receptor subunit expression changes in hippocampal dentate gyrus after experimental traumatic brain injury in a rodent model of depression. Int. J. Mol. Sci. 22:8086. doi: 10.3390/ijms22158086
Koponen, S., Taiminen, T., Hiekkanen, H., and Tenovuo, O. (2011). Axis I and II psychiatric disorders in patients with traumatic brain injury: a 12-month follow-up study. Brain Inj. 25, 1029–1034. doi: 10.3109/02699052.2011.607783
Lewis, C., Roberts, N. P., Gibson, S., and Bisson, J. I. (2020). Dropout from psychological therapies for post-traumatic stress disorder (PTSD) in adults: systematic review and meta-analysis. Eur. J. Psychotraumatol. 11:1709709.
Loignon, A., Ouellet, M. C., and Belleville, G. (2020). A systematic review and meta-analysis on PTSD following TBI among military/veteran and civilian populations. J. Head Trauma Rehabil. 35, E21–E35. doi: 10.1097/htr.0000000000000514
Long, R. P., Aroniadou-Anderjaska, V., Prager, E. M., Pidoplichko, V. I., Figueiredo, T. H., and Braga, M. F. (2016). Repeated isoflurane exposures impair long-term potentiation and increase basal GABAergic activity in the basolateral amygdala. Neural Plasticity 2016:8524560. doi: 10.1155/2016/8524560
Maren, S. (2001). Neurobiology of Pavlovian fear conditioning. Annu. Rev. Neurosci. 24, 897–931. doi: 10.1146/annurev.neuro.24.1.897
Meyer, D. L., Davies, D. R., Barr, J. L., Manzerra, P., and Forster, G. L. (2012). Mild traumatic brain injury in the rat alters neuronal number in the limbic system and increases conditioned fear and anxiety-like behaviors. Exp. Neurol. 235, 574–587. doi: 10.1016/j.expneurol.2012.03.012
Monfils, M. H., Lee, H. J., Keller, N. E., Roquet, R. F., Quevedo, S., Agee, L., et al. (2019). Predicting extinction phenotype to optimize fear reduction. Psychopharmacology 236, 99–110. doi: 10.1007/s00213-018-5005-6
Nonaka, M., Taylor, W. W., Bukalo, O., Tucker, L. B., Fu, A. H., Kim, Y., et al. (2021). Behavioral and myelin-related abnormalities after blast-induced mild traumatic brain injury in mice. J. Neurotrauma 38, 1551–1571. doi: 10.1089/neu.2020.7254
Okie, S. (2005). Traumatic brain injury in the war zone. N. Engl. J. Med. 352, 2043–2047. doi: 10.1056/NEJMp058102
Osier, N. D., and Dixon, C. E. (2016). The controlled cortical impact model: applications, considerations for researchers, and future directions. Front. Neurol. 7:134. doi: 10.3389/fneur.2016.00134
Perry, D. C., Sturm, V. E., Peterson, M. J., Pieper, C. F., Bullock, T., Boeve, B. F., et al. (2016). Association of traumatic brain injury with subsequent neurological and psychiatric disease: a meta-analysis. J. Neurosurg. 124, 511–526. doi: 10.3171/2015.2.Jns14503
Pinheiro, J., Bates, D., DebRoy, S., Sarkar, D., Heisterkamp, S., Van Willigen, B., et al. (2017). Package ‘nlme’. Linear and nonlinear mixed effects models, version 3.
Ponsford, J., Alway, Y., and Gould, K. R. (2018). Epidemiology and natural history of psychiatric disorders after TBI. J. Neuropsychiatry Clin. Neurosci. 30, 262–270. doi: 10.1176/appi.neuropsych.18040093
R Development Core Team. (2018). R: A language and enviorment for statistical computing. Vienna: R Foundation for Statistical Computing.
Reger, M. L., Poulos, A. M., Buen, F., Giza, C. C., Hovda, D. A., and Fanselow, M. S. (2012). Concussive brain injury enhances fear learning and excitatory processes in the amygdala. Biol. Psychiatry 71, 335–343. doi: 10.1016/j.biopsych.2011.11.007
Rothbaum, B. O., and Davis, M. (2003). Applying learning principles to the treatment of post-trauma reactions. Ann. N.Y. Acad. Sci. 1008, 112–121. doi: 10.1196/annals.1301.012
Saatman, K. E., Duhaime, A. C., Bullock, R., Maas, A. I., Valadka, A., and Manley, G. T. (2008). Classification of traumatic brain injury for targeted therapies. J. Neurotrauma 25, 719–738. doi: 10.1089/neu.2008.0586
Sato, M., Chang, E., Igarashi, T., and Noble, L. J. (2001). Neuronal injury and loss after traumatic brain injury: time course and regional variability. Brain Res. 917, 45–54. doi: 10.1016/s0006-8993(01)02905-5
Schneider, B. L., Ghoddoussi, F., Charlton, J. L., Kohler, R. J., Galloway, M. P., Perrine, S. A., et al. (2016). Increased cortical gamma-aminobutyric acid precedes incomplete extinction of conditioned fear and increased hippocampal excitatory tone in a mouse model of mild traumatic brain injury. J. Neurotrauma 33, 1614–1624. doi: 10.1089/neu.2015.4190
Sears, R. M., Fink, A. E., Wigestrand, M. B., Farb, C. R., De Lecea, L., and LeDoux, J. E. (2013). Orexin/hypocretin system modulates amygdala-dependent threat learning through the locus coeruleus. Proc. Natl. Acad. Sci. U.S.A. 110, 20260–20265. doi: 10.1073/pnas.1320325110
Semple, B. D., Trivedi, A., Gimlin, K., and Noble-Haeusslein, L. J. (2015). Neutrophil elastase mediates acute pathogenesis and is a determinant of long-term behavioral recovery after traumatic injury to the immature brain. Neurobiol. Dis. 74, 263–280. doi: 10.1016/j.nbd.2014.12.003
Sharko, A. C., Fadel, J. R., Kaigler, K. F., and Wilson, M. A. (2017). Activation of orexin/hypocretin neurons is associated with individual differences in cued fear extinction. Physiol. Behav. 178, 93–102. doi: 10.1016/j.physbeh.2016.10.008
Shumake, J., Furgeson-Moreira, S., and Monfils, M. H. (2014). Predictability and heritability of individual differences in fear learning. Anim. Cogn. 17, 1207–1221. doi: 10.1007/s10071-014-0752-1
Shumake, J., Jones, C., Auchter, A., and Monfils, M. H. (2018). Data-driven criteria to assess fear remission and phenotypic variability of extinction in rats. Philos. Trans. R. Soc. Lond. B. Biol. Sci. 373:20170035. doi: 10.1098/rstb.2017.0035
Sierra-Mercado, D., McAllister, L. M., Lee, C. C., Milad, M. R., Eskandar, E. N., and Whalen, M. J. (2015). Controlled cortical impact before or after fear conditioning does not affect fear extinction in mice. Brain Res. 1606, 133–141. doi: 10.1016/j.brainres.2015.02.031
Telch, M. J., Rosenfield, D., Lee, H. J., and Pai, A. (2012). Emotional reactivity to a single inhalation of 35% carbon dioxide and its association with later symptoms of posttraumatic stress disorder and anxiety in soldiers deployed to Iraq. Arch. Gen. Psychiatry 69, 1161–1168. doi: 10.1001/archgenpsychiatry.2012.8
Telch, M. J., Smits, J. A., Brown, M., Dement, M., Powers, M. B., Lee, H., et al. (2010). Effects of threat context and cardiac sensitivity on fear responding to a 35% CO2 challenge: a test of the context-sensitivity panic vulnerability model. J. Behav. Ther. Exp. Psychiatry 41, 365–372. doi: 10.1016/j.jbtep.2010.03.008
Tennant, K. A., Kerr, A. L., Adkins, D. L., Donlan, N., Thomas, N., Kleim, J. A., et al. (2015). Age-dependent reorganization of peri-infarct “premotor” cortex with task-specific rehabilitative training in mice. Neurorehabil. Neural Repair 29, 193–202. doi: 10.1177/1545968314541329
Vasterling, J. J., Jacob, S. N., and Rasmusson, A. (2018). Traumatic brain injury and posttraumatic stress disorder: conceptual, diagnostic, and therapeutic considerations in the context of co-occurrence. J. Neuropsychiatry Clin. Neurosci. 30, 91–100. doi: 10.1176/appi.neuropsych.17090180
Whelan-Goodinson, R., Ponsford, J. L., Schönberger, M., and Johnston, L. (2010). Predictors of psychiatric disorders following traumatic brain injury. J. Head Trauma Rehabil. 25, 320–329. doi: 10.1097/HTR.0b013e3181c8f8e7
Whelan-Goodinson, R., Ponsford, J., Johnston, L., and Grant, F. (2009). Psychiatric disorders following traumatic brain injury: their nature and frequency. J Head Trauma Rehabil 24, 324–332. doi: 10.1097/HTR.0b013e3181a712aa
Wojcik, B. E., Stein, C. R., Bagg, K., Humphrey, R. J., and Orosco, J. (2010). Traumatic brain injury hospitalizations of US army soldiers deployed to Afghanistan and Iraq. Am. J. Prev. Med. 38, S108–S116.
Xiong, Y., Mahmood, A., and Chopp, M. (2013). Animal models of traumatic brain injury. Nat. Rev. Neurosci. 14, 128–142. doi: 10.1038/nrn3407
Zhao, J., Huynh, J., Hylin, M. J., O’Malley, J. J., Perez, A., Moore, A. N., et al. (2018). Mild traumatic brain injury reduces spine density of projection neurons in the medial prefrontal cortex and impairs extinction of contextual fear memory. J. Neurotrauma 35, 149–156. doi: 10.1089/neu.2016.4898
Keywords: traumatic brain injury, CO2, fear conditioning, extinction, individual differences
Citation: Smith KA, Raskin MR, Donovan MH, Raghunath V, Mansoorshahi S, Telch MJ, Shumake J, Noble-Haeusslein LJ and Monfils MH (2023) Examining the long-term effects of traumatic brain injury on fear extinction in male rats. Front. Behav. Neurosci. 17:1206073. doi: 10.3389/fnbeh.2023.1206073
Received: 14 April 2023; Accepted: 26 May 2023;
Published: 16 June 2023.
Edited by:
Seth Davin Norrholm, Wayne State University, United StatesReviewed by:
Christopher Cain, Nathan Kline Institute for Psychiatric Research, United StatesCraig Weiss, Northwestern University, United States
Christopher Olsen, Medical College of Wisconsin, United States
Jeff L. Weiner, Wake Forest University, United States
Shane Alan Perrine, Wayne State University, United States
Srini Kallakuri, Wayne State University, United States in collaboration with reviewer SP
Stefano Gaburro, Tecniplast, Italy
Copyright © 2023 Smith, Raskin, Donovan, Raghunath, Mansoorshahi, Telch, Shumake, Noble-Haeusslein and Monfils. This is an open-access article distributed under the terms of the Creative Commons Attribution License (CC BY). The use, distribution or reproduction in other forums is permitted, provided the original author(s) and the copyright owner(s) are credited and that the original publication in this journal is cited, in accordance with accepted academic practice. No use, distribution or reproduction is permitted which does not comply with these terms.
*Correspondence: M. H. Monfils, TWFyaWUubW9uZmlsc0B1dGV4YXMuZWR1