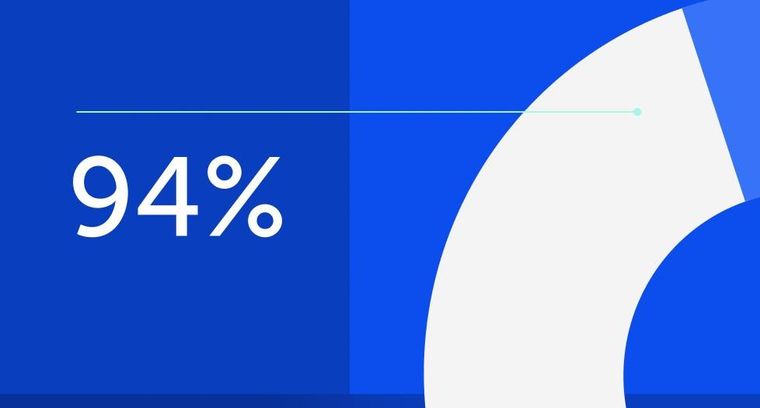
94% of researchers rate our articles as excellent or good
Learn more about the work of our research integrity team to safeguard the quality of each article we publish.
Find out more
REVIEW article
Front. Behav. Neurosci., 24 March 2023
Sec. Motivation and Reward
Volume 17 - 2023 | https://doi.org/10.3389/fnbeh.2023.1123733
This article is part of the Research TopicInsights in Motivation and Reward: 2022View all 7 articles
Over the past 20 years there has been an increasing number of brain imaging studies on the mechanisms underlying reward motivation in humans. This narrative review describes studies on the neural mechanisms associated with reward motivation and their relationships with cognitive function in healthy human participants. The brain’s meso-limbic dopamine reward circuitry in humans is known to control reward-motivated behavior in humans. The medial and lateral Pre-Frontal Cortex (PFC) integrate motivation and cognitive control during decision-making and the dorsolateral PFC (dlPFC) integrates and transmits signals of reward to the mesolimbic and meso-cortical dopamine circuits and initiates motivated behavior. The thalamus and insula influence incentive processing in humans and the motor system plays a role in response to action control. There are reciprocal relationships between reward motivation, learning, memory, imagery, working memory, and attention. The most common method of assessing reward motivation is the monetary incentive delay task (DMRT) and there are several meta-analyses of this paradigm. Genetics modulates motivation reward, and dopamine provides the basis for the interaction between motivational and cognitive control. There is some evidence that male adolescents take more risky decisions than female adolescents and that the lateralization of reward-related DA release in the ventral striatum is confined to men. These studies have implications for our understanding of natural reward and psychiatric conditions like addiction, depression and ADHD. Furthermore, the association between reward and memory can help develop treatment techniques for drug addiction that interfere with consolidation of memory. Finally, there is a lack of research on reward motivation, genetics and sex differences and this can improve our understanding of the relationships between reward, motivation and the brain.
There has been a significant growth in research on the brain mechanisms mediating reward motivation in humans. The purpose of this narrative review is to summarize studies on the neural mechanisms associated with reward motivation and cognitive function in healthy human participants from 2000 until now. A PubMed search has used “Reward motivation and brain imaging” as search words for publications between 2000 and October 2022. There were 2,127 records which were screened and papers that were published in English language in peer-reviewed journals and measured brain imaging in human subjects were included. Based on these criteria, 2,058 records were excluded, and 64 records were included.
The brain’s meso-limbic dopamine reward circuitry is known to control reward-motivated behavior in humans. In this review, in the first section, we have described first the brain’s meso-limbic dopamine reward circuitry (1.1) and then its interactions with decision-making (1.2), anticipation of reward (1.3), the coding of reward in the human brain (1.4), motivated cognitive control (1.5), prefrontal cortex (PFC) integration of cognitive control (1.6), reward motivation of the motor system (1.7) and finally, how reward motivation affects incentives like food, money and social reward (1.8). In the following section we have discussed how reward motivation is associated with cognitive processing such as learning (2.1), memory and dopaminergic activity in the striatum (2.2), Memory formation (2.3) Memory encoding and consolidation (2.4) Memory recollection (2.5) Long-term memory of novelty (2.6) Episodic memory retrieval (2.7), motor imagery and imagery of future events (2.8), working memory (2.9) and attention (2.10). Finally, we discuss the most common method of assessing reward motivation, the monetary incentive delay task (DMRT) and the meta-analyses of this paradigm (3.1), implications for drug abuse mental and neurological disorders (3.2) we summarize motivation reward, learning, memory and attention (3.3), motivation reward, pharmacological effects and genetics of dopamine (3.4), reward motivation and sex differences (3.5), and our conclusions (3.6).
The brain’s meso-limbic dopamine reward circuit is known to control reward-motivated behavior in humans. Schott et al. (2008) have measured neural activity in the meso-limbic reward circuit in participants who performed on a delayed monetary incentive task in fMRI while measuring dopamine (DA) release by using [11C]raclopride in PET. They have shown that neural activity in areas responsible for dopamine neurotransmission (substantia nigra and ventral tegmental area SN/VTA), and DA release (ventral striatum and nucleus accumbens VS/NAcc) in fMRI correlated with DA release in the NAcc measured in PET (Schott et al., 2008). Furthermore, DA release correlated with activity in the amygdala and the hippocampus. This study combined neurochemical and magnetic imaging in order to study reward motivation emotion and cognitive function. Kasanova et al. (2017) have measured dopamine receptors D2 and D3 occupancy with [18F]fallypride in PET while participants performed on a probabilistic reinforcement learning task with daily life reward. They have reported an association between reward-related DA release in the putamen, caudate and the VS and task performance. Furthermore, DA release in the right caudate and VS have regulated pleasurable behavior. This study demonstrates the relationships between DA activity, motivation and rewarding behavior. Taken altogether, the combination of measuring dopamine release in the striatum while monitoring brain activity during natural reward improves our understanding of the neurochemical and neuronal mechanisms underlying reward motivation, emotion and cognition in the human brain.
The process of decision-making involves various stages such as a selection phase and an anticipation phase. The wheel of fortune task with monetary gains was used to assess decision-making in fMRI (Ernst et al., 2004). The selection phase has employed visual-spatial attention by activating the occipital-parietal pathway, conflict resolution by activating the ACC, quantity manipulations by activating the parietal cortex and preparation for action by the pre-motor area. The anticipation phase involved reward processing in the VS. The VS was activated during high-reward and risk conditions during selection but not during anticipation. Selection of high-risk and reward and anticipation has activated the OFC. These findings imply that different neural networks are involved during the anticipation and selection phases while performing on a monetary reward task, and that selection and anticipation of risky decision affect VS and the OFC differently.
Anticipation of reward is an important skill in a constantly changing environment and decision-making requires an evaluation of anticipated outcomes and future actions. Symmonds et al. (2010) have used a sequential choice task in order to investigate models of decision valuation and choice of strategy in fMRI. They have reported that participants have evaluated decisions and updated strategies during assessment of actions. Sequential choice was associated with monitoring of future reward. Although the anticipation of reward involves both dorsal and ventral striatal circuits, only the dorsal striatum and its connected cortical network is involved in the direct modulation of motor behavior by incentive motivation. Harsay et al. (2011) have used a reward-cued anti-saccade paradigm to investigate how motivational goals modulate patterns of neural activation and functional connectivity to improve preparation for performance. They have found that reward anticipation was associated with increased activation in the ventral and dorsal striatum, and cortical oculomotor regions.
Potential rewards have an incentive motivation for improving performance. Vassena et al. (2014) have used a mental arithmetic task with manipulations of in fMRI. They have found that a difficult task and high reward have activated the striatum and ACC confirming their role in motivated reward. Reward anticipation is important for directing behavior toward stimuli with positive valence in order to form motivational salience. Mori et al. (2019) have used a monetary incentive delay task gain in order to investigate reward anticipation in fMRI. They have found that functional connectivity at rest included the areas of the VS, the PFC, and occipital and temporal cortices. There are relatively few studies that have examined motivation in situations when a decision is made in the absence of choice. Clithero et al. (2011) have used a task with anticipation of either money or and candies in fMRI. Activation of the NAcc and anterior insula was associated with individual variation in reward choice, and it shows that the NAcc modulates free choice.
There is evidence for a modular view of reward value coding in the brain which is indicated by a posterior-anterior organization in the OFC. Sescousse et al. (2010) have used an incentive delay task that has compared brain activation patterns to monetary and erotic rewards. They have shown that the VS, anterior insula, ACC and midbrain, encode the subjective value of rewards. The anterior lateral OFC processes monetary gains, whereas the posterior lateral OFC processes more basic erotic stimuli. Sescousse et al. (2015) have used cues predicting monetary or erotic rewards, with no decision required in fMRI. The VS was activated by both cues and its activity correlated with the motivational value of these rewards. Striatal reward value was activated when choice was not required but it can modulate motivated behavior.
There is little research on the difference between expected information and reward particularly when the payoff and goals are unknown. Filimon et al. (2020) have used a visual probabilistic categorization task in fMRI and they have discovered that expectation of information has activated the left lateral VS. The NAcc, medial PFC and the OFC during information expectation were differently activated than during reward-related processing.
There is evidence for dissociable neural responses to reward and penalties that are dependent on the psychological context in which they are experienced. Elliott et al. (2000) have used a gambling task with financial rewards and penalties in fMRI. They have shown an activation of the midbrain and VS to financial rewards and hippocampal activation to financial penalties. The globus pallidus, thalamus, and subgenual cingulate were activated in response to high reward. Increased penalties have activated the caudate, insula, and ventral prefrontal cortex.
Decision-making involves a cost-benefit analysis and brain imaging studies have shown that the process activates the VS and dorsal ACC. Schouppe et al. (2014) have used a decision-making task that involved a choice between an easy and a difficult flanker task. The cost-benefit analysis has been shown by activation of the striatum by a cognitively demanding option and not by forced-choice trials. Finally, models of reward implicate cortical-striatal loops and the DA system, with special emphasis on D2 receptors in the NAcc. Johansson et al. (2014) scanned participants in PET a [11C] raclopride dynamic scan during rewarded and non-rewarded task switching. Rewarded task switching (relative to baseline task switching) has decreased [11C] raclopride binding in the NAcc. Decreased NAcc [11C] raclopride binding correlated with performance on the task (reaction times). These PET findings provide evidence for striatal DA release during motivated cognitive control, and that DA release in the NAcc predicts the task’s reaction time benefits of reward.
The medial and lateral Pre-Frontal Cortex (PFC) play an important role in motivation reward by integrating motivation and cognitive control during decision-making.
Kouneiher et al. (2009) have reported that the medial and lateral PFC are organized hierarchically from posterior to anterior areas for motivation and behavior selection. Furthermore, functional connectivity analyses have shown that interactions in these regions transmit motivational incentives and regulates top-down control mechanisms. The PFC therefore integrates motivation and cognitive control for the purpose of decision making between short-term or long-term goals (Kouneiher et al., 2009). The PFC can exercise voluntary control by inhibiting activity of the NAcc during situations that require impulse control. Diekhof and Gruber (2010) have assessed the process of deciding between short term and long-term goals in human participants in fMRI. They have found that behavior that favors long-term goals over immediate reward was associated with an interaction between the antero-ventral PFC with the Nacc and VTA. The degree of this interaction predicted behavioral success during pursuit of the long-term goal, and it was associated with trait impulsivity. These findings demonstrate how voluntary action controls immediate desires via the inhibition of the PFC (Diekhof and Gruber, 2010). This evidence has important implications for the study of adolescents who are hypersensitive to reward due to an imbalance between reward and cognitive control.
Recent research has suggested that the brain’s reward mechanisms function differently in adolescence. It has been argued that sensitivity to reward is high in adolescence due to an imbalance in development of the striatum and prefrontal cortex, and that this motivation tendency may lead to subsequent substance abuse. It is not clear whether adolescents are involved in risk-taking behavior since they overestimate potential or actual reward. The anticipation, receipt, and omission of reward were investigated in adolescents and young adults in fMRI (Van Leijenhorst et al., 2010). They have reported that in anticipation of uncertain outcomes, the anterior insula was more active in adolescents compared with young adults and that the VS was activated in middle adolescence, whereas young adults showed OFC activation to omitted reward. These findings suggest that adolescents are hypersensitive to reward and that could result in risk-taking behavior.
The monetary incentive delay (MID) task was employed to assess the association between personality traits and reward mechanisms in young adolescents and young adults in fMRI (Joseph et al., 2016). Unlike the predictions, adolescents did not show higher sensitivity to gains than losses compared with adults during either anticipatory or feedback phases. They did show lower sensitivity to incentive magnitude in the meso-limbic circuit during anticipation and feedback stages. This response was mediated by personality, so impulsive or low avoidance adolescents showed greater gain sensitivity and high avoidance adolescents showed high loss sensitivity during cue anticipation. In adults, impulsivity has modulated meso-limbic response and impulsive adults showed reduced magnitude sensitivity during both anticipation and feedback. These findings imply that impulsivity modulates activity of the mesolimbic reward circuit during both adolescence and adulthood but in adolescence, avoidance and approach also affect this activity.
The OFC has an important function during reward anticipation and consummation. Yan et al. (2016) have found that the OFC encoded magnitude and valence (win vs. loss) but not outcome (favorable vs. unfavorable) during reward consummation and that the lateral OFC but not the medial OFC encoded information about loss. The dorsolateral PFC (dlPFC) integrates and transmits signals of reward to the mesolimbic and meso-cortical DA circuits and initiates motivated behavior. Ballard et al. (2011) have studied participants who have anticipated and prepared for opportunities to get reward in fMRI. They have reported that the availability of reward activated the dlPFC which monitored activation of the VTA. The dlPFC therefore integrates and transmits signals to the mesolimbic and meso-cortical DA systems, thus initiating motivated behavior (Ballard et al., 2011).
Although medial frontal brain regions are implicated in valuation of rewards, there are few studies on reward motivation using focal lesions to these areas. Manohar and Husain (2016) have studied patients with isolated, focal damage in ventral-medial PFC. Medial frontal damage was associated with reduced reward effects on saccadic velocity and autonomic (pupil), although few patients showed abnormally strong reward motivation effects. Increased sensitivity to rewards within the lesion group correlated with damage in subgenual ventral-medial PFC areas. Although medial frontal lesions may generally reduce reward sensitivity, damage to key areas paradoxically protects from this effect. Finally, Etzel et al. (2016) have used task switching with random reward incentive and no-incentive trials in fMRI and they have found that information on the task could be elucidated from activation of frontal-parietal regions associated with task control.
There is further evidence that reward motivation activates the motor system which integrates information about reward value to motivate performance. The executive control mechanisms involved in maintaining a balance between executing and withholding an action were investigated by Lee et al. (2017). They have manipulated prospective rewards in a stop-signal task in fMRI where both the proactive and reactive control were equally emphasized and showed that inhibition activated the anterior caudate (motivation status) and pre-SMA (action). The pre-SMA was activated in response to the need for action control, whereas the right inferior frontal cortex was activated during action inhibition. Galaro et al. (2019) have used a motor incentive motivation task for prospective monetary gains and losses together with motor cortical excitability measurement with transcranial magnetic stimulation (TMS). They have found that prospective gains and losses improved individuals’ performance. Loss aversion predicted behavioral sensitivity to incentive and motor cortical sensitivity to prospective gains. These findings suggest that motor cortical activity integrates information about the subjective value of reward to enhance incentive-motivated performance. Finally, Cho et al. (2013) used the MID task to study adults and youth in fMRI. They have found that the thalamus and insula have modulated activity of the NAcc during incentive processing. They have suggested that in both adults and adolescents, anticipation of gain or loss involves an “alerting” signal from the thalamus that together with interoceptive signals from the insula shapes selection programs in the VS.
Reward motivation also affects executive control mechanisms when an individual estimates future actions based on motivational states. Setton et al. (2019) have compared the effects of hunger and satiety states on participant’s bids on snack foods in fMRI. They have shown that activation of reward and control areas is affected by motivational states like hunger and satiety and that congruence of present and future motivational states affects any predictions about the future.
The motivation to obtain and consume primary rewards such as food is regulated by devaluation procedures but not by secondary rewards such as money. Yang et al. (2021) have investigated devaluation for primary reinforcers like chocolate milk and secondary reinforcers like money in fMRI. Functional near-infrared spectroscopy (fNIRS) measures obtained during the incentive delay paradigm showed that increasing value of secondary reward was linked with increasing anticipatory reward that has activated the lateral OFC, whereas during the consummation phase, the secondary reward activated the medial OFC irrespective of devaluation stage. These findings imply that secondary reinforcers like money can increase incentive motivation with repeated reward.
Dubey et al. (2020) have used behavioral measures of social motivation in order to assess the neural correlates of social and object rewards. They have used the “Choose-a-Movie-CAM” which quantifies the motivation for seeking social rewards including stimulus value and the effort required to obtain it (cost-benefit) in fMRI. They have reported that the precuneus and medial OFC were involved in social choice whereas the ventral and dorsal striatum were involved in object choice. These activations can be seen during the decision phase even before the rewards have been consumed, indicating a transfer of the rewarding value of social stimuli to its cues. The left insula and bilateral inferior occipital gyrus and the inferior parietal lobe were recruited for increasing effort investment. Finally, Brandl et al. (2019) have performed meta-analysis of studies using cognitive reward control of one’s craving for hedonic stimuli, like food, sex, or drugs in fMRI. They have discovered cognitive reward control activation mainly in the supplementary motor area (SMA), dlPFC, and ventrolateral PFC across studies. The cognitive reward control was activated across stimulus types, and it was similar to areas mediating cognitive emotion regulation. Table 1 describes reward motivation studies in fMRI.
Individuals are motivated to act upon either internal urges or interests or external triggers like money and this process of orientation can affect positive or negative feedback and learning. Linke et al. (2010) have used a probabilistic reversal learning task in order to investigate how motivation regulates learning. Extrinsic motivation correlated with activation of the ACC, amygdala and putamen, whereas intrinsic motivation negatively correlated with activation in these brain regions. These findings indicate that motivational orientation regulates response to reward in the ACC, amygdala, NAcc, and the putamen.
Learning motivation activates different brain regions than motivation to gain monetary reward. Mizuno et al. (2008) have reported that learning motivation correlates with activation of the putamen, whereas monetary reward has also activated the putamen bilaterally, though there was no association between activation and monetary reward.
Murayama et al. (2010) have used a stopwatch task in fMRI and showed that performance-based monetary reward has undermined intrinsic motivation indicated by reduced task engagement and decreased activity in the anterior striatum and PFC areas. It seems that the cortical-basal ganglia reward valuation system integrates extrinsic and intrinsic task reward value. Miura et al. (2017) have used variations of the stopwatch task that manipulated controllability and outcome in fMRI. The stopwatch task with the action-outcome contingency activated greater enjoyment and the VS and midbrain, indicating intrinsic motivation.
Previous studies suggest that external rewards like money and verbal praise can enhance motivation and self-determination. Albrecht et al. (2014) have reported that positive performance feedback activated the anterior striatum and midbrain while monetary rewards were administered. Verbal rewards have activated these regions when participants received success compared to failure feedback. Finally, after the verbal rewards were administered and withdrawn, activity in the lateral PFC has increased.
It is unclear how intrinsic rewards that are associated with a task motivate cognitive control. Huskey et al. (2018) have used a video game and showed that high levels of intrinsic reward, with a balanced task difficulty and individual ability, were associated with increased activation of cognitive control areas (dlPFC), orienting attention (precentral gyrus SPL), attention alert (insula), and reward (putamen). However, a discrepancy between task difficulty and individual ability reduced intrinsic reward and has activated the default mode network, including the insula and the salience network.
Reward motivation and information processing by cues was investigated by Shashidhara and Erez (2021). They have used a cued target detection task together with monetary reward and they have found that reward activated the frontal-parietal network when the cue and subsequent object were presented. However, unexpectedly, reward did not increase discrimination between conditions when context information was provided.
The striatal dopamine reward circuit has dense dopaminergic innervation which is responsible for motor control and higher-order cognitive brain functions. The limbic striatum, that is responsible for reward processing, has been associated with episodic memory. Cervenka et al. (2008) have investigated the association between cognitive performance and striatal dopamine D2- receptor binding with [11C] raclopride in PET. Receptor availability in the limbic striatum was related to performance in tests of episodic memory, but not to tests of verbal fluency and general knowledge. By contrast, D2 binding in associative and sensory-motor striatum was associated with non-episodic tasks. These findings provide evidence for a functional compartmentalization of human striatum and serve as a starting point for a more detailed investigation of striatal biomarkers in the normal brain as well as in neurodegenerative disorders.
Activity in the VS works together with the frontal-parietal attention network to enhance memory formation. Duan et al. (2020) have shown that both external monetary reward and internal curiosity have enhanced memory performance, without evidence for an interaction between the two. Curiosity activated the VS reward circuit and the frontal-parietal attention circuit to enhance memory formation. The external monetary reward effect on memory was associated with reduced activity in parietal midline regions. Curiosity therefore enhances memory performance by allocation of attention and reward resources whereas monetary reward does so by suppression of task-irrelevant processing.
Memory encoding and consolidation is affected by activation of the hippocampus and the reward circuit. Adcock et al. (2006) have used a monetary incentive encoding task with reward cues for memorizing an upcoming scene in fMRI. Rewarding cues presented before remembered scenes have activated the Nacc, VTA and the hippocampus, and they have been associated with improved declarative memory. Supporting evidence for the role of reward motivation in activation of memory in the hippocampus is provided by Murty and Adcock (2014). They have used a task presenting participants with goal-irrelevant expectancy violations during high or low-reward motivation. Reward motivation has activated the hippocampus in response to declarative memory for expectancy violations and it enhanced anticipatory VTA-cortical-hippocampal interactions. Murty et al. (2016) have exposed participants to unexpected events during reward or punishment in fMRI. The hippocampus was activated by perceptual surprises during reward motivation, whereas surprises have activated the parahippocampal cortex during punishment. These findings indicate that separate parts of the MTL regulate anticipation of reward and avoidance of punishment, affecting learning and handling of surprises in order to make predictions about the environment. Miendlarzewska et al. (2016) have reviewed the evidence of how motivation can prioritize information for memory encoding and consolidation. The evidence suggests that dopamine enhances the formation of declarative memory for reward information and may also control the generalization of reward values. In particular, activity in the hippocampus and in the reward circuit affects decision-making and integration.
Reward motivation also plays also a role in modulating memory recollection (Elward et al., 2015). Participants have discriminated between studied and unstudied pictures of objects, and they have indicated the identity of the coin paired with the object at study. Correct judgments earned a reward corresponding to the value of the coin, whereas incorrect judgments were penalized. Accurate responses have activated the hippocampus and different striatal sub-regions demonstrated recollection effects, reward effects, and overlap between the two effects. The left angular gyrus and medial prefrontal cortex were additively responsive to source accuracy and reward.
Novelty and reward were investigated by using a scene encoding paradigm in fMRI (Bunzeck et al., 2012). The striatum and the SN/VTA have modulated long-term memory activity in the MTL and contextual exploration in the hippocampus. This evidence suggests that long-term memory of novelty stored in the MTL is associated with reward-prediction in the medial OFC, which in turn affects reward responses in the striatum.
Reward motivation and emotion have also distinct effects on episodic memory. Shigemune et al. (2010) have reported a study in which subjects were engaged in encoding of photographs during an imaging scan using H215O in PET. During encoding, emotional exposure to negative pictures has activated the left amygdala whereas the left OFC was activated during encoding of high reward pictures relative to low reward pictures, and the hippocampus was activated in both conditions. It has been suggested that the hippocampus integrates the effects of emotion in the amygdala and monetary reward in the OFC on encoding of episodic memory (Shigemune et al., 2010). Shigemune et al. (2017) have shown that reward motivation enhanced episodic memory of Japanese words and it has activated the SN/VTA, MTL, dorsomedial prefrontal cortex (dmPFC), and dlPFC during retrieval of memories with high difficulty. Finally, Frank et al. (2019) have found that connectivity between memory and reward regions reflected individual differences in reward modulation of memory, in the AC, OFC, and VS. This evidence suggests that there is a broader set of reward regions regulating memory than considered previously and these relate to individual differences in how reward impacts memory.
Reward-related cues presented during motor imagery have activated the VS, the amygdala and the motor cortex, increasing the desire and urge to obtain goals (Mendelsohn et al., 2014). Reward motivation also affects imagery of personal future events. Bulganin and Wittmann (2015) have used a reward learning task in which words have either received a monetary reward or not. They then imagined personal future events based on these and novel words. Reward and imagery of future events have activated the striatum, VTA and the hippocampus. Functional connectivity between these areas was enhanced during imagery of reward and novel words. This study indicates that past motivated reward contributes to our expectation of the future.
Motivation can also enhance attention, goal achievement and working memory.
Jimura et al. (2010) showed that individuals who are reward-sensitive improved working memory on trials that were not rewarded which has activated the right lateral prefrontal cortex. Motivation can also be integrated in executive function areas such as the dorsal ACC to compute the expected value of goal-directed cognitive control. Yee et al. (2021) have used a task that quantifies the combined effects of liquid and monetary incentives on cognitive task performance. They have found that monetary incentives adjusted dorsal ACC activation and this adjustment predicted changes in cognitive performance and self-report motivation ratings. The dorsal ACC therefore encoded the incentives in terms of their motivational value, which correlated with task performance.
Reward and motivation have beneficial effects on accuracy and variability during sustained attention. Locke and Braver (2008) have used the Continuous Performance Test (CPT) under different motivational conditions. They have found that reward was associated with an activation of the parietal and prefrontal cortex. Activation of reward and PFC was associated with motivation-related performance enhancement and personality variables. Esterman et al. (2017) have used the gradual-onset continuous performance task with alternating rewarded and unrewarded blocks. Rewarded blocks have activated brain regions responsible for preparation for upcoming targets, which in turn has improved accuracy. These findings suggest that motivated individuals use task-positive resources proactively during sustained attention. Table 2 describes studies on learning memory and attention in fMRI.
The brain’s meso-limbic dopamine reward circuitry is known to control reward-motivated behavior in humans. There is evidence that reward motivation is associated with activation of the human reward system [NAcc and the putamen, anterior cingulate cortex (ACC) and the amygdala], it is regulated by areas in the PFC (medial and lateral Pre Frontal Cortex (PFC), the dlPFC, it is modulated by the thalamus, and insula and may activate action plans in the motor system (SMA).
One of the most common methods of assessing reward motivation is the monetary incentive delay task (DMRT) and there were nine meta-analyses of this paradigm.
The monetary incentive delay (MID) task in fMRI by Knutson and Greer (2008) requires participants to perform on a reaction time task in which they press a key in response to a white square. The white square is preceded by a circle which is a cue that a successful performance will result with winning money or by a triangle which is a cue that indicates that successful performance will not be rewarded. Feedback about whether money has been won is given visually immediately after the response. Participants are trained until they succeed in two-thirds of the trials. Afterward, they perform the same task in the fMRI scanner. Event-related fMRI is used to measure brain activity during reward anticipation and receiving feedback.
In an initial meta-analysis of studies using the Monetary Incentive Delay (MID) task, Knutson and Greer (2008) have found that reward anticipation has activated the medial frontal gyrus, the NAcc, the anterior insula, the putamen and the thalamus. More recently, Oldham et al. (2018) in a meta-analysis of studies using the MID task has found that reward anticipation has activated the VS and dorsal striatum, the thalamus and amygdala, the midbrain, the insula, the premotor cortex and supplementary motor area, the occipital cortex and the cuneus. Unlike Knutson and Greer’s (2008) meta-analysis, the medial frontal cortex was not activated. Reward delivery activated the VS and the amygdala, medial frontal, OFC and the posterior cingulate cortex. Wilson et al. (2018) had used data from group maps provided by authors and they have found more extensive activation of cortical regions compared with Oldham et al. (2018). Other meta-analyses have used a broad including a wide range of paradigms of anticipation and/or delivery of monetary reward as well as natural reward (Liu et al., 2011; Diekhof et al., 2012; Bartra et al., 2013). Liu et al. (2011) has conducted a meta-analysis on 142 neuroimaging studies and they have found that reward-related decision-making tasks have activated the NAcc, caudate, putamen, thalamus, OFC, anterior insula, ACC and posterior cingulate cortex (PCC), the inferior parietal lobe and the PFC. The NAcc was activated by both positive and negative rewards during anticipation, outcome, and evaluation of reward. The medial OFC and PCC were activated in response to positive rewards, whereas the ACC, anterior insula, and lateral PFC were activated in response to negative rewards. A meta-analysis on the role of reward prediction and its consumption by Diekhof et al. (2012) has found that the VS plays a role in reward anticipation and consumption and may also be sensitive to different reward magnitudes, while the medial OFC and VMPFC process the magnitude during reward receipt. Bartra et al. (2013) have performed a meta-analysis of 206 studies investigating subjective value (SV) of choice alternatives. They have reported neural networks a subjective valuation system of decision making including the dorsal and posterior striatum, dorsomedial PFC, anterior insula and the thalamus. Dugré et al. (2018) have conducted a meta-analysis of 35 studies of brain activations during anticipation and monetary loss. In both anticipation and loss, participants showed activations of the striatum, (anterior) insula, and anterior cingulate gyrus relative to loss. Loss anticipation activated the ventral-lateral PFC whereas loss receipt has activated the medial PFC. Finally, a meta-analysis of 45 studies of reward anticipation has shown activations in the VS, the middle cingulate cortex, supplementary motor area and the insula (Jauhar et al., 2021). This meta-analysis has indicated that monetary reward anticipation and delivery activate the VS but not the dorsal striatum and are associated with different patterns of cortical activation. The studies shown so far indicate that there are variations in activation depending on the anticipation of win or loss and the method of image-analysis in the assessment of reward (see Jauhar et al., 2021 for review).
Alterations in reward motivation are important for the development of psychiatric disorders particularly during adolescence (Fairchild, 2011). Psychiatric conditions involve alterations in motivational processes or disturbances in reward processing that entail hypersensitivity or hyposensitivity to reward at different processing stages such as reward anticipation or receipt. Externalizing disorders and substance use disorders, are related to reduced activity in the motivation reward circuit during anticipation of reward, whereas enhanced responses to rewarding outcomes and reduced sensitivity to punishment is associated with externalizing disorders such as conduct disorder or oppositional defiant disorder. Adolescents with conduct disorder and comorbid substance dependence who performed on the Iowa Gambling Task showed impaired reward-related decision making (Schutter et al., 2010). In contrast, depression is associated with impaired striatal responses to rewarding outcomes. Depressed adolescents who performed on a behavioral decision-making task involving varying probability and magnitude of reward failed to distinguish between options involving small or large possible reward during conditions involving a high probability of winning. These faulty reward choices at the age of 11 predicted depressive symptoms a year later (Forbes et al., 2007). These results show an attenuation of reward seeking in depression or at those with high risk for developing depression. A meta-analytic review of fMRI studies using the MID task to asses reward anticipation in patients with ADHD has shown VS hypo-responsiveness in ADHD during reward anticipation and the opposite in healthy volunteers (impulsivity-scores positively correlated with VS activation during reward processing) (Plichta and Scheres, 2014). These findings indicate impaired reward anticipation mechanisms in ADHD. The following section will describe the clinical implications of these studies for drug abuse, mental and neurological disorders.
The research so far has implications for understanding drug abuse and other mental and neurological disorders. It has been suggested that when there is a dysfunction in the brain reward system, which could be caused by certain genetic variants of DA, it could result in high risk for multiple addictive, impulsive and compulsive behavioral disorders such as alcohol and drug use disorders, pathological gambling, compulsive sexual behavior, ADHD, Tourette’s Syndrome, conduct disorder and antisocial behavior (Blum et al., 2000). Psychostimulant drugs like cocaine and amphetamines activate the meso-cortico-limbic system by increasing dopamine release within the NAcc resulting in long-term changes and dysregulation of the meso-limbic dopamine reward circuit (Koob and Volkow, 2010). Recent studies have also shown that natural rewards like computer games are associated with DA release to a similar magnitude as psychostimulants (Koepp et al., 1998; Weinstein, 2010). Brain imaging studies have shown that Internet and Gaming Disorder (IGD) is like other addictions by showing activation in brain regions mediating reward, reduced impulse control and impaired decision making; and reduced functional connectivity in areas responsible for cognitive control, executive function, motivation, and reward (Weinstein et al., 2017; Weinstein and Lejoyeux, 2020). Exposure to gambling cues has activated areas of the DA reward system like drugs of abuse (Potenza et al., 2003; Crockford et al., 2005). Exposure to explicit sexual stimuli has activated these areas in individuals with compulsive sexual behavior disorder CSBD (Voon et al., 2014). It has been suggested that disruption of the dopamine reward system is associated with motivation deficits in ADHD adults, which may contribute to attention deficits and supports the use of therapeutic interventions to enhance motivation in ADHD (Volkow et al., 2011). Furthermore, reduced reward function is a diagnostic criterion for depression, and Anhedonia which is a diminished pleasure and or decreased reactivity to pleasurable stimuli is a core feature of depression that frequently persists after treatment (Admon and Pizzagalli, 2015).
There are reciprocal relationships between motivation reward, learning, memory and attention. Memory formation is enhanced by VS and frontal-parietal attention networks activation whereas memory encoding and consolidation is modulated by activity in the hippocampus and reward areas. The striatum is also associated with long-term memory of novelty coded in the MTL and reward-predicting properties in the OFC. Long-term memory and contextual exploration in the hippocampus are modulated by parts of the reward circuit (VS, SN and VTA). Reward motivation and emotion have also effects on episodic memory, attention and working memory relying on a broad network of connectivity consisting of the ACC, OFC, and VS.
Since reward is important to learning and memory processes such as encoding and consolidation of memory, there are important implications for drug and behavioral addictions. It is known that memories are not permanent and there has been an effort lately to use reconsolidation in order to eliminate or reduce the impact of bad memories in order to treat mental health disorders like trauma and drug addiction. Although reconsolidation helps to keep memories “alive,” amnesic medications during the reconsolidation period can habituate or weaken bad memories. Early proof-of-principle studies have established reconsolidation as a potential therapeutic strategy for relapse prevention in drug addiction by using pharmacological agents operating on DA receptors or glucocorticoid receptors or others, although there are no definite results of these studies yet (Sorg, 2012; Exton-McGuinness and Milton, 2018). Rafei et al. (2021) have also proposed a novel framework that combines different cognitive interventions including cue-exposure, memory reconsolidation, and episodic future thinking, in order to reshape maladaptive drug-related memories toward more adaptive memories to support addiction recovery.
Genetics may also play an important role in modulating motivation reward, and DA has been hypothesized to provide the basis for the interaction between motivational and cognitive control. Studies have used a rewarding guessing task (Yacubian et al., 2007; Forbes et al., 2009) and the MID task (Dreher et al., 2009) in fMRI have investigated the association between the DA transporter DAT1 gene (SLC6A3) and reward anticipation. Increased VS activity was linked with a DAT1 9-repeat Variable Number Tandem Repeat (VNTR), related to lower synaptic discharge of DA. Striatal activity was also linked with a DRD4 VNTR (postsynaptic inhibition) and a DRD2 -141C deletion (inhibitory DRD2 receptors). Aarts et al. (2010) have used a pre-cued switching task in fMRI and have also found that carriers of the 9-repeat 9R allele which is associated with high striatal DA turnover, showed greater activity in the dorsal-medial striatum during reward anticipation than homozygotes for the 10-repeat allele, replicating the previous genetic imaging studies. In addition, variations of the catechol-O-methyltransferase (COMT) Val/Met-polymorphism were associated with PFC activation during anticipation of reward. Met/Met carriers of COMT showed increased tonic DA-levels during performance of the task (Yacubian et al., 2007; Dreher et al., 2009). The studies reviewed so far have investigated the link between brain regions and single selected genes. However, reward motivation is a complex neurobiological mechanism that requires an approach studying multiple genes performed by a Genome Wide Association Study (GWAS) (Loth et al., 2011). Furthermore, dopaminergic medication may have different effects on the different stages of drug use such as anticipation and consummation (Webber et al., 2021). The relationship between DA and reward function appears unlikely to be linear and the ability to investigate the effects of DA medications depends on the behavioral and brain-imaging measures that have been utilized. Finally, DA activity is modulated by DA dopamine synthesis and release, re-uptake, receptor activity and metabolism, as well as by other neurotransmitters like opioids, serotonin 5-HT, acetylcholine, gamma-aminobutyric acid (GABA), brain-derived neurotrophic factor (BDNF) and endocannabinoids (Koob and Volkow, 2010).
Very few studies have investigated sex differences in reward motivation using brain imaging techniques. A study has assessed striatal DA response to unpredictable monetary rewards on a “slot-machine” task by using [11C] raclopride in PET (Martin-Soelch et al., 2011). They have unexpectedly found a bilateral reduction in binding potential in women during the reward condition while in men the reduction was only in the right ventral striatum. This evidence suggests that DA release in response to unpredictable reward is lateralized in the human ventral striatum, particularly in males. Another study by Alarcón et al. (2017) has examined sex differences during a risky decision-making task in fMRI. Male adolescents made more risky decisions during the task’s performance, and they showed increased NAcc activation in fMRI relative to female adolescents, that was not mediated by sex hormones. The results support previous evidence that increased motivation and salience of reinforcers is associated with robust striatal response and hence reward sensitivity is affected by sex and psychosocial factors.
The studies reviewed so far describe brain mechanisms of reward motivation in humans including anticipation and consummation of reward. Regions in the dopamine reward circuit including the striatum together with areas in the PFC, insula and thalamus serve to regulate reward motivation. The reward motivation circuit also interacts or facilitates a variety of cognitive function abilities like attention, memory including working memory and decision-making. These studies improve our understanding of human processes of reward in healthy populations, but they also provide insights in deficient reward mechanisms and their manifestation in clinical conditions like drug addiction, behavioral addictions and neurological disorders like ADHD. There is little research on the genetic mechanisms underlying reward motivation and sex differences in reward motivation. Future studies would be able to fill the gaps in our current knowledge of this important topic.
The author confirms being the sole contributor of this work and has approved it for publication.
The author declares that the research was conducted in the absence of any commercial or financial relationships that could be construed as a potential conflict of interest.
All claims expressed in this article are solely those of the authors and do not necessarily represent those of their affiliated organizations, or those of the publisher, the editors and the reviewers. Any product that may be evaluated in this article, or claim that may be made by its manufacturer, is not guaranteed or endorsed by the publisher.
Aarts, E., Roelofs, A., Franke, B., Rijpkema, M., Fernández, G., Helmich, R. C., et al. (2010). Striatal dopamine mediates the interface between motivational and cognitive control in humans: Evidence from genetic imaging. Neuropsychopharmacology 35, 1943–1951. doi: 10.1038/npp.2010.68
Adcock, R. A., Thangavel, A., Whitfield-Gabrieli, S., Knutson, B., and Gabrieli, J. D. (2006). Reward-motivated learning: Mesolimbic activation precedes memory formation. Neuron 50, 507–517. doi: 10.1016/j.neuron.2006.03.036
Admon, R., and Pizzagalli, D. A. (2015). Dysfunctional reward processing in depression. Curr. Opin. Psychol. 4, 114–118. doi: 10.1016/j.copsyc.2014.12.011
Alarcón, G., Cservenka, A., and Nagel, B. J. (2017). Adolescent neural response to reward is related to participant sex and task motivation. Brain Cogn. 111, 51–62. doi: 10.1016/j.bandc.2016.10.003
Albrecht, K., Abeler, J., Weber, B., and Falk, A. (2014). The brain correlates of the effects of monetary and verbal rewards on intrinsic motivation. Front. Neurosci. 8:303. doi: 10.3389/fnins.2014.00303
Ballard, I., Murty, V. P., Carter, R. M., MacInnes, J. J., Huettel, S. A., and Adcock, R. A. (2011). Dorsolateral prefrontal cortex drives mesolimbic dopaminergic regions to initiate motivated behavior. J. Neurosci. 31, 10340–10346. doi: 10.1523/JNEUROSCI.0895-11.2011
Bartra, O., McGuire, J. T., and Kable, J. W. (2013). The valuation system: A coordinate-based meta-analysis of BOLD fMRI experiments examining neural correlates of subjective value. Neuroimage 76, 412–427. doi: 10.1016/j.neuroimage.2013.02.063
Blum, K., Braverman, E. R., Holder, J. M., Lubar, J. F., Monastra, V. J., Miller, D., et al. (2000). Reward deficiency syndrome: A biogenetic model for the diagnosis and treatment of impulsive, addictive, and compulsive behaviors. J. Psychoactive Drugs 32, 1–112. doi: 10.1080/02791072.2000.10736099
Brandl, F., Le Houcq Corbi, Z., Mulej Bratec, S., and Sorg, C. (2019). Cognitive reward control recruits medial and lateral frontal cortices, which are also involved in cognitive emotion regulation: A coordinate-based meta-analysis of fMRI studies. Neuroimage 200, 659–673. doi: 10.1016/j.neuroimage.2019.07.008
Bulganin, L., and Wittmann, B. C. (2015). Reward and novelty enhance imagination of future events in a motivational-episodic network. PLoS One 10:e0143477. doi: 10.1371/journal.pone.0143477
Bunzeck, N., Doeller, C. F., Dolan, R. J., and Duzel, E. (2012). Contextual interaction between novelty and reward processing within the mesolimbic system. Hum. Brain Mapp. 33, 1309–1324. doi: 10.1002/hbm.21288
Cervenka, S., Bäckman, L., Cselényi, Z., Halldin, C., and Farde, L. (2008). Associations between dopamine D2-receptor binding and cognitive performance indicate functional compartmentalization of the human striatum. Neuroimage 40, 1287–1295. doi: 10.1016/j.neuroimage.2007.12.063
Cho, Y. T., Fromm, S., Guyer, A. E., Detloff, A., Pine, D. S., Fudge, J. L., et al. (2013). Nucleus accumbens, thalamus and insula connectivity during incentive anticipation in typical adults and adolescents. Neuroimage 66, 508–521. doi: 10.1016/j.neuroimage.2012.10.013
Clithero, J. A., Reeck, C., Carter, R. M., Smith, D. V., and Huettel, S. A. (2011). Nucleus accumbens mediates relative motivation for rewards in the absence of choice. Front. Hum. Neurosci. 5:87. doi: 10.3389/fnhum.2011.00087
Crockford, D. N., Goodyear, B., Edwards, J., Quickfall, J., and El-Guebaly, N. (2005). Cue-induced brain activity in pathological gamblers. Biol. Psychiatry 58, 787–795. doi: 10.1016/j.biopsych.2005.04.037
Diekhof, E. K., and Gruber, O. (2010). When desire collides with reason: Functional interactions between anteroventral prefrontal cortex and nucleus accumbens underlie the human ability to resist impulsive desires. J. Neurosci. 30, 1488–1493. doi: 10.1523/JNEUROSCI.4690-09.2010
Diekhof, E. K., Kaps, L., Falkai, P., and Gruber, O. (2012). The role of the human ventral striatum and the medial orbitofrontal cortex in the representation of reward magnitude—an activation likelihood estimation meta analysis of neuroimaging studies of passive reward expectancy and outcome processing. Neuropsychologia 50, 1252–1266. doi: 10.1016/j.neuropsychologia.2012.02.007
Dreher, J. C., Kohn, P., Kolachana, B., Weinberger, D. R., and Berman, K. F. (2009). Variation in dopamine genes influences responsivity of the human reward system. Proc. Natl. Acad. Sci. U.S.A. 106, 617–622. doi: 10.1073/pnas.0805517106
Duan, H., Fernández, G., van Dongen, E., and Kohn, N. (2020). The effect of intrinsic and extrinsic motivation on memory formation: Insight from behavioral and imaging study. Brain Struct. Funct. 225, 1561–1574. doi: 10.1007/s00429-020-02074-x
Dubey, I., Georgescu, A. L., Hommelsen, M., Vogeley, K., Ropar, D., and Hamilton, A. F. C. (2020). Distinct neural correlates of social and object reward seeking motivation. Eur. J. Neurosci. 52, 4214–4229. doi: 10.1111/ejn.14888
Dugré, J. R., Dumais, A., Bitar, N., and Potvin, S. (2018). Loss anticipation and outcome during the monetary incentive delay task: A neuroimaging systematic review and meta-analysis. PeerJ 6:e4749. doi: 10.7717/peerj.4749
Elliott, R., Friston, K. J., and Dolan, R. J. (2000). Dissociable neural responses in human reward systems. J. Neurosci. 20, 6159–6165. doi: 10.1523/JNEUROSCI.20-16-06159.2000
Elward, R. L., Vilberg, K. L., and Rugg, M. D. (2015). Motivated memories: Effects of reward and recollection in the core recollection network and beyond. Cereb. Cortex 25, 3159–3166. doi: 10.1093/cercor/bhu109
Ernst, M., Nelson, E. E., McClure, E. B., Monk, C. S., Munson, S., Eshel, N., et al. (2004). Choice selection and reward anticipation: An fMRI study. Neuropsychologia 42, 1585–1597. doi: 10.1016/j.neuropsychologia.2004.05.011
Esterman, M., Poole, V., Liu, G., and DeGutis, J. (2017). Modulating reward induces differential neurocognitive approaches to sustained attention. Cereb. Cortex 27, 4022–4032. doi: 10.1093/cercor/bhw214
Etzel, J. A., Cole, M. W., Zacks, J. M., Kay, K. N., and Braver, T. S. (2016). Reward motivation enhances task coding in frontoparietal cortex. Cereb Cortex. 26, 1647–1659. doi: 10.1093/cercor/bhu327
Exton-McGuinness, M. T. J., and Milton, A. L. (2018). Reconsolidation blockade for the treatment of addiction: Challenges, new targets, and opportunities. Learn. Mem. 25, 492–500. doi: 10.1101/lm.046771.117
Fairchild, G. (2011). The developmental psychopathology of motivation in adolescence. Dev. Cogn. Neurosci. 1, 414–429. doi: 10.1016/j.dcn.2011.07.009
Filimon, F., Nelson, J. D., Sejnowski, T. J., Sereno, M. I., and Cottrell, G. W. (2020). The ventral striatum dissociates information expectation, reward anticipation, and reward receipt. Proc. Natl. Acad. Sci. U.S.A. 117, 15200–15208. doi: 10.1073/pnas.1911778117
Forbes, E. E., Brown, S. M., Kimak, M., Ferrell, R. E., Manuck, S. B., and Hariri, A. R. (2009). Genetic variation in components of dopamine neurotransmission impacts ventral striatal reactivity associated with impulsivity. Mol. Psychiatry 14, 60–70. doi: 10.1038/sj.mp.4002086
Forbes, E. E., Shaw, D. S., and Dahl, R. E. (2007). Alterations in reward-related decision making in boys with recent and future depression. Biol. Psychiatry 61, 633–639. doi: 10.1016/j.biopsych.2006.05.026
Frank, L. E., Preston, A. R., and Zeithamova, D. (2019). Functional connectivity between memory and reward centers across task and rest track memory sensitivity to reward. Cogn. Affect. Behav. Neurosci. 19, 503–522. doi: 10.3758/s13415-019-00700-8
Galaro, J. K., Celnik, P., and Chib, V. S. (2019). Motor cortex excitability reflects the subjective value of reward and mediates its effects on incentive-motivated performance. J. Neurosci. 39, 1236–1248. doi: 10.1523/JNEUROSCI.1254-18.2018
Harsay, H. A., Cohen, M. X., Oosterhof, N. N., Forstmann, B. U., Mars, R. B., and Ridderinkhof, K. R. (2011). Functional connectivity of the striatum links motivation to action control in humans. J. Neurosci. 31, 10701–10711. doi: 10.1523/JNEUROSCI.5415-10.2011
Huskey, R., Craighead, B., Miller, M. B., and Weber, R. (2018). Does intrinsic reward motivate cognitive control? a naturalistic-fMRI study based on the synchronization theory of flow. Cogn. Affect. Behav. Neurosci. 18, 902–924. doi: 10.3758/s13415-018-0612-6
Jauhar, S., Fortea, L., Solanes, A., Albajes-Eizagirre, A., McKenna, P. J., and Radua, J. (2021). Brain activations associated with anticipation and delivery of monetary reward: A systematic review and meta-analysis of fMRI studies. PLoS One 16:e0255292. doi: 10.1371/journal.pone.0255292
Jimura, K., Locke, H. S., and Braver, T. S. (2010). Prefrontal cortex mediation of cognitive enhancement in rewarding motivational contexts. Proc. Natl. Acad. Sci. U.S.A. 107, 8871–8876. doi: 10.1073/pnas.1002007107
Johansson, L. S., Axelsson, J., Riklund, K., Braver, T. S., Ögren, M., Bäckman, L., et al. (2014). Dopamine release in nucleus accumbens during rewarded task switching measured by [11C]raclopride. Neuroimage 99, 357–364. doi: 10.1016/j.neuroimage.2014.05.047
Joseph, J. E., Zhu, X., Lynam, D., and Kelly, T. H. (2016). Modulation of meso-limbic reward processing by motivational tendencies in young adolescents and adults. Neuroimage 129, 40–54. doi: 10.1016/j.neuroimage.2015.12.005
Kasanova, Z., Ceccarini, J., Frank, M. J., Amelsvoort, T. V., Booij, J., Heinzel, A., et al. (2017). Striatal dopaminergic modulation of reinforcement learning predicts reward-oriented behavior in daily life. Biol. Psychol. 127, 1–9. doi: 10.1016/j.biopsycho.2017.04.014
Knutson, B., and Greer, S. M. (2008). Anticipatory affect: Neural correlates and consequences for choice. Philos. Trans. R. Soc. Lond. B Biol. Sci. 363, 3771–3786. doi: 10.1098/rstb.2008.0155
Koepp, M. J., Gunn, R. N., Lawrence, A. D., Cunningham, V. J., Dagher, A., Jones, T., et al. (1998). Evidence for striatal dopamine release during a video game. Nature 393, 266–268. doi: 10.1038/30498
Koob, G. F., and Volkow, N. D. (2010). Neurocircuitry of addiction. Neuropsychopharmacology 35, 217–238. doi: 10.1038/npp.2009.110
Kouneiher, F., Charron, S., and Koechlin, E. (2009). Motivation and cognitive control in the human prefrontal cortex. Nat. Neurosci. 12, 939–945. doi: 10.1038/nn.2321
Lee, H. J., Lin, F. H., and Kuo, W. J. (2017). The neural mechanism underpinning balance calibration between action inhibition and activation initiated by reward motivation. Sci. Rep. 7:9722. doi: 10.1038/s41598-017-10539-z
Linke, J., Kirsch, P., King, A. V., Gass, A., Hennerici, M. G., Bongers, A., et al. (2010). Motivational orientation modulates the neural response to reward. Neuroimage 49, 2618–2625. doi: 10.1016/j.neuroimage.2009.09.013
Liu, X., Hairston, J., Schrier, M., and Fan, J. (2011). Common and distinct networks underlying reward valence and processing stages: A meta analysis of functional neuroimaging studies. Neurosci. Biobehav. Rev. 35, 1219–1236. doi: 10.1016/j.neubiorev.2010.12.012
Locke, H. S., and Braver, T. S. (2008). Motivational influences on cognitive control: Behavior, brain activation, and individual differences. Cogn. Affect. Behav. Neurosci. 8, 99–112. doi: 10.3758/cabn.8.1.99
Loth, E., Carvalho, F., and Schumann, G. (2011). The contribution of imaging genetics to the development of predictive markers for addictions. Trends Cogn. Sci. 15, 436–446. doi: 10.1016/j.tics.2011.07.008
Manohar, S. G., and Husain, M. (2016). Human ventromedial prefrontal lesions alter incentivisation by reward. Cortex 76, 104–120. doi: 10.1016/j.cortex.2016.01.005
Martin-Soelch, C., Szczepanik, J., Nugent, A., Barhaghi, K., Rallis, D., Herscovitch, P., et al. (2011). Lateralization and gender differences in the dopaminergic response to unpredictable reward in the human ventral striatum. Eur. J. Neurosci. 33, 1706–1715. doi: 10.1111/j.1460-9568.2011.07642.x
Mendelsohn, A., Pine, A., and Schiller, D. (2014). Between thoughts and actions: Motivationally salient cues invigorate mental action in the human brain. Neuron 81, 207–217. doi: 10.1016/j.neuron.2013.10.019
Miendlarzewska, E. A., Bavelier, D., and Schwartz, S. (2016). Influence of reward motivation on human declarative memory. Neurosci. Biobehav. Rev. 61, 156–176. doi: 10.1016/j.neubiorev.2015.11.015
Miura, N., Tanabe, H. C., Sasaki, A. T., Harada, T., and Sadato, N. (2017). Neural evidence for the intrinsic value of action as motivation for behavior. Neuroscience 352, 190–203. doi: 10.1016/j.neuroscience.2017.03.064
Mizuno, K., Tanaka, M., Ishii, A., Tanabe, H. C., Onoe, H., Sadato, N., et al. (2008). The neural basis of academic achievement motivation. Neuroimage 42, 369–378. doi: 10.1016/j.neuroimage.2008.04.253
Mori, A., Klöbl, M., Okada, G., Reed, M. B., Takamura, M., Michenthaler, P., et al. (2019). Predicting ventral striatal activation during reward anticipation from functional connectivity at rest. Front. Hum. Neurosci. 13:289. doi: 10.3389/fnhum.2019.00289
Murayama, K., Matsumoto, M., Izuma, K., and Matsumoto, K. (2010). Neural basis of the undermining effect of monetary reward on intrinsic motivation. Proc. Natl. Acad. Sci. U.S.A. 107, 20911–20916. doi: 10.1073/pnas.1013305107
Murty, V. P., and Adcock, R. A. (2014). Enriched encoding: Reward motivation organizes cortical networks for hippocampal detection of unexpected events. Cereb. Cortex 24, 2160–2168. doi: 10.1093/cercor/bht063
Murty, V. P., LaBar, K. S., and Adcock, R. A. (2016). Distinct medial temporal networks encode surprise during motivation by reward versus punishment. Neurobiol. Learn. Mem. 134, 55–64. doi: 10.1016/j.nlm.2016.01.018
Oldham, S., Murawski, C., Fornito, A., Youssef, G., Yucel, M., and Lorenzetti, V. (2018). The anticipation and outcome phases of reward and loss processing: A neuroimaging meta-analysis of the monetary incentive delay task. Hum. Brain Mapp. 39, 3398–3418. doi: 10.1002/hbm.24184
Plichta, M. M., and Scheres, A. (2014). Ventral-striatal responsiveness during reward anticipation in ADHD and its relation to trait impulsivity in the healthy population: A meta-analytic review of the fMRI literature. Neurosci. Biobehav. Rev. 38, 125–134. doi: 10.1016/j.neubiorev.2013.07.012
Potenza, M., Steinberg, M. A., Skudlarski, P., Fulbright, R., Lacadie, C., Wilber, M., et al. (2003). Gambling urges in pathological gambling: A functional magnetic resonance imaging study. Arch. Gen. Psychiatry 60, 828–836. doi: 10.1001/archpsyc.60.8.828
Rafei, P., Rezapour, T., Bickel, W. K., and Ekhtiari, H. (2021). Imagining the future to reshape the past: A path to combine cue extinction and memory reconsolidation with episodic foresight for addiction treatment. Front. Psychiatry 12:692645. doi: 10.3389/fpsyt.2021.692645
Schott, B. H., Minuzzi, L., Krebs, R. M., Elmenhorst, D., Lang, M., Winz, O. H., et al. (2008). Mesolimbic functional magnetic resonance imaging activations during reward anticipation correlate with reward-related ventral striatal dopamine release. J. Neurosci. 28, 14311–14319. doi: 10.1523/JNEUROSCI.2058-08.2008
Schouppe, N., Demanet, J., Boehler, C. N., Ridderinkhof, K. R., and Notebaert, W. (2014). The role of the striatum in effort-based decision-making in the absence of reward. J. Neurosci. 34, 2148–2154. doi: 10.1523/JNEUROSCI.1214-13.2014
Schutter, D. J., van Bokhoven, I., Vanderschuren, L. J., Lochman, J. E., and Matthys, W. (2010). Risky decision making in substance dependent adolescents with a disruptive behavior disorder. J. Abnorm. Child Psychol. 39, 333–339. doi: 10.1007/s10802-010-9475-1
Sescousse, G., Li, Y., and Dreher, J. C. (2015). A common currency for the computation of motivational values in the human striatum. Soc. Cogn. Affect. Neurosci. 10, 467–473. doi: 10.1093/scan/nsu074
Sescousse, G., Redouté, J., and Dreher, J. C. (2010). The architecture of reward value coding in the human orbitofrontal cortex. J. Neurosci. 30, 13095–13104. doi: 10.1523/JNEUROSCI.3501-10.2010
Setton, R., Fisher, G., and Spreng, R. N. (2019). Mind the gap: Congruence between present and future motivational states shapes prospective decisions. Neuropsychologia 132:107130. doi: 10.1016/j.neuropsychologia.2019.107130
Shashidhara, S., and Erez, Y. (2021). Reward motivation increases univariate activity but has limited effect on coding of task-relevant information across the frontoparietal cortex. Neuropsychologia 160:107981. doi: 10.1016/j.neuropsychologia.2021.107981
Shigemune, Y., Abe, N., Suzuki, M., Ueno, A., Mori, E., Tashiro, M., et al. (2010). Effects of emotion and reward motivation on neural correlates of episodic memory encoding: A PET study. Neurosci. Res. 67, 72–79. doi: 10.1016/j.neures.2010.01.003
Shigemune, Y., Tsukiura, T., Nouchi, R., Kambara, T., and Kawashima, R. (2017). Neural mechanisms underlying the reward-related enhancement of motivation when remembering episodic memories with high difficulty. Hum. Brain Mapp. 38, 3428–3443. doi: 10.1002/hbm.23599
Sorg, B. A. (2012). Reconsolidation of drug memories. Neurosci. Biobehav. Rev. 36, 1400–1417. doi: 10.1016/j.neubiorev.2012.02.004
Symmonds, M., Bossaerts, P., and Dolan, R. J. (2010). A behavioral and neural evaluation of prospective decision-making under risk. J. Neurosci. 30, 14380–14389. doi: 10.1523/JNEUROSCI.1459-10.2010
Van Leijenhorst, L., Zanolie, K., Van Meel, C. S., Westenberg, P. M., Rombouts, S. A., and Crone, E. A. (2010). What motivates the adolescent? Brain regions mediating reward sensitivity across adolescence. Cereb. Cortex 20, 61–69. doi: 10.1093/cercor/bhp078
Vassena, E., Silvetti, M., Boehler, C. N., Achten, E., Fias, W., and Verguts, T. (2014). Overlapping neural systems represent cognitive effort and reward anticipation. PLoS One 9:e91008. doi: 10.1371/journal.pone.0091008
Volkow, N. D., Wang, G. J., Newcorn, J. H., Kollins, S. H., Wigal, T. L., Telang, F., et al. (2011). Motivation deficit in ADHD is associated with dysfunction of the dopamine reward pathway. Mol. Psychiatry 16, 1147–1154. doi: 10.1038/mp.2010.97
Voon, V., Mole, T. B., Banca, P., Porter, L., Morris, L., Mitchell, S., et al. (2014). Neural correlates of sexual cue reactivity in individuals with and without compulsive sexual behaviours. PLoS One 9:e102419. doi: 10.1371/journal.pone.0102419
Webber, H. E., Lopez-Gamundi, P., Stamatovich, S. N., de Wit, H., and Wardle, M. C. (2021). Using pharmacological manipulations to study the role of dopamine in human reward functioning: A review of studies in healthy adults. Neurosci. Biobehav. Rev. 120, 123–158. doi: 10.1016/j.neubiorev.2020.11.004
Weinstein, A., and Lejoyeux, M. (2020). Neurobiological mechanisms underlying Internet and Gaming Disorder (IGD). Dialogues Clin. Neurosci. 22, 113–126. doi: 10.31887/dcns.2020.22.2/aweinstein
Weinstein, A., Livny, A., and Weizman, A. (2017). New developments in brain research of internet and gaming disorder. Neurosci. Biobehav. Rev. 75, 314–330. doi: 10.1016/j.neubiorev.2017.01.040
Weinstein, A. M. (2010). Computer and video game addiction-a comparison between game users and non-game users. Am. .J Drug Alcohol Abuse. 36, 268–276. doi: 10.3109/00952990.2010.491879
Wilson, R. P., Colizzi, M., Bossong, M. G., Allen, P., Kempton, M., and Bhattacharyya, S. (2018). The neural substrate of reward anticipation in health: A meta-analysis of fMRI findings in the monetary incentive delay task. Neuropsychol. Rev. 28, 496–506. doi: 10.1007/s11065-018-9385-5
Yacubian, J., Sommer, T., Schroeder, K., Gläscher, J., Kalisch, R., Leuenberger, B., et al. (2007). Gene-gene interaction associated with neural reward sensitivity. Proc. Natl. Acad. Sci. U.S.A. 104, 8125–8130. doi: 10.1073/pnas.0702029104
Yan, C., Su, L., Wang, Y., Xu, T., Yin, D. Z., Fan, M. X., et al. (2016). Multivariate neural representations of value during reward anticipation and consummation in the human orbitofrontal cortex. Sci. Rep. 6:29079. doi: 10.1038/srep29079
Yang, X., Liu, X., Zeng, Y., Wu, R., Zhao, W., Xin, F., et al. (2021). Secondary rewards acquire enhanced incentive motivation via increasing anticipatory activity of the lateral orbitofrontal cortex. Brain Struct. Funct. 226, 2339–2355. doi: 10.1007/s00429-021-02333-5
Keywords: brain imaging, dopamine, memory, motivation, reward
Citation: Weinstein AM (2023) Reward, motivation and brain imaging in human healthy participants – A narrative review. Front. Behav. Neurosci. 17:1123733. doi: 10.3389/fnbeh.2023.1123733
Received: 14 December 2022; Accepted: 10 March 2023;
Published: 24 March 2023.
Edited by:
Liana Fattore, CNR Neuroscience Institute (IN), ItalyReviewed by:
Vincenzo Micale, University of Catania, ItalyCopyright © 2023 Weinstein. This is an open-access article distributed under the terms of the Creative Commons Attribution License (CC BY). The use, distribution or reproduction in other forums is permitted, provided the original author(s) and the copyright owner(s) are credited and that the original publication in this journal is cited, in accordance with accepted academic practice. No use, distribution or reproduction is permitted which does not comply with these terms.
*Correspondence: Aviv M. Weinstein, YXZpdndlQGFyaWVsLmFjLmls
Disclaimer: All claims expressed in this article are solely those of the authors and do not necessarily represent those of their affiliated organizations, or those of the publisher, the editors and the reviewers. Any product that may be evaluated in this article or claim that may be made by its manufacturer is not guaranteed or endorsed by the publisher.
Research integrity at Frontiers
Learn more about the work of our research integrity team to safeguard the quality of each article we publish.