- 1University of Puerto Rico-Cayey, Cayey, PR, United States
- 2Department of Cell Biology and Physiology, Neuroscience Center, University of North Carolina at Chapel Hill, Chapel Hill, NC, United States
- 3University of Maryland, College Park, College Park, MD, United States
- 4Center for Neuroscience Research, Children’s National Medical Center, Washington, DC, United States
- 5Departments of Pediatrics and Pharmacology and Physiology, The George Washington University School of Medicine and Health Sciences, Washington, DC, United States
Angelman syndrome (AS) is a single-gene neurodevelopmental disorder associated with cognitive and motor impairment, seizures, lack of speech, and disrupted sleep. AS is caused by loss-of-function mutations in the UBE3A gene, and approaches to reinstate functional UBE3A are currently in clinical trials in children. Behavioral testing in a mouse model of AS (Ube3am–/p+) represents an important tool to assess the effectiveness of current and future treatments preclinically. Existing behavioral tests effectively model motor impairments, but not cognitive impairments, in Ube3am–/p+ mice. Here we tested the hypothesis that the 5-choice serial reaction time task (5CSRTT) can be used to assess cognitive behaviors in Ube3am–/p+ mice. Ube3am–/p+ mice had more omissions during 5CSRTT training than wild-type littermate controls, but also showed impaired motor function including open field hypoactivity and delays in eating pellet rewards. Motor impairments thus presented an important confound for interpreting this group difference in omissions. We report that despite hypoactivity during habituation, Ube3am–/p+ mice had normal response latencies to retrieve rewards during 5CSRTT training. We also accounted for delays in eating pellet rewards by assessing omissions solely on trials where eating delays would not impact results. Thus, the increase in omissions in Ube3am–/p+ mice is likely not caused by concurrent motor impairments. This work underscores the importance of considering how known motor impairments in Ube3am–/p+ mice may affect behavioral performance in other domains. Our results also provide guidance on how to design a 5CSRTT protocol that is best suited for future studies in Ube3a mutants.
Introduction
Angelman syndrome (AS) is a rare neurodevelopmental disorder characterized by cognitive and motor impairment, lack of speech, seizures, abnormal EEG patterns, disrupted sleep, short attention span, and a signature behavioral profile that includes hypersociability (Angelman, 1965; Thibert et al., 2013; Bird, 2014; Buiting et al., 2016). Cognitive impairment and motor dysfunction are among the most common features of AS, both affecting nearly all individuals (Thibert et al., 2013). AS is caused by loss-of-function mutations in the maternally inherited UBE3A gene, which encodes UBE3A protein, an E3 ubiquitin ligase involved in regulating protein degradation (Kishino et al., 1997; Lee et al., 2014; Bonello et al., 2017). No effective treatment currently exists for AS, but approaches to unsilence the dormant paternal UBE3A allele have been successful in mouse models (Huang et al., 2011; Meng et al., 2015; Lee et al., 2018; Wolter et al., 2020; Elgersma and Sonzogni, 2021; Milazzo et al., 2021; Schmid et al., 2021) and are now in clinical trials in children (Copping et al., 2021).
The widely used Ube3am–/p+ mouse model (Jiang et al., 1998) recapitulates some of the most common features of AS, including locomotor dysfunction, seizures, abnormal EEG, and sleep impairments (Jiang et al., 1998; Colas et al., 2005; Heck et al., 2008; Allensworth et al., 2011; Ehlen et al., 2015; Shi et al., 2015; Born et al., 2017; Sidorov et al., 2017; Sonzogni et al., 2018; Rotaru et al., 2020; Copping and Silverman, 2021). Mouse behavior has provided a valuable readout to demonstrate the preclinical effectiveness of paternal Ube3a unsilencing and other treatment strategies (van Woerden et al., 2007; Daily et al., 2011; Meng et al., 2015; Sonzogni et al., 2020; Wolter et al., 2020; Milazzo et al., 2021; Schmid et al., 2021). However, cognitive impairment has proven more difficult to model in Ube3am–/p+ mice. Prefrontal cortex is critical for executive function and cognitive control in humans, and impaired prefrontal structure and function has been observed in individuals with autism and other neurodevelopmental and neuropsychiatric disorders (Miller and Cohen, 2001; O’Hearn et al., 2008; Solomon et al., 2014, 2016). Expanding the suite of Ube3am–/p+ behavioral testing to include complex, prefrontally-encoded tasks will enable a wider assessment of the effectiveness of treatments. Recent evidence suggests that loss of Ube3a results in circuit-level impairments in mice in two prefrontal subregions: infralimbic cortex and anterior cingulate cortex (ACC) (Rotaru et al., 2018; Sidorov et al., 2018, 2020). We previously demonstrated that infralimbic circuit dysfunction in Ube3am–/p+ mice can be assessed behaviorally using an operant extinction task (Sidorov et al., 2018). Here, we tested the hypothesis that attentional behavior, regulated in part by ACC circuits, would be impaired in Ube3am–/p+ mice.
The five-choice serial reaction time task (5CSRTT) is a commonly used behavioral test for assessing attention and impulsivity in rodents (Robbins, 2002; Asinof and Paine, 2014; Higgins and Silenieks, 2017). Briefly, food restricted mice are trained to respond to a light cue with a nosepoke to receive a food reward. The light cue has a fixed short duration, and the number of trials omitted (“omissions”) provides a readout of attention, while the number of premature responses during an intertrial interval provides a readout of impulsivity. Lesion studies and chemogenetic manipulations have demonstrated that rodent ACC regulates attentional performance during the 5CSRTT (Chudasama et al., 2003; Koike et al., 2016; Norman et al., 2021). The 5CSRTT has been widely used in rodent models of a variety of neurodevelopmental and neuropsychiatric disorders, including autism, addiction, and attention deficit hyperactivity disorder (Kramvis et al., 2013; Lloyd et al., 2013; Dommett, 2014; Anshu et al., 2017; Caballero-Puntiverio et al., 2017; Justinussen et al., 2020).
Here we report that Ube3am–/p+ mice have both increased omissions and motor impairments during the 5CSRTT. Gross motor hypoactivity in Ube3am–/p+ mice does not drive the change in omissions. However, Ube3am–/p+ mice take longer to eat pellet rewards, confounding interpretations of omissions on adjacent trials. By evaluating only non-adjacent trials, we are able to successfully disassociate omissions from potential motor confounds. This study demonstrates the need to carefully account for motor impairments in Ube3am–/p+ mice when assessing complex behavior.
Results
Ube3am–/p+ mice are hypoactive
We tested the performance of Ube3am–/p+ mice and wild-type (WT) littermates on the 5CSRTT (Figure 1). Prior to training, food restricted mice were first habituated to the behavioral chamber for one session (Figure 2A). During habituation, Ube3am–/p+ mice were less active than WT littermates [Figures 2B,C; t(28) = 6.971, p< 0.0001] but spent similar amounts of time in the center of the chamber [Figure 2D; t(28) = 0.01024, p = 0.9919]. Open field hypoactivity in Ube3am–/p+ mice is expected and has been reported by many groups (Allensworth et al., 2011; Huang et al., 2013; Born et al., 2017; Sonzogni et al., 2018). Following habituation, mice underwent 2 days of magazine training, where pellet rewards were delivered upon every nosepoke into the illuminated food magazine. WT and Ube3am–/p+ mice did not differ in the amount of rewards received during this unrestricted phase [Figure 2E; t(28) = 0.3704, p = 0.7139], suggesting no gross difference in motivation between groups.
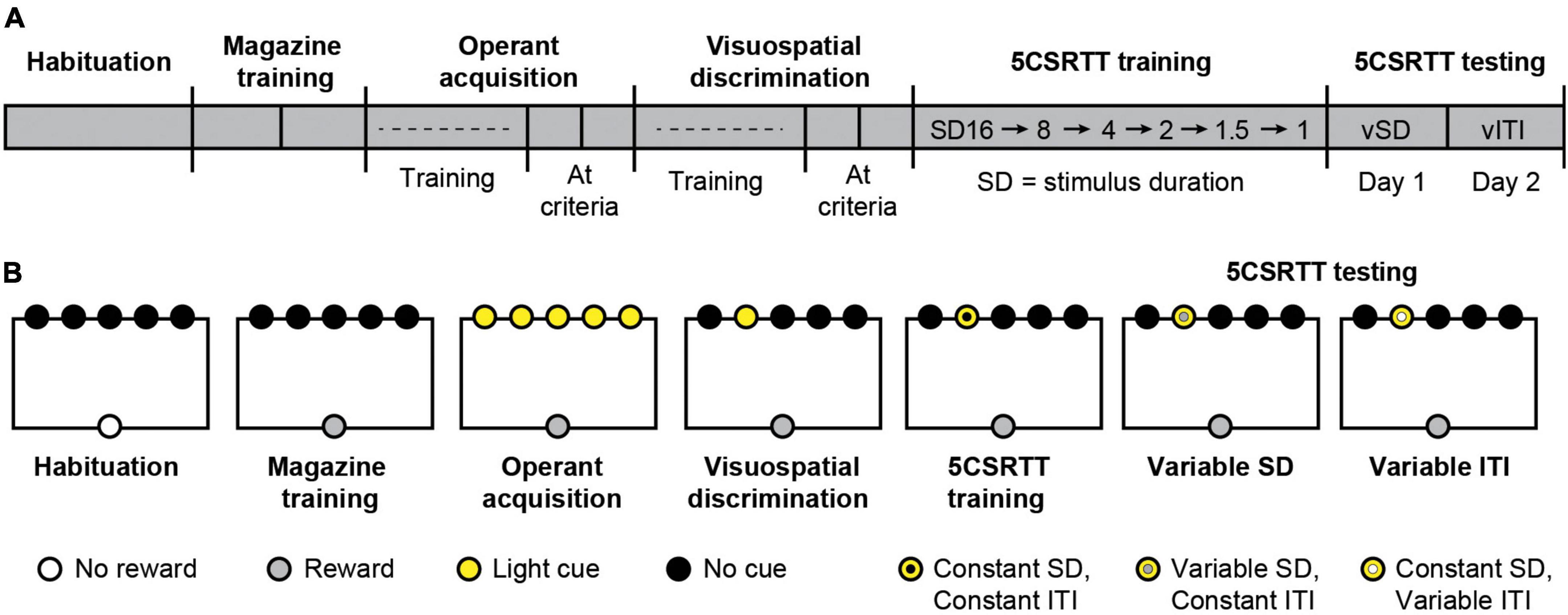
Figure 1. The five-choice serial reaction time task (5CSRTT) measures attention and impulsivity in mice. (A) Timeline of 5CSRTT. vSD, variable stimulus duration; vITI, variable intertrial interval. (B) Schematic of cues and rewards across all stages of 5CSRTT.
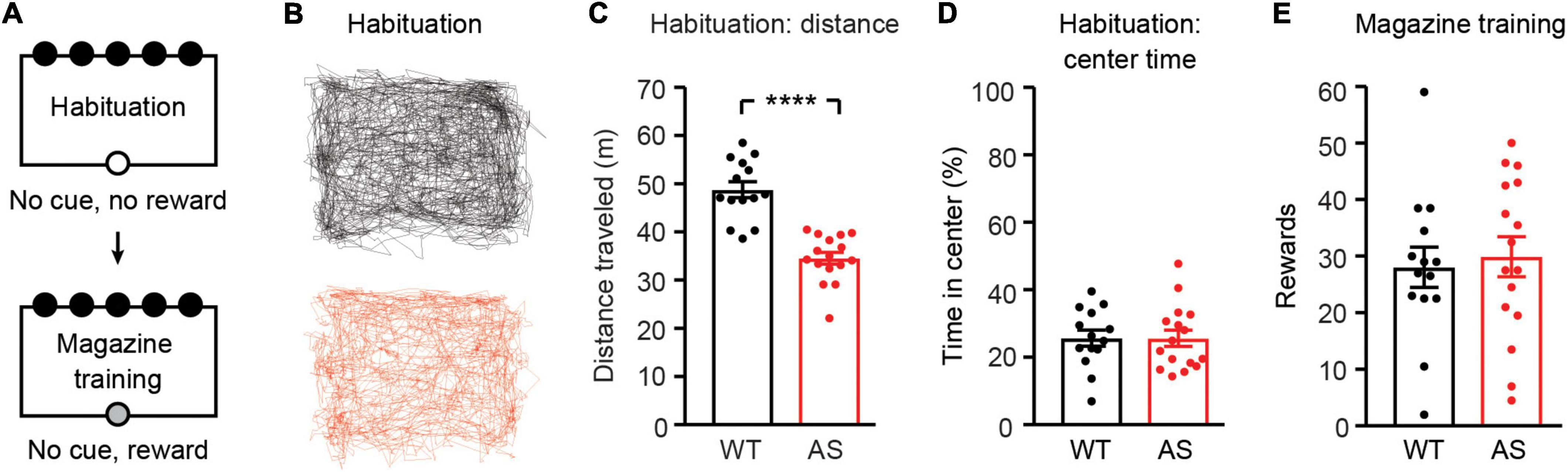
Figure 2. Ube3am–/p+ mice are hypoactive during habituation and receive normal rewards during magazine training. WT: black, n = 14. Ube3am–/p+ (AS): red, n = 16. (A) Schematic of habituation and magazine training phases. (B) Example paths of individual WT and Ube3am–/p+ mice during habituation. (C) Distance traveled and (D) time spent in the center of the arena during habituation. (E) Average rewards received across 2 days of magazine training. ****p< 0.0001. Error bars indicate ± SEM.
Ube3am–/p+ mice show expected impairments in operant acquisition and visuospatial discrimination
Following magazine training, mice underwent operant acquisition and visuospatial discrimination training (Figure 3A). During operant acquisition, a nosepoke into any of five illuminated apertures resulted in reward. During visuospatial discrimination, a nosepoke into one illuminated aperture (that varied each trial) resulted in reward. Operant acquisition and visuospatial discrimination stages were considered complete when mice reached pre-determined performance criteria (see section “Materials and methods”). Ube3am–/p+ mice took longer than WT littermates to complete operant acquisition training (Figures 3B,C; t(28) = 4.251, p = 0.0002). There was no difference between groups in the amount of trials per session during the final 2 days of acquisition [Figure 3D; t(28) = 0.7584, p = 0.4545]. Ube3am–/p+ mice completed visuospatial discrimination training in fewer sessions than WT controls [Figures 3E,F; t(28) = 2.840, p = 0.0083]. Ube3am–/p+ mice completed visuospatial discrimination training faster than WT littermates because they had fewer incorrect responses and a similar amount of correct responses (Supplementary Figure 1), resulting in an increased accuracy (Figure 3E). However, their performance once they reached criteria was not statistically different: mice in both groups averaged ∼60–65% accuracy [criteria = 50%; Figure 3G; t(28) = 1.555, p = 0.1312] and had a similar number of trials per session [Figure 3H; t(28) = 1.451, p = 0.1580].
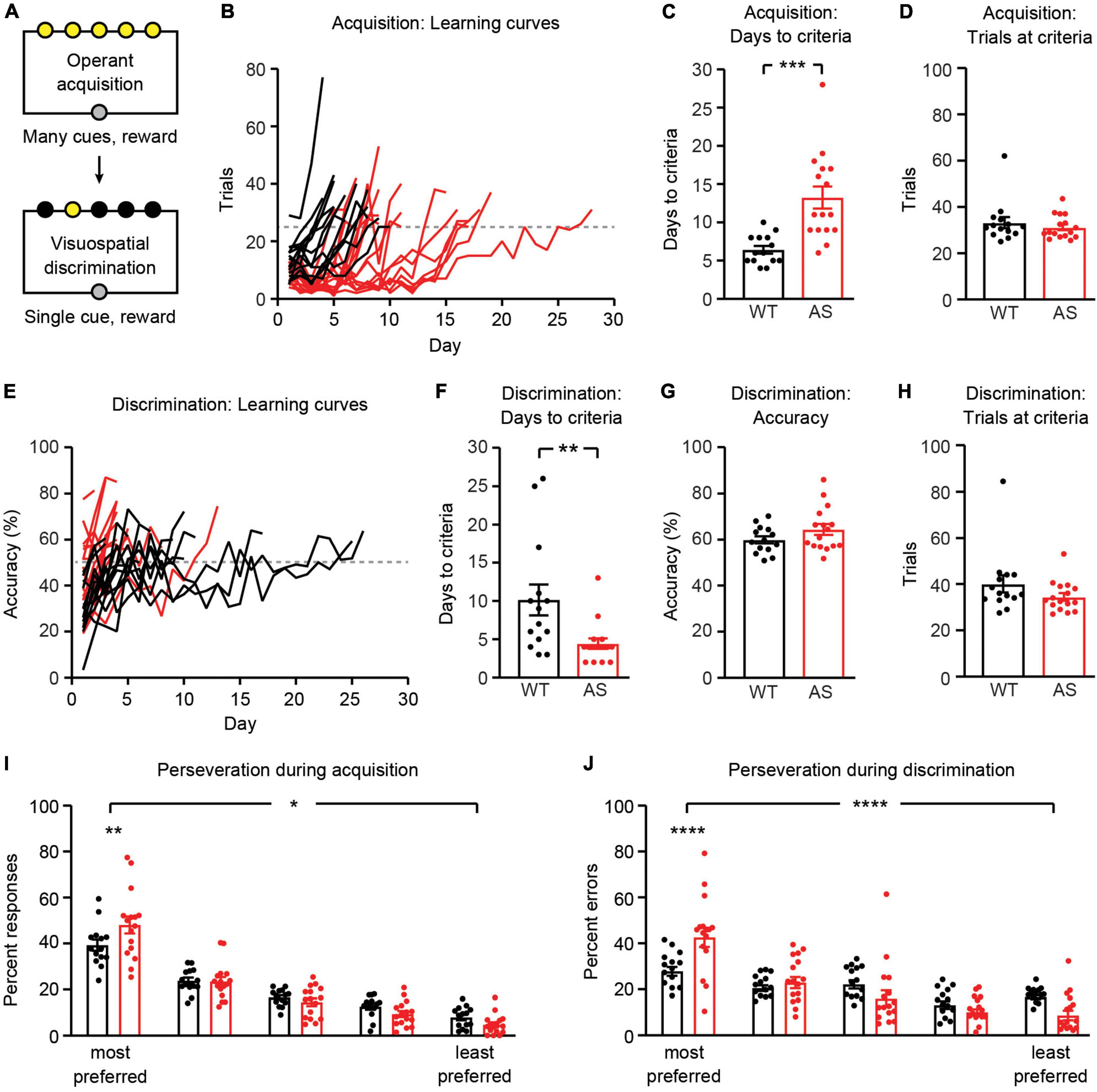
Figure 3. Ube3am–/p+ mice have abnormal performance during operant acquisition and visuospatial discrimination. WT: black, n = 14. Ube3am–/p+ (AS): red, n = 16. (A) Schematic of operant acquisition and visuospatial discrimination phases. (B) Learning curves during operant acquisition; each line represents one mouse and dotted line represents 25 trial per session threshold. (C) Days to reach operant acquisition criteria (>25 trials per session on two consecutive days). (D) Average trials per session on two finals days of operant acquisition, when mice have reached criteria. (E) Learning curves during visuospatial discrimination. Dotted line represents 50% accuracy threshold. (F) Days to reach visuospatial discrimination criteria (>25 trials per session and >50% accuracy on two consecutive days). (G) Average accuracy on two final days of visuospatial discrimination (at criteria). (H) Average trials per session on two final days of visuospatial discrimination (at criteria). (I) Perseveration during operant acquisition: nosepokes are rank-ordered by preference. (J) Perseveration during visuospatial discrimination. *p < 0.05, **p < 0.01, ***p< 0.001, ****p< 0.0001. Error bars indicate ± SEM.
Operant acquisition and the transition from acquisition to discrimination provided an opportunity to assess perseveration in Ube3am–/p+ mice. During operant acquisition, we measured the number of nosepokes into each of five illuminated apertures and rank-ordered the apertures from most preferred to least preferred (by number of nosepokes) for each mouse. Ube3am–/p+ mice were more likely to nosepoke into preferred apertures [Figure 3I; genotype X aperture interaction: F(4, 140) = 3.424, p = 0.0105]. In addition, in Ube3am–/p+ mice, errors during visuospatial discrimination were more commonly made in apertures where animals demonstrated a preference during acquisition [Figure 3J; genotype X aperture interaction: F(4, 140) = 7.143, p < 0.0001]. Together, these results suggest that Ube3am–/p+ mice have increased perseveration and are consistent with our previous study of visuospatial discrimination in Ube3am–/p+ mice (Sidorov et al., 2018).
Ube3am–/p+ mice have increased omissions during five-choice serial reaction time task training
5CSRTT training was similar to visuospatial discrimination in that only one target was illuminated per trial. However, during 5CSRTT training, the target was illuminated only for a fixed stimulus duration (Figure 4A). Thus, in addition to correct and incorrect responses, 5CSRTT trials could also result in two additional outcomes: omissions and premature responses (Figure 4B). Omissions were defined when mice did not respond during either the light cue or during a 4 s limited hold period immediately following light presentation. Premature responses were defined when mice responded during a 5 s intertrial interval prior to light cue. The stimulus duration was constant within each session, and it gradually decreased from 16 to 1 s across sessions, as mice reached pre-determined performance criteria (see section “Materials and methods”). Both Ube3am–/p+ mice and WT mice performed the task with high accuracy (typically > 80%) across all stimulus durations tested (Figure 4C). Accuracy in Ube3am–/p+ mice was statistically higher than WT littermates [main effect of genotype: F(1, 28) = 5.077, p = 0.0323]. Post-hoc tests revealed that the small overall increase in accuracy in Ube3am–/p+ mice was significant only at a stimulus duration of 16 s (p = 0.0196). The increase in accuracy in Ube3am–/p+ mice at the beginning of 5CSRTT training is consistent with the trend toward increased accuracy seen at the end of visuospatial discrimination (Figure 3G) and is driven by Ube3am–/p+ mice having fewer incorrect trials (Supplementary Figure 2).
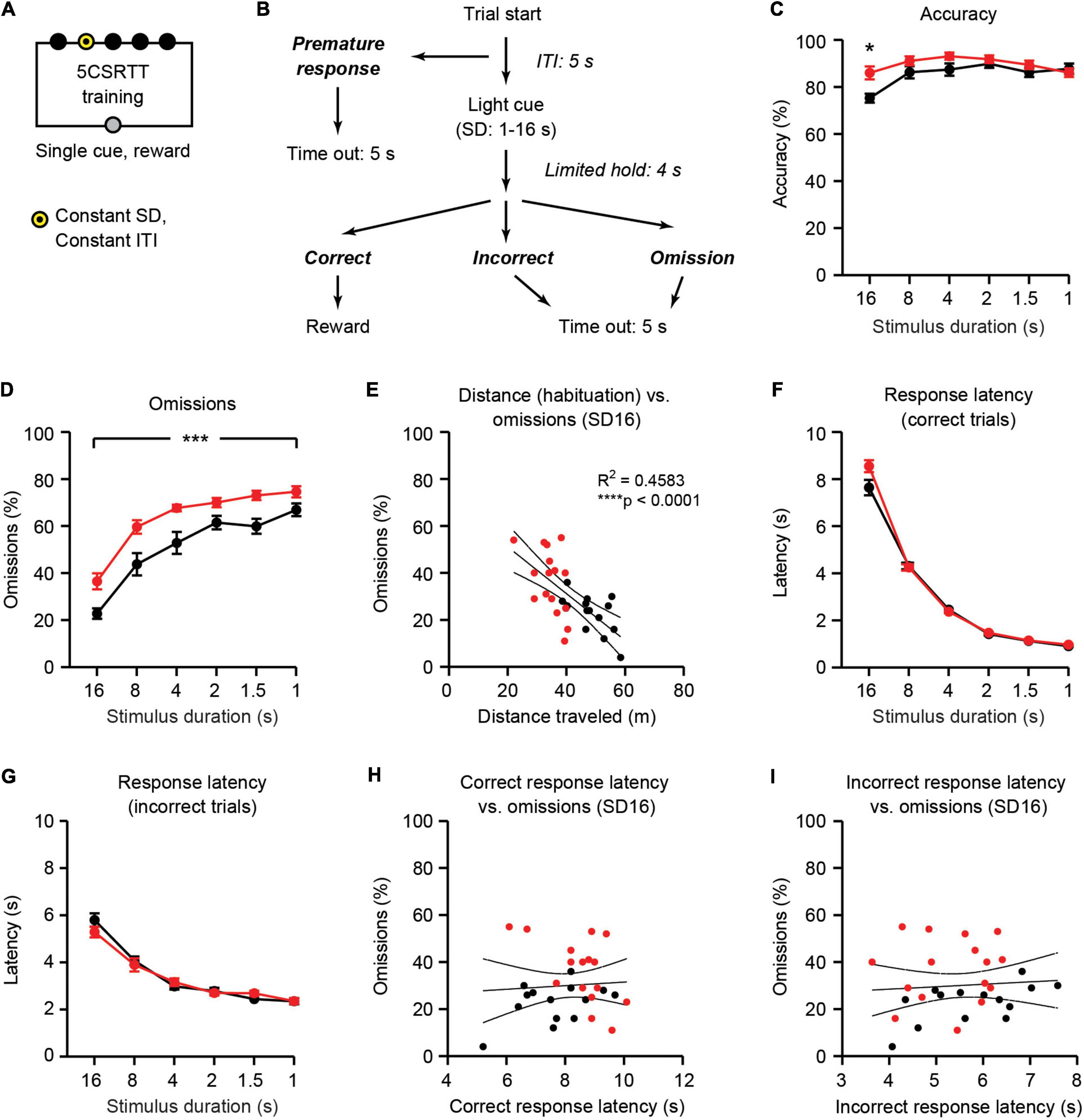
Figure 4. Ube3am–/p+ mice have increased omissions and motor impairments during 5CSRTT training. WT: black, n = 14. Ube3am–/p+ (AS): red, n = 16. (A) Schematic of 5CSRTT training. SD: stimulus duration, ITI: intertrial interval. (B) Trial structure for individual trials (adapted from Asinof and Paine, 2014). (C) Accuracy [correct/(correct + incorrect)] on the final session at each stimulus duration. (D) Omissions during 5CSRTT training are increased in Ube3am–/p+ mice. (E) Distance traveled during habituation is negatively correlated with omissions. (F) Response latency on correct trials. (G) Response latency on incorrect trials. (H) Correct response latency and (I) incorrect response latency are not correlated with omissions. *p < 0.05, ***p< 0.001, ****p< 0.0001. Error bars indicate ± SEM.
As expected, omissions increased across 5CSRTT training as the stimulus duration decreased [Figure 4D; main effect of stimulus duration: F(5, 140) = 105.1, p < 0.0001]. Ube3am–/p+ mice had more omissions than WT littermates across the duration of 5CSRTT training [Figure 4D; main effect of genotype: F(1, 28) = 14.55, p = 0.0007]. There was no interaction between genotype and stimulus duration [F(5, 140) = 1.317, p = 0.2602]. A trend toward increased omissions was also observed in Ube3am–/p+ mice during both 5CSRTT testing phases (Supplementary Figure 3). Impulsivity, defined as the number of premature responses during the intertrial interval, was normal in Ube3am–/p+ mice during 5CSRTT testing (Supplementary Figure 4).
Hypoactivity in Ube3am–/p+ mice confounds measurement of omissions
Increased omissions in Ube3am–/p+ mice during 5CSRTT training are difficult to interpret because of the potential confound of hypoactivity (Figure 2C). Indeed, distance traveled during habituation was negatively correlated with omissions during 5CSRTT training (Figure 4E; R2 = 0.4583, p< 0.0001). We reasoned that if hypoactivity is the underlying cause of increased omissions in Ube3am–/p+ mice, this hypoactivity would be observed both during habituation and during motivated 5CSRTT training sessions. Therefore, we assessed the response latency during correct and incorrect trials during 5CSRTT training. Response latency was defined as the time from light cue to either correct response in the cued aperture or incorrect response in a dark aperture. Response latency on both correct trials and incorrect trials was not different between WT and Ube3am–/p+ mice [Figures 4F,G; main effect of genotype: F(1, 28) = 3.378, p = 0.0767 for correct, F(1, 28) = 0.2395, p = 0.6284 for incorrect]. In addition, neither correct nor incorrect response latency was correlated with omissions within sessions (Figures 4H,I; R2 = 0.004682, p = 0.7194 for correct, R2 = 0.006026, p = 0.6835 for incorrect). Together, these results suggest that it is unlikely that gross motor impairments are the acute cause of increased omissions: at each stimulus duration tested, Ube3am–/p+ mice are able to reach the illuminated aperture in the same amount of time as WT littermates.
Increased omissions in Ube3am–/p+ mice are driven partially, but not fully, by delays in eating rewards from previous trials
Hypoactivity does not seem to be the acute cause of increased omissions in Ube3am–/p+ mice (Figures 4F–I). However, the strong negative correlation between hypoactivity during habituation and omissions during 5CSRTT training (Figure 4E) motivated us to consider other potential confounds in Ube3am–/p+ mice. When observing video recordings of sessions, we noticed that during some omissions, mice were not attending to the stimulus because they had not yet finished eating the reward pellet from the previous trial. We reasoned that if Ube3am–/p+ mice have delays in eating rewards, then this might account for some or all of the increase in omissions observed. Therefore, we asked two related questions: (a) are omissions more common following correct trials, and (b) do Ube3am–/p+ mice take longer to eat pellet rewards?
When the stimulus duration was 1 s, omissions after correct trials (OAC) occurred on >90% of trials in both WT and Ube3am–/p+ groups (Figure 5A). OAC were greater in Ube3am–/p+ mice than in WT littermates [Figure 5A; main effect of genotype: F(1, 28) = 17.24, p = 0.0003]. Ube3am–/p+ mice took significantly longer than WT littermates to eat pellet rewards [Figure 5B; t(26) = 4.234, p = 0.0003]. Eating time was strongly correlated with OAC (Figure 5C; R2 = 0.7716, p < 0.0001). Therefore, we conclude that increased omissions in Ube3am–/p+ mice are confounded by delays in eating rewards from prior trials.
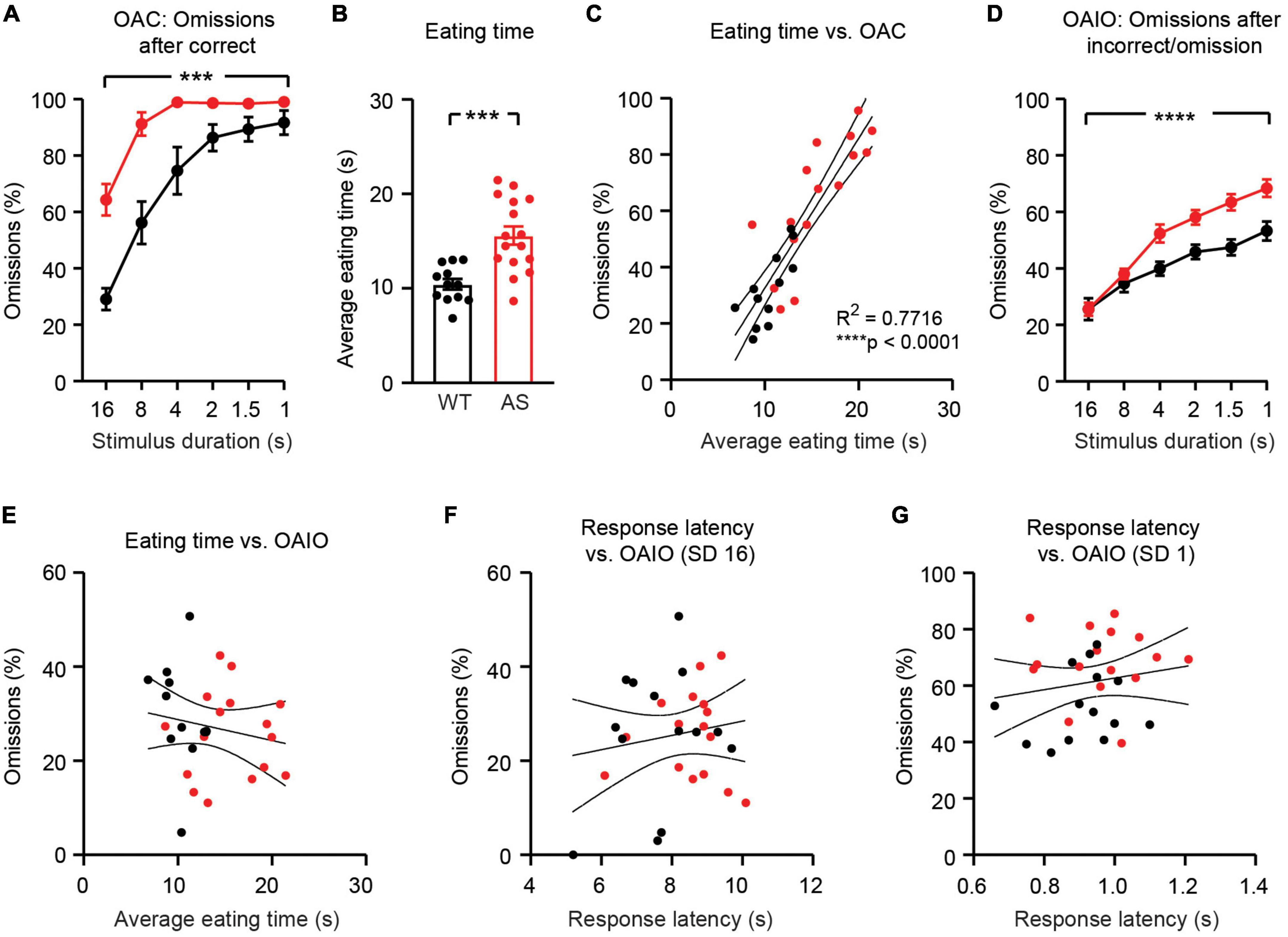
Figure 5. Increased omissions persist in Ube3am–/p+ mice after controlling for motor confounds. WT: black, n = 14 (except n = 12 in (B); two videos were corrupted and not analyzed). Ube3am–/p+ (AS): red, n = 16. (A) Omissions after correct responses (OAC) are greater in Ube3am–/p+ mice. (B) Eating time is greater in Ube3am–/p+ mice. (C) Eating time is tightly correlated with OAC. (D) Omissions after non-correct trials (OAIO) are greater in Ube3am–/p+ mice at low stimulus durations. (E) Eating time is not correlated with OAIO. (F) Response latency is not correlated with OAIO during SD 16 sessions. (G) Response latency is not correlated with OAIO during SD1 sessions.
To remove the confound of delayed pellet eating in Ube3am–/p+ mice, we analyzed omissions on trials that did not follow a correct trial (omissions after incorrect/omission; OAIO). On these trials, no reward is present from the prior trial. OAIO were increased in Ube3am–/p+ mice relative to WT littermates [Figure 5D; main effect of genotype: F(1, 28) = 9.156, p = 0.0053]. Interestingly, increases in OAIO in Ube3am–/p+ mice emerged as the stimulus duration decreased [Figure 5D; genotype × SD interaction: F(5, 140) = 6.008, p < 0.0001]. OAIO were not correlated with eating time (Figure 5E; R2 = 0.03134, p = 0.3675) or response latency (Figures 5F,G; R2 = 0.02314, p = 0.4222 and R2 = 0.01947, p = 0.4621), but were correlated with performance during operant acquisition (Supplementary Figure 5). Expanding the definition of OAIO to also exclude trials following a (correct + omission) sequence did not meaningfully affect results (Supplementary Figure 6). OAIO and pellet eating phenotypes remained statistically robust in Ube3am–/p+ mice after controlling for the age of animals (Supplementary Figure 7), but statistically meaningful group differences in OAIO were not observed during vSD and vITI test days (Supplementary Figure 8). We conclude that OAIO represent an alternative measure of omissions that is impaired in Ube3am–/p+ mice and is not confounded by motor impairments or eating delays.
Discussion
We used the 5CSRTT to test the hypothesis that attentional behavior is impaired in Ube3am–/p+ mice. Ube3am–/p+ mice displayed increased omissions during 5CSRTT training (Figure 4D), suggesting impaired attentional performance. However, Ube3am–/p+ mice were also hypoactive during habituation to the testing environment (Figure 2C). Hypoactivity has been widely reported in Ube3am–/p+ mice (Allensworth et al., 2011; Huang et al., 2013; Born et al., 2017; Sonzogni et al., 2018), and must be considered as a potential confound in any behavioral test using this line. Despite hypoactivity during habituation, Ube3am–/p+ mice had normal response latency during the 5CSRTT (Figures 4F,G). In addition, response latency did not correlate with omissions within individual sessions (Figures 4H,I). Thus, we conclude that hypoactivity is not the cause of increased omissions in Ube3am–/p+ mice. Rather, increased omissions in Ube3am–/p+ were driven partially, but not fully, by delays in eating rewards from prior trials (Figure 5B). Ube3am–/p+ mice averaged 15.6 ± 1.0 s to retrieve and eat rewards, whereas wild-type littermates averaged 10.4 ± 0.6 s. We used an intertrial interval of 5 s (Loos et al., 2009; Koike et al., 2016); thus, omissions on trials immediately following correct trials (Figure 5A) likely reflected when animals were eating rewards. By isolating trials where no prior reward was present (OAIO; omissions after incorrect or omission), we dissociated omissions from confounding eating delays in the Ube3am–/p+ mouse model. Using this approach, we reported a significant interaction between stimulus duration and omissions during 5CSRTT training: omissions were increased only with shorter stimulus durations (Figure 5D). This result suggests that increased omissions may emerge in Ube3am–/p+ mice as the attentional demand of the task increases.
Behavioral performance on the 5CSRTT demonstrates the need to carefully control for motor impairments when studying Ube3am–/p+ mice. While gross motor hypoactivity did not drive omissions, we were surprised to find that Ube3am–/p+ mice took longer to eat pellet rewards than wild-type littermates (Figure 5). It is unlikely that this delay is caused by a lack of motivation or the salience of reward, as Ube3am–/p+ and WT mice earned comparable rewards during unrestricted magazine training (Figure 2) and completed a comparable number of trials during both operant acquisition and visuospatial discrimination (Figure 3). However, we did not explicitly test motivation (e.g., using a progressive ratio test) in the animals used for this study. It is also unlikely that this delay is caused by eating more of the reward pellet: all mice typically ate the entire pellet. Pellet eating time was defined by video analysis as time elapsed from initial head poke into the food magazine to retrieve reward until the reward was fully eaten or dropped. Thus, we hypothesize that the likeliest explanation for increased pellet eating time in Ube3am–/p+ mice is impaired fine motor function (relevant for extracting and holding the pellet) and/or impairments in swallowing and chewing. Swallowing and chewing issues have been reported in individuals with AS (Varela et al., 2004; Glassman et al., 2017), but it is not known whether the Ube3am–/p+ mouse model recapitulates these features. Unfortunately, the video resolution during this study was not sufficient to precisely dissect the cause of the increased pellet eating time observed in Ube3am–/p+ mice. Further investigation is needed to evaluate if swallowing dysfunction, mouth malformations, and/or fine motor impairments are present in the Ube3am–/p+ mouse model.
Future work using the 5CSRTT may consider adjusting task parameters to account for delayed eating time in Ube3am–/p+ mice, regardless of its underlying cause. First, extension of the intertrial interval beyond 5 s could enable all mice to finish eating rewards before the start of the next trial. A 20–30 s intertrial interval would allow mice to finish eating rewards on most trials (Figure 5B). Alternatively, water rewards could be used instead of pellet rewards (Birtalan et al., 2020). Additionally, the 5CSRTT can be automated to allow ad libitium 24 h access to the task via a tube connecting the homecage to the testing chamber (Remmelink et al., 2017; Bruinsma et al., 2019). Self-paced 5CSRTT protocols require an active nosepoke into the empty food magazine to initiate trials, eliminating potential confounds related to pellet eating time on prior trials.
Attentional impairments are common in children with AS, typically manifesting as a short attention span (Tan et al., 2011; Sadhwani et al., 2019). The 5CSRTT provides a tool to assess attentional improvement following drug treatment in rodent models of AS. Typically, attention is assessed on a 5CSRTT test day with variable stimulus duration (Asinof and Paine, 2014). Here, we report a trend toward increased omissions (Supplementary Figure 3, p = 0.06) and OAIO (Supplementary Figure 7, p = 0.07) in Ube3am–/p+ mice during the vSD test day, but these potential differences were not statistically meaningful. Instead, we report group differences in omissions during the 5CSRTT training phase. Impaired attentional performance is thus one of several potential explanations for the increased OAIO seen in Ube3am–/p+ mice. For example, it is possible that Ube3am–/p+ mice have difficulty achieving high rates of operant responding or that they have impairments in behavioral flexibility that are separate from attention. For future 5CSRTT studies in Ube3am–/p+ mice with optimized task parameters, the vSD test day is likely the most appropriate place to assess true attentional performance. Beyond the 5CSRTT, other tasks, such as the continuous performance task, could also be used to assess attentional processing while engaging prefrontal circuits in Ube3am–/p+ mice (Kim et al., 2015; Cope and Young, 2017; Hvoslef-Eide et al., 2018).
We hypothesize that the 5CSRTT may be used in the future as a behavioral readout of prefrontal circuit function in Ube3am–/p+ mice. In rodents, the activity of ACC, a prefrontal subregion, is tightly linked to omissions on the 5CSRTT (Chudasama et al., 2003; Koike et al., 2016; Norman et al., 2021), though other circuits beyond ACC also contribute to attentional processing on the 5CSRTT and related tasks (Chudasama et al., 2012; Aoki et al., 2015; Kim et al., 2016; Wulaer et al., 2020). Future work may test the hypothesis that manipulating Ube3a levels in ACC neurons will selectively affect omissions on the 5CSRTT. More broadly, existing behavioral assessments in Ube3am–/p+ mice are robust and reliable (Sonzogni et al., 2018), but lack test(s) that are driven primarily by prefrontal circuits. Developing readouts of prefrontal function in mouse models of AS will be critical to evaluate the overall effectiveness of treatments. We propose that the 5CSRTT represents an effective way to assess attention while engaging prefrontal circuits.
In addition to increased omissions, Ube3am–/p+ mice also displayed behavioral phenotypes on other phases of the 5CSRTT task. Some of these differences, such as delayed operant acquisition (Figure 3C) and increased perseveration (Figures 3I,J), have been previously reported in Ube3am–/p+ mice (Sidorov et al., 2018). Surprisingly, Ube3am–/p+ mice reached visuospatial discrimination learning criteria faster than WT littermates (Figure 3F). This result implies that Ube3am–/p+ mice were faster learners. The primary criterion used to assess visuospatial discrimination was accuracy, defined as ([correct responses]/[correct responses + incorrect responses]). Ube3am–/p+ mice had a similar amount of correct responses, but had fewer incorrect responses, driving this delay in reaching criteria (Supplementary Figure 1). We hypothesize that this decrease in incorrect responses may be related to our prior finding that Ube3am–/p+ mice have exaggerated operant extinction (Sidorov et al., 2018). While visuospatial discrimination (one light on, changing each trial) is typically considered a test of cognitive flexibility, it may also be interpreted as extinction of a prior rule (all lights on, poke any to receive reward). In this context, fewer incorrect responses would align with our prior finding of exaggerated operant extinction in Ube3am–/p+ mice.
A limit to this study was the sole use of male mice for 5CSRTT experiments. While sex differences in 5CSRTT omissions would not be expected in wild-type mice (Papaleo et al., 2012; Ciampoli et al., 2017; Grissom and Reyes, 2019), sex differences have been reported for certain behaviors in Ube3am–/p+ mice (Sonzogni et al., 2018; Koyavski et al., 2019). In addition, Ube3am–/p+ mice may display sex differences in their responsiveness to environmental enrichment as a treatment in certain behavioral domains (Cosgrove et al., 2022). Future work using a modified 5CSRTT is needed to explicitly assess the role of sex on task performance in Ube3am–/p+ mice. Another potential challenge in the interpretation of results is that Ube3am–/p+ mice were, on average, younger than WT controls (Supplementary Figure 8A). This difference occurred by chance due to the Mendelian inheritance of the mutant Ube3a allele (Supplementary Figure 9). By using age as a covariate for statistical analysis, we confirmed that age differences between groups did not affect our main behavioral findings (Supplementary Figure 8C). While not planned in this case, the group difference in age was beneficial in that it enabled us to study behavior in Ube3am–/p+ mice in groups that were weight-matched (Supplementary Figure 8B). Increased weight has been widely reported in Ube3am–/p+ mice (van Woerden et al., 2007; Huang et al., 2013; Born et al., 2017; Judson et al., 2017, 2021; Sonzogni et al., 2018; Wolter et al., 2020), and weight differences have the potential to confound behavioral tests. Here, behavioral phenotypes were present on the 5CSRTT in Ube3am–/p+ mice that were the same weight as WT controls. Future behavioral studies using Ube3am–/p+ mice may consider weight matching adult mice instead of age matching.
Overall, the 5CSRTT can be used to assess attention and impulsivity in Ube3am–/p+ mice and can be optimized in the future to account for other behavioral impairments in this mouse model. The 5CSRTT can be used in conjunction with existing behavioral assessments to extend the range of testing to include more complex tasks that are likely to be regulated by prefrontal circuits.
Materials and methods
Animals
All methods were carried out in accordance with relevant guidelines and regulations. Procedures were approved by the Institutional Animal Care and Use Committee of the University of North Carolina at Chapel Hill. Mice were group housed on a 12 h light/dark cycle. Experimental AS model mice (Ube3am–/p+) (Jiang et al., 1998) and wild-type littermates (WT; Ube3am+/p+) on a C57BL/6J congenic background were generated by crossing wild-type males and females with paternal Ube3a inheritance (Ube3am+/p–). Mice were genotyped using polymerase chain reaction (PCR) using the following primers: WT Forward (5′-GCTCAAGGTTGTATGCCTTGGTGCT-3′), Mutant Forward (5′- TGCATCGCATTGTGTGAGTAGGTGTC), and WT reverse (5′-ACTTCTCAAGGTAAGCTGAGCTTGC- 3′). Adult male mice (∼P70-P160 at the beginning of study) were used for behavioral experiments (WT mean: 136 ± 6 days, Ube3am–/p+ mean: 109 ± 8 days). A table with information on all breeders and experimental mice is included in Supplementary Figure 9.
Behavioral equipment
We used modular operant conditioning chambers (MED-Associates, ENV-307 W) equipped with five response apertures on one wall and a food magazine on the opposite wall. A chamber light over the magazine illuminated the whole chamber. All chambers were placed in sound-attenuating ventilated cubicles. The response apertures and magazine contained yellow LED stimulus lights and infrared response detectors. Stimulus lights inside the response apertures were controlled individually to provide visual cues as noted.
Five-choice serial reaction time task
We performed the 5CSRTT based on well-established protocols (Pattij et al., 2007; Loos et al., 2009; Koike et al., 2016) with minor modifications. Sample sizes (n = 14–16 mice per group) were determined a priori by availability of mice. Food restricted mice performed multiple stages of the task sequentially: habituation, magazine training, operant acquisition, visuospatial discrimination, 5CSRTT training, and 5CSRTT testing (Figure 1). At each stage, mice performed a single session per day. Testing occurred 7 days a week during the light phase at the same time each day. For the duration of experiments, mice received 2 h of unrestricted feeding immediately after testing with ad libitum access to water. Mice had ad libitum access to water in their home cage at all times. During the task, 20 mg dustless precision pellets (BioServ) were delivered as rewards where noted.
Habituation
Mice were habituated for 25 min inside the behavioral chamber for one session. During habituation, only the house light was on, and no rewards were delivered. Motion was recorded using a camera above the arena (Logitech) and tracked manually using Tracker video analysis software.
Magazine training
Magazine training consisted of sessions on two consecutive days where pellets were delivered into the food magazine with pseudorandom intertrial intervals (ITIs) of 4, 8, 16, and 32 s. Pellet delivery coincided with switching on the magazine light. Retrieval of a pellet initiated the next trial. The magazine light was off during the ITI. Sessions lasted until mice had retrieved 50 pellets or 25 min, whichever came first.
Operant acquisition
Operant acquisition trials began by illuminating the chamber light and all five stimulus apertures. The chamber light remained on for the entire session. A response into any of the five illuminated apertures turned off all the stimulus lights, switched on the magazine light, and delivered a food pellet reward. A trial, defined as a correct response and retrieval of pellet from the magazine, was considered complete when the pellet was retrieved, at which time the magazine light would turn off and the aperture lights would turn back on. Sessions lasted 60 trials or 25 min, whichever came first (Loos et al., 2009). Operant acquisition was considered complete, and mice advanced to visuospatial discrimination, after performing > 25 trials in two consecutive sessions. Mice were “primed” in order to train them to nose poke sufficiently deep into the stimulus apertures to trigger the infrared beam and register a poke. Priming consisted of pellets placed in each of five apertures on the first 2 days of operant acquisition. If less than 5 trials occurred in a subsequent session, mice were primed the following day.
Visuospatial discrimination
Trials began by illuminating the chamber light and only one of the five stimulus apertures. The illuminated aperture varied randomly on a trial-by-trial basis. A response into the illuminated aperture (“correct response”) switched off the stimulus light, switched on the magazine light, and triggered the delivery of a reward. Reward retrieval initiated an ITI of 5 s before the onset of the next trial. Sessions lasted 60 trials or 25 min, whichever came first (Loos et al., 2009). An incorrect response was defined as a response into a non-illuminated aperture. To advance to 5CSRTT training, mice had to reach criteria of > 25 trials and > 50% accuracy (defined as correct responses/[correct responses + incorrect responses]) in two consecutive sessions.
Five-choice serial reaction time task training
Trials (trial structure illustrated in Figure 4B) were similar to visuospatial discrimination, except visual stimuli were presented with a fixed stimulus duration (SD). Correct responses occurred and rewards were delivered if the mouse responded in the illuminated aperture either when the aperture was illuminated or in the 4 s limited hold period after the light turned off. Incorrect responses into a non-illuminated aperture, premature responses, and omissions resulted in a 5 s time-out period, during which all stimulus lights and chamber lights were turned off (Pattij et al., 2007; Loos et al., 2009). The SD remained constant throughout each individual session, and the ITI (5 s) was constant across all sessions. Mice began with a 16 s SD which was gradually decreased in subsequent sessions to 8, 4, 2, 1.5, and 1 s as the subject reached pre-determined criteria (< 30% omissions, > 60% accuracy, > 50 trials) in a single session or after 10 sessions at the same SD if mice did not reach criteria (Figure 1). Sessions lasted 30 min or 100 trials, whichever came first.
Five-choice serial reaction time task testing
5CSRTT testing sessions had a similar trial structure to 5CSRTT training, except that either the SD or ITI varied pseudorandomly within a single session. We performed 2 days of testing, with a variable SD to test attention (Day 1) and a variable ITI to test impulsivity (Day 2) (Figure 1). On Day 1, sessions for test days lasted 30 min or 100 trials, whichever came first. The attentional load was increased by manipulating the SD (1 s; 0.5 s; 0.2 s). On Day 1, the ITI was 5 s, the limited hold was 4 s, and the time out was 5 s. On Day 2, sessions lasted 45 min or 100 trials, whichever came first. Inhibitory control was increased by shortening the manipulating the ITI (5 s; 7.5 s; 12.5 s). On Day 2, the SD was 1 s, the limited hold was 4 s, and the time out was 5 s.
Data analysis
To assess perseveration, we first rank-ordered the five illuminated apertures based on the number of responses into each aperture during operant acquisition (Figure 3I). Next, we carried over these rankings and assessed the number of errors that were made in each aperture during the visuospatial discrimination phase (Figure 3J). This approach (Krueger et al., 2011; Sidorov et al., 2018) allowed us to ask both whether Ube3am–/p+ mice have increased perseveration, and whether this initial perseveration results in errors later on subsequent phases of the task. During 5CSRTT training and testing, response latency was defined as the amount of time from visual cue onset to nosepoke in either the correct or incorrect aperture. Omissions after correct responses (OAC; Figure 5A) were defined as the percentage of omissions on trials immediately following a correct trial. Omissions after incorrect responses or omissions (OAIO; Figure 5D) were defined as the percentage of omissions of trials immediately following an incorrect trial or an omission. Eating time was defined as the amount of time that elapsed from initial head poke to retrieve pellet until the pellet was eaten or dropped. Eating time analysis in Figure 5B was performed in the final session of 5CSRTT training with a SD of 16 s. Two videos at this stage from WT mice were corrupted; thus, n = 12 for WT in Figure 5B.
Statistics
Reported “n” represents animals, and no animals were excluded from behavioral analysis. All error bars indicate ± SEM. Experimenters were blind to genotype and all studies were performed using littermate controls. Student’s t-tests were used in Figures 2C–E, 3C,D,F–H, 5B and Supplementary Figures 8A,B. Two-way ANOVA was used for Figures 3I,J, and Two-way RM ANOVA was used in Figures 4C,D,F,G, 5A,D and Supplementary Figures 1B,C, 2A–C, 3B–D,F–H, 4B, 6A,B, 8A,B, and two-way ANOVA was used in Figures 3I,J. For ANOVAs, post hoc Bonferroni tests were used when there was a main effect of genotype or interaction between genotype and the second factor. Linear regression was used in Figures 4E,H,I, 5C,E–G and Supplementary Figures 5, 7C. Age as a covariate was added in all models in Supplementary Figure 7D using the appropriate lm() or lmer() function in R. Statistical tests were performed using GraphPad Prism 9 and R.
Data availability statement
The original contributions presented in this study are included in the article/Supplementary material, further inquiries can be directed to the corresponding author/s.
Ethics statement
The animal study was reviewed and approved by the Institutional Animal Care and Use Committee of the University of North Carolina at Chapel Hill.
Author contributions
MS and PN-M contributed to conception and design of the study and wrote the first draft of the manuscript. PN-M performed behavioral studies. PN-M, DD, CG, and MS contributed to data analysis and statistical analysis and contributed to sections of the manuscript. All authors contributed to manuscript revision, read, and approved the submitted version.
Funding
This work was supported by NIH R00EY028964 to MS.
Acknowledgments
We thank Matt Leff and Courtney Davis for assisting with behavioral studies.
Conflict of interest
The authors declare that the research was conducted in the absence of any commercial or financial relationships that could be construed as a potential conflict of interest.
Publisher’s note
All claims expressed in this article are solely those of the authors and do not necessarily represent those of their affiliated organizations, or those of the publisher, the editors and the reviewers. Any product that may be evaluated in this article, or claim that may be made by its manufacturer, is not guaranteed or endorsed by the publisher.
Supplementary material
The Supplementary Material for this article can be found online at: https://www.frontiersin.org/articles/10.3389/fnbeh.2022.968159/full#supplementary-material
References
Allensworth, M., Saha, A., Reiter, L. T., and Heck, D. H. (2011). Normal social seeking behavior, hypoactivity and reduced exploratory range in a mouse model of Angelman syndrome. BMC Genet. 12:7. doi: 10.1186/1471-2156-12-7
Angelman, H. (1965). “Puppet” children. A report on three cases. Dev. Med. Child Neurol. 7, 681–688. doi: 10.1111/j.1469-8749.1965.tb07844.x
Anshu, K., Nair, A. K., Kumaresan, U. D., Kutty, B. M., Srinath, S., and Laxmi, T. R. (2017). Altered attentional processing in male and female rats in a prenatal valproic acid exposure model of autism spectrum disorder. Autism Res. 10, 1929–1944. doi: 10.1002/aur.1852
Aoki, S., Liu, A. W., Zucca, A., Zucca, S., and Wickens, J. R. (2015). Role of Striatal Cholinergic Interneurons in Set-Shifting in the Rat. J. Neurosci. 35, 9424–9431. doi: 10.1523/JNEUROSCI.0490-15.2015
Asinof, S. K., and Paine, T. A. (2014). The 5-choice serial reaction time task: a task of attention and impulse control for rodents. J. Vis. Exp. 90:e51574. doi: 10.3791/51574
Bird, L. M. (2014). Angelman syndrome: review of clinical and molecular aspects. Appl. Clin. Genet. 7, 93–104. doi: 10.2147/TACG.S57386
Birtalan, E., Banhidi, A., Sanders, J. I., Balazsfi, D., and Hangya, B. (2020). Efficient training of mice on the 5-choice serial reaction time task in an automated rodent training system. Sci. Rep. 10:22362. doi: 10.1038/s41598-020-79290-2
Bonello, D., Camilleri, F., and Calleja-Agius, J. (2017). Angelman Syndrome: Identification and Management. Neonatal. Netw. 36, 142–151. doi: 10.1891/0730-0832.36.3.142
Born, H. A., Dao, A. T., Levine, A. T., Lee, W. L., Mehta, N. M., Mehra, S., et al. (2017). Strain-dependence of the Angelman Syndrome phenotypes in Ube3a maternal deficiency mice. Sci. Rep. 7:8451. doi: 10.1038/s41598-017-08825-x
Bruinsma, B., Terra, H., de Kloet, S. F., Luchicchi, A., Timmerman, A. J., Remmelink, E., et al. (2019). An automated home-cage-based 5-choice serial reaction time task for rapid assessment of attention and impulsivity in rats. Psychopharmacology 236, 2015–2026. doi: 10.1007/s00213-019-05189-0
Buiting, K., Williams, C., and Horsthemke, B. (2016). Angelman syndrome - insights into a rare neurogenetic disorder. Nat. Rev. Neurol 12, 584–593. doi: 10.1038/nrneurol.2016.133
Caballero-Puntiverio, M., Fitzpatrick, C. M., Woldbye, D. P., and Andreasen, J. T. (2017). Effects of amphetamine and methylphenidate on attentional performance and impulsivity in the mouse 5-Choice Serial Reaction Time Task. J. Psychopharmacol. 31, 272–283. doi: 10.1177/0269881116684339
Chudasama, Y., Doobay, V. M., and Liu, Y. (2012). Hippocampal-prefrontal cortical circuit mediates inhibitory response control in the rat. J. Neurosci. 32, 10915–10924. doi: 10.1523/JNEUROSCI.1463-12.2012
Chudasama, Y., Passetti, F., Rhodes, S. E., Lopian, D., Desai, A., and Robbins, T. W. (2003). Dissociable aspects of performance on the 5-choice serial reaction time task following lesions of the dorsal anterior cingulate, infralimbic and orbitofrontal cortex in the rat: differential effects on selectivity, impulsivity and compulsivity. Behav. Brain Res. 146, 105–119. doi: 10.1016/j.bbr.2003.09.020
Ciampoli, M., Contarini, G., Mereu, M., and Papaleo, F. (2017). Attentional Control in Adolescent Mice Assessed with a Modified Five Choice Serial Reaction Time Task. Sci. Rep. 7:9936. doi: 10.1038/s41598-017-10112-8
Colas, D., Wagstaff, J., Fort, P., Salvert, D., and Sarda, N. (2005). Sleep disturbances in Ube3a maternal-deficient mice modeling Angelman syndrome. Neurobiol. Dis. 20, 471–478. doi: 10.1016/j.nbd.2005.04.003
Cope, Z. A., and Young, J. W. (2017). The Five-Choice Continuous Performance Task (5C-CPT): A Cross-Species Relevant Paradigm for Assessment of Vigilance and Response Inhibition in Rodents. Curr. Protoc. Neurosci. 78, 9.56.1–9.56.18. doi: 10.1002/cpns.20
Copping, N. A., and Silverman, J. L. (2021). Abnormal electrophysiological phenotypes and sleep deficits in a mouse model of Angelman Syndrome. Mol. Autism 12:9. doi: 10.1186/s13229-021-00416-y
Copping, N. A., McTighe, S. M., Fink, K. D., and Silverman, J. L. (2021). Emerging Gene and Small Molecule Therapies for the Neurodevelopmental Disorder Angelman Syndrome. Neurotherapeutics 18, 1535–1547 doi: 10.1007/s13311-021-01082-x
Cosgrove, J. A., Kelly, L. K., Kiffmeyer, E. A., and Kloth, A. D. (2022). Sex-dependent influence of postweaning environmental enrichment in Angelman syndrome model mice. Brain Behav. 12:e2468. doi: 10.1002/brb3.2468
Daily, J. L., Nash, K., Jinwal, U., Golde, T., Rogers, J., Peters, M. M., et al. (2011). Adeno-associated virus-mediated rescue of the cognitive defects in a mouse model for Angelman syndrome. PLoS One. 6:e27221. doi: 10.1371/journal.pone.0027221
Dommett, E. J. (2014). Using the five-choice serial reaction time task to examine the effects of atomoxetine and methylphenidate in the male spontaneously hypertensive rat. Pharmacol. Biochem. Behav. 124, 196–203. doi: 10.1016/j.pbb.2014.06.001
Ehlen, J. C., Jones, K. A., Pinckney, L., Gray, C. L., Burette, S., Weinberg, R. J., et al. (2015). Maternal Ube3a Loss Disrupts Sleep Homeostasis But Leaves Circadian Rhythmicity Largely Intact. J. Neurosci. 35, 13587–13598. doi: 10.1523/JNEUROSCI.2194-15.2015
Elgersma, Y., and Sonzogni, M. (2021). UBE3A reinstatement as a disease-modifying therapy for Angelman syndrome. Dev. Med. Child Neurol 63, 802–807. doi: 10.1111/dmcn.14831
Glassman, L. W., Grocott, O. R., Kunz, P. A., Larson, A. M., Zella, G., Ganguli, K., et al. (2017). Prevalence of gastrointestinal symptoms in Angelman syndrome. Am. J. Med. Genet. A 173, 2703–2709. doi: 10.1002/ajmg.a.38401
Grissom, N. M., and Reyes, T. M. (2019). Let’s call the whole thing off: evaluating gender and sex differences in executive function. Neuropsychopharmacology 44, 86–96. doi: 10.1038/s41386-018-0179-5
Heck, D. H., Zhao, Y., Roy, S., LeDoux, M. S., and Reiter, L. T. (2008). Analysis of cerebellar function in Ube3a-deficient mice reveals novel genotype-specific behaviors. Hum. Mol. Genet. 17, 2181–2189. doi: 10.1093/hmg/ddn117
Higgins, G. A., and Silenieks, L. B. (2017). Rodent Test of Attention and Impulsivity: The 5-Choice Serial Reaction Time Task. Curr. Protoc. Pharmacol. 78, 5491–534. doi: 10.1002/cpph.27
Huang, H. S., Allen, J. A., Mabb, A. M., King, I. F., Miriyala, J., Taylor-Blake, B., et al. (2011). Topoisomerase inhibitors unsilence the dormant allele of Ube3a in neurons. Nature 481, 185–189. doi: 10.1038/nature10726
Huang, H. S., Burns, A. J., Nonneman, R. J., Baker, L. K., Riddick, N. V., Nikolova, V. D., et al. (2013). Behavioral deficits in an Angelman syndrome model: effects of genetic background and age. Behav. Brain Res. 243, 79–90. doi: 10.1016/j.bbr.2012.12.052
Hvoslef-Eide, M., Nilsson, S. R., Hailwood, J. M., Robbins, T. W., Saksida, L. M., Mar, A. C., et al. (2018). Effects of anterior cingulate cortex lesions on a continuous performance task for mice. Brain Neurosci. Adv. 2:2398212818772962 doi: 10.1177/2398212818772962
Jiang, Y. H., Armstrong, D., Albrecht, U., Atkins, C. M., Noebels, J. L., Eichele, G., et al. (1998). Mutation of the Angelman Ubiquitin Ligase in Mice Causes Increased Cytoplasmic p53 and Deficits of Contextual Learning and Long-Term Potentiation. Neuron 21, 799–811. doi: 10.1016/S0896-6273(00)80596-6
Judson, M. C., Burette, A. C., Thaxton, C. L., Pribisko, A. L., Shen, M. D., Rumple, A. M., et al. (2017). Decreased Axon Caliber Underlies Loss of Fiber Tract Integrity. Disproportional Reductions in White Matter Volume, and Microcephaly in Angelman Syndrome Model Mice. J. Neurosci. 37, 7347–7361. doi: 10.1523/JNEUROSCI.0037-17.2017
Judson, M. C., Shyng, C., Simon, J. M., Davis, C. R., Punt, A. M., Salmon, M. T., et al. (2021). Dual-isoform hUBE3A gene transfer improves behavioral and seizure outcomes in Angelman syndrome model mice. JCI Insight 6:e144712 doi: 10.1172/jci.insight.144712
Justinussen, J., Dall, C., Dencker, D., Gjedde, A., Fink-Jensen, A., and Thomsen, M. (2020). Revealing a compulsive phenotype in cholinergic M4(-/-) mice depends on the inter-trial interval initiation settings in a five choice serial reaction time task. Behav. Brain Res. 389:112649. doi: 10.1016/j.bbr.2020.112649
Kim, C. H., Hvoslef-Eide, M., Nilsson, S. R., Johnson, M. R., Herbert, B. R., Robbins, T. W., et al. (2015). The continuous performance test (rCPT) for mice: a novel operant touchscreen test of attentional function. Psychopharmacology 232, 3947–3966. doi: 10.1007/s00213-015-4081-0
Kim, H., Ahrlund-Richter, S., Wang, X., Deisseroth, K., and Carlen, M. (2016). Prefrontal Parvalbumin Neurons in Control of Attention. Cell 164, 208–218. doi: 10.1016/j.cell.2015.11.038
Kishino, T., Lalande, M., and Wagstaff, J. (1997). UBE3A/E6-AP mutations cause Angelman syndrome. Nat. Genet. 15, 70–73. doi: 10.1038/ng0197-70
Koike, H., Demars, M. P., Short, J. A., Nabel, E. M., Akbarian, S., Baxter, M. G., et al. (2016). Chemogenetic Inactivation of Dorsal Anterior Cingulate Cortex Neurons Disrupts Attentional Behavior in Mouse. Neuropsychopharmacology 41, 1014–1023. doi: 10.1038/npp.2015.229
Koyavski, L., Panov, J., Simchi, L., Rayi, P. R., Sharvit, L., Feuermann, Y., et al. (2019). Sex-Dependent Sensory Phenotypes and Related Transcriptomic Expression Profiles Are Differentially Affected by Angelman Syndrome. Mol. Neurobiol. 56, 5998–6016. doi: 10.1007/s12035-019-1503-8
Kramvis, I., Mansvelder, H. D., Loos, M., and Meredith, R. (2013). Hyperactivity, perseveration and increased responding during attentional rule acquisition in the Fragile X mouse model. Front. Behav. Neurosci. 7:172. doi: 10.3389/fnbeh.2013.00172
Krueger, D. D., Osterweil, E. K., Chen, S. P., Tye, L. D., and Bear, M. F. (2011). Cognitive dysfunction and prefrontal synaptic abnormalities in a mouse model of fragile X syndrome. Proc. Natl. Acad. Sci. U.S.A. 108, 2587–2592. doi: 10.1073/pnas.1013855108
Lee, H. M., Clark, E. P., Kuijer, M. B., Cushman, M., Pommier, Y., and Philpot, B. D. (2018). Characterization and structure-activity relationships of indenoisoquinoline-derived topoisomerase I inhibitors in unsilencing the dormant Ube3a gene associated with Angelman syndrome. Mol. Autism 9, 45. doi: 10.1186/s13229-018-0228-2
Lee, S. Y., Ramirez, J., Franco, M., Lectez, B., Gonzalez, M., Barrio, R., et al. (2014). Ube3a, the E3 ubiquitin ligase causing Angelman syndrome and linked to autism, regulates protein homeostasis through the proteasomal shuttle Rpn10. Cell Mol. Life Sci. 71, 2747–2758. doi: 10.1007/s00018-013-1526-7
Lloyd, S. A., Oltean, C., Pass, H., Phillips, B., Staton, K., Robertson, C. L., et al. (2013). Prenatal exposure to psychostimulants increases impulsivity, compulsivity, and motivation for rewards in adult mice. Physiol. Behav. 119, 43–51. doi: 10.1016/j.physbeh.2013.05.038
Loos, M., van der Sluis, S., Bochdanovits, Z., van Zutphen, I. J., Pattij, T., Stiedl, O., et al. (2009). Activity and impulsive action are controlled by different genetic and environmental factors. Genes. Brain Behav. 8, 817–828. doi: 10.1111/j.1601-183X.2009.00528.x
Meng, L., Ward, A. J., Chun, S., Bennett, C. F., Beaudet, A. L., and Rigo, F. (2015). Towards a therapy for Angelman syndrome by targeting a long non-coding RNA. Nature 518, 409–412. doi: 10.1038/nature13975
Milazzo, C., Mientjes, E. J., Wallaard, I., Rasmussen, S. V., Erichsen, K. D., Kakunuri, T., et al. (2021). Antisense oligonucleotide treatment rescues UBE3A expression and multiple phenotypes of an Angelman syndrome mouse model. JCI Insight 6:e145991 doi: 10.1172/jci.insight.145991
Miller, E. K., and Cohen, J. D. (2001). An integrative theory of prefrontal cortex function. Annu. Rev. Neurosci. 24, 167–202. doi: 10.1146/annurev.neuro.24.1.167
Norman, K. J., Koike, H., McCraney, S. E., Garkun, Y., Bateh, J., Falk, E. N., et al. (2021). Chemogenetic suppression of anterior cingulate cortical neurons projecting to the visual cortex disrupts attentional behavior in mice. Neuropsychopharmacol. Rep. 41, 207–214. doi: 10.1002/npr2.12176
O’Hearn, K., Asato, M., Ordaz, S., and Luna, B. (2008). Neurodevelopment and executive function in autism. Dev. Psychopathol. 20, 1103–1132. doi: 10.1017/S0954579408000527
Papaleo, F., Erickson, L., Liu, G., Chen, J., and Weinberger, D. R. (2012). Effects of sex and COMT genotype on environmentally modulated cognitive control in mice. Proc. Natl. Acad. Sci. U.S.A. 109, 20160–20165. doi: 10.1073/pnas.1214397109
Pattij, T., Janssen, M. C., Loos, M., Smit, A. B., Schoffelmeer, A. N., and van Gaalen, M. M. (2007). Strain specificity and cholinergic modulation of visuospatial attention in three inbred mouse strains. Genes Brain Behav. 6, 579–587. doi: 10.1111/j.1601-183X.2006.00284.x
Remmelink, E., Chau, U., Smit, A. B., Verhage, M., and Loos, M. A. (2017). one-week 5-choice serial reaction time task to measure impulsivity and attention in adult and adolescent mice. Sci. Rep. 7:42519. doi: 10.1038/srep42519
Robbins, T. W. (2002). The 5-choice serial reaction time task: behavioural pharmacology and functional neurochemistry. Psychopharmacology 163, 362–380. doi: 10.1007/s00213-002-1154-7
Rotaru, D. C., Mientjes, E. J., and Elgersma, Y. (2020). Angelman Syndrome: From Mouse Models to Therapy. Neuroscience 445, 172–189. doi: 10.1016/j.neuroscience.2020.02.017
Rotaru, D. C., van Woerden, G. M., Wallaard, I., and Elgersma, Y. (2018). Adult Ube3a Gene Reinstatement Restores the Electrophysiological Deficits of Prefrontal Cortex Layer 5 Neurons in a Mouse Model of Angelman Syndrome. J. Neurosci. 38, 8011–8030. doi: 10.1523/JNEUROSCI.0083-18.2018
Sadhwani, A., Willen, J. M., LaVallee, N., Stepanians, M., Miller, H., Peters, S. U., et al. (2019). Maladaptive behaviors in individuals with Angelman syndrome. Am. J. Med. Genet. A 179, 983–992. doi: 10.1002/ajmg.a.61140
Schmid, R. S., Deng, X., Panikker, P., Msackyi, M., Breton, C., and Wilson, J. M. (2021). CRISPR/Cas9 directed to the Ube3a antisense transcript improves Angelman syndrome phenotype in mice. J. Clin. Invest. 131:e142574 doi: 10.1172/JCI142574
Shi, S. Q., Bichell, T. J., Ihrie, R. A., and Johnson, C. H. (2015). Ube3a imprinting impairs circadian robustness in Angelman syndrome models. Curr. Biol. 25, 537–545. doi: 10.1016/j.cub.2014.12.047
Sidorov, M. S., Deck, G. M., Dolatshahi, M., Thibert, R. L., Bird, L. M., Chu, C. J., et al. (2017). Delta rhythmicity is a reliable EEG biomarker in Angelman syndrome: a parallel mouse and human analysis. J. Neurodev. Disord. 9:17. doi: 10.1186/s11689-017-9195-8
Sidorov, M. S., Judson, M. C., Kim, H., Rougie, M., Ferrer, A. I., Nikolova, V. D., et al. (2018). Enhanced Operant Extinction and Prefrontal Excitability in a Mouse Model of Angelman Syndrome. J. Neurosci. 38, 2671–2682. doi: 10.1523/JNEUROSCI.2828-17.2018
Sidorov, M. S., Kim, H., Rougie, M., Williams, B., Siegel, J. J., Gavornik, J. P., et al. (2020). Visual Sequences Drive Experience-Dependent Plasticity in Mouse Anterior Cingulate Cortex. Cell. Rep. 32:108152. doi: 10.1016/j.celrep.2020.108152
Solomon, M., McCauley, J. B., Iosif, A. M., Carter, C. S., and Ragland, J. D. (2016). Cognitive control and episodic memory in adolescents with autism spectrum disorders. Neuropsychologia 89, 31–41. doi: 10.1016/j.neuropsychologia.2016.05.013
Solomon, M., Yoon, J. H., Ragland, J. D., Niendam, T. A., Lesh, T. A., Fairbrother, W., et al. (2014). The development of the neural substrates of cognitive control in adolescents with autism spectrum disorders. Biol. Psychiatry 76, 412–421. doi: 10.1016/j.biopsych.2013.08.036
Sonzogni, M., Wallaard, I., Santos, S. S., Kingma, J., du Mee, D., van Woerden, G. M., et al. (2018). A behavioral test battery for mouse models of Angelman syndrome: a powerful tool for testing drugs and novel Ube3a mutants. Mol. Autism. 9:47. doi: 10.1186/s13229-018-0231-7
Sonzogni, M., Zhai, P., Mientjes, E. J., van Woerden, G. M., and Elgersma, Y. (2020). Assessing the requirements of prenatal UBE3A expression for rescue of behavioral phenotypes in a mouse model for Angelman syndrome. Mol. Autism 11:70. doi: 10.1186/s13229-020-00376-9
Tan, W. H., Bacino, C. A., Skinner, S. A., Anselm, I., Barbieri-Welge, R., Bauer-Carlin, A., et al. (2011). Angelman syndrome: Mutations influence features in early childhood. Am. J. Med. Genet. A. 155A, 81–90. doi: 10.1002/ajmg.a.33775
Thibert, R. L., Larson, A. M., Hsieh, D. T., Raby, A. R., and Thiele, E. A. (2013). Neurologic manifestations of Angelman syndrome. Pediatr. Neurol. 48, 271–279. doi: 10.1016/j.pediatrneurol.2012.09.015
van Woerden, G. M., Harris, K. D., Hojjati, M. R., Gustin, R. M., Qiu, S., de Avila Freire, R., et al. (2007). Rescue of neurological deficits in a mouse model for Angelman syndrome by reduction of alphaCaMKII inhibitory phosphorylation. Nat. Neurosci. 10, 280–282. doi: 10.1038/nn1845
Varela, M. C., Kok, F., Otto, P. A., and Koiffmann, C. P. (2004). Phenotypic variability in Angelman syndrome: comparison among different deletion classes and between deletion and UPD subjects. Eur. J. Hum. Genet. 12, 987–992. doi: 10.1038/sj.ejhg.5201264
Wolter, J. M., Mao, H., Fragola, G., Simon, J. M., Krantz, J. L., Bazick, H. O., et al. (2020). Cas9 gene therapy for Angelman syndrome traps Ube3a-ATS long non-coding RNA. Nature 587, 281–284. doi: 10.1038/s41586-020-2835-2
Keywords: Angelman syndrome, behavior, attention, motor, five-choice serial reaction time task
Citation: Negrón-Moreno PN, Diep DT, Guoynes CD and Sidorov MS (2022) Dissociating motor impairment from five-choice serial reaction time task performance in a mouse model of Angelman syndrome. Front. Behav. Neurosci. 16:968159. doi: 10.3389/fnbeh.2022.968159
Received: 13 June 2022; Accepted: 05 September 2022;
Published: 23 September 2022.
Edited by:
Hirofumi Morishita, Icahn School of Medicine at Mount Sinai, United StatesReviewed by:
Miguel Skirzewski, Rodent Cognition Research and Innovation Core/University of Western Ontario, CanadaAdam C. Mar, Grossman School of Medicine, New York University, United States
Copyright © 2022 Negrón-Moreno, Diep, Guoynes and Sidorov. This is an open-access article distributed under the terms of the Creative Commons Attribution License (CC BY). The use, distribution or reproduction in other forums is permitted, provided the original author(s) and the copyright owner(s) are credited and that the original publication in this journal is cited, in accordance with accepted academic practice. No use, distribution or reproduction is permitted which does not comply with these terms.
*Correspondence: Michael S. Sidorov, msidorov@childrensnational.org