- 1Physical Fitness Research Institute, Meiji Yasuda Life Foundation of Health and Welfare, Tokyo, Japan
- 2Extreme Environments Laboratory, School of Sport, Health and Exercise Science, University of Portsmouth, Portsmouth, United Kingdom
- 3Institute of Sport, University of Chichester, Chichester, United Kingdom
- 4Graduate School of Informatics and Engineering, The University of Electro-Communications, Chofu, Japan
It is well established that acute moderate-intensity exercise improves cognitive performance. However, the effects of acute high-intensity aerobic exercise on cognitive performance have not been well characterized. In this review, we summarize the literature investigating the exercise-cognition interaction, especially focusing on high-intensity aerobic exercise. We discuss methodological and physiological factors that potentially mediate cognitive performance in response to high-intensity exercise. We propose that the effects of high-intensity exercise on cognitive performance are primarily affected by the timing of cognitive task (during vs. after exercise, and the time delay after exercise). In particular, cognitive performance is more likely to be impaired during high-intensity exercise when both cognitive and physiological demands are high and completed simultaneously (i.e., the dual-task paradigm). The effects may also be affected by the type of cognitive task, physical fitness, exercise mode/duration, and age. Second, we suggest that interactions between changes in regional cerebral blood flow (CBF), cerebral oxygenation, cerebral metabolism, neuromodulation by neurotransmitters/neurotrophic factors, and a variety of psychological factors are promising candidates that determine cognitive performance in response to acute high-intensity exercise. The present review has implications for recreational, sporting, and occupational activities where high cognitive and physiological demands are required to be completed concurrently.
Introduction
A growing body of evidence suggests that acute moderate-intensity exercise improves cognitive performance (Lambourne and Tomporowski, 2010; Chang et al., 2012; Ando et al., 2020; McMorris, 2021). It has been speculated that the relationship between exercise intensity and cognitive performance is inverted-U shaped (Lambourne and Tomporowski, 2010; Chang et al., 2012; McMorris, 2021). In the inverted-U theory, acute exercise gradually increases arousal to an optimal level from rest to moderate intensity and thus improves cognitive performance. A recent review summarized that improvements in cognitive performance following moderate-intensity exercise are frequently accompanied by the changes in brain activation assessed by electroencephalogram (EEG) (Kao et al., 2020), which appears to support the theory that acute exercise alters brain activity and that this is associated with cognitive performance. Acute high-intensity aerobic exercise leads to metabolic, circulatory, and neurohormonal changes at the level of the brain (Ide and Secher, 2000; Meeusen et al., 2001; Nybo and Secher, 2004; Ogoh and Ainslie, 2009; Seifert and Secher, 2011). In contrast to moderate-intensity exercise, theoretically, high-intensity exercise may, therefore, also lead to altered, and potentially impaired cognitive performance. Indeed, the inverted-U theory predicts that high-intensity exercise increases arousal levels beyond the optimal level and leads to a temporary reduction in cognitive performance. However, the current literature base detailing the effects of high-intensity exercise on cognitive performance is not fully supportive of this theory and is somewhat ambiguous and contradictory (Browne et al., 2017; Moreau and Chou, 2019; Cantelon and Giles, 2021; McMorris, 2021; Zheng et al., 2021).
Dietrich and Audiffren (2011) proposed the hypofrontality hypothesis to explain how acute high-intensity exercise affects cognitive performance. The prefrontal cortex (PFC) orchestrates higher-order brain function including cognitive function (Miller and Cohen, 2001; Cools and Arnsten, 2021) and is thought to play a central role in cognitive performance. Acute exercise activates brain regions including motor and sensory cortices, insular cortex, and cerebellum (Williamson et al., 1997; Christensen et al., 2000; Hiura et al., 2014). Hence, the hypofrontality theory speculates that extensive activation of motor and sensory systems during high-intensity exercise likely attenuates higher-order functions of the PFC as the brain has finite metabolic resources (Dietrich and Audiffren, 2011). More recently, McMorris proposed an interoceptive model to explain the effects of high-intensity exercise on cognitive performance (McMorris, 2021). This model offers a more holistic overview of the interaction as it incorporates motivation, perceived effort costs, and perceived availability of resources, together with regional activations and neurotransmitter releases in the brain. Nevertheless, to date, the physiological and psychological mechanism(s) mediating the effects of acute high-intensity exercise on cognitive performance are poorly understood.
In this review, we first summarize the findings of studies investigating the exercise-cognition interaction, especially focusing on high-intensity aerobic exercise. Then, we explore methodological and physiological factors which may alter cognitive performance in response to high-intensity exercise. This review has implications for recreational, sporting, and occupational activities where high cognitive and physiological demands are simultaneously required.
Methodology
A literature search was undertaken using Pubmed to identify studies that examined the effects of high-intensity aerobic exercise on cognitive performance, assessed during and/or after exercise. The reference lists of relevant articles were also searched. The searches were undertaken in February 2022 and relevant articles were obtained. This review focused on healthy adults, and no restrictions were placed on publication date, study design, methodology, or method of assessing cognitive performance. High-intensity aerobic exercise was defined as exercise equating to ≥ 80% maximum power output (Browne et al., 2017), ≥ 80% maximal oxygen uptake () (McMorris, 2016b), or equivalent [e.g., ≥ 80% maximal heart rate (HR)]. Physiological demands are different between continuous and intermittent high-intensity exercise. Thus, we included high-intensity aerobic exercise in this review, and studies incorporating high-intensity interval exercise (HIIE) were considered outside the scope of this review. If specifically interested in this, readers are referred to a recent review that has already explored the effects of HIIE on executive function (Hsieh et al., 2021). In addition, we did not include studies conducted in extreme environments, such as hypoxia and hot/cold environments. However, we referred to evidence from HIIE studies, or studies in extreme environments, for discussion since physiological mechanisms underlying cognitive improvement/impairment in response to HIIE exercise or exercise in extreme environments are, at least partly, shared with those induced by high-intensity aerobic exercise.
Results
Details of the included studies are shown in Table 1, comprising a total of 40 studies (assessed during exercise only, n = 20; assessed both during and after exercise, n = 3; assessed after exercise only, n = 17). In many studies, cognitive performance was impaired during high-intensity exercise (Chmura et al., 1994; McMorris and Keen, 1994; Brisswalter et al., 1997; McMorris et al., 2009; Labelle et al., 2013; Wang et al., 2013; Dutke et al., 2014; Mekari et al., 2015; Schmit et al., 2015; Smith et al., 2016; Gonzalez-Fernandez et al., 2017; Tempest et al., 2017; Komiyama et al., 2020; Stone et al., 2020). In these studies, impairments in both reaction time (RT) and accuracy were frequently observed. Seven studies reported no changes in cognitive performance (Travlos and Marisi, 1995; Fery et al., 1997; Ando et al., 2011; Dutke et al., 2014; Ciria et al., 2019; Tempest and Reiss, 2019; Komiyama et al., 2020). Five studies reported improvement in RT and/or accuracy during high-intensity exercise (McMorris and Graydon, 1997; Huertas et al., 2011; Shields et al., 2011; Davranche et al., 2015; Tempest et al., 2017).
Conversely, cognitive performance after high-intensity exercise is heterogeneous; with improvements (Winter et al., 2007; Luft et al., 2009; Thomson et al., 2009; Griffin et al., 2011; Etnier et al., 2016; Hwang et al., 2016; Hill et al., 2019; Coco et al., 2020a; Loprinzi et al., 2021), impairments (Fery et al., 1997; McMorris et al., 2005; Coco et al., 2009, 2020a; Thomson et al., 2009; Zimmer et al., 2017; Hill et al., 2019), and no changes (Travlos and Marisi, 1995; Brisswalter et al., 1997; Kamijo et al., 2004a,b; Luft et al., 2009; Griffin et al., 2011; Chang et al., 2017; Sudo et al., 2017; Du Rietz et al., 2019; Marin Bosch et al., 2021) reported within the literature. These findings suggest that cognitive performance after high-intensity exercise appears to be dependent on experimental design (see below). In the following sections, we discuss the methodological, physiological, and psychological factors that affect cognitive performance “during” and “after” high-intensity exercise.
Methodological factors
Here we discuss the potential methodological and experimental factors that contribute to the inconsistent findings. These include the following: timing of cognitive task, type of cognitive task, physical fitness, exercise mode/duration, and age.
Timing of cognitive task
When participants perform cognitive tasks during exercise, they perform the exercise and cognitive tasks simultaneously (i.e., a dual-task paradigm). However, when cognitive tasks are performed after exercise, participants only perform a single task. A meta-analysis reported higher effect sizes in single-task conditions (after exercise) when compared with dual-task conditions (during exercise) (Lambourne and Tomporowski, 2010), while another meta-analysis reported that effect sizes were not different between single and dual-task conditions (Chang et al., 2012). Furthermore, McMorris and Hale (2012) undertook statistical analyses and found that there were no differences in effect sizes obtained during compared after exercise. Nevertheless, as recently highlighted (McMorris, 2021), the timing of the cognitive tasks is typically less considered within the literature.
Table 1 indicates that the adverse effects are most prominent during high-intensity exercise. These findings are corroborated by a recent review and suggest that impairments in cognitive performance are more likely to occur during high-intensity exercise (Zheng et al., 2021). Based on the assumption that metabolic resources are limited in the brain, extensive activation in several brain regions (e.g., motor and sensory cortices) may attenuate higher-order functions of the PFC and impair cognitive performance (Dietrich and Audiffren, 2011). Furthermore, in the majority of the included studies, cognitive performance was assessed using manual responses where activations of the motor-related areas are required. Given a limited capacity of the brain to simultaneously activate multiple regions involved in cognitive performance and high-intensity exercise, it is plausible that cognitive performance is more likely to be impaired during high-intensity exercise, particularly when both cognitive and physiological demands are high. Indeed, in four studies reporting cognitive improvement during high-intensity exercise (Huertas et al., 2011; Shields et al., 2011; Davranche et al., 2015; Tempest et al., 2017), exercise intensities were relatively less demanding (i.e., HR < 170 bpm). Relatively lower physiological demands may be responsible for cognitive improvements during high-intensity exercise in these studies. Furthermore, McMorris (2021) argued that performance would depend to a large extent on the perception of task costs (demands) and resources available. These two judgments may be difficult to make in the dual-task situation, leading to over-or under-confidence. This could alter motivation, which would affect cognitive performance.
A recent meta-analysis demonstrated that acute high-intensity exercise had a small, significant facilitating effect on cognitive performance after high-intensity exercise (Moreau and Chou, 2019). In the current review, we observed that cognitive performance after high-intensity exercise is inconsistent: and improvements, impairments, and no changes were reported. EEG studies reported reductions in P3 amplitudes after high intensity (Kamijo et al., 2004b) or HIIE (Kao et al., 2017). On the contrary, Du Rietz and colleagues reported improvements in P3 amplitude and delta power reflecting executive and sustained attention after high-intensity exercise (Du Rietz et al., 2019). These findings suggest that brain activity after exercise may be dependent on the experimental design employed (e.g., exercise intensity, time delay after exercise). Indeed, most physiological changes start to recover immediately after high-intensity exercise (Ide et al., 2000; Gonzalez-Alonso et al., 2004; Curtelin et al., 2017; Sudo et al., 2017). Thus, rapid recovery of physiological variables to homeostatic resting levels may, at least in part, explain the contradictory findings related to cognitive performance after high-intensity exercise. To explore this possibility, we summarized the impacts of the timeframe in which cognitive performance was assessed after exercise (Table 2). When multiple cognitive tasks were examined in a single study, or when both improvement and impairment were reported in a cognitive task (e.g., improvement in RT and impairment in accuracy), all results are reported. We observed heterogeneous findings when cognitive performance was assessed within 5 min of completing high-intensity exercise. Intriguingly, however, no impairments were reported when the timing of cognitive tasks was > 6 min after exercise. These findings support the notion that a rapid recovery to homeostatic resting levels is critical for cognitive performance after high-intensity exercise. Taken collectively, we propose that the effects of high-intensity exercise on cognitive performance are closely related to, and impacted by, the timing of cognitive task (during vs. after exercise, and the time delay after exercise).
Type of cognitive task
Different brain regions are thought to be activated during different cognitive tasks (Macintosh et al., 2014; Chen A. G. et al., 2016; Won et al., 2019). Thus, we can assume that the type of cognitive task is one of the factors that determine how acute high-intensity exercise impacts cognitive performance. This may be particularly relevant when exercise and cognitive tasks are concurrently performed since multiple brain regions are presumably activated. In this review, we classified the type of cognitive task into executive function, psychomotor, memory, and attentional tasks (Table 3). Executive function encompasses several subdomains and consists of basic components of inhibition, working memory, and cognitive flexibility (Diamond, 2013). Thus, based on the included studies, we classified executive function into response inhibition (Go/No-Go task), interference control (Flanker task, Simon task, and Stroop task), working memory (n-back task and spatial delayed response task), and others (soccer-specific task, Wisconsin card sorting task, Cedar Operator Workload Assessment Tool, Tower of London, and Trail making test).
During high-intensity exercise, impairments in cognitive performance were prominent in executive function and psychomotor tasks (Table 3), which is thought to be closely related to dual-task paradigm. Thus, in most cognitive tasks, adverse effects are more likely to occur during high-intensity exercise. Conversely, we found improvements in several studies. In three studies (Huertas et al., 2011; Davranche et al., 2015; Tempest et al., 2017), cognitive improvements were observed in interference control (i.e., Flanker task and Simon task). These findings imply that performances in these cognitive tasks may benefit from high-intensity exercise relative to the other subcomponents of executive function. Furthermore, in the study reporting improvements in soccer-specific cognitive tasks (both soccer-specific and attentional tasks) in college soccer players (McMorris and Graydon, 1997), the cognitive tasks were probably autonomous to the soccer players and therefore less demanding. Hence, cognitive improvements during high-intensity exercise appear to be related to cognitive demands.
A meta-analysis review reported that facilitating effects of performance were similarly observed in subcomponents of executive function (i.e., working memory, inhibitory control, cognitive flexibility, and attention) after high-intensity exercise (Moreau and Chou, 2019). In the present review, we failed to find clear associations between the type of cognitive task and cognitive performance after high-intensity exercise. It should be noted, however, that improvements in memory performance were often observed after high-intensity exercise (Winter et al., 2007; Griffin et al., 2011; Etnier et al., 2016; Loprinzi et al., 2021). In particular, high-intensity exercise may be beneficial for retention (Winter et al., 2007; Etnier et al., 2016; Loprinzi et al., 2021). Additional studies are necessary to further elucidate potential physiological mechanisms underlying the improvement. In this review, we may not have been sufficiently powered to identify the effects of different cognitive tasks/domains. Further research is required to establish the effects of high-intensity exercise on a variety of cognitive tasks and domains.
Physical fitness level of participants
It has been previously speculated that exercise-cognition interaction is influenced by physical fitness (Lambourne and Tomporowski, 2010; Chang et al., 2012). For example, despite matched relative exercise intensity, individuals with lower physical fitness levels were more susceptible to cognitive impairments during high-intensity exercise, when compared with those who had higher aerobic capacities (Brisswalter et al., 1997; Labelle et al., 2013). Furthermore, choice RT performance gradually improves during incremental exercise until at ∼75% max in young soccer players (Chmura et al., 1994). Aerobic capacity has been suggested to be one of the moderators that affect cognitive performance in response to high-intensity exercise (Browne et al., 2017). McMorris (2021) claimed that fitness levels would affect the individual’s perception of effort costs and, hence, their motivation level. Further, well-powered studies are also necessary to clarify the relationship between cognitive performance and physical fitness before this theory can be confirmed.
Exercise mode
A previous meta-analysis suggested that exercise mode is one of the factors that affect exercise-cognition interaction (Lambourne and Tomporowski, 2010). We summarized the impacts of high-intensity cycling and running in Table 4. The effects of high-intensity exercise on cognitive performance during cycling were inconsistent, but reductions in cognitive performance appeared to be more likely during cycling. Running is kinematically less stable compared to cycling, and thus it may be difficult to complete cognitive performance during high-intensity running. Indeed, only two studies examined cognitive performance during high-intensity running, and both reported impairments in cognitive performance (Smith et al., 2016; Stone et al., 2020). Given the nature of the dual-task paradigm, running may be more detrimental to cognitive performance relative to cycling during high-intensity exercise.
After high-intensity cycling, in most cases, we observed no changes or impairments in cognitive performance. Conversely, after high-intensity running, improvements or no changes in cognitive performance were predominant. Since participants perform only a cognitive task after exercise (i.e., single task) regardless of exercise mode, the findings are somewhat unexpected. There are several physiological differences in ventilatory and metabolic responses, HR, and motor unit recruitment between cycling and running (Millet et al., 2009). Hence, the recovery of physiological variables to resting levels could be different between high-intensity cycling and running. Collectively, these findings suggest that the mode of exercise is one of the factors that affect cognitive performance, and these effects are presumably influenced by the timing of cognitive task (during vs. after exercise, and the time delay after exercise). Future studies are required to understand how exercise mode influences cognitive performance after high-intensity exercise.
Exercise duration
Exercise duration also potentially affects cognitive performance in response to high-intensity exercise. We summarized the relationship between exercise duration and cognitive performance in Table 5. During exercise, cognitive impairments were observed when exercise duration was < 10 min. The findings were inconsistent when exercise duration was > 11 min or exhaustive exercise. Thus, it is less likely that exercise duration per se affects cognitive performance. Rather, interactions between exercise duration and intensity would be more important for cognitive performance during high-intensity exercise. In most studies that assessed cognitive performance after high-intensity exercise, exercise was continued until exhaustion (Table 5). Nevertheless, the results were heterogeneous and suggest that exercise duration in isolation is not critical for cognitive performance after exhaustive exercise. Assuming that the duration of high-intensity exercise is likely limited, exercise duration alone is, therefore, not likely to determine cognitive performance.
Age
Coco and colleagues compared the effects of exhaustive exercise on the cognitive performance of young and older adults (Coco et al., 2020a). They reported impairments in the performance of simple RT and the Stroop color-word test after exhaustive exercise in both groups. However, improvements in trail making test were observed only in the younger group. These results suggest that the effects of high-intensity exercise on cognitive performance may be different in young and older adults. Cerebral perfusion appears to be lower in older individuals during high-intensity exercise, although the cerebral extraction of glucose, lactate, and oxygen is similar (Braz and Fisher, 2016). Thus, age may be one factor that may influence cognitive performance and reduced cerebral perfusion could affect cognitive performance in older individuals in particular. Given the brevity of studies in this area, definitive conclusion on the impact of age is not yet feasible.
Physiological factors
As noted above, high-intensity exercise induces a variety of physiological effects within the human brain. Here, we summarize and discuss the physiological factors that are linked to cognitive performance during and after high-intensity exercise and we identify some of the potential physiological factors that may contribute to the inconsistent findings. These include the separate and combined effects of cerebral blood flow (CBF), cerebral oxygenation, cerebral metabolism, neuromodulation by neurotransmitters and neurotrophic factors, and various psychological factors.
Cerebral blood flow
During exercise, CBF is regulated by complex interactions between neural activity and metabolism, partial pressure of oxygen, carbon dioxide (CO2), blood pressure, cardiac output, and sympathetic nervous system activity (Ogoh and Ainslie, 2009; Smith and Ainslie, 2017). CBF gradually increases during mild- to moderate-intensity exercise in response to neural activity and metabolism (Ogoh and Ainslie, 2009). However, during high-intensity exercise, hyperventilation-induced hypocapnia constricts the cerebral vessels, thereby reducing CBF (Ogoh and Ainslie, 2009; Smith and Ainslie, 2017). This suggests that brain metabolic demands might be inadequate during high-intensity exercise. Ogoh et al. (2014) reported that an increase in CBF, achieved using CO2 inhalation, did not affect cognitive performance during prolonged moderate-intensity exercise (Ogoh et al., 2014). More recently, Komiyama and colleagues tested the hypothesis that a reduction in CBF is directly linked to impairment in cognitive performance during high-intensity exercise (Komiyama et al., 2020). By restoring CBF via CO2 inhalation, the authors demonstrated that middle cerebral artery (MCA) velocity (a surrogate for CBF) did not prevent impaired cognitive performance during high-intensity exercise. These results suggest that a reduction in CBF per se may not be responsible for impaired cognitive performance during high-intensity exercise. However, given that CBF supplies oxygen and nutrients, the association between cognitive performance and regional CBF (e.g., blood flow to the PFC) in response to high-intensity exercise should be further investigated. In particular, a recent study indicated physiological “uncoupling” between the PFC oxygenation and MCA velocity during high-intensity exercise with CO2 inhalation (Hansen et al., 2020). Follow-up studies are needed to fully understand the association between regional CBF and cognitive performance in response to high-intensity exercise.
Cerebral oxygenation
Cerebral oxygenation reflects the balance between cerebral oxygen availability and utilization (Boushel et al., 2001; Komiyama et al., 2017), which is generally measured from the PFC. Cerebral oxygenation reduces during high-intensity exercise close to maximal intensity (Rooks et al., 2010). Some studies suggest that a reduction in cerebral oxygenation is not associated with impairments in cognitive performance during high-intensity exercise (Ando et al., 2011; Schmit et al., 2015; Tempest et al., 2017). In contrast, others have indicated that impairments in cognitive performance were accompanied by reduction in cerebral oxygenation during high-intensity exercise (Mekari et al., 2015; Stone et al., 2020). The latter studies suggest that impairments in cognitive performance may be associated with attenuated PFC oxygenation. However, several studies have shown that cognitive performance improved during acute moderate-intensity exercise in hypoxia despite substantial reductions in cerebral oxygenation (Ando et al., 2013; Komiyama et al., 2015, 2017). Hence, it is likely that a reduction in cerebral oxygenation, in isolation, does not result in impaired cognitive performance. However, reduction in cerebral oxygenation during high-intensity exercise may impair cognitive performance in concert with other physiological factors.
Cerebral oxygenation starts to recover immediately after maximal exercise (Gonzalez-Alonso et al., 2004; Sudo et al., 2017). Notably, the degree of recovery of cerebral oxygenation following maximal exercise may be associated with cognitive performance (Sudo et al., 2017). This finding suggests that the recovery of cerebral oxygenation after high-intensity exercise may, at least in part, account for the differential effects of high-intensity exercise on cognitive performance between single (i.e., after) and dual (i.e., during) conditions.
Cerebral metabolism
It is generally accepted that blood glucose is the primary energy source for the brain at rest (Gold, 1995). Komiyama et al. (2016) reported that cognitive performance improves during moderate-intensity exercise after skipping breakfast. This suggests that substrates other than glucose may compensate for the reduced availability of blood glucose during moderate-intensity exercise. It is plausible that the same would be true for high-intensity exercise. Indeed, blood glucose uptake is thought to be reduced in the brain during high-intensity exercise (Kemppainen et al., 2005). In contrast, blood lactate substantially increases during/after high-intensity exercise, and it is taken up by the brain (Ide et al., 2000; Gonzalez-Alonso et al., 2004; Quistorff et al., 2008; Siebenmann et al., 2021). Several studies suggested that blood lactate would provide energy that contributes to improvements in cognitive performance following HIIE (Tsukamoto et al., 2016; Hashimoto et al., 2018; Herold et al., 2022). In particular, Hashimoto et al. (2018) directly measured lactate uptake in the brain after HIIE and suggested that lactate production in extra-cerebral tissues supports brain function. On the contrary, Coco et al. (2020b) suggested that high levels of blood lactate have detrimental effects on cognitive performance. Interestingly, Coco et al. (2009) indicated that intravenous lactate infusion of a lactate solution impaired attentional performance. Hence, further studies are warranted to investigate how blood lactate acts as a mediator of exercise-induced alterations in cognitive performance (Ando et al., 2022).
Neuromodulation by neurotransmitters and neurotrophic factors
In humans, it is less clear how acute exercise alters central neurotransmitter release due to technical and methodological challenges. Nevertheless, given that rodent studies indicate that acute exercise releases neurotransmitters in the brain (Meeusen et al., 2001; Hasegawa et al., 2011; Goekint et al., 2012; Chen C. et al., 2016), acute exercise is likely to influence brain circuits involving a number of neurotransmitters including dopamine and noradrenaline (McMorris, 2016a; Ando et al., 2020). Dopamine and noradrenaline modulate the strength of the PFC network connections, and regulation of dopamine and noradrenaline is required for appropriate prefrontal cognitive function (Arnsten, 2011). Furthermore, excess noradrenaline and dopamine appear to weaken the signal-to-noise ratio, which may result in impairments in the PFC function (Arnsten, 2011; Cools and Arnsten, 2021). Hence, the available literature suggests that excess neuromodulators in the brain may have adverse effects on cognitive performance during/after high-intensity exercise. High-intensity exercise also seems to increase brain-derived neurotrophic factors (BDNFs) (Ferris et al., 2007; Winter et al., 2007; Fernandez-Rodriguez et al., 2021) and insulin-like growth hormone factor-1 (IGF-1) (Sudo et al., 2017). Several studies have implicated that changes in BDNF are associated with cognitive improvement induced by acute exercise (Winter et al., 2007; Lee et al., 2014; Skriver et al., 2014; Hwang et al., 2016). However, BDNF and IGF-1 are known to play a crucial role in angiogenesis, synaptogenesis, and neurogenesis following long-term exercise (Cotman and Berchtold, 2002; Voss et al., 2011; Nieto-Estevez et al., 2016). High concentrations of dopamine, noradrenaline, and BDNF are necessary for long-term potentiation, which is essential for long-term memory (McMorris, 2021). A couple of studies have shown positive effects of high-intensity exercise on long-term memory (Winter et al., 2007; Griffin et al., 2011). At present, it is premature to conclude that changes in BDNF and IGF-1 play a role in cognitive performance during/after high-intensity exercise.
Psychological factors
In most studies, psychological factors are typically not well considered when attempting to elucidate the effects of high intensity on cognitive performance. However, as suggested by McMorris (2021), psychological factors such as the motivation and perception of effort may affect the acute exercise–catecholamine–cognition interaction. Cantelon and Giles also suggested that psychological factors are moderating factors that affect exercise–cognition interaction (Cantelon and Giles, 2021). At present, and given the lack of empirical evidence, further investigations are needed to investigate the association between psychological factors and cognitive performance during and after high-intensity exercise.
Integration of physiological and psychological factors
It is likely that the effects of high-intensity exercise on cognitive performance are multifactorial and determined by the integration of several physiological and psychological factors (Figure 1). We propose that interactions of these factors influence neural activity associated with cognitive performance and that this determines cognitive performance during and after high-intensity exercise. This is consistent with McMorris (2021) claiming that the perception of physiological stress affects the motivation and perception of effort costs. However, the current literature base is insufficient to substantiate this speculation and this should be the focus of future research in this area. A recent fNIRS study detected cognitive task-related hemodynamic changes from the left PFC during high-intensity exercise (Tempest and Reiss, 2019). Furthermore, an fMRI study indicated that HIIE decreased brain activation associated with cognitive performance (Mehren et al., 2019). Thus, future studies using sophisticated neuroimaging methods (e.g., fNIRS, fMRI, and PET) are required to fully understand how a single bout of high-intensity exercise affects cognitive performance.
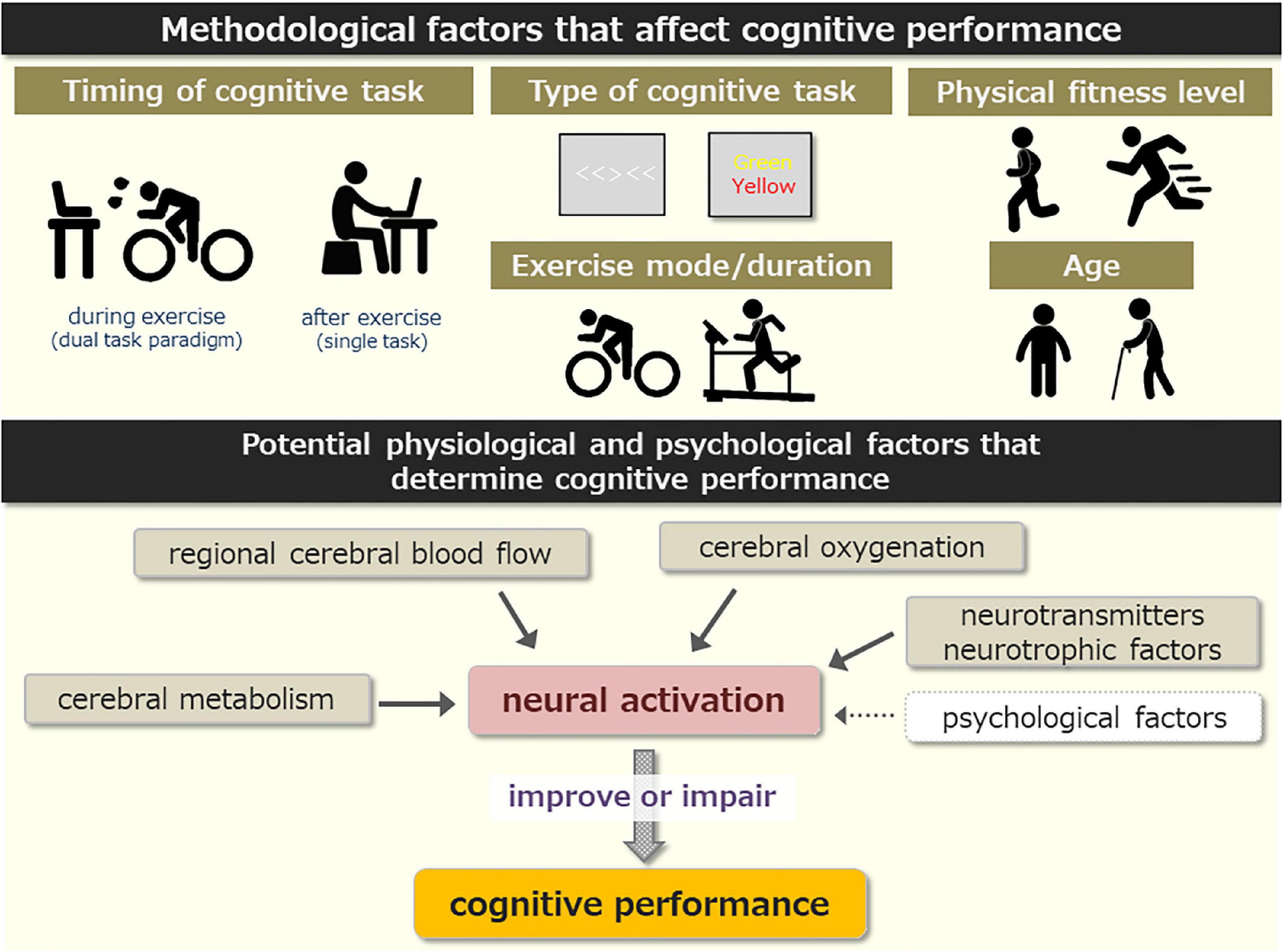
Figure 1. (Upper) Summary of methodological factors that affect cognitive performance in response to high-intensity exercise. (Lower) Potential physiological and psychological factors that mediate cognitive performance.
Conclusion
This narrative review summarized the literature examining the effects of acute high-intensity exercise on cognitive performance. We propose that the effects of high-intensity exercise on cognitive performance are primarily affected by a variety of methodological, physiological, and psychological factors. Specifically, these include the timing of cognitive task (during vs. after exercise, and the time delay after exercise), cognitive task(s), fitness level, exercise mode/duration, and age. It is also likely that a complex interaction between changes in regional CBF, cerebral oxygenation, cerebral metabolism, neurotransmitters/neurotrophic factors, and a variety of psychological factors contributes to the heterogeneous findings reported. The review is likely to have implications for recreational, sporting, and occupational activities where high cognitive and physiological demands are required simultaneously.
Author contributions
MS, JC, TM, and SA drafted the manuscript. All authors approved the final version of the manuscript.
Conflict of interest
The authors declare that the research was conducted in the absence of any commercial or financial relationships that could be construed as a potential conflict of interest.
Publisher’s note
All claims expressed in this article are solely those of the authors and do not necessarily represent those of their affiliated organizations, or those of the publisher, the editors and the reviewers. Any product that may be evaluated in this article, or claim that may be made by its manufacturer, is not guaranteed or endorsed by the publisher.
References
Ando, S., Hatamoto, Y., Sudo, M., Kiyonaga, A., Tanaka, H., and Higaki, Y. (2013). The effects of exercise under hypoxia on cognitive function. PLoS One 8:e63630. doi: 10.1371/journal.pone.0063630
Ando, S., Kokubu, M., Yamada, Y., and Kimura, M. (2011). Does cerebral oxygenation affect cognitive function during exercise? Eur. J. Appl. Physiol. 111, 1973–1982.
Ando, S., Komiyama, T., Sudo, M., Higaki, Y., Ishida, K., Costello, J. T., et al. (2020). The interactive effects of acute exercise and hypoxia on cognitive performance: a narrative review. Scand. J. Med. Sci. Sports 30, 384–398. doi: 10.1111/sms.13573
Ando, S., Komiyama, T., Tanoue, Y., Sudo, M., Costello, J. T., Uehara, Y., et al. (2022). Cognitive improvement after aerobic and resistance exercise is not associated with peripheral biomarkers. Front. Behav. Neurosci. 16:853150. doi: 10.3389/fnbeh.2022.853150
Arnsten, A. F. (2011). Catecholamine influences on dorsolateral prefrontal cortical networks. Biol. Psychiatry 69, e89–e99.
Boushel, R., Langberg, H., Olesen, J., Gonzales-Alonzo, J., Bulow, J., and Kjaer, M. (2001). Monitoring tissue oxygen availability with near infrared spectroscopy (NIRS) in health and disease. Scand. J. Med. Sci. Sports 11, 213–222.
Braz, I. D., and Fisher, J. P. (2016). The impact of age on cerebral perfusion, oxygenation and metabolism during exercise in humans. J. Physiol. 594, 4471–4483.
Brisswalter, J., Arcelin, R., Audiffren, M., and Delignieres, D. (1997). Influence of physical exercise on simple reaction time: Effect of physical fitness. Percept Mot. Skills 85, 1019–1027.
Browne, S. E., Flynn, M. J., O’neill, B. V., Howatson, G., Bell, P. G., and Haskell-Ramsay, C. F. (2017). Effects of acute high-intensity exercise on cognitive performance in trained individuals: a systematic review. Prog. Brain Res. 234, 161–187.
Cantelon, J. A., and Giles, G. E. (2021). A review of cognitive changes during acute aerobic exercise. Front. Psychol. 12:653158. doi: 10.3389/fpsyg.2021.653158
Chang, H., Kim, K., Jung, Y. J., and Kato, M. (2017). Effects of acute high-intensity resistance exercise on cognitive function and oxygenation in prefrontal cortex. J. Exerc. Nutr. Biochem. 21, 1–8. doi: 10.20463/jenb.2017.0012
Chang, Y. K., Labban, J. D., Gapin, J. I., and Etnier, J. L. (2012). The effects of acute exercise on cognitive performance: a meta-analysis. Brain Res 1453, 87–101.
Chen, A. G., Zhu, L. N., Yan, J., and Yin, H. C. (2016). Neural basis of working memory enhancement after acute aerobic exercise: fMRI study of preadolescent children. Front. Psychol. 7:1804. doi: 10.1016/j.ijpsycho.2020.09.018
Chen, C., Nakagawa, S., Kitaichi, Y., An, Y., Omiya, Y., Song, N., et al. (2016). The role of medial prefrontal corticosterone and dopamine in the antidepressant-like effect of exercise. Psychoneuroendocrinology 69, 1–9. doi: 10.1016/j.psyneuen.2016.03.008
Chmura, J., Nazar, K., and Kaciuba-Uscilko, H. (1994). Choice reaction time during graded exercise in relation to blood lactate and plasma catecholamine thresholds. Int. J. Sports Med. 15, 172–176. doi: 10.1055/s-2007-1021042
Christensen, L. O. D., Johannsen, P., Sinkjaer, T., Petersen, N., Pyndt, H. S., and Nielsen, J. B. (2000). Cerebral activation during bicycle movements in man. Exp. Brain Res. 135, 66–72.
Ciria, L. F., Luque-Casado, A., Sanabria, D., Holgado, D., Ivanov, P. C., and Perakakis, P. (2019). Oscillatory brain activity during acute exercise: Tonic and transient neural response to an oddball task. Psychophysiology 56:e13326. doi: 10.1111/psyp.13326
Coco, M., Buscemi, A., Guerrera, C. S., Di Corrado, D., Cavallari, P., Zappala, A., et al. (2020a). Effects of a bout of intense exercise on some executive functions. Int. J. Environ. Res. Public Health 17:898. doi: 10.3390/ijerph17030898
Coco, M., Buscemi, A., Ramaci, T., Tusak, M., Corrado, D. D., Perciavalle, V., et al. (2020b). Influences of blood lactate levels on cognitive domains and physical health during a sports stress. Brief review. Int. J. Environ. Res. Public Health 17:9043. doi: 10.3390/ijerph17239043
Coco, M., Di Corrado, D., Calogero, R. A., Perciavalle, V., Maci, T., and Perciavalle, V. (2009). Attentional processes and blood lactate levels. Brain Res. 1302, 205–211.
Cools, R., and Arnsten, A. F. T. (2021). Neuromodulation of prefrontal cortex cognitive function in primates: THE powerful roles of monoamines and acetylcholine. Neuropsychopharmacology 47, 309–328. doi: 10.1038/s41386-021-01100-8
Cotman, C. W., and Berchtold, N. C. (2002). Exercise: a behavioral intervention to enhance brain health and plasticity. Trends Neurosci. 25, 295–301. doi: 10.1016/s0166-2236(02)02143-4
Curtelin, D., Morales-Alamo, D., Torres-Peralta, R., Rasmussen, P., Martin-Rincon, M., Perez-Valera, M., et al. (2017). Cerebral blood flow, frontal lobe oxygenation and intra-arterial blood pressure during sprint exercise in normoxia and severe acute hypoxia in humans. J. Cereb. Blood Flow Metab. 38, 136–150. doi: 10.1177/0271678X17691986
Davranche, K., Brisswalter, J., and Radel, R. (2015). Where are the limits of the effects of exercise intensity on cognitive control? J. Sport Health Sci. 4, 56–63.
Dietrich, A., and Audiffren, M. (2011). The reticular-activating hypofrontality (RAH) model of acute exercise. Neurosci. Biobehav. Rev. 35, 1305–1325. doi: 10.1016/j.neubiorev.2011.02.001
Du Rietz, E., Barker, A. R., Michelini, G., Rommel, A. S., Vainieri, I., Asherson, P., et al. (2019). Beneficial effects of acute high-intensity exercise on electrophysiological indices of attention processes in young adult men. Behav. Brain Res. 359, 474–484. doi: 10.1016/j.bbr.2018.11.024
Dutke, S., Jaitner, T., Berse, T., and Barenberg, J. (2014). Acute physical exercise affected processing efficiency in an auditory attention task more than processing effectiveness. J. Sport Exerc. Psychol. 36, 69–79. doi: 10.1123/jsep.2013-0044
Etnier, J. L., Wideman, L., Labban, J. D., Piepmeier, A. T., Pendleton, D. M., Dvorak, K. K., et al. (2016). The effects of acute exercise on memory and brain-derived neurotrophic factor (BDNF). J. Sport Exerc. Psychol. 38, 331–340.
Fernandez-Rodriguez, R., Alvarez-Bueno, C., Martinez-Ortega, I. A., Martinez-Vizcaino, V., Mesas, A. E., and Notario-Pacheco, B. (2021). Immediate effect of high-intensity exercise on brain-derived neurotrophic factor in healthy young adults: a systematic review and meta-analysis. J. Sport Health Sci. 11, 367–375. doi: 10.1016/j.jshs.2021.08.004
Ferris, L. T., Williams, J. S., and Shen, C. L. (2007). The effect of acute exercise on serum brain-derived neurotrophic factor levels and cognitive function. Med. Sci. Sports Exerc. 39, 728–734.
Fery, Y. A., Ferry, A., Vom Hofe, A., and Rieu, M. (1997). Effect of physical exhaustion on cognitive functioning. Percept Mot. Skills 84, 291–298.
Goekint, M., Bos, I., Heyman, E., Meeusen, R., Michotte, Y., and Sarre, S. (2012). Acute running stimulates hippocampal dopaminergic neurotransmission in rats, but has no influence on brain-derived neurotrophic factor. J. Appl. Physiol (1985) 112, 535–541. doi: 10.1152/japplphysiol.00306.2011
Gold, P. E. (1995). Role of glucose in regulating the brain and cognition. Am. J. Clin. Nutr. 61, 987S–995S.
Gonzalez-Alonso, J., Dalsgaard, M. K., Osada, T., Volianitis, S., Dawson, E. A., Yoshiga, C. C., et al. (2004). Brain and central haemodynamics and oxygenation during maximal exercise in humans. J. Physiol. 557, 331–342.
Gonzalez-Fernandez, F., Etnier, J. L., Zabala, M., and Sanabria, D. (2017). Vigilance performance during acute exercise. Inter. J. Sport Psychol. 48, 435–447.
Griffin, E. W., Mullally, S., Foley, C., Warmington, S. A., O’mara, S. M., and Kelly, A. M. (2011). Aerobic exercise improves hippocampal function and increases BDNF in the serum of young adult males. Physiol. Behav. 104, 934–941.
Hansen, R. K., Nielsen, P. S., Schelske, M. W., Secher, N. H., and Volianitis, S. (2020). CO2 supplementation dissociates cerebral oxygenation and middle cerebral artery blood velocity during maximal cycling. Scand. J. Med. Sci. Sports 30, 399–407. doi: 10.1111/sms.13582
Hasegawa, H., Takatsu, S., Ishiwata, T., Tanaka, H., Sarre, S., and Meeusen, R. (2011). Continuous monitoring of hypothalamic neurotransmitters and thermoregulatory responses in exercising rats. J. Neurosci. Methods 202, 119–123. doi: 10.1016/j.jneumeth.2011.05.024
Hashimoto, T., Tsukamoto, H., Takenaka, S., Olesen, N. D., Petersen, L. G., Sorensen, H., et al. (2018). Maintained exercise-enhanced brain executive function related to cerebral lactate metabolism in men. FASEB J. 32, 1417–1427. doi: 10.1096/fj.201700381RR
Herold, F., Behrendt, T., Meißner, C., Müller, N., and Schega, L. (2022). The influence of acute sprint interval training on cognitive performance of healthy younger adults. Int. J. Environ. Res. Public Health 19:613. doi: 10.3390/ijerph19010613
Hill, M., Walsh, S., Talbot, C., Price, M., and Duncan, M. (2019). Exercise intensity-dependent effects of arm and leg-cycling on cognitive performance. PLoS One 14:e0224092. doi: 10.1371/journal.pone.0224092
Hiura, M., Nariai, T., Ishii, K., Sakata, M., Oda, K., Toyohara, J., et al. (2014). Changes in cerebral blood flow during steady-state cycling exercise: a study using oxygen-15-labeled water with PET. J. Cereb. Blood Flow Metab. 34, 389–396. doi: 10.1038/jcbfm.2013.220
Hsieh, S. S., Chueh, T. Y., Huang, C. J., Kao, S. C., Hillman, C. H., Chang, Y. K., et al. (2021). Systematic review of the acute and chronic effects of high-intensity interval training on executive function across the lifespan. J. Sports Sci. 39, 10–22. doi: 10.1080/02640414.2020.1803630
Huertas, F., Zahonero, J., Sanabria, D., and Lupianez, J. (2011). Functioning of the attentional networks at rest vs. During acute bouts of aerobic exercise. J. Sport Exerc. Psychol. 33, 649–665. doi: 10.1123/jsep.33.5.649
Hwang, J., Brothers, R. M., Castelli, D. M., Glowacki, E. M., Chen, Y. T., Salinas, M. M., et al. (2016). Acute high-intensity exercise-induced cognitive enhancement and brain-derived neurotrophic factor in young, healthy adults. Neurosci. Lett. 630, 247–253.
Ide, K., and Secher, N. H. (2000). Cerebral blood flow and metabolism during exercise. Prog. Neurobiol. 61, 397–414.
Ide, K., Schmalbruch, I. K., Quistorff, B., Horn, A., and Secher, N. H. (2000). Lactate, glucose and O2 uptake in human brain during recovery from maximal exercise. J. Physiol. 522(Pt 1), 159–164.
Kamijo, K., Nishihira, Y., Hatta, A., Kaneda, T., Kida, T., Higashiura, T., et al. (2004a). Changes in arousal level by differential exercise intensity. Clin. Neurophysiol. 115, 2693–2698.
Kamijo, K., Nishihira, Y., Hatta, A., Kaneda, T., Wasaka, T., Kida, T., et al. (2004b). Differential influences of exercise intensity on information processing in the central nervous system. Eur. J. Appl. Physiol. 92, 305–311. doi: 10.1007/s00421-004-1097-2
Kao, S. C., Cadenas-Sanchez, C., Shigeta, T. T., Walk, A. M., Chang, Y. K., Pontifex, M. B., et al. (2020). A systematic review of physical activity and cardiorespiratory fitness on P3b. Psychophysiology 57:e13425. doi: 10.1111/psyp.13425
Kao, S. C., Westfall, D. R., Soneson, J., Gurd, B., and Hillman, C. H. (2017). Comparison of the acute effects of high-intensity interval training and continuous aerobic walking on inhibitory control. Psychophysiology 54, 1335–1345. doi: 10.1111/psyp.12889
Kemppainen, J., Aalto, S., Fujimoto, T., Kalliokoski, K. K., Langsjo, J., Oikonen, V., et al. (2005). High intensity exercise decreases global brain glucose uptake in humans. J. Physiol. 568, 323–332. doi: 10.1113/jphysiol.2005.091355
Komiyama, T., Katayama, K., Sudo, M., Ishida, K., Higaki, Y., and Ando, S. (2017). Cognitive function during exercise under severe hypoxia. Sci. Rep. 7:10000.
Komiyama, T., Sudo, M., Higaki, Y., Kiyonaga, A., Tanaka, H., and Ando, S. (2015). Does moderate hypoxia alter working memory and executive function during prolonged exercise? Physiol. Behav. 139, 290–296. doi: 10.1016/j.physbeh.2014.11.057
Komiyama, T., Sudo, M., Okuda, N., Yasuno, T., Kiyonaga, A., Tanaka, H., et al. (2016). Cognitive function at rest and during exercise following breakfast omission. Physiol. Behav. 157, 178–184. doi: 10.1016/j.physbeh.2016.02.013
Komiyama, T., Tanoue, Y., Sudo, M., Costello, J. T., Uehara, Y., Higaki, Y., et al. (2020). Cognitive impairment during high-intensity exercise: Influence of cerebral blood flow. Med. Sci. Sports Exerc. 52, 561–568. doi: 10.1249/MSS.0000000000002183
Labelle, V., Bosquet, L., Mekary, S., and Bherer, L. (2013). Decline in executive control during acute bouts of exercise as a function of exercise intensity and fitness level. Brain Cogn. 81, 10–17. doi: 10.1016/j.bandc.2012.10.001
Lambourne, K., and Tomporowski, P. (2010). The effect of exercise-induced arousal on cognitive task performance: a meta-regression analysis. Brain Res. 1341, 12–24. doi: 10.1016/j.brainres.2010.03.091
Lee, J. K., Koh, A. C., Koh, S. X., Liu, G. J., Nio, A. Q., and Fan, P. W. (2014). Neck cooling and cognitive performance following exercise-induced hyperthermia. Eur. J. Appl. Physiol. 114, 375–384. doi: 10.1007/s00421-013-2774-9
Loprinzi, P. D., Day, S., Hendry, R., Hoffman, S., Love, A., Marable, S., et al. (2021). The effects of acute exercise on short- and long-term memory: considerations for the timing of exercise and phases of memory. Eur. J. Psychol. 17, 85–103. doi: 10.5964/ejop.2955
Luft, C. D., Takase, E., and Darby, D. (2009). Heart rate variability and cognitive function: effects of physical effort. Biol. Psychol. 82, 164–168.
Macintosh, B. J., Crane, D. E., Sage, M. D., Rajab, A. S., Donahue, M. J., Mcilroy, W. E., et al. (2014). Impact of a single bout of aerobic exercise on regional brain perfusion and activation responses in healthy young adults. PLoS One 9:e85163. doi: 10.1371/journal.pone.0085163
Marin Bosch, B., Bringard, A., Logrieco, M. G., Lauer, E., Imobersteg, N., Thomas, A., et al. (2021). A single session of moderate intensity exercise influences memory, endocannabinoids and brain derived neurotrophic factor levels in men. Sci. Rep. 11:14371. doi: 10.1038/s41598-021-93813-5
McMorris, T. (2016b). “History of research into the acute exercise–cognition interaction,” in Exercise-Cognition Interaction: Neuroscience Perspective, ed. T. Mcmorris (Amsterdam: Elsevier).
McMorris, T. (2016a). Developing the catecholamines hypothesis for the acute exercise-cognition interaction in humans: Lessons from animal studies. Physiol. Behav. 165, 291–299. doi: 10.1016/j.physbeh.2016.08.011
McMorris, T. (2021). The acute exercise-cognition interaction: From the catecholamines hypothesis to an interoception model. Int. J. Psychophysiol. 170, 75–88. doi: 10.1016/j.ijpsycho.2021.10.005
McMorris, T., and Graydon, J. (1997). The effect of exercise on cognitive performance in soccer-specific tests. J. Sports Sci. 15, 459–468.
McMorris, T., and Hale, B. J. (2012). Differential effects of differing intensities of acute exercise on speed and accuracy of cognition: a meta-analytical investigation. Brain Cogn. 80, 338–351. doi: 10.1016/j.bandc.2012.09.001
McMorris, T., and Keen, P. (1994). Effect of exercise on simple reaction times of recreational athletes. Percept Mot. Skills 78, 123–130.
McMorris, T., Davranche, K., Jones, G., Hall, B., Corbett, J., and Minter, C. (2009). Acute incremental exercise, performance of a central executive task, and sympathoadrenal system and hypothalamic-pituitary-adrenal axis activity. Int. J. Psychophysiol. 73, 334–340. doi: 10.1016/j.ijpsycho.2009.05.004
McMorris, T., Delves, S., Sproule, J., Lauder, M., and Hale, B. (2005). Effect of incremental exercise on initiation and movement times in a choice response, whole body psychomotor task. Br. J. Sports Med. 39, 537–541. doi: 10.1136/bjsm.2004.014456
Meeusen, R., Piacentini, M. F., and De Meirleir, K. (2001). Brain microdialysis in exercise research. Sports Med. 31, 965–983.
Mehren, A., Diaz Luque, C., Brandes, M., Lam, A. P., Thiel, C. M., Philipsen, A., et al. (2019). Intensity-dependent effects of acute exercise on executive function. Neural Plast. 2019:8608317.
Mekari, S., Fraser, S., Bosquet, L., Bonnery, C., Labelle, V., Pouliot, P., et al. (2015). The relationship between exercise intensity, cerebral oxygenation and cognitive performance in young adults. Eur. J. Appl. Physiol. 115, 2189–2197.
Miller, E. K., and Cohen, J. D. (2001). An integrative theory of prefrontal cortex function. Annu. Rev. Neurosci. 24, 167–202.
Millet, G. P., Vleck, V. E., and Bentley, D. J. (2009). Physiological differences between cycling and running: lessons from triathletes. Sports Med. 39, 179–206. doi: 10.2165/00007256-200939030-00002
Moreau, D., and Chou, E. (2019). The acute effect of high-intensity exercise on executive function: a meta-analysis. Perspect Psychol. Sci. 14, 734–764.
Nieto-Estevez, V., Defterali, C., and Vicario-Abejon, C. (2016). IGF-I: A key growth factor that regulates neurogenesis and synaptogenesis from embryonic to adult stages of the brain. Front. Neurosci. 10:52. doi: 10.3389/fnins.2016.00052
Nybo, L., and Secher, N. H. (2004). Cerebral perturbations provoked by prolonged exercise. Prog. Neurobiol. 72, 223–261.
Ogoh, S., and Ainslie, P. N. (2009). Cerebral blood flow during exercise: Mechanisms of regulation. J. Appl. Physiol. 107, 1370–1380.
Ogoh, S., Tsukamoto, H., Hirasawa, A., Hasegawa, H., Hirose, N., and Hashimoto, T. (2014). The effect of changes in cerebral blood flow on cognitive function during exercise. Physiol. Rep. 2:e12163.
Quistorff, B., Secher, N. H., and Van Lieshout, J. J. (2008). Lactate fuels the human brain during exercise. FASEB J. 22, 3443–3449.
Rooks, C. R., Thom, N. J., Mccully, K. K., and Dishman, R. K. (2010). Effects of incremental exercise on cerebral oxygenation measured by near-infrared spectroscopy: a systematic review. Prog. Neurobiol. 92, 134–150. doi: 10.1016/j.pneurobio.2010.06.002
Schmit, C., Davranche, K., Easthope, C. S., Colson, S. S., Brisswalter, J., and Radel, R. (2015). Pushing to the limits: The dynamics of cognitive control during exhausting exercise. Neuropsychologia 68, 71–81. doi: 10.1016/j.neuropsychologia.2015.01.006
Seifert, T., and Secher, N. H. (2011). Sympathetic influence on cerebral blood flow and metabolism during exercise in humans. Prog. Neurobiol. 95, 406–426.
Shields, M. R., Larson, C. L., Swartz, A. M., and Smith, J. C. (2011). Visual threat detection during moderate- and high-intensity exercise. Emotion 11, 572–581. doi: 10.1037/a0021251
Siebenmann, C., Sorensen, H., Bonne, T. C., Zaar, M., Aachmann-Andersen, N. J., Nordsborg, N. B., et al. (2021). Cerebral lactate uptake during exercise is driven by the increased arterial lactate concentration. J. Appl. Physiol. 131, 1824–1830. doi: 10.1152/japplphysiol.00505.2021
Skriver, K., Roig, M., Lundbye-Jensen, J., Pingel, J., Helge, J. W., Kiens, B., et al. (2014). Acute exercise improves motor memory: exploring potential biomarkers. Neurobiol. Learn Mem. 116, 46–58. doi: 10.1016/j.nlm.2014.08.004
Smith, K. J., and Ainslie, P. N. (2017). Regulation of cerebral blood flow and metabolism during exercise. Exp. Physiol. 102, 1356–1371.
Smith, M., Tallis, J., Miller, A., Clarke, N. D., Guimaraes-Ferreira, L., and Duncan, M. J. (2016). The effect of exercise intensity on cognitive performance during short duration treadmill running. J. Hum. Kinet. 51, 27–35. doi: 10.1515/hukin-2015-0167
Stone, B. L., Beneda-Bender, M., Mccollum, D. L., Sun, J., Shelley, J. H., Ashley, J. D., et al. (2020). Understanding cognitive performance during exercise in reserve officers’. training corps: Establishing the executive function-exercise intensity relationship. J. Appl. Physiol. 129, 846–854. doi: 10.1152/japplphysiol.00483.2020
Sudo, M., Komiyama, T., Aoyagi, R., Nagamatsu, T., Higaki, Y., and Ando, S. (2017). Executive function after exhaustive exercise. Eur. J. Appl. Physiol. 117, 2029–2038.
Tempest, G. D., and Reiss, A. L. (2019). The utility of functional near-infrared spectroscopy for measuring cortical activity during cycling exercise. Med. Sci. Sports Exerc. 51, 979–987.
Tempest, G. D., Davranche, K., Brisswalter, J., Perrey, S., and Radel, R. (2017). The differential effects of prolonged exercise upon executive function and cerebral oxygenation. Brain Cogn. 113, 133–141. doi: 10.1016/j.bandc.2017.02.001
Thomson, K., Watt, A. P., and Liukkonen, J. (2009). Differences in ball sports athletes speed discrimination skills before and after exercise induced fatigue. J. Sports Sci. Med. 8, 259–264.
Travlos, A. K., and Marisi, D. Q. (1995). Information processing and concentration as a function of fitness level and exercise-induced activation to exhaustion. Percept Mot. Skills 80, 15–26. doi: 10.2466/pms.1995.80.1.15
Tsukamoto, H., Suga, T., Takenaka, S., Tanaka, D., Takeuchi, T., Hamaoka, T., et al. (2016). Repeated high-intensity interval exercise shortens the positive effect on executive function during post-exercise recovery in healthy young males. Physiol. Behav. 160, 26–34. doi: 10.1016/j.physbeh.2016.03.029
Voss, M. W., Nagamatsu, L. S., Liu-Ambrose, T., and Kramer, A. F. (2011). Exercise, brain, and cognition across the life span. J. Appl. Physiol. 111, 1505–1513.
Wang, C. C., Chu, C. H., Chu, I. H., Chan, K. H., and Chang, Y. K. (2013). Executive function during acute exercise: the role of exercise intensity. J. Sport Exerc. Psychol. 35, 358–367.
Williamson, J. W., Nobrega, A. C., Mccoll, R., Mathews, D., Winchester, P., Friberg, L., et al. (1997). Activation of the insular cortex during dynamic exercise in humans. J. Physiol. 503(Pt 2), 277–283. doi: 10.1111/j.1469-7793.1997.277bh.x
Winter, B., Breitenstein, C., Mooren, F. C., Voelker, K., Fobker, M., Lechtermann, A., et al. (2007). High impact running improves learning. Neurobiol. Learn Mem. 87, 597–609. doi: 10.1016/j.nlm.2006.11.003
Won, J., Alfini, A. J., Weiss, L. R., Callow, D. D., and Smith, J. C. (2019). Brain activation during executive control after acute exercise in older adults. Int. J. Psychophysiol. 146, 240–248. doi: 10.1016/j.ijpsycho.2019.10.002
Zheng, K., Zou, L., Wei, G., and Huang, T. (2021). Concurrent performance of executive function during acute bouts of exercise in adults: a systematic review. Brain Sci. 11:1364. doi: 10.3390/brainsci11101364
Keywords: cognition, dual task, cerebral blood flow, cerebral oxygenation, cerebral metabolism, neuromodulation
Citation: Sudo M, Costello JT, McMorris T and Ando S (2022) The effects of acute high-intensity aerobic exercise on cognitive performance: A structured narrative review. Front. Behav. Neurosci. 16:957677. doi: 10.3389/fnbeh.2022.957677
Received: 31 May 2022; Accepted: 29 August 2022;
Published: 23 September 2022.
Edited by:
Chong Chen, Yamaguchi University Graduate School of Medicine, JapanReviewed by:
Tao Huang, Shanghai Jiao Tong University, ChinaZhaowei Kong, University of Macau, Macao SAR, China
Lei Cui, Beijing Normal University, China
Copyright © 2022 Sudo, Costello, McMorris and Ando. This is an open-access article distributed under the terms of the Creative Commons Attribution License (CC BY). The use, distribution or reproduction in other forums is permitted, provided the original author(s) and the copyright owner(s) are credited and that the original publication in this journal is cited, in accordance with accepted academic practice. No use, distribution or reproduction is permitted which does not comply with these terms.
*Correspondence: Mizuki Sudo, bWktc3Vkb0BteS16YWlkYW4ub3IuanA=; Soichi Ando, c29pY2hpLmFuZG9AdWVjLmFjLmpw