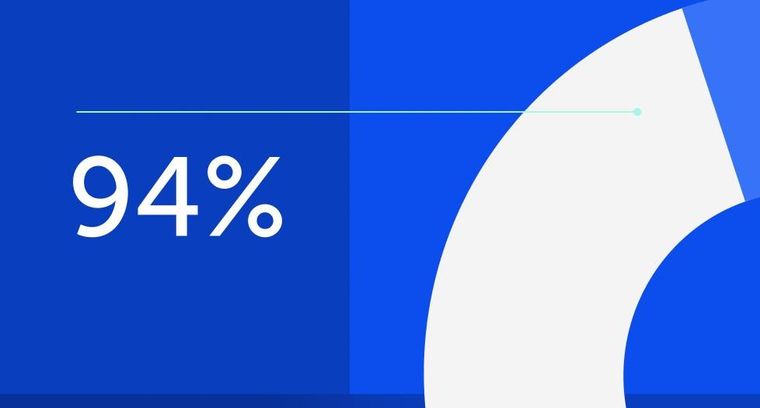
94% of researchers rate our articles as excellent or good
Learn more about the work of our research integrity team to safeguard the quality of each article we publish.
Find out more
ORIGINAL RESEARCH article
Front. Behav. Neurosci., 06 December 2022
Sec. Learning and Memory
Volume 16 - 2022 | https://doi.org/10.3389/fnbeh.2022.951268
This article is part of the Research TopicNeurobiology of Spontaneous Object Exploration in Recognition MemoryView all 13 articles
Cognitive impairments appear at or before motor signs in about one third of patients with Parkinson’s disease (PD) and have a cumulative prevalence of roughly 80% overall. These deficits exact an unrelenting toll on patients’ quality and activities of daily life due in part to a lack of available treatments to ameliorate them. This study used three well-validated novel object recognition-based paradigms to explore the suitability of rats with knockout of the PTEN-induced putative kinase1 gene (Pink1) for investigating factors that induce cognitive decline in PD and for testing new ways to mitigate them. Longitudinal testing of rats from 3–9 months of age revealed significant impairments in male Pink1–/– rats compared to wild type controls in Novel Object Recognition, Novel Object Location and Object-in-Place tasks. Task-specific differences in the progression of object discrimination/memory deficits across age were also seen. Finally, testing using an elevated plus maze, a tapered balance beam and a grip strength gauge showed that in all cases recognition memory deficits preceded potentially confounding impacts of gene knockout on affect or motor function. Taken together, these findings suggest that knockout of the Pink1 gene negatively impacts the brain circuits and/or neurochemical systems that support performance in object recognition tasks. Further investigations using Pink1–/– rats and object recognition memory tasks should provide new insights into the neural underpinnings of the visual recognition memory and visuospatial information processing deficits that are often seen in PD patients and accelerate the pace of discovery of better ways to treat them.
Parkinson’s disease (PD) is a common neurodegenerative disorder that is characterized by motor deficits such as bradykinesia, postural instability and resting tremor (Bloem et al., 2021; Vazquez-Velez and Zoghbi, 2021). However, many PD patients also experience non-motor symptoms including impairments in cognition and memory (Aarsland et al., 2010, 2017; Goldman et al., 2018; Fang et al., 2020). These impairments appear at or before motor signs in about one third of all PD patients and have a cumulative prevalence of more than 80% overall (Aarsland et al., 2017; Papagno and Trojano, 2018; Fang et al., 2020). Although termed ‘mild cognitive impairments’ to distinguish these earlier occurring deficits from those associated with Parkinson’s disease-related dementia (PDD), these cognitive deficits exact a significant toll on patients’ quality and activities of daily life (Leroi et al., 2012; Kudlicka et al., 2014; Oosterveld et al., 2015; Rodriguez-Blazquez et al., 2015; Barone et al., 2017; Saredakis et al., 2019). They also predict a more rapid and more severe clinical course of cognitive and motor decline and are associated with increased risk for freezing, falls and for developing PDD (Pigott et al., 2015; Mack and Marsh, 2017; Cholerton et al., 2018; Goldman et al., 2018). Equally concerning is the lack of available treatments that can effectively combat these impairments (Goldman and Weintraub, 2015; Mack and Marsh, 2017; Goldman et al., 2018; Fang et al., 2020). Using a series of novel object recognition-based paradigms, the studies presented here provide behavioral evidence indicating the potential suitability of rats bearing knockout of the PTEN (phosphatase and tensin homolog)-induced putative kinase1 gene (Pink1–/–) for facilitating the preclinical studies that are necessary to better understand and better treat cognitive and memory dysfunction in PD.
Currently, there are few available therapeutic options that are effective in preventing or slowing the progression of cognitive and memory decline in PD (Goldman and Weintraub, 2015; Mack and Marsh, 2017; Goldman et al., 2018; Fang et al., 2020). Thus, in addition to clinical trials, animal and especially rodent models are being used to support controlled investigations into the risk factors and pathophysiological mechanisms that contribute to cognitive disturbance in PD and to test new ways of mitigating them (Fan et al., 2021). There are several reasons to predict that Pink1–/– rats may be well-suited for these purposes. First, Pink1–/– rats have construct validity for the recessively inherited loss of function Pink1 mutations that are the second most common mutation among autosomal recessive forms of PD; these mutations are also causally linked to early onset familial cases of PD (Valente et al., 2004; Kumazawa et al., 2008; Scarffe et al., 2014). In addition to disrupting mitochondrial function (Borsche et al., 2020), Pink1 mutations in PD patients have also been shown to increase central nervous system vulnerability to reactive oxygen species, to dysregulate dopamine (DA) synthesis and reuptake (Gautier et al., 2008; Bus et al., 2020; Goncalves and Morais, 2021), to induce ferritin accumulation and iron toxicity in midbrain DA neurons (Hagenah et al., 2008) and to promote alpha-synuclein aggregation (LSamaranch et al., 2010; Takanashi et al., 2016; Nybo et al., 2020). A rapidly growing literature documents characteristics similar to these in Pink1 knockout rat lines (Urrutia et al., 2014; Villeneuve et al., 2016; Creed and Goldberg, 2018; Ren and Butterfield, 2021). Further, although the data are not entirely consistent (de Haas et al., 2019), this rat strain has also been shown to undergo progressive loss of midbrain DA and brainstem norepinephrine (NE) neurons (Dave et al., 2014; Grant et al., 2015; Villeneuve et al., 2016; Cullen et al., 2018; Kelm-Nelson et al., 2018b). Earlier occurring, presumed compensatory changes in neostriatal concentrations and/or basal and potassium stimulated release of DA, acetylcholine (ACh), serotonin and other PD-relevant neurotransmitter systems have also been reported (Dave et al., 2014; Creed et al., 2019). Finally, Pink1–/– rats display behavioral deficits in motor and non-motor functions that mimic those experienced by PD patients. For example, in addition to age-related decline in gait coordination and grip strength (Dave et al., 2014), Pink1–/– rats also demonstrate early-appearing deficits in sensorimotor cranial/otolaryngeal functions that negatively impact vocalizations, chewing and swallowing (Grant et al., 2015; Cullen et al., 2018; Kelm-Nelson et al., 2018a,2021). In addition, Pink1–/– rats also show behavioral correlates reflecting increased anxiety, e.g., changes in distress vocalizations, social approach, open vs. closed arm entries in elevated plus maze testing (Kelm-Nelson et al., 2018a; Cai et al., 2019; Hoffmeister et al., 2021, 2022). This suggests face validity for the mood disturbances that are common in PD patients– including those with causal mutations in the Pink1 gene (Ephraty et al., 2007; Ricciardi et al., 2014). However, there has been little systematic effort to determine whether Pink1–/– rats also model PD-relevant cognitive or memory phenotypes. This is despite evidence that among genetically determined forms of PD, patients with Pink1 mutations have the greatest incidence of cognitive dysfunction and decline (Piredda et al., 2020; Gonzalez-Latapi et al., 2021). To fill this gap in knowledge, longitudinal testing using Novel Object Recognition (NOR), Novel Object Location (NOL), and Object in Place (OiP) paradigms was used to determine whether and when Pink1–/– rats express deficits similar to the impairments in visual recognition memory and//or visuospatial information processing that commonly occur in PD patients (Owen et al., 1993; Higginson et al., 2005; Possin et al., 2008; Fang et al., 2020; Fernandez-Baizan et al., 2020).
Object recognition-based behavioral paradigms are well-validated and widely used for evaluation of mnemonic constructs similar to those that are frequently at risk in neuropsychiatric disorders including Alzheimer’s disease, schizophrenia, PD and others (Grayson et al., 2015). Further, these single-trial tasks require no formal training, leverage spontaneous behaviors, are minimally stressful and can require minimal physical exertion (Ennaceur, 2010; Luine, 2015; Aggleton and Nelson, 2020; Chao et al., 2020). These features are especially important for studying cognition and memory in preclinical models of PD where potentially confounding disease-related features of anhedonia, mood disturbance and motor impairment may be present. Finally, there is a rich, task-specific literature for object recognition paradigms describing the brain regions, networks and neurochemical systems that provide essential support for the different forms of recognition memories that these paradigms measure (Dere et al., 2007; Brown et al., 2012; Aggleton and Nelson, 2020; Barker and Warburton, 2020a,b; Chao et al., 2020). Thus, there is a powerful interpretive framework at hand for gaining insights into the neural circuits that may be most affected by pathophysiology and where targeted therapeutics may be most beneficial. Given these benefits, it is not surprising that NOR, NOL and to a lesser extent OiP tasks continue to be widely used to assess cognitive deficits in a range of rodent models of PD (Grayson et al., 2015; Johnson and Bobrovskaya, 2015; Haghparast et al., 2018; Ikram and Haleem, 2019; Kyser et al., 2019; Bharatiya et al., 2020; Boi et al., 2020; Fan et al., 2021; Kakoty et al., 2021; Pinizzotto et al., 2022). This study extends this utilization for the first time to Pink1–/– rats in longitudinal comparative evaluations of object recognition memories in single cohorts of knockout and wildtype (WT) male Long Evans rats. In addition to object exploration and discrimination, the analyses presented below include assessments of motor function and affect that were made in conjunction with object recognition testing. Rats were also further evaluated at the beginning and end of the object recognition testing sequence using elevated plus maze testing, analyses of forelimb and hindlimb grip strength and assessments of foot slips in traversing a tapered balance beam.
Male Long Evans rats that were either WT (n = 8) or Pink1 knockouts [Pink1–/–, (LE-Pink1em1Sage–/–) n = 16] were purchased at 6 or 7 weeks of age (Envigo, Madison, WI, United States). All rats were double housed by genotype for the duration of the study in standard translucent tub cages (Lab Products, Inc., Seaford, DE, United States) filled with ground corn cob bedding (Bed O’ Cobs, The Anderson Inc., Maumee, OH, United States). Rats were kept under a 12-h non-reversed light-dark cycle with food (Purina PMI Lab Diet: ProLab RMH 3000) and water available ad libitum. Enrichment objects (Nyla Bones, Nylabone, Neptune, NJ, United States) were also present in each cage. During the intervals when rats were not being behaviorally tested, they spent roughly 1 h per week in groups of 2–6 in a large, dimly lit 6 ft square enclosure that contained tunnels, platforms and other larger scale objects for them to interact with. All procedures involving animals were approved by the Institutional Animal Care and Use Committee at Stony Brook University and were performed in accordance with the U.S. Public Health Service Guide for Care and Use of Laboratory Animals to minimize their discomfort. Rats were weighed every month as part of a measure of continued good health and to confirm an expected phenotype of greater body mass in age-matched Pink1–/– compared to WT control rats (Figure 1).
Figure 1. Line graphs showing changes in average weights in grams (g) of the male rats with knockout of the PTEN (phosphatase and tensin homolog)-induced putative kinase1 gene (Pink1–/–, triangles, dashed line) and the wild type (WT, circles, solid line) control male rats used in this study as they matured from 3 to 9 months (mos) of age. All rats continued to gain weight as the study progressed. As expected, the average weights of Pink1–/– rats were consistently greater than that of the WT cohort.
Habituation and behavioral testing was conducted in a dedicated core facility that includes a central home cage holding room and 5 adjacent 10–12 ft square sound attenuated testing rooms. Each testing room had adjustable high contrast spatial cues on the walls and digital cameras to archive trials. Habituation and testing were conducted during rats’ subjective days between the hours of 9:00 am and 1:00 pm under ambient white lighting (∼260 lux).
Object recognition tasks were carried out in open rectangular testing arenas (32 in long, 19 in wide, 13 in high) made of translucent polypropylene. The arenas sat on a table 36 in high. One of the long walls of the arena was made opaque and adjustable, small, high contrast cues were affixed to the outsides of the other three arena walls. These cues as well as distal room cues remained fixed during a given testing period and were rearranged across bimonthly testing sessions.
The elevated plus maze used was constructed of white laminate. It consisted of two open arms (5.5 in × 20.5 in), two closed arms (5.5 in × 20.5 in × 11.25) and an open central platform (5.5 in × 5.5 in). The maze was located 3 feet off the ground.
Grip strength was measured using a San Diego Instruments Animal Grip Strength System outfitted with two push/pull wire mesh force gauges (San Diego Instruments, San Diego, CA, United States).
The tapered balance beam used was composed of black plastic composite (Lafayette Instrument, Lafayette, IN, United States). The top surface was rough to provide grip. The beam was 165 cm in length and tapered in width from 6 to 2 cm. Colored rulers were affixed to the sides of the beam that divided it into wide, medium and narrow thirds. A 2 cm wide ledge ran beside and below both sides of the beam surface to provide a crutch/step off position for rats to use if needed. Two digital cameras were used to record trials from the left and right sides that rendered all four feet visible.
One week after their arrival at Stony Brook University, rats were habituated to handling, to the central room of the testing facility and to gentle transfer between home cages and other enclosures. One week prior to the start of formal behavioral testing, rats were also habituated to the testing arenas and to the opaque start cylinders used in the object recognition tasks. This habituation consisted of daily exposures during which rats were placed in the start cylinder at the center of the arena; after 10 s the cylinder was lifted and rats were given 10 min to explore the empty arena. This was repeated 2–3 times per day at roughly 60 min intervals for 5 days. The first round of object recognition testing began 3 days later; this and all subsequent rounds of object recognition testing began with an initial 5 min habituation trial in the empty arena.
Rats were behaviorally tested at 3, 5, 7, and 9 months of age on the NOR, NOL and OiP paradigms; these tasks were given pseudorandom order with 48 h off in between each paradigm. Each trial began by placing rats in an opaque start cylinder located at the center of the arena. After a 10 s delay, the cylinder was lifted and rats were free to explore. Different sets of sample and test objects were used for each task and for each time a given task was delivered. The arena and objects were cleaned with 70% EtOH before and after every trial.
Novel Object Recognition testing consisted of three 3 min sample trials, each separated by 1 h (Figure 2A), and one 3 min test trial separated from the last Sample Trial by 90 min (Figure 2B). Rats were returned to home cages during intertrial intervals. During sample trials, two identical objects were placed in adjacent corners of the arena leaving at least 4-inch clearance from the walls. During the test trial rats explored objects that were in the same locations as during sample trials, albeit with one object from the sample trials and one that was novel. The pairs of objects used in NOR testing (Figures 2C–F) were similar in overall size and/or shape but differed along dimensions including complexity of shape (e.g., 3 months, Figure 2C), composite material (glass or metal, e.g., 5 months, Figure 2D), height and color/contrast (e.g., 7, 9 months, Figures 2E,F) and/or surface features, e.g., smooth vs. grooved (e.g., 5, 7 months, Figures 2D,E). The objects that served as sample vs. novel objects and their locations in the arena were counterbalanced across rats in both groups.
Figure 2. (A,B) Schematic diagrams showing trial structure for the Novel Object Recognition paradigm. (C–F) Black and white photographs showing the items that were used as sample or novel objects for testing at each of the four ages evaluated [3, 5, 7, and 9 months (mos) of age]. The objects are displayed at a 45 degree angle relative to each other to provide spatial perspective. The objects used in testing at 3 mos of age (C) were made of cast iron. The objects used in testing at 5 mos of age (D) were made of aluminum (left) or glass (right). Both items used for testing at 7 (E) and 9 mos of age (F) were made of glass. Scale bars = 50 mm.
Novel Object Location testing consisted of three 3 min sample trials each separated by 1 h (Figure 3A) and a 3 min test trial separated from the last sample trial by 1 h (Figure 3B). Rats spent all intertrial intervals in home cages. During the sample trial, two identical objects were placed in adjacent corners of the arena with a 4-inch clearance from the walls. During test trials rats explored the same two objects but with one located in a corner that was occupied during the sample trial and the other placed in a previously unoccupied corner. Because there is no need to match the valence between objects within trials, the pairs of objects used in NOL had features such as depressions and handles that encouraged close exploration. Across trials, the objects used were made of plastic (Figure 3C), ceramic (Figure 3D), glass (Figure 3E) or a combination of plastic and glass (Figure 3F). The arena corners that served as sample vs. novel locations were counterbalanced across rats in both groups.
Figure 3. (A,B) Schematic diagrams showing trial structure for the Novel Object Location paradigm. (C–F) Black and white photographs show the paired items that were used as sample objects for testing at each of the four ages evaluated [3, 5, 7, and 9 months (mos) of age]. The objects used in testing at 3 mos of age (C) were made of plastic. The objects used in testing at 5 mos of age (D) were made of ceramic. The objects used in testing at 7 mos of age (E) was made of glass micglass, and objects used in testing at 9 mos of age (F) were made of glass and plastic. Scale bars = 50 mm.
Object-in-Place (OiP) testing consisted of three 3 min sample trials each separated by 5 min (Figure 4A) and a 3 min test trial separated from the last sample trial by a 5-min inter-trial interval (Figure 4B). Rats were returned to home cages during the intertrial intervals. For sample trials, 4 distinct objects were placed near each of the arena’s corners (4-inches from the walls). During Test trials, rats explored the same four objects, albeit with two occupying original positions and two occupying positions that were switched with each other. The groups of objects used in OiP testing were grossly matched in terms of size but each differed from the others along dimensions including color/contrast, composite material (plastic, ceramic, glass or metal), general shape and/or surface features. The objects used for testing at 3, 5, 7, and 9 months of age are shown in Figures 4C–F, respectively. The positions and pairs of objects that occupied switched vs. stationary positions were counterbalanced across subjects in both groups.
Figure 4. (A,B) Schematic diagrams showing trial structure for the Object in Place paradigm. (C–F) Black and white photographs showing the four distinct items used as sample objects for testing at each of the four ages evaluated [3, 5, 7, and 9 months (mos) of age]. From left to right, the objects used in testing at 3 mos of age (C) were made of cast iron, ceramic, steel or glass. The objects used in testing at 5 mos of age (D) were made of glazed ceramic, aluminum, contoured glass or smooth glass (left to right). The objects used in testing at 7 mos of age (E) were made of ceramic, molded plastic, glass, or smooth plastic with metal. The objects used in testing at 9 mos of age (F) were all made of glass. Scale bars = 50 mm.
Rats were tested on the elevated plus maze at 3.5 and 9.5 months of age approximately 1 week after completing object recognition testing. At the start of the trial, rats were placed on the center portion of the maze facing away from the handler and were given a single 5 min trial to freely explore. All maze surfaces were cleaned with 70% ethanol before and after each trial.
Rats were held parallel to the center platform of the apparatus. Once they grasped the forelimb force plate, they were gently pulled backwards, away from it. After they released the forelimb plate, they continued to be drawn across the hindlimb force plate, which rats grabbed onto with hind feet while rats attempted to push forward. Thus, single trials were used to measure forelimb pull strength and hindlimb push/compressive strength. During each session, rats were given three trials that were separated by 30 s to 1 min. The system automatically collects values of maximal force which were used for analyses.
Wire tops were removed from rats’ home cages and the edge of the open home cage was used to support the narrow end of the balance beam. Rats were removed from the home cage and habituated to beam crossing by first placing them on the narrow end of the beam within a few steps of the home cage. Once they left the beam, they were given 1–2 min in the home cage as reward before being returned to the beam at wider and wider points (farther from the home cage). This was repeated until rats traversed the beam length with minimal stopping. All rats acquired this level of performance quickly, usually in less than three full length runs. Rats were then rested for about 15 min before being given three sequential full length trials.
Behavioral data were analyzed from digitally recorded trials by trained observers who were blind to genotype/group. Event-capture software [Behavioral Observation Research Interactive Software (BORIS) version 7.8.2, open access] was used to quantify the timing, instances and durations of specific behaviors defined below.
Total time in seconds rats spent actively exploring objects using vibrissae or snout. Sample trial object exploration was additionally evaluated for.
Total times in seconds rats spent actively exploring objects located in a given corner, quadrant or half of the arena.
Total times in seconds rats spent actively exploring distinct objects either presented simultaneously (OiP) or counterbalanced across subjects (NOR, NOL).
Total time in seconds rats spent actively exploring objects using vibrissae or snout. Test trial object exploration was additionally evaluated for:
NOR: Total time (in seconds) rats spent investigating novel (NO) vs. familiar objects (FO), expressed as percent of total object exploration time. This index was calculated by the following formula:
[NO] − [FO]/[NO] + [FO]
NOL: Total time (in seconds) rats spent investigating objects in new (Nw) vs. original positions (Or), expressed as percent of total object exploration time. This index was calculated by the following formula:
[Nw] − [Or]/[Nw] + [Or]
OiP: Total time (seconds) rats spent investigating two objects in switched (Sw) compared to original (Or) positions, expressed as percent of total object exploration time. This index was calculated by the following formula:
[(Sw-Or)/(Sw + Or)].
Test trials were analyzed for four major behaviors other than object exploration. The behaviors were defined as below:
• Rearing: total time (seconds) rats spent standing on hind paws either assisted by forepaw contact with objects or walls, or without assistance.
• Grooming: total time (seconds) rats spent preening any part of the head or body.
• Ambulation: total time (seconds) rats made forward motion via steps involving all four paws.
• Stationary: total time (seconds) rats sat at a given location and did not engage in grooming or object investigation.
Rats were evaluated for:
• Arm entries: Forward locomotion culminating in all four paws being inside a given arm. Separate counts were made of total arm entries, entries into closed arms and entries into open arms.
• Total times (in seconds) rats spent in open arms, closed arms or on the center platform of the maze.
• Duration (in percent total open arm occupation time) of major activities in open arms.
° Head dipping- Investigation, with head and shoulders positioned over the edge of the open arm.
° Ambulation- As per “Other Behaviors” above
• Duration (in percent total closed arm occupation time) of major activities in closed arms.
° Rearing- As per “Other Behaviors” above
° Grooming-As per “Other Behaviors” above
° Ambulation- As per “Other Behaviors” above
° Stationary-As per “Other Behaviors” above
• Duration (in percent total center platform occupation time) of major activities in center platform.
° Stretch attend/scanning- total times rats spent making forward and back or side-to-side exploratory movements of the forebody with hindlimbs and tail remaining in place.
° Rearing- As per “Other Behaviors” above
° Ambulation- As per “Other Behaviors” above
° Head dipping- Investigation with head and shoulders positioned over the edge of the open central platform.
The automated system was used to measure pull force of forelimbs and push/compressive force of hindlimbs. Values of maximal force recorded were normalized to body mass/weight prior to analysis.
Rats were evaluated for foot slips made while traversing the full length of the balance beam. Data were collected separately for wide, middle and narrow portions of the beam. Because foot slips were rare for rats in both groups, these data were collapsed into measures of total numbers of foot slips per traversal for analysis. The percentage of rats per group committing some vs. no foot slips was also recorded.
Statistical analyses were performed using IBM SPSS, Version 25 (SPSS, Inc., Chicago, IL, United States). The data were first assessed for descriptive statistics, including Levine’s F-test for equality of variance. Comparisons of single measures across group/genotype were made using one-way analyses of variance (ANOVA), comparisons of measures across age were made using within-groups, one-way ANOVAs with repeated measures designs and comparisons of multiple measures made across groups used two-way repeated measures ANOVA. For all repeated measures comparisons, Mauchly’s test for sphericity of the covariance matrix was applied and degrees of freedom were adjusted as indicated using the Huynh-Feldt epsilon. Discrimination index (DI) data were additionally evaluated within groups using one sample t-tests to determine whether DI values were significantly different than zero, and relationships between individual measures of DI and rats’ total times spent exploring objects during sample and test trials were also assessed within groups by calculating Pearson’s correlation coefficients. All comparisons were additionally evaluated for effect sizes by calculating eta squared (η2) for ANOVAs or using Cohen’s D for t-tests.
At 3 months of age, Pink1–/– rats spent nearly twice as much total sample trial time investigating objects as did WT controls (Pink1–/– = 93 s, WT = 52 s, Figure 5A); this difference was significant [ANOVA, F(1,21) = 26.61, p < 0.001, η2 = 0.56]. During subsequent testing Pink1–/– rats also tended to spend more time exploring sample objects (Figure 5A). However, the differences in sample observation times seen at these later ages were relatively small (5 months = 142 vs. 128 s; 7 months = 62 vs. 56 s; 9 months = 75 vs. 75 s) and were not significantly different across genotype (η2 = 0.01–0.05).
Figure 5. Novel Object Recognition data. Bar graphs showing total times in seconds (sec) that wild type (WT, black bars) and rats with knockout of the PTEN (phosphatase and tensin homolog)-induced putative kinase1 gene (Pink1–/–, gray bars) spent actively exploring objects during Sample Trials (A) and during Test Trials (B) in testing at 3, 5, 7, and 9 months (mos) of age. Overall, Pink1–/– rats spent more time than WT rats investigating objects; asterisks identify these differences as significant at 3 mos of age for Sample Trials (A), and for 5 mos of age for Test Trials (B). (C) Bar graphs showing calculated discrimination index (DI) for WT (black bars) and Pink1–/– rats (gray bars). This measure of object recognition memory was similar in rats of both genotypes at 3 months of age. At all other ages, DIs were significantly greater (*) in WT than in Pink1–/– rats. Within-groups comparisons of DI across age showed that in Pink1–/– rats, DIs measured at 5, 7, and 9 months of age were significantly lower (#) than DI measured at 3 months of age. (D) Tables showing R2 and p-values for regression analyses that compared DIs to total sample object exploration times and to test trial object exploration times in WT and Pink1–/– rats at each age tested. No significant or near significant correlations were found among these measures.
At all ages tested, rats in both groups tended to divide total times investigating sample objects more or less equally among the two objects present. This was confirmed in a series of within-groups repeated measures ANOVAs that in most cases found no significant main effects of object position on object exploration (3 Pink1–/–, WT, η2 = 0.007, 0.013; 9 months: Pink1–/–, WT, η2 = 0.023, 0.15). The only exception occurred among WT controls at 5 months of age. For this timepoint, a significant main effect of Object Position [F(1,2) = 9.16, p = 0.019, η2 = 0.57] was found that was driven by WT rats dividing total sample object investigation times among the two objects present according to a ratio of roughly 60–40. There were no significant group differences noted in sample trial object exploration times based on which object was used as sample at any age (η2 = 0–0.064).
Rats of both genotypes spent roughly 10–30% of test trial times exploring objects (Figure 5B). On average Pink1–/– rats spent more time exploring objects than WT controls (Figure 5B). However, these differences were generally less than 5 s (3 months = 54 vs. 50 s; 5 months = 42 vs. 33 s; 7 months = 25 vs. 24 s; 9 months = 22 vs. 20 s). Analyses of variance showed that main effects of genotype on this measure were only significant at 5 months of age [F(1,21) = 4.44, p = 0.047, η2 = 0.17].
Rats of both genotypes demonstrated robust discrimination of novel compared to familiar objects at 3 months of age (WT DI = 0.51; Pink1–/– DI = 0.40, Figure 5C). An ANOVA confirmed that there were no significant differences between these two values (η2 = 0.068) and one-sided t-tests showed that DI values for rats of both genotype were significantly different/greater than zero [WT: t(7) = 7.56, Pink1–/–: t(14) = 7.26, p < 0.001, d = 0.19 and 0.21, respectively]. During subsequent testing, WT rats maintained robust levels of novel object discrimination (DIs = 0.38 – 0.42, Figure 5C). A within-groups, repeated measures ANOVA further confirmed that DI values in this group were unchanged from 3 to 9 months (η2 = 0.11), and one-sided t-tests showed that all DI values were significantly different/greater than zero [t(7) = 3.6–4.8, p < 0.001–0.006, d = 0.22–0.31]. In contrast, novel object discrimination in 5-month-old Pink1–/– rats dropped dramatically to very low levels that were maintained up to 9 months of age (DIs = 0.02–0.05, Figure 5C). A within-groups ANOVA identified significant impacts of age on DI in the Pink1–/– group [F(3,42) = 11.12, p < 0.001, η2 = 0.44] and follow-up comparisons confirmed that the DIs measured at 5, 7, and 9 months in these knockout rats were significantly lower than that measured at 3 months (p < 0.001 for all ages). A series of one-sided t-tests also showed that none of the DI values measured in Pink1–/– rats at 5–9 months of age were significantly different than zero (d = 0.17–0.27). Finally, ANOVAs that compared groups identified main effects of genotype on DI in rats that were significant at 5, 7, and 9 but not 3 months of age [5 months: F(1,21) = 12.05, P = 0.002; 7 months: F(1,20) = 13.00, p = 0.002; 9 months: F(1,21) = 9.65, p = 0.005, η _^2 = 0.32–0.39, Figure 5C]. Regression analyses confirmed that there were no significant or near significant positive correlations between DIs and measures of object exploration during sample or test trials for either genotype at any age (R2 = 0–0.35, p = 0.13–0.98, Figure 5D).
Analyses of ambulation, rearing, grooming and remaining stationary during NOR test trials identified significant main effects in the way that animals apportioned test trial times across these behaviors [F(1.52–2.20, 30.92–56.60) = 17.43–33.41, p < 0.001 for all, η2 = 0.45–0.61]. For all testing except at 7 months of age, significant main effects of genotype [F(1, 21) = 4.66, p = 0.043, η2 = 0.18] and/or significant interactions between genotype and behavior [F(1.194–2.69, 40.20–56.57) = 3.76–9.64, p < 0.001–0.027, η2 = 0.15–0.32) were also found. Follow up comparisons further showed that at every testing age Pink1–/– rats spent significantly less time grooming than the WT rats (3 months = 1.4 vs. 25 s, p < 0.001; 5 months = 6.3 vs. 25 s, p < 0.001; 9 months = 11 vs. 32 s, p = 0.001). At 9 months of age Pink1–/– rats were also found to spend significantly more time ambulating (29 vs. 19 s, p = 0.017) and rearing (57 vs. 29 s, p = 0.002) and significantly less time remaining stationary (60 vs. 81 s, p = 0.011) than WT controls. At all other testing ages, rats of both genotypes spent similar amounts of NOR test trial times engaged in these activities.
At 3 months of age, an ANOVA confirmed that the Pink1–/– rats spent significantly more total sample trial times investigating objects than WT subjects [121 vs. 82 s, F(1,21) = 7.05, p = 0.015, η2 = 0.25, Figure 6A]. However, group differences (Pink1–/– vs. WT) in sample object exploration at subsequent ages were all negligible (5 months = 54 vs. 55 s; 7 months = 39 vs. 40 s; 9 months = 48 vs. 53 s) and were not significant (η2 = 0.001–0.013).
Figure 6. Novel Object Location data. Bar graphs showing total times in seconds (sec) that wild type (WT, black bars) and rats with knockout of the PTEN (phosphatase and tensin homolog)-induced putative kinase1 gene (Pink1–/–, gray bars) spent actively exploring objects during Sample Trials (A) and during Test Trials (B) in testing at 3, 5, 7, and 9 months (mos) of age. In general, Pink1–/– rats spent equal or more time than WT rats investigating objects; asterisks identify object exploration times as significantly greater in the Pink1–/– compared to WT cohort for testing at 3 mos of age during Sample Trials (A), and for testing at 3 and 9 mos of age during Test Trials (B). (C) Bar graphs showing calculated discrimination index (DI) for WT (black bars) and Pink1–/– rats (gray bars). This measure of object location memory was similar in rats of both genotypes at 3 months of age. At all other ages, DIs were significantly greater (*) in WT than in Pink1–/– rats. (D) Tables showing R2 and p-values for regression analyses comparing DIs to total sample object exploration times and to test trial object exploration times in WT and Pink1–/– rats at each age tested. No significant or near significant positive correlations were found among these measures. However, a significant negative correlation (*) between increased sample trial object exploration and lower DI values was found for Pink1–/– rats at 9 months of age.
Analyses of total sample trial object explorations as functions of object position showed that rats of both genotypes investigated the two sample objects present to similar extents. The largest difference seen in exploring one vs. the other object was for 3-month-old WT rats, where an average difference on the order of about 10 s was seen. However, within-groups repeated measures ANOVAs confirmed that this difference and most others were not significant (η2 = 0.001–0.22). The single exception was for 9 months old WT rats, where relatively small differences in the amounts of times spent investigating objects located in each the two corners (23 vs. 29 s) proved significant [F(1,7) = 5.96, p = 0.045, η2 = 0.46].
Analyses of total times spent exploring objects during NOL test trials showed that Pink1–/– rats generally spent more time investigating objects than the WT controls (3 months = 38 vs. 14 s; 5 months = 21 vs. 24 s; 7 months = 18 vs. 13 s; 9 months = 19 vs. 13 sec, Figure 6B). These group differences were significant for rats at 3 [F(1,21) = 19.58, p < 0.001, η2 = 0.50] and 9 months of age [F(1,21) = 6.06, p = 0.023, η2 = 0.22] but did not reach a critical difference at the other two testing ages (η2 = 0.01, 0.15).
At 3 months of age, rats of both genotypes showed modest discrimination of objects in novel compared to familiar locations (WT DI = 0.26; Pink1–/– DI = 0.22, Figure 6C). Thereafter, object location discrimination rose to and remained at considerably higher levels in WT rats for the duration of testing (DI = 0.49–0.64, Figure 6C). In contrast, NOL discrimination in the Pink1–/– group remained low across all subsequent testing (DI = 0.12–0.17, Figure 6C). Nonetheless, one sample t-tests showed that all DI values for both groups were significantly different/higher than zero [WT: t(7) = 2.10- 10.54, p < 0.001–0.04, d = 0.13–0.33; Pink1–/–: t(14) = 2.37–3.83, p < 0.001–0.017, d = 0.17–0.25]. Within-groups, repeated measures ANOVAs also showed that there were no significant main effects of age on DIs for rats of either genotype (η2 = 0.059–0.25). However, across-groups ANOVAs confirmed that DIs in WT rats were significantly higher than those of the Pink1–/– cohort at 5, 7, and 9 months of age [F(1,21) = 11.18–21.28, p < 0.001–0.003, η2 = 0.36–0.50, Figure 6C]. Regression analyses also confirmed that for both groups there were no significant or near significant positive correlations between DIs and measures of sample or test trial object exploration at any age (R2 = 0.001–0.19, p = 0.10–0.91, Figure 6D). However, at 9 months of age a significant negative correlation (greater object exploration/lower DI) was identified between total sample trial objective exploration and DI in the Pink1–/– group [F(1,13) = 7.11, p = 0.019, R2 = 0.35, Figure 6D].
Analyses of ambulation, rearing, stationary and grooming revealed significant differences in the amounts of time rats of all ages allotted to these activities [F(1.46–2.15, 27.81–45.15) = 21.56–43.74, p < 0.001 for all, η2 = 0.53–0.68]. Significant main effects of genotype [F(1,20) = 5.40–13.13, p = 0.002-0.031, η2 = 0.22–0.40] and/or significant interactions between genotype/group and behavior [F(1.55–2.15, 30.93–45.15) = 5.67–14.40, p < 0.001–0.005, η2 = 0.21–0.42] were also identified at all testing ages. Although there was some variance in the data, in general, main effects were driven by Pink1–/– rats spending more time engaged in active behaviors (rearing, ambulation) and less time being sedentary (stationary, grooming) than WT rats. This was borne out in follow up comparisons that showed Pink1–/– rats groomed significantly less than the controls at all ages (3 months = 4 vs. 26 s, p < 0.001; 5 months = 5 vs. 17 s, p = 0.002; 7 months = 3 vs. 22 s, p = 0.001; 9 months = 11 vs. 32 s, p < 0.001) and spent significantly less time stationary than WT rats in testing at 3 and 5 months of age (3 months = 33 vs. 61 s, p < 0.001; 5 months = 32 vs. 64 s, p = 0.004). The Pink1–/– group also spent significantly more time ambulating than WT controls in testing at 5 and 7 months of age (5 months = 39 vs. 29 s, p = 0.017; 7 months = 38 vs. 28 s, p = 0.004) and significantly more time rearing at 5 months of age (81 vs. 44 s, p = 0.001).
At 3 months of age, Pink1–/– rats spent significantly more time investigating sample objects than WT rats [127 vs. 91 s, F(1,21) = 11.73, p = 0.003, η2 = 0.36, Figure 7A]. However, at 5 months of age, WT rats spent significantly more time investigating the samples than the Pink1–/– cohort [111 vs. 88 s, F(1, 21) = 7.66, p = 0.012, η2 = 0.27, Figure 7A]. In testing at 7 and 9 months of age there were no significant main effects of group/genotype on total sample object exploration times between Pink1–/– and WT rats (7 months = 74 vs. 85 s, η2 = 0.09; 9 months = 77 vs. 80 s, η2 = 0.005, Figure 7A).
Figure 7. Object-in-Place data. Bar graphs showing total times in seconds (sec) that wild type (WT, black bars) and rats with knockout of the PTEN (phosphatase and tensin homolog)-induced putative kinase1 gene (Pink1–/–, gray bars) spent actively exploring objects during Sample Trials (A) and during Test Trials (B) in testing at 3, 5, 7, and 9 months (mos) of age. In general, the amounts of time spent exploring objects were comparable among the Pink1–/– WT groups. However, asterisks in (A) identify object exploration times that were significantly greater in the Pink1–/– compared to WT cohort for testing at 3 mos of age, and that were significantly greater in the WT compared to Pink1–/– rats for testing at 5 mos of age during. (C) Bar graphs showing calculated discrimination index (DI) for WT (black bars) and Pink1–/– rats (gray bars). This measure of integrated object recognition memory was similar in rats of both genotypes at 3 and 5 months of age. At all other ages, DIs were significantly greater (*) in WT than in Pink1–/– rats. Within groups comparisons of DI across age showed that in Pink1–/– rats, DIs measured at 7 and 9 months of age were significantly lower (#) then DI measured at 3 and 5 months of age. (D) Tables showing R2 and p-values for regression analyses comparing DIs to total sample object exploration times and to test trial object exploration times in wild type and Pink1–/– rats at each age tested. No significant or near significant correlations were found among these measures.
Rats in both groups investigated each of the four sample items present approximately equally and divided observation times similarly across objects located in each of the arena’s four corners. Thus, there were no indications of bias based on object type or position. This was confirmed in a series of within-groups, repeated-measures ANOVAs that found no significant main effects of object type or arena corner (Pink1–/–: η2 = 0.011–0.11; Control: η2 = 0.008–0.24) on measures of object exploration.
Analyses of total times spent exploring objects during test trials showed that Pink1–/– and Control rats both spent similar amounts of test trial times exploring objects (3 months = 28 s, both; 5 months = 19 vs. 22 s; 7 months = 15 s, both; 9 months = 19 vs. 16 s, Figure 7B). There were no significant main effects of genotype on this measure (η2 = 0.00–0.031).
At 3 months of age, WT and Pink1–/– rats both showed similar ability to discriminate among objects located in exchanged compared to original positions (WT DI = 0.20; Pink1–/– DI = 0.22, Figure 7C); one sample t-tests showed that all DI’s measured in both groups were significantly different/greater than zero [WT: t(7) = 1.95, p = 0.046 and d = 0.28; Pink1–/–: t(13) = 2.52, p = 0.013 and d = 0.33, respectively]. However, testing at later time points showed that DIs in WT rats tended to incrementally increase (5 months DI = 0.29; 7 months DI = 0.41; 9 months DI = 0.37, Figure 7C). One sample t-tests confirmed that all WT DI values were significantly different/greater than zero [t(7) = 2.49–5.77, p < 0.001–0.021, d = 0.18–0.46]. However, within-groups repeated measures ANOVAs showed that the incremental increases in DI observed across age were not significant (η2 = 0.09). In contrast, average DIs in the Pink1–/– group showed a stepwise decline from 5 to 9 months of age (5 months DI = 0.10; 7 months DI = –0.13; 9 months DI = –0.15, Figure 7C). One sample t-tests showed that DI’s measured across this interval were not significantly different than zero (d = 0.32–0.37) and a within-groups repeated measures ANOVAs confirmed DI’s significantly declined with age [F(3,30) = 5.18, p < 0.005, η2 = 0.34]. Follow-up comparisons specifically identified DI’s measured at 3 and 5 months as significantly greater than those measured at 7 and 9 months of age (p = 0.003–0.047, Figure 7C). Finally, across-groups ANOVAs showed that while DIs between the WT and Pink1–/– rats were initially similar, their diverging trajectories culminated in significant group differences at 7 and 9 months of age [F(1,21) = 8.94–13.95, p < 0.001–0.008, η2 = 0.33–0.40, Figure 7C]. Importantly, regression analyses confirmed that there were no significant or near significant positive correlations between DI and measures of object exploration during sample or test trials for any group at any age (R2 = 0.004-0.31, p = 0.15–0.84, Figure 7D).
Analyses of ambulation, rearing, stationary behavior and grooming revealed significant differences in the amounts of time rats of all ages allotted to these activities [F(1.39–3, 23.59–60) = 12.56–90.36, p < 0.001 for all, η2 = 0.43–0.81]. However, there were no significant main effects of genotype (η2 = 0–0.047) and no significant interactions between genotype/group and behavior (η2 = 0.03–0.12) at any testing age.
At 3.5 months of age, rats in both groups made comparable numbers of total arm entries (WT = 9.14, Pink1–/– = 9.60) that for both genotypes were biased toward entries into closed vs. open arms by more than 2 to 1 (Figure 8A). However, a repeated measures ANOVA that compared times spent in a given sector of the maze (open arms, closed arms, center platform, Figure 8B) identified significant main effects of maze location [F(2,40) = 89.12, p < 0.001, η2 = 0.82] and a significant interaction between maze location and genotype/group [F(2,40) = 11.99, p < 0.001, η2 = 0.38]. Follow-up pairwise comparisons showed that these effects were driven by WT rats spending significantly more time in closed arms (129 vs. 85 s, p = 0.005) and significantly less time in the maze center (136 vs. 190 s, p < 0.001) compared to the Pink1–/– cohort (Figure 8B). Analyses of major behaviors exhibited within each maze location (expressed as percent total times spent within these sectors) also revealed group differences (Figures 8C–E). For all three maze compartments, significant main effects were identified for times allotted to particular major behaviors [F(1.61–2.34, 32.10–46.69) = 42.95–51.48, p < 0.001 for all, η2 = 0.68–0.72]. However, significant interactions between group and compartment specific behaviors were only significant for open [F(1,20) = 18.73, p < 0.001, η2 = 0.48, Figure 8C] and closed [F(2.34,46.69) = 9.18, p < 0.001, η2 = 0.32, Figure 8D] arm locations. For the open arms, main effects were driven by WT rats spending about 30% more time head-dipping than Pink1–/– rats (p < 0.001, Figure 8C). For the closed arms, these effects were driven by WT rats spending roughly 12% less time rearing and 16% more time grooming compared to Pink1–/– rats (p < 0.001 for both, Figure 8D). In the center platform, WT and Pink 1–/– rats engaged in stretch attend/scanning (41.6, 49.3% of time), ambulating (14.6, 13.4% of time), head dipping (15.7, 9.5% of time) and rearing (10.0, 13.7% of time) similarly (Figure 8E). There were no significant group differences in these allotted times (η2 = 0.065, 0.001).
Figure 8. Elevated Plus Maze data. (A) Stacked bar graphs showing total arm entries divided into total entries made into open (white) and closed (black) arms for wild type (WT) and rats with knockout of the PTEN (phosphatase and tensin homolog)-induced putative kinase1 gene (Pink1–/–) for testing at 3.5 and 9.5 months (mos) of age. The black asterisk shows that at 9.5 mos of age, rats in the Pink1–/– group made significantly more closed arm entries than WT rats; the white asterisk shows that Pink1–/– rats also made significantly more entries into closed arms than WT rats. (B) Bar graphs showing total amounts of time WT and Pink1–/– rats spent in the open arms (white), closed arms (black) and the center platform (striped) of the maze during testing at 3.5 and 9.5 mos of age. Asterisks show that at both 3.5 and 9.5 months of age, Pink1–/– rats spent significantly less time in closed arms and significantly more time on the center platform than WT rats. Stacked bar graphs showing percentages of total times Pink1–/– and WT rats spent on major behaviors within the open arms (C), closed arms (D), and center platform (E) during testing at 3.5 and 9.5 mos of age. Major behaviors examined included stationary behavior (black), ambulation (dark gray), rearing (light gray), grooming (white) head dipping (slanted stripes) and engaging in stretch-attend/scanning (vertical stripe) Significant group differences in the percentages of total times that rats devoted to a given behavior are marked by asterisks within the bar graphs of the group where significantly more time was spent.
At 9.5 months of age, a one-way ANOVA showed that WT rats made significantly fewer total arm entries compared to Pink1–/– subjects [5.71 vs. 8.87, F(1,20) = 7.90, p = 0.011, η2 = 0.52, Figure 8A]. A repeated measures ANOVA further identified significant main effects of arm type [F(1,20) = 145.21, p < 0.001, η2 = 0.88), a significant main effect of group [F(1,20) = 8.70, p = 0.008, η2 = 0.30] and a significant interaction between these two [F(1,20) = 5.95, p = 0.024, η2 = 0.23]. These effects were driven by Pink1–/– rats entering closed arms nearly twice as often (7 vs. 5) as WT rats (p = 0.002, Figure 8A). In terms of times spent, a repeated measures ANOVA also revealed significant main effects of maze location [open arm, closed arm, center platform, F(1.31,26.20) = 109.26, p < 0.001, η2 = 0.85] and a significant interaction between maze location and group [F(1.31, 26.20) = 6.01, p = 0.015, η2 = 0.23). Follow-up pairwise comparisons showed that these effects were driven by WT rats spending significantly more time in closed arms (146 vs. 109 s, p = 0.023) and significantly less time in the maze center (141 vs. 178 s, p = 0.016) compared to the Pink1–/– group (Figure 8B). Rats of both genotypes spent roughly 12 s in the open arms of the arena (Figure 8B). Finally, analyses of major behaviors exhibited in each portion of the maze found no significant main effects of behavior for open arms (η2 = 0.10). For the closed arms and center platform, significant main effects of behavior [F(2.35–3.08, 47.01–61.53) = 12.61–217.62, p < 0.001 for both, η2 = 0.39–0.92] and significant interactions between behavior and group [F(2.35–3.08,47.01–61.53) = 5.93–6.16, p < 0.001–0.003, η2 = 0.23–0.24] were found. For the closed arms (Figure 8D), these effects were driven by WT rats spending roughly 9% less time ambulating (p = 0.008) and about 20% more time grooming compared to the Pink1–/– cohort (p < 0.001). For the center platform (Figure 8E), effects were driven by WT rats spending approximately 10% less time engaged in stretch attend/scanning (p = 0.008), about 5% less time rearing (p = 0.023) and 5–6% more time head dipping (p = 0.042) and ambulating (p < 0.001) compared to the Pink1–/– group.
Forelimb and hindlimb grip strength was measured in rats at 3.5 and 9.5 months of age. All measurements were normalized to total body weight. At both timepoints, the pull force exerted by forelimbs was greater than pushing/compressive force measured for hindlimbs in both rat groups (Figures 9A,B). However, there were no significant main effects of genotype/group on either of these measures at either age tested (forelimb:η2 = 0–0.11; hindlimb:η2 = 0–0.03). There were, however, significant main effects of age on normalized grip strength measures for both groups [F(1,23) = 8.21–41.60, p < 0.001–0.009, η2 = 0.26–0.60]. These main effects were driven by increased normalized hindlimb grip strength forces in 9.5 compared to 3.5-month-old rats of both genotypes and increased forelimb grip strength for only WT controls.
Figure 9. Grip Strength and Tapered Balance Beam data. Bar graphs showing measures of maximum forelimb (A) and hindlimb (B) grip strength/force normalized to body mass [grams of force (gf)/body weight in grams (g)] in wild type (WT, black bars) and rats with knockout of the PTEN (phosphatase and tensin homolog)-induced putative kinase1 gene (Pink1–/–, gray bars) measured at 3.5 and 9.5 months (mos) of age. There were no significant group differences in these measures of muscle strength at either age. Bar graphs showing average total numbers of foot slips committed by WT (black bars) or Pink1–/– rats (gray bars) in tapered balance beam traversal trials at 3.5 (C) and 9.5 (D) mos of age. Pie graph inserts show the proportion/percent of rats in each group that did or did not commit foot slips during the trial. Numbers of foot slips were minimal in both groups at both ages. However, proportionally more Pink1–/– rats made foot slips compared to WT rats at 9.5 mos of age. The asterisk on the X-axis (D) denotes that five rats in the Pink1–/– group were removed from testing at 9.5 mos of age due to inability to cross the beam.
Tapered balance beam performance was assessed in rats at 3.5 (Figure 9C) and 9.5 months of age (Figure 9D). It is important to note that five of the Pink1–/– rats were no longer able to navigate the beam at the later time point and did not contribute to group data for this age. None of the WT rats were removed from these analyses. Average numbers of foot slips were slightly greater among Pink1–/– that did complete the task compared to WT rats at both ages. These differences, however, were small, not significant (η2 = 0.01–0.02) and represented small numbers of actual step offs/slips. The numbers/percentages of animals in each group that did and did not commit step offs/foot slips were also assessed; these percentages were similar among both groups of rats at 3.5 months of age (Figure 9C) but were greater in the Pink1–/– compared to WT rats at 9.5 months of age (Figure 9D).
Cognitive impairments associated with PD are disabling for a considerable proportion of patients (Leroi et al., 2012; Kudlicka et al., 2014; Rodriguez-Blazquez et al., 2015; Barone et al., 2017). These impairments are also resistant to most available treatments (Goldman and Weintraub, 2015; Mack and Marsh, 2017; Goldman et al., 2018; Fang et al., 2020). Left unchecked, what may initially be mild deficits often progressively worsen and increase the likelihood of patients experiencing freezing and falls and developing PDD– which are leading causes of hospitalization, institutionalization and death among PD patients (Pigott et al., 2015; Peterson et al., 2016; Cholerton et al., 2018). While Pink1–/– rats have been shown to recapitulate several key bio-behavioral aspects of PD, it has been largely unknown whether these rats also model the cognitive and/or memory sequelae associated with this disease. To address this question, the present study used three object recognition memory tasks to explore the face validity of this genetic rat model for the deficits in visual recognition memory and/or visuospatial information processing that commonly occur in PD patients (Owen et al., 1993; Higginson et al., 2005; Possin et al., 2008; Fang et al., 2020; Fernandez-Baizan et al., 2020). These analyses revealed significant impairments in the Pink1–/– cohort in NOR, NOL, and OiP performance as well as task-specific differences in the progression of these object discrimination/memory deficits across age. To summarize, at 3 months of age, rats of both genotypes showed robust ability to discriminate novel objects. The WT rats sustained these high levels of discrimination across all subsequent testing ages. However, in the Pink1–/– cohort, NOR performance declined sharply by 5 months of age and remained extremely low from this age on. In contrast, for the NOL task, rats of both genotypes initially (3 months of age) showed only modest ability to discriminate objects based on their location. However, by 5 months of age, NOL performance in WT rats rose to and remained at higher, more expected degrees discrimination while performance in the Pink1–/– group remained moderate to low at all ages. Finally, at 3 months of age the integrative recognition memory functions tapped in the OiP task were moderate in both WT and Pink1–/– rats. With successive testing, however, performance in WT rats incrementally increased and performance in Pink1–/– rats steadily declined. From these data it is tempting to speculate that knockout of the Pink1 gene negatively impacts the brain circuits and/or neurochemical systems that are essential for performance in these tasks. However, given previous evidence for motor and affective disturbances in Pink1–/– rats (below) it is important to determine whether and how such non-mnemonic factors may have influenced the behavioral outcome measures observed. As discussed below, this was done by incorporating measures of motor function and affect/anxiety into analyses of object recognition task performance, and by bracketing the longitudinal object recognition testing sequence with elevated plus maze testing, measurements of hind- and forelimb grip strengths and assessments of motor coordination in traversing a tapered balance beam.
As for many preclinical models of PD, it is important that analyses of object recognition memory testing take into consideration the possibility that motor and/or non-motor deficits could be confounding to data interpretation. For example, some muscular/motor effort is required for rats to get to and interact with the objects presented. In addition, disturbances in affect or anxiety can influence animals’ willingness to approach or explore objects, particularly those that are unfamiliar (Ennaceur et al., 2005, 2006, 2009). The previous studies showing that Pink1–/– rats experience progressive motor deficits and/or show an affective phenotype discussed below underscore the need for careful assessments to assure that the data from object recognition testing reported here reflect cognitive and/or mnemonic status. Accordingly, the present studies incorporated concurrent analyses of motor activity and affect into assessments of object recognition task performance, further evaluated motor function using a grip strength gauge and a tapered balance beam and further evaluated affect and anxiety using elevated plus maze testing.
Pink1–/– rats are notable in part for progressive motor phenotypes. For example, this strain holds important and perhaps unique translational value for recapitulating early cranial and otolaryngeal sensorimotor deficits of PD (Kelm-Nelson et al., 2021). As in PD patients (Ho et al., 1998; Miller et al., 2006a,b), Pink1–/– rats have been shown to have difficulty in sustained chewing and swallowing and show diminished vocalizing and vocalization volumes (Grant et al., 2015; Cullen et al., 2018; Kelm-Nelson et al., 2018a; Johnson et al., 2020). Other studies have shown that Pink1–/– rats also experience progressive somatic motor deficits. The most potentially concerning for the present studies are data identifying decreased novel open field locomotion and rearing, reduced hindlimb grip strength and increased commission of foot slips in traversing a tapered balance beam that in some (but not all) studies have been seen in Pink1–/– rats as young as 4 months of age (Dave et al., 2014; Grant et al., 2015). In the present study, all rats were qualitatively evaluated for ability to freely locomote within the empty testing arena during the habituation/re-habituation trials that preceded every object recognition testing block. Although several Pink1–/– rats developed what appeared to be an uncoordinated gait at around 7-month-old, all were able to navigate the relatively small testing arena used and none were excluded from object recognition testing on this basis. Additional motor assessments made in conjunction with object recognition testing also showed that during test trials Pink1–/– rats often spent more time rearing and/or ambulating than WT rats. There were also no significant ‘before or after’ group differences in measures of fore- or hindlimb grip limb strength or commissions of foot slips on a tapered balance beam showed in Pink1–/– rats. It was noted, however, that Pink1–/– rats made slightly more foot slips than WT rats, that proportionally more Pink1–/– rats committed step offs than WT rats and that 5 of the Pink1–/– rats and none of the WT controls had to be removed from tapered balance beam testing at 9.5 months of age due to difficulty in remaining on the widest portions of the beam. Thus, we did find evidence of an emergent motor phenotype in the Pink1–/– cohort. However, further, more nuanced analyses are needed to resolve its nature. In the meantime, the qualitative and quantitative data in hand argue against motor impacts in the Pink1–/– group as interfering with object exploration or object recognition testing. Importantly, the data also suggest that somatic motor deficits in Pink1–/– rats manifest later than do impairments in the cognitive and memory processes tapped in the NOR, NOL, and OiP tasks. This could signal an additional dimension of face validity for the Pink1–/– rat model, as impairments in cognition and memory typically present during prodromal phases of illness, i.e., before the onset of measurable motor deficits, in PD patients (Caviness et al., 2007; Pigott et al., 2015; Aarsland et al., 2017; Baiano et al., 2020; Fang et al., 2020).
Previous observations in Pink1–/– rats include behavioral measures suggesting increased anxiety (Kelm-Nelson et al., 2018b; Cai et al., 2019; Hoffmeister et al., 2022). Such traits are potentially relevant for modeling aspects of mood disturbance that are common in PD—including clinical cases that are causally linked to loss of function Pink1 gene mutations (Ephraty et al., 2007; Ricciardi et al., 2014). However, these traits could also adversely influence performance in object recognition testing. Specifically, while object recognition paradigms themselves are noted for provoking minimal stress or anxiety, baseline differences in anxiety can express as neophobia which reduces rats’ contact with objects–especially unfamiliar ones, and significantly erodes the discrimination indices typically used to quantify recognition memories (Ennaceur et al., 2006; Ennaceur, 2010). Among the ‘other behaviors’ measured during object recognition testing were stationary behavior and grooming. The stationary behaviors observed were distinct from freezing. Accordingly, the significantly reduced times that Pink1–/– compared to WT rats spent stationary may be most likely to reflect diminished adaptation or habituation to the testing environment. The grooming that was observed occurred intermittently and included both cephalic and sequential grooming from head to body. Thus, interpretations with respect to decreased grooming in the Pink1–/– group leave it uncertain as to whether this difference reflects decreased or increased anxiety. To gain further clarity into this, rats were also tested on an elevated plus maze. Previous studies examining rats at 4 and 12 months of age showed that Pink1–/– rats entered and spent significantly more time in closed arms than controls (Hoffmeister et al., 2021). However, in the present study, Pink1–/– rats made more entries but spent less time in the closed arms than did WT rats. Further, while neither group spent much time in the open arms, Pink1–/– rats spent significantly more time in the center platform than WT rats. Finally, Pink1–/– rats spent significantly more time rearing and/or ambulating and less time grooming in the closed arms, and significantly more time engaged in rearing and stretch-attend/scanning and less time head dipping and ambulating in the center platform. Thus, the data are mixed with respect to behaviors classically aligned with increased or decreased anxiety. While these findings provide no indication of a Pink1–/– phenotype that would be likely to compromise object recognition testing, there is no question that there are significant differences in the ways in which Pink1–/– rats govern behaviors during object recognition and elevated plus maze testing compared to WT rats. Characterizing these differences more thoroughly and resolving their bases are important areas for future investigation.
The Pink1–/– rats assessed in this study were generated on a Long Evans background. Previous studies in this rat strain have demonstrated powerful effects of intermittent sample trial object exposure on subsequent discrimination of novelty. Specifically, it was shown that multiple, shorter exposures to sample objects greatly enhanced rats’ sensitivity to novelty demonstrated in test trials compared to a single, longer exposure period (Anderson et al., 2008; Shimoda et al., 2021). These findings drove the decision to incorporate multiple sample trials (3) in the testing protocols used here. Importantly, however, all trials were time-limited and thus subject to unintended impacts of differences in the time spent gaining familiarity with sample objects on later measures of memory strength or recall. Accordingly, analyses included evaluations of any group differences in total times rats spent with objects during both sample and test trial periods. These analyses showed that the generally more active state noted above in Pink1–/– compared to WT rats included knockout rats typically spending more to significantly more time actively exploring objects in all trial types. This argues against neophobia and argues against differential exposure to samples as negatively impacting measures of DI in the gene knockout group. The latter was further supported in findings of no significant or near significant positive correlations between the durations of sample or test trial object explorations and DI for any group for any task at any age. Careful analyses of sample trial object explorations also ruled out contributions of innate spatial bias or bias toward object type(s) as contributing to the group, task and age-specific patterns of differential object exploration/discrimination seen in test trials. Rather, as discussed further below, the data in hand may be explained by deleterious consequences of knockout of the Pink1 gene for the brain circuits and neurochemical systems that mediate object recognition memory functions.
To our knowledge, there has been only one previous assessment of cognition or memory in Pink1–/– rats. This study included Barnes maze and NOR testing as part of a larger in vivo brain imaging study that examined male rats at 6–8 months of age (Cai et al., 2019). The data presented were in some cases limited. For example, because the data from Barnes maze testing were collapsed across trials, information about spatial working memory or spatial learning strategies was not available. However, measures of average daily latency to find the goal location showed no differences in performance within or across groups over four sequential testing days. Thus, rats of both genotypes appeared to learn and retain task information similarly. The latter is consistent with findings from other rodent models of PD that often do not recapitulate the long-term reference memory deficits that are characteristic of later stages of disease and PDD (Miyoshi et al., 2002; Da Cunha et al., 2006; Betancourt et al., 2016). For NOR testing, a single sample exposure (5 min) and a 60 min intertrial interval was used. While the Pink1–/– group showed no discrimination deficits, these data are difficult to interpret because–perhaps owing to the use of single sample trials, the control cohort showed no preference for novelty. Key methodological details were also lacking, including a description of habituation, information as to whether rats were tested during subjective days or nights, how object exploration was defined and measured and whether rats were tested before or after undergoing in vivo imaging. Thus, it is uncertain what may have driven the substantial differences between this prior and the present study where robust deficits in all object recognition memory domains assessed were present in Pink1–/– rats by 6–8 months of age.
The present studies used a longitudinal testing strategy to gain insights into the potentially progressive impacts of a PD-relevant gene perturbation on cognition and/or memory. This revealed diverging trajectories in object recognition memory testing performance in WT and Pink1–/– rats between 3 and 9 months of age. This was related in part to some unexpected evolutions in object recognition performance in WT rats across this span. Specifically, for NOL testing, WT rats initially showed moderate levels of discrimination that jumped to much higher, asymptotic levels by 5 months of age. Similarly, for OiP, an initially moderate level of discrimination seen in testing at 3 months of age increased, albeit more incrementally, over the next 6 months. While developmental trajectories in object recognition memory performance have been noted, these are described for much younger rats and suggest that adult levels of performance are in place within the first months of life (Reger et al., 2009; Ainge and Langston, 2012; Westbrook et al., 2014; Contreras et al., 2019). Thus, the bases for the age-to-age differences noted in the WT rats of study are unclear. Importantly, however, the generally upward trajectory of their performances indicates that WT rats continued to engage in these tasks and were not negative affected by test–retest contingencies.
Longitudinal testing showed that the Pink1–/– cohort examined developed robust discrimination deficits in NOR, NOL, and OiP tasks according to task-specific timelines. These rats continue to be tested for motor function. Thus, direct pathophysiological correlates to these behavioral profiles are not available. However, previous multimodal in vivo magnetic resonance imaging (MRI) studies in Pink1–/– rats have identified significant changes in brain regions and circuits known to be critical for object recognition memories. For example, volumetric analyses have shown that areas including perirhinal and entorhinal cortex, dentate, subicular, CA1 and CA3 fields of the hippocampal formation, nucleus reuniens of the thalamus and several amygdaloid nuclei are significantly smaller in Pink1–/– compared to WT rats (Cai et al., 2019). Diffusion weighted MRI has also identified significantly decreased anisotropy in many of these same regions and resting state functional MRI has identified significantly reduced connectivity between neostriatum, midbrain DA regions, hypothalamus and thalamus and increased connectivity between ventral midbrain DA regions and hippocampus in Pink1–/– compared to wild type rats (Ferris et al., 2018; Cai et al., 2019). Together these findings show that many of the brain regions and networks known to be critical for object recognition memory (Aggleton and Nelson, 2020; Barker and Warburton, 2020a,b; Chao et al., 2020) are vulnerable to the Pink1–/– genotype. In addition, although findings with respect to DA cell body loss have been variable (de Haas et al., 2019), NE cell loss, increased neostriatal concentrations of DA and decreased levels of basal and potassium-stimulated neostriatal release of DA, ACh and others have also been identified in Pink1–/– compared to control rats between the ages of 4 and 12 months (Dave et al., 2014; Grant et al., 2015; Villeneuve et al., 2016; Cullen et al., 2018; Creed et al., 2019). Although little is currently known about the status of neurochemistry in other subcortical or cortical regions, these data nonetheless show patterns of dysregulation induced by the Pink1–/– genotype that involve neurotransmitters known to play pivotal roles in object recognition memories (Dere et al., 2007; Bus et al., 2020). Further, all of the indices of pathophysiology described above are present in Pink1–/– rats over time frames when the results of this study predict that significant impairments in multiple object recognition memory domains would be present. Future studies that combine in vivo imaging with behavioral analyses may be in an especially powerful position to map the progression of brain pathophysiology to the evolution of domain specific object recognition memory deficits. Although MRI analyses can be brain wide, current understanding of the points of overlap and divergence among the neural systems that underlie performance in discrete object recognition memory tasks can be used to generate and/or prioritize narrower, more specific hypotheses to be tested by these means.
Novel object recognition, NOL and OiP testing continues to be extensively used to evaluate recognition memory and visuospatial information processing deficits that are similar to those experienced by PD patients in a range of different preclinical rodent models of disease (Grayson et al., 2015; Haghparast et al., 2018; Kyser et al., 2019; Bharatiya et al., 2020; Boi et al., 2020; Kakoty et al., 2021). The present studies identified robust deficits in all three of these tasks in Pink1–/– rats. This is the first demonstration of face validity in this model for commonly occurring cognitive and memory impairments associated with PD. The longitudinal testing scheme used along with companion assessments of motor and affective function also showed that object recognition memory deficits in Pink1–/– rats progressively worsen and precede the onset of potentially confounding motor signs. The need for treatments that prevent or slow the course of cognitive or memory decline in PD– and especially those that do so without interfering with treatment of motor signs, is urgent (Goldman and Weintraub, 2015; Goldman et al., 2018). The present findings of progressive cognitive and memory deficits along with the emergence of motor signs identify Pink1–/– rats as well suited for accelerating the pace discovery needed to fill this therapeutic gap. Key directions for future investigations using this model include assessments of long-term object recognition user longer, e.g., 24 h delay periods, evaluation of additional at-risk behavioral domains including executive function and exploration of potential face validity of Pink1–/– rats for the sex differences that characterize the incidence and severity of mild cognitive impairments in PD (Janvin et al., 2006; Cereda et al., 2016; Liu et al., 2017; Cholerton et al., 2018; Oltra et al., 2021). The benefits of continued use of object recognition memory tasks for these purposes include their proven utility for evaluating sex and sex hormone impacts in rodent models of PD (Luine, 2015; Costa et al., 2020; Lima et al., 2021; Pinizzotto et al., 2022). This along with the undisputed value of these tasks in identifying candidate neural substrates (Dere et al., 2007; Brown et al., 2012; Aggleton and Nelson, 2020; Barker and Warburton, 2020b; Chao et al., 2020) could ultimately help resolve points of common pathophysiological ground that render object recognition memories vulnerable not only in PD but also in other neurodegenerative disorders including Alzheimer’s disease and schizophrenia (Grayson et al., 2015).
The raw data supporting the conclusions of this article will be made available by the authors, without undue reservation.
This animal study was reviewed and approved by IACUC, Stony Brook University.
CP: participated in all phases of animal testing, data collection, and data analyses, assisted co-authors in their responsibilities and primary responsibilities for creating figures and assisted in writing the manuscript. KD: primary responsibility for data analysis for Elevated Plus Maze testing, assisted in creating figures and writing of the manuscript. OA: primary responsibility for data analysis for Novel Object Location testing, assisted in creating figures and writing of the manuscript. RC: primary responsibility for behavioral testing and data analysis for Grip Strength and Tapered Balance Beam testing, assisted in creating figures and writing of the manuscript. KM: assisted in data analysis for Novel Object Recognition testing, assisted in creating figures and writing of the manuscript. MK: assisted in all phases of animal testing, data collection, data analyses, and creating figures, primary responsibility for writing the manuscript. All authors contributed to the article and approved the submitted version.
This work was supported by a Pilot Award from the Thomas Hartman Parkinson’s Research Center (to MK) and by a grant from the National Institutes of Health (R21 NS11000 to MK).
The authors declare that the research was conducted in the absence of any commercial or financial relationships that could be construed as a potential conflict of interest.
All claims expressed in this article are solely those of the authors and do not necessarily represent those of their affiliated organizations, or those of the publisher, the editors and the reviewers. Any product that may be evaluated in this article, or claim that may be made by its manufacturer, is not guaranteed or endorsed by the publisher.
The Supplementary Material for this article can be found online at: https://www.frontiersin.org/articles/10.3389/fnbeh.2022.951268/full#supplementary-material
Aarsland, D., Bronnick, K., Williams-Gray, C., Weintraub, D., Marder, K., Kulisevsky, J., et al. (2010). Mild cognitive impairment in Parkinson disease: a multicenter pooledanalysis. Neurology 75, 1062–1069. doi: 10.1212/WNL.0b013e3181f39d0e
Aarsland, D., Creese, B., Politis, M., Chaudhuri, K. R., Ffytche, D. H., Weintraub, D., et al. (2017). Cognitive decline in Parkinson disease. Nat. Rev. Neurol. 13, 217–231. doi: 10.1038/nrneurol.2017.27
Aggleton, J. P., and Nelson, A. J. D. (2020). Distributed interactive brain circuits for object-in- place memory: A place for time? Brain Neurosci. Adv. 4:2398212820933471. doi: 10.1177/2398212820933471
Ainge, J. A., and Langston, R. F. (2012). Ontogeny of neural circuits underlying spatial memoryin the rat. Front Neural Circuits 6, 8. doi: 10.3389/fncir.2012.00008
Anderson, M. J., Jablonski, S. A., and Klimas, D. B. (2008). Spaced initial stimulus familiarization enhances novelty preference in Long-Evans rats. Behav. Processes 78, 481–486. doi: 10.1016/j.beproc.2008.02.005
Baiano, C., Barone, P., Trojano, L., and Santangelo, G. (2020). Prevalence and clinical aspects of mild cognitive impairment in Parkinson’s disease: A meta-analysis. Mov. Dis. 35, 45–54. doi: 10.1002/mds.27902
Barker, G. R., and Warburton, E. C. (2020a). Multi-level analyses of associative recognition memory: the whole is greater than the sum of its parts. Curr. Opin. Behav. Sci. 32, 80–87. doi: 10.1016/j.cobeha.2020.02.004
Barker, G. R. I., and Warburton, E. C. (2020b). Putting objects in context: A prefrontal- hippocampal-perirhinal cortex network. Brain Neurosci. Adv. 4, 2398212820937621. doi: 10.1177/2398212820937621
Barone, P., Erro, R., and Picillo, M. (2017). Quality of Life and Non-motor Symptoms in Parkinson’s Disease. Int. Rev. Neurobiol. 133, 499–516. doi: 10.1016/bs.irn.2017.05.023
Betancourt, E., Wachtel, J., Michaelos, M., Haggerty, M., Conforti, J., and Kritzer, M. F. (2016). The impact of biological sex and sex hormones on cognition in a rat model of early, pre-motor Parkinson’s disease. Neuroscience 345, 297–314. doi: 10.1016/j.neuroscience.2016.05.041
Bharatiya, R., Bratzu, J., Lobina, C., Corda, G., Cocco, C., De Deurwaerdere, P., et al. (2020). The pesticide fipronil injected into the substantia nigra of male rats decreases striatal dopamine content: A neurochemical, immunohistochemical and behavioral study. Behav. Brain Res. 384:112562. doi: 10.1016/j.bbr.2020.112562
Bloem, B. R., Okun, M. S., and Klein, C. (2021). Parkinson’s disease. Lancet 397, 2284–2303. doi: 10.1016/S0140-6736(21)00218-X
Boi, L., Pisanu, A., Palmas, M. F., Fusco, G., Carboni, E., Casu, M. A., et al. (2020). Modeling Parkinson’s Disease Neuropathology and Symptoms by Intranigral Inoculation of Preformed Human alpha-Synuclein Oligomers. Int. J. Mol. Sci. 21:8535. doi: 10.3390/ijms21228535
Borsche, M., Konig, I. R., Delcambre, S., Petrucci, S., Balck, A., Bruggemann, N., et al. (2020). Mitochondrial damage-associated inflammation highlights biomarkers in PRKN/PINK1 parkinsonism. Brain 143, 3041–3051. doi: 10.1093/brain/awaa246
Brown, M. W., Barker, G. R., Aggleton, J. P., and Warburton, E. C. (2012). What pharmacologicalinterventions indicate concerning the role of the perirhinal cortex in recognition memory. Neuropsychologia 50, 3122–3140. doi: 10.1016/j.neuropsychologia.2012.07.034
Bus, C., Zizmare, L., Feldkaemper, M., Geisler, S., Zarani, M., Schaedler, A., et al. (2020). Human Dopaminergic Neurons Lacking PINK1 Exhibit Disrupted Dopamine Metabolism Related to Vitamin B6 Co-Factors. iScience. 23, 101797. doi: 10.1016/j.isci.2020.101797
Cai, X., Qiao, J., Knox, T., Iriah, S., Kulkarni, P., Madularu, D., et al. (2019). In search ofearly neuroradiological biomarkers for Parkinson’s Disease: Alterations in restingstate functional connectivity and gray matter microarchitecture in PINK1 –/– rats. Brain Res. 1706, 58–67. doi: 10.1016/j.brainres.2018.10.033
Caviness, J. N., Driver-Dunckley, E., Connor, D. J., Sabbagh, M. N., Hentz, J. G., Noble, B., et al. (2007). Defining mild cognitive impairment in Parkinson’s disease. Mov. Disord. 22, 1272–1277. doi: 10.1002/mds.21453
Cereda, E., Cilia, R., Klersy, C., Siri, C., Pozzi, B., Reali, E., et al. (2016). Dementia in Parkinson’s disease: Is male gender a risk factor? Parkinsonism Relat. Disord. 26, 67–72. doi: 10.1016/j.parkreldis.2016.02.024
Chao, O. Y., de Souza Silva, M. A., Yang, Y. M., and Huston, J. P. (2020). The medial prefrontal cortex – hippocampus circuit that integrates information of object, place and time to construct episodic memory in rodents: Behavioral, anatomical and neurochemical properties. Neurosci. Biobehav. Rev. 113, 373–407. doi: 10.1016/j.neubiorev.2020.04.007
Cholerton, B., Johnson, C. O., Fish, B., Quinn, J. F., Chung, K. A., Peterson-Hiller, A. L., et al. (2018). Sex differences in progression to mild cognitive impairment and dementia in Parkinson’s disease. Parkinsonism Relat. Disord. 50, 29–36. doi: 10.1016/j.parkreldis.2018.02.007
Contreras, M. P., Born, J., and Inostroza, M. (2019). The expression of allocentric object-placerecognition memory during development. Behav. Brain Res. 372:112013. doi: 10.1016/j.bbr.2019.112013
Costa, G., Sisalli, M. J., Simola, N., Della Notte, S., Casu, M. A., Serra, M., et al. (2020). Gender Differences in Neurodegeneration, Neuroinflammation and Na(+)-Ca(2 +)Exchangers in the Female A53T Transgenic Mouse Model of Parkinson’s Disease. Front. Aging Neurosci. 12:118. doi: 10.3389/fnagi.2020.00118
Creed, R. B., and Goldberg, M. S. (2018). Analysis of alpha-Synuclein Pathology in PINK1 Knockout Rat Brains. Front. Neurosci. 12, 1034. doi: 10.3389/fnins.2018.01034
Creed, R. B., Menalled, L., Casey, B., Dave, K. D., Janssens, H. B., Veinbergs, I., et al. (2019). Basal and Evoked Neurotransmitter Levels in Parkin, DJ-1, PINK1 andLRRK2 Knockout Rat Striatum. Neuroscience 409, 169–179. doi: 10.1016/j.neuroscience.2019.04.033
Cullen, K. P., Grant, L. M., Kelm-Nelson, C. A., Brauer, A. F. L., Bickelhaupt, L. B., Russell, J. A., et al. (2018). Pink1 –/– Rats Show Early-Onset Swallowing Deficits and Correlative Brainstem Pathology. Dysphagia 33, 749–758. doi: 10.1007/s00455-018-9896-5
Da Cunha, C., Silva, M. H., Wietzikoski, S., Wietzikoski, E. C., Ferro, M. M., Kouzmine, I., et al. (2006). Place learning strategy of substantia nigra pars compacta-lesioned rats. Behav. Neurosci. 120, 1279–1284. doi: 10.1037/0735-7044.120.6.1279
Dave, K. D., De Silva, S., Sheth, N. P., Ramboz, S., Beck, M. J., Quang, C., et al. (2014). Phenotypic characterization of recessive gene knockout rat models of Parkinson’sdisease. Neurobiol 70, 190–203. doi: 10.1016/j.nbd.2014.06.009
de Haas, R., Heltzel, L., Tax, D., van den Broek, P., Steenbreker, H., Verheij, M. M. M., et al. (2019). To be or not to be pink(1): contradictory findings in an animal model for Parkinson’s disease. Brain Commun. 1:fcz016. doi: 10.1093/braincomms/fcz016
Dere, E., Huston, J. P., and De Souza Silva, M. A. (2007). The pharmacology, neuroanatomy and neurogenetics of one-trial object recognition in rodents. Neurosci. Biobehav. Rev. 31, 673–704. doi: 10.1016/j.neubiorev.2007.01.005
Ennaceur, A. (2010). One-trial object recognition in rats and mice: methodological and theoretical issues. Behav. Brain Res. 215, 244–254. doi: 10.1016/j.bbr.2009.12.036
Ennaceur, A., Michalikova, S., and Chazot, P. L. (2006). Models of anxiety: responses of rats to novelty in an open space and an enclosed space. Behav. Brain Res. 171, 26–49. doi: 10.1016/j.bbr.2006.03.016
Ennaceur, A., Michalikova, S., and Chazot, P. L. (2009). Do rats really express neophobia towards novel objects? Experimental evidence from exposure to novelty and to an object recognition task in an open space and an enclosed space. Behav. Brain Res. 197, 417–434. doi: 10.1016/j.bbr.2008.10.007
Ennaceur, A., Michalikova, S., Bradford, A., and Ahmed, S. (2005). Detailed analysis of the behavior of Lister and Wistar rats in anxiety, object recognition and object location tasks. Behav. Brain Res. 159, 247–266. doi: 10.1016/j.bbr.2004.11.006
Ephraty, L., Porat, O., Israeli, D., Cohen, O. S., Tunkel, O., Yael, S., et al. (2007). Neuropsychiatric and cognitive features in autosomal-recessive early parkinsonism due to PINK1 mutations. Mov. Disord. 22, 566–569. doi: 10.1002/mds.21319
Fan, Y., Han, J., Zhao, L., Wu, C., Wu, P., Huang, Z., et al. (2021). Experimental Models of Cognitive Impairment for Use in Parkinson’s Disease Research: The Distance Between Reality and Ideal. Front. Aging Neurosci. 13, 745438. doi: 10.3389/fnagi.2021.745438
Fang, C., Lv, L., Mao, S., Dong, H., and Liu, B. (2020). Cognition Deficits in Parkinson’s Disease: Mechanisms and Treatment. Parkinsons Dis. 2020:2076942.
Fernandez-Baizan, C., Paula Fernandez Garcia, M., Diaz-Caceres, E., Menendez-Gonzalez, M., Arias, J. L., and Mendez, M. (2020). Patients with Parkinson’s Disease ShowAlteration in their Visuospatial Abilities and in their Egocentric and Allocentric SpatialOrientation Measured by Card Placing Tests. J. Parkinsons Dis. 10, 1807–1816. doi: 10.3233/JPD-202122
Ferris, C. F., Morrison, T. R., Iriah, S., Malmberg, S., Kulkarni, P., Hartner, J. C., et al. (2018). Evidence of Neurobiological Changes in the Presymptomatic PINK1 Knockout Rat. J. Parkinsons Dis. 8, 281–301. doi: 10.3233/JPD-171273
Gautier, C. A., Kitada, T., and Shen, J. (2008). Loss of PINK1 causes mitochondrial functional defects and increased sensitivity to oxidative stress. Proc. Natl. Acad. Sci. U.S.A 105, 11364–11369. doi: 10.1073/pnas.0802076105
Goldman, J. G., and Weintraub, D. (2015). Advances in the treatment of cognitive impairment in Parkinson’s disease. Mov. Disord. 30, 1471–1489. doi: 10.1002/mds.26352
Goldman, J. G., Vernaleo, B. A., Camicioli, R., Dahodwala, N., Dobkin, R. D., Ellis, T., et al. (2018). Cognitive impairment in Parkinson’s disease: a report from a multidisciplinary symposium on unmet needs and future directions to maintain cognitive health. NPJ Parkinsons Dis. 4:19. doi: 10.1038/s41531-018-0055-3
Goncalves, F. B., and Morais, V. A. (2021). PINK1: A Bridge between Mitochondria and Parkinson’s Disease. Life 11:371. doi: 10.3390/life11050371
Gonzalez-Latapi, P., Bayram, E., Litvan, I., and Marras, C. (2021). Cognitive Impairment in Parkinson’s Disease: Epidemiology, Clinical Profile, Protective and Risk Factors. Behav. Sci. 11:74. doi: 10.3390/bs11050074
Grant, L. M., Kelm-Nelson, C. A., Hilby, B. L., Blue, K. V., Paul Rajamanickam, E. S., Pultorak, J. D., et al. (2015). Evidence for early and progressive ultrasonic vocalizationand oromotor deficits in a PINK1 gene knockout rat model of Parkinson’s disease. J. Neurosci. Res. 93, 1713–1727. doi: 10.1002/jnr.23625
Grayson, B., Leger, M., Piercy, C., Adamson, L., Harte, M., and Neill, J. C. (2015). Assessment ofdisease-related cognitive impairments using the novel object recognition (NOR) task in rodents. Behav. Brain Res. 285, 176–193. doi: 10.1016/j.bbr.2014.10.025
Hagenah, J. M., Becker, B., Bruggemann, N., Djarmati, A., Lohmann, K., Sprenger, A., et al. (2008). Transcranial sonography findings in a large family with homozygous and heterozygous PINK1 mutations. J. Neurol. Neurosurg. Psychiatry 79, 1071–1074. doi: 10.1136/jnnp.2007.142174
Haghparast, E., Esmaeili-Mahani, S., Abbasnejad, M., and Sheibani, V. (2018). Apelin-13 ameliorates cognitive impairments in 6-hydroxydopamine-induced substantia nigra lesion in rats. Neuropeptides 68, 28–35. doi: 10.1016/j.npep.2018.01.001
Higginson, C. I., Wheelock, V. L., Carroll, K. E., and Sigvardt, K. A. (2005). Recognition memory in Parkinson’s disease with and without dementia: evidence inconsistent with the retrieval deficit hypothesis. J. Clin. Exp. Neuropsychol. 27, 516–528. doi: 10.1080/13803390490515469
Ho, A. K., Iansek, R., Marigliani, C., Bradshaw, J. L., and Gates, S. (1998). Speech impairment in a large sample of patients with Parkinson’s disease. Behav. Neurol. 11, 131–137. doi: 10.1155/1999/327643
Hoffmeister, J. D., Kelm-Nelson, C. A., and Ciucci, M. R. (2021). Quantification of brainstem norepinephrine relative to vocal impairment and anxiety in the Pink1–/– rat model of Parkinson disease. Behav. Brain Res. 414:113514. doi: 10.1016/j.bbr.2021.113514
Hoffmeister, J. D., Kelm-Nelson, C. A., and Ciucci, M. R. (2022). Manipulation of vocalcommunication and anxiety through pharmacologic modulation of norepinephrine inthe Pink1–/– rat model of Parkinson disease. Behav. Brain Res. 418:113642. doi: 10.1016/j.bbr.2021.113642
Ikram, H., and Haleem, D. J. (2019). Repeated treatment with a low dose of reserpine as a progressive model of Parkinson’s dementia.. Pak. J. Pharm. Sci. 32, 555–562.
Janvin, C. C., Larsen, J. P., Aarsland, D., and Hugdahl, K. (2006). Subtypes of mild cognitive impairment in Parkinson’s disease: progression to dementia. Mov. Disord. 21, 1343–1349. doi: 10.1002/mds.20974
Johnson, M. E., and Bobrovskaya, L. (2015). An update on the rotenone models of Parkinson’sdisease: their ability to reproduce the features of clinical disease and model gene-environment interactions. Neurotoxicology 46, 101–116. doi: 10.1016/j.neuro.2014.12.002
Johnson, R. A., Kelm-Nelson, C. A., and Ciucci, M. R. (2020). Changes to Ventilation, Vocalization, and Thermal Nociception in the Pink1–/– Rat Model of Parkinson’s Disease. J. Parkinsons Dis. 10, 489–504. doi: 10.3233/JPD-191853
Kakoty, V., Sk, C., Yang, C. H., Kumari, S., Dubey, S. K., and Taliyan, R. (2021). Neuroprotective Effects of Trehalose and Sodium Butyrate on Preformed Fibrillar Form of alpha-Synuclein- Induced Rat Model of Parkinson’s Disease. ACS Chem. Neurosci. 12, 2643–2660. doi: 10.1021/acschemneuro.1c00144
Kelm-Nelson, C. A., Brauer, A. F. L., Barth, K. J., Lake, J. M., Sinnen, M. L. K., Stehula, F. J., et al. (2018a). Characterization of early-onset motor deficits in the Pink1–/– mousemodel of Parkinson disease. Brain Res. 1680, 1–12. doi: 10.1016/j.brainres.2017.12.002
Kelm-Nelson, C. A., Trevino, M. A., and Ciucci, M. R. (2018b). Quantitative Analysis ofCatecholamines in the Pink1 –/– Rat Model of Early-onset Parkinson’s Disease. Neuroscience 379, 126–141. doi: 10.1016/j.neuroscience.2018.02.027
Kelm-Nelson, C. A., Lechner, S. A., Lettenberger, S. E., Kaldenberg, T. A. R., Pahapill, N. K., Regenbaum, A., et al. (2021). Pink1(–/–) rats are a useful tool to study earlyParkinson disease. Brain Commun. 3:fcab077. doi: 10.1093/braincomms/fcab077
Kudlicka, A., Clare, L., and Hindle, J. V. (2014). Quality of life, health status and caregiver burdenin Parkinson’s disease: relationship to executive functioning. Int. J. Geriatr. Psychiatry 29, 68–76. doi: 10.1002/gps.3970
Kumazawa, R., Tomiyama, H., Li, Y., Imamichi, Y., Funayama, M., Yoshino, H., et al. (2008). Mutation analysis of the PINK1 gene in 391 patients with Parkinson disease. Arch. Neurol. 65, 802–808. doi: 10.1001/archneur.65.6.802
Kyser, T. L., Dourson, A. J., McGuire, J. L., Hemmerle, A. M., Williams, M. T., and Seroogy, K. B. (2019). Characterization of Motor and Non-Motor Behavioral Alterations in the Dj-1 (PARK7) Knockout Rat. J. Mol. Neurosci. 69, 298–311. doi: 10.1007/s12031-019-01358-0
Leroi, I., McDonald, K., Pantula, H., and Harbishettar, V. (2012). Cognitive impairment in Parkinson disease: impact on quality of life, disability, and caregiver burden. J. Geriatr. Psychiatry Neurol. 25, 208–214. doi: 10.1177/0891988712464823
Lima, A. C., Meurer, Y. S. R., Bioni, V. S., Cunha, D. M. G., Goncalves, N., Lopes-Silva, L. B., et al. (2021). Female Rats Are Resistant to Cognitive, Motor and Dopaminergic Deficits in the Reserpine-Induced Progressive Model of Parkinson’s Disease. Front. Aging Neurosci. 13, 757714. doi: 10.3389/fnagi.2021.757714
Liu, G., Locascio, J. J., Corvol, J. C., Boot, B., Liao, Z., and Page, K. (2017). Prediction of cognition in Parkinson’s disease with a clinical-genetic score: a longitudinal analysis of nine cohorts. Lancet Neurol. 16, 620–629. doi: 10.1016/S1474-4422(17)30122-9
Samaranch, L., Lorenzo-Betancor, O., Arbelo, J. M., Ferrer, I., Lorenzo, E., and Irigoyen, J. (2010). PINK1-linked parkinsonism is associated with Lewy body pathology. Brain 133, 1128–1142. doi: 10.1093/brain/awq051
Luine, V. (2015). Recognition memory tasks in neuroendocrine research. Behav. Brain Res. 285, 158–164. doi: 10.1016/j.bbr.2014.04.032
Mack, J., and Marsh, L. (2017). Parkinson’s Disease: Cognitive Impairment. Focus 15, 42–54. doi: 10.1176/appi.focus.20160043
Miller, N., Noble, E., Jones, D., and Burn, D. (2006a). Hard to swallow: dysphagia in Parkinson’s disease. Age Ageing 35, 614–618. doi: 10.1093/ageing/afl105
Miller, N., Noble, E., Jones, D., and Burn, D. (2006b). Life with communication changes in Parkinson’s disease. Age Ageing 35, 235–239. doi: 10.1093/ageing/afj053
Miyoshi, E., Wietzikoski, S., Camplessei, M., Silveira, R., Takahashi, R. N., and Da Cunha, C. (2002). Impaired learning in a spatial working memory version and in a cued version of the water maze in rats with MPTP-induced mesencephalic dopaminergic lesions. Brain Res. Bull. 58, 41–47. doi: 10.1016/s0361-9230(02)00754-2
Nybo, C. J., Gustavsson, E. K., Farrer, M. J., and Aasly, J. O. (2020). Neuropathological findings inPINK1-associated Parkinson’s disease. Parkinsonism Relat. Disord. 78, 105–108. doi: 10.1016/j.parkreldis.2020.07.023
Oltra, J., Segura, B., Uribe, C., Monte-Rubio, G. C., Campabadal, A., Inguanzo, A., et al. (2021). Sex differences in brain atrophy and cognitive impairment in Parkinson’sdisease patients with and without probable rapid eye movement sleep behavior disorder. J. Neurol. 269, 1591–1599. doi: 10.1007/s00415-021-10728-x
Oosterveld, L. P., Allen, J. C. Jr., Reinoso, G., Seah, S. H., Tay, K. Y., Au, W. L., et al. (2015). Prognostic factors for early mortality in Parkinson’s disease. Parkinsonism Relat. Disord. 21, 226–230.
Owen, A. M., Beksinska, M., James, M., Leigh, P. N., Summers, B. A., Marsden, C. D., et al. (1993). Visuospatial memory deficits at different stages of Parkinson’s disease. Neuropsychologia 31, 627–644.
Papagno, C., and Trojano, L. (2018). Cognitive and behavioral disorders in Parkinson’s disease: an update. I: cognitive impairments. Neurol. Sci. 39, 215–223.
Peterson, D. S., King, L. A., Cohen, R. G., and Horak, F. B. (2016). Cognitive Contributions to Freezing of Gait in Parkinson Disease: Implications for Physical Rehabilitation. Phys. Ther. 96, 659–670.
Pigott, K., Rick, J., Xie, S. X., Hurtig, H., Chen-Plotkin, A., Duda, J. E., et al. (2015). Longitudinal study of normal cognition in Parkinson disease. Neurology 85, 1276–1282.
Pinizzotto, C. C., Patwardhan, A., Aldarondo, D., and Kritzer, M. F. (2022). Task-specific effects ofbiological sex and sex hormones on object recognition memories in a 6-hydroxydopamine-lesion model of Parkinson’s disease in adult male and female rats. Horm. Behav. 144:105206. doi: 10.1016/j.yhbeh.2022.105206
Piredda, R., Desmarais, P., Masellis, M., and Gasca-Salas, C. (2020). Cognitive and psychiatric symptoms in genetically determined Parkinson’s disease: a systematic review. Eur. J. Neurol. 27, 229–234.
Possin, K. L., Filoteo, J. V., Song, D. D., and Salmon, D. P. (2008). Spatial and object working memory deficits in Parkinson’s disease are due to impairment in different underlying processes. Neuropsychology 22, 585–595.
Reger, M. L., Hovda, D. A., and Giza, C. C. (2009). Ontogeny of Rat Recognition Memory measured by the novel object recognition task. Dev. Psychobiol. 51, 672–678.
Ren, X., and Butterfield, D. A. (2021). Fidelity of the PINK1 knockout rat to oxidative stress and other characteristics of Parkinson disease. Free Radic. Biol. Med. 163, 88–101. doi: 10.1016/j.freeradbiomed.2020.12.004
Ricciardi, L., Petrucci, S., Guidubaldi, A., Ialongo, T., Serra, L., Ferraris, A., et al. (2014). Phenotypic variability of PINK1 expression: 12 Years’ clinical follow-up oftwo Italian families. Mov. Disord. 29, 1561–1566. doi: 10.1002/mds.25994
Rodriguez-Blazquez, C., Forjaz, M. J., Lizan, L., Paz, S., and Martinez-Martin, P. (2015). Estimating the direct and indirect costs associated with Parkinson’s disease. Expert Rev. Pharmacoecon. Outcomes Res. 15, 889–911.
Saredakis, D., Collins-Praino, L. E., Gutteridge, D. S., Stephan, B. C. M., and Keage, H. A. D. (2019). Conversion to MCI and dementia in Parkinson’s disease: a systematic review and meta-analysis. Parkinsonism Relat. Disord. 65, 20–31.
Scarffe, L. A., Stevens, D. A., Dawson, V. L., and Dawson, T. M. (2014). Parkin and PINK1: much more than mitophagy. Trends Neurosci. 37, 315–324.
Shimoda, S., Ozawa, T., Ichitani, Y., and Yamada, K. (2021). Long-term associative memory in rats: Effects of familiarization period in object-place-context recognition test. PLoS One 16:e0254570. doi: 10.1371/journal.pone.0254570
Takanashi, M., Li, Y., and Hattori, N. (2016). Absence of Lewy pathology associated with PINK1 homozygous mutation. Neurology 86, 2212–2213. doi: 10.1212/WNL.0000000000002744
Urrutia, P. J., Mena, N. P., and Nunez, M. T. (2014). The interplay between iron accumulation, mitochondrial dysfunction, and inflammation during the execution step of neurodegenerative disorders. Front. Pharmacol. 5:38. doi: 10.3389/fphar.2014.00038
Valente, E. M., Abou-Sleiman, P. M., Caputo, V., Muqit, M. M., Harvey, K., Gispert, S., et al. (2004). Hereditary early-onset Parkinson’s disease caused by mutations in PINK1. Science 304, 1158–1160.
Vazquez-Velez, G. E., and Zoghbi, H. Y. (2021). Parkinson’s Disease Genetics and Pathophysiology. Annu. Rev. Neurosci. 44, 87–108.
Villeneuve, L. M., Purnell, P. R., Boska, M. D., and Fox, H. S. (2016). Early Expression of Parkinson’s Disease-Related Mitochondrial Abnormalities in PINK1 Knockout Rats. Mol. Neurobiol. 53, 171–186. doi: 10.1007/s12035-014-8927-y
Keywords: cognition, PTEN-induced putative kinase1, spatial memory, perirhinal cortex, hippocampus
Citation: Pinizzotto CC, Dreyer KM, Aje OA, Caffrey RM, Madhira K and Kritzer MF (2022) Spontaneous Object Exploration in a Recessive Gene Knockout Model of Parkinson’s Disease: Development and Progression of Object Recognition Memory Deficits in Male Pink1–/– Rats. Front. Behav. Neurosci. 16:951268. doi: 10.3389/fnbeh.2022.951268
Received: 23 May 2022; Accepted: 20 June 2022;
Published: 06 December 2022.
Edited by:
Flávio F. Barbosa, Federal University of Paraíba, BrazilReviewed by:
Marta Méndez, University of Oviedo, SpainCopyright © 2022 Pinizzotto, Dreyer, Aje, Caffrey, Madhira and Kritzer. This is an open-access article distributed under the terms of the Creative Commons Attribution License (CC BY). The use, distribution or reproduction in other forums is permitted, provided the original author(s) and the copyright owner(s) are credited and that the original publication in this journal is cited, in accordance with accepted academic practice. No use, distribution or reproduction is permitted which does not comply with these terms.
*Correspondence: Claudia C. Pinizzotto, Q2xhdWRpYS5waW5penpvdHRvQHN0b255YnJvb2suZWR1
Disclaimer: All claims expressed in this article are solely those of the authors and do not necessarily represent those of their affiliated organizations, or those of the publisher, the editors and the reviewers. Any product that may be evaluated in this article or claim that may be made by its manufacturer is not guaranteed or endorsed by the publisher.
Research integrity at Frontiers
Learn more about the work of our research integrity team to safeguard the quality of each article we publish.