- 1Department of Biology, Waseda University, Tokyo, Japan
- 2Laboratory of Biochemistry, Faculty of Pharmaceutical Sciences, Tokushima Bunri University, Tokushima, Japan
- 3Koltzov Institute of Developmental Biology, Russian Academy of Sciences, Moscow, Russia
- 4Graduate Institute of Medicine, School of Medicine, Kaohsiung Medical University, Kaohsiung, Taiwan
Insulin and insulin-like peptides (ILP) help to maintain glucose homeostasis, whereas insulin-like growth factor (IGF) promotes the growth and differentiation of cells in both vertebrates and invertebrates. It is sometimes difficult to distinguish between ILP and IGF in invertebrates, however, because in some cases ILP has the same function as IGF. In the present review, therefore, we refer to these peptides as ILP/IGF signaling (IIS) in invertebrates, and discuss the role of IIS in memory formation after classical conditioning in invertebrates. In the arthropod Drosophila melanogaster, IIS is involved in aversive olfactory memory, and in the nematode Caenorhabditis elegans, IIS controls appetitive/aversive response to NaCl depending on the duration of starvation. In the mollusk Lymnaea stagnalis, IIS has a critical role in conditioned taste aversion. Insulin in mammals is also known to play an important role in cognitive function, and many studies in humans have focused on insulin as a potential treatment for Alzheimer’s disease. Although analyses of tissue and cellular levels have progressed in mammals, the molecular mechanisms, such as transcriptional and translational levels, of IIS function in cognition have been far advanced in studies using invertebrates. We anticipate that the present review will help to pave the way for studying the effects of insulin, ILPs, and IGFs in cognitive function across phyla.
Introduction
In 1988, a News and Views article, titled “Invertebrate neuroendocrinology. Insulin found at last?”, was published in Nature (Thorpe and Duve, 1988). This article introduced another report published in the same issue by Smit and colleagues from Vrije Universiteit, Amsterdam, describing the discovery of an insulin-related peptide in the central nervous system (CNS) of the gastropod mollusk Lymnaea stagnalis with convincing evidence that the functionally important peptide structure was conserved through a long period of evolution (Smit et al., 1988). The sequence data of this insulin-related peptide showed the presence of cysteines at positions in the A and B chains typical for the insulin family, suggesting the formation of an insulin-like tertiary structure with conserved (or alternative) hydrophobic core residues.
The background regarding the “first” insulin discovered in invertebrates, however, is somewhat complicated. In 1986 (2 years ahead of the study by Smit et al., 1988), Nagasawa et al. (1986) determined the complete amino acid sequence of 4K-PTTH-II, one of the three forms of the prothoracicotropic hormone, i.e., insulin-like peptide (ILP), of the silkworm Bombyx mori. 4K-PTTH-II is made up of two nonidentical peptide chains (A and B chains). This peptide has considerable sequence homology (40%) with human insulin, and the identical distribution of the six cysteine residues also indicates that 4K-PTTH-II belongs to the “insulin family”. Therefore, the definitive structural information on ILPs was obtained in Bombyx mori in 1986, but the first ILP DNA sequence was identified in Lymnaea stagnalis in 1988. In any case, these two studies together accelerated the functional analysis of ILPs in invertebrates (Ebberink et al., 1989).
In both vertebrates and invertebrates, not only do ILPs, including insulin, and insulin-like growth factors (IGFs) have similar structures (Blundell et al., 1978; Rinderknecht and Humbel, 1978; Smit et al., 1998; Brogiolo et al., 2001; Pierce et al., 2001; Li et al., 2003; Garelli et al., 2012), but the receptors to which they bind also have similar structures (Fernandez et al., 1995; Roovers et al., 1995; Kimura et al., 1997). The receptors for ILP and IGF have a structure that penetrates the cell membrane, with a binding site for ILP and IGF on the outside of the cell membrane. The receptor is a preformed, disulfide-bridged dimer. The ligand binding is thought to induce a conformational change in the receptor that initiates trans-phosphorylation of the two cytoplasmic domains and induces tyrosine kinase activity, thereby phosphorylating various substrates in the cell, which triggers downstream signals that result in a cellular response. With regard to insulin in mammals, the intracellular signaling mechanism by which the metabolism of glucose is regulated is well clarified (Saltiel and Kahn, 2001). IGF signals to cells that sufficient nutrients are available for cells to undergo hypertrophy and cell division, and these signals inhibit cell apoptosis and increase the production of cellular proteins (Shimizu, 2021). Although the signal transduction of IGF has been elucidated in terms of its commonalities with insulin, little is known about how the long-lasting cellular response caused by IGF is triggered (Andoh, 2021).
In invertebrates, many ILPs have also specific growth-promoting roles (Kenyon, 2010). For example, Lymnaea ILP, named “molluscan insulin-related peptide (MIP)”, was first identified as the product of cells noted for their role in controlling growth in invertebrates (Smit et al., 1988). Lymnaea MIPs also have a role in reducing the hemolymph glucose level (Mita et al., 2014a). It is therefore sometimes difficult to discriminate between invertebrate ILPs and IGFs. In the present article, we refer to ILP and IGF together as “ILP/IGF signaling (IIS)” in invertebrates.
IIS plays an important role in cognitive function in both vertebrates and invertebrates (Lin et al., 2010; Chen et al., 2011; Chambers et al., 2015). In mammals, especially humans, insulin is mostly investigated in relation to diabetes. Nasal administration of insulin is successfully used as a treatment for Alzheimer’s disease, and type-2 diabetes is associated with the risk of developing Alzheimer’s disease (Reger et al., 2008; Craft et al., 2012; Akinola, 2016). Insulin is not thought to pass through the blood-brain barrier, but it can be transported from the blood into the brain via receptors on vascular endothelial cells, which is called a receptor-mediated transporter pathway (Rhea and Banks, 2019). The effectiveness of insulin for Alzheimer’s disease may be explained from the viewpoint of metabolic stress (Wakabayashi et al., 2019) as follows: metabolic stress causes the onset of insulin resistance and lowers the removal rate of amyloid β proteins from the brain, thereby promoting their accumulation. Accordingly, insulin has been emphasized as a therapeutic drug for Alzheimer’s disease.
Insulin receptors are widely expressed in the brain of vertebrates, and insulin signaling regulates energy metabolism in the hypothalamus, motor function in the cerebellum, memory control, and nerve regeneration in the hippocampus, and emotional and cognitive function control in the cerebral cortex (Arnold et al., 2018). Administration of insulin into the ventricles of rats promotes Akt-dependent translocation of glucose transporter type 4 (GLUT4) in the hippocampus (Grillo et al., 2009). Furthermore, hippocampal-specific suppression of insulin signaling reduces long-term potentiation in the hippocampus and significantly impairs memory and learning ability (Grillo et al., 2015). As mentioned above, type 2 diabetes is associated with an increased risk for Alzheimer’s disease and impaired cognitive function (sometimes referred to as type 3 diabetes Steen et al., 2005). Insulin is a useful treatment, and thus many studies using mammalian brains have focused on the effectiveness of insulin against this disease. The molecular and cellular events behind the therapeutic effect of insulin, however, remain unknown. On the other hand, studies of the involvement of IIS in learning and memory have been performed in invertebrates. We therefore suggest that the studies using invertebrates to elucidate the mechanisms of IIS are particularly important for clarifying the function of insulin in cognition.
In the present review, we outline the studies of IIS mechanisms of memory regulation performed in three well-established invertebrate neuroscience model organisms, Drosophila melanogaster, Caenorhabditis elegans, and Lymnaea stagnalis. To the best of our knowledge, the molecular mechanisms of memory influenced by IIS function are not yet clarified in invertebrates other than these three species, even in the silkworm Bombyx mori. We will present the deduced pathways of IIS for memory by considering the experimental results in these three species, which may help to gain insight into the general scheme of the relationship between IIS function and memory.
IIS and Memory in Drosophila melanogaster
In Drosophila, IIS is involved in memory formation, circadian rhythm formation, growth, development, reproduction, metabolism, stress resistance, aging, and lifespan (Barber et al., 2016; Semaniuk et al., 2021). IIS is required for both short-term memory and long-term memory of olfactory associative learning (Figure 1; Chambers et al., 2015). The main molecules of IIS—ILPs (Dilps), insulin receptor (InR), and insulin receptor substrate (CHICO)—are well studied in mutants. InR and CHICO are required for short-term memory based on studies of chico mutants (Naganos et al., 2012), for intermediate-term memory-based on studies of InR mutants (Tanabe et al., 2017), and for long-term memory based on studies of RNA interference for inR and chico (Chambers et al., 2015). Drosophila has eight types of Dilps (Nässel et al., 2013, 2015; Nässel and Vanden Broeck, 2016). In addition to binding to the receptor tyrosine kinase InR, Dilps can also interact with G protein-coupled receptors. For example, Dilp7 and Dilp8 activate the relaxin receptor-like LGR4 and LGR3, respectively (Colombani et al., 2015; Gontijo and Garelli, 2018; Mita, 2019; Veenstra et al., 2021; Veenstra, 2021). Moreover, Dilp3 functions in intermediate-term memory of aversive olfactory conditioning (Tanabe et al., 2017). In aged flies, a Dilp3 deficit causes memory loss.
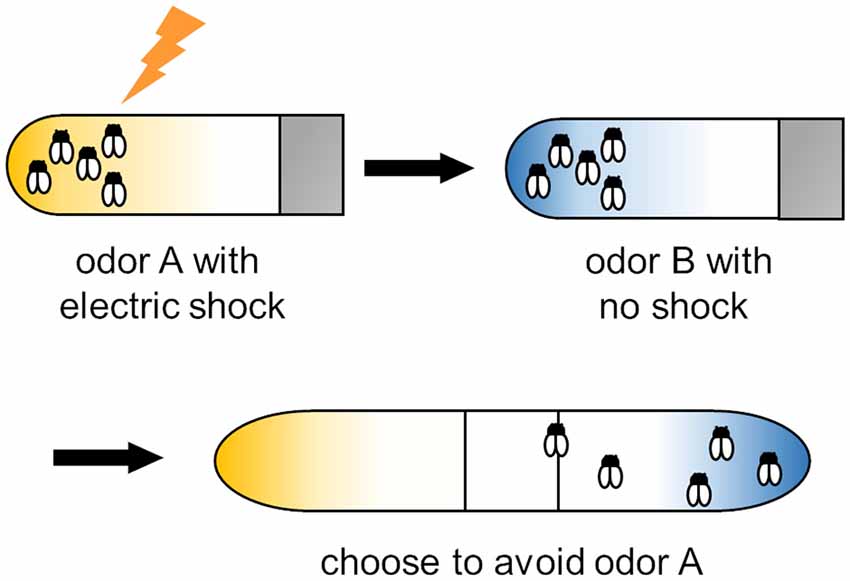
Figure 1. Training protocol for olfactory associative learning in Drosophila. Flies were exposed to odor A with an electric shock and then to odor B with no shock. After training, flies are placed at the choice point of a T maze with odor A or B diffused from both ends. Trained flies avoid the shock-paired odor (i.e., odor A).
The insulin receptor, InR, is involved in protein synthesis-independent “larval” anesthesia-resistant memory (lARM; Eschment et al., 2020). InR expressed in the mushroom body Kenyon cells suppresses lARM formation and contributes to the formation of protein synthesis-dependent longer-lasting memory in Drosophila larvae.
Mutant studies have demonstrated that the insulin receptor substrate CHICO plays an important role in olfactory associative learning (Naganos et al., 2012). CHICO was found to be essential for the development of a CNS region required for olfactory associative learning. CHICO also functions in food-finding latency (Egenriether et al., 2015). The food-finding latency differs between young and aged flies, with a shorter latency in aged flies. Even in young flies, however, the latency becomes shorter if they are starved or if the insulin signaling is reduced in chico mutants. Conversely, the latency of aged flies becomes longer when insulin signaling is enhanced. Learning deficiencies in chico mutants are due to a decrease in cAMP signaling (Naganos et al., 2016).
In 2013, a relationship between starvation and food aversive learning was demonstrated by Hirano and Saitoe (Hirano et al., 2013; Hirano and Saitoe, 2013). When cAMP-response element-binding protein (CREB) binds to cAMP-regulated transcriptional coactivator (CRTC), CREB upregulates some genes (Yin et al., 1994, 1995). CRTC is activated when insulin signaling is downregulated in starved flies (Wang et al., 2008). Thus, starved flies exhibit food aversive learning in one trial because of downregulation of the insulin signaling pathway and upregulation of the CRTC signaling pathway. Using chico mutants, Hirano et al. (2013) found that CRTC accumulated in the mushroom body nuclei (i.e., neurons necessary for memory), and reported that this mutant exhibited 1-trial learning even when satiated.
IIS and Memory in Caenorhabditis elegans
IIS in Caenorhabditis elegans is involved in longevity, stress tolerance, and memory formation (Kenyon et al., 1993; Paradis et al., 1999; Stein and Murphy, 2012; Sasakura and Mori, 2013). The main components of the C. elegans IIS pathway include INS-1 (1 of 40 ILPs), DAF-2 (insulin receptor), AGE-1 (phosphoinositide 3-kinase: PI3K), DAF-16 (forkhead box protein O: FOXO), DAF-18 (phosphatase and tensin homolog deleted on chromosome 10: PTEN), and AKT-1 (serine/threonine kinase; Paradis et al., 1999; Pierce et al., 2001; Li et al., 2003; Tomioka et al., 2006; Murphy and Hu, 2013; Kaletsky et al., 2016; Kim and Webb, 2017). Several reports have demonstrated a relationship between IIS and memory in C. elegans. In IIS mutants, the declines with aging in isothermal tracking, motor activity, and positive butanone associative learning were suppressed, whereas the defects occurred in salt chemotaxis learning and benzaldehyde-starvation avoidance learning (Murphy and Hu, 2013). IIS controls salt chemotactic learning due to two types of changes, an increased Ca2+ response and decreased synaptic release, in the properties of a salt-sensing neuron, ASER, after prolonged NaCl exposure (Oda et al., 2011). A neuropeptide encoded by an insulin-like gene, ins-11, negatively regulates neuronal signaling that controls avoidance behavior toward a pathogen (Pseudomonas aeruginosa; Lee and Mylonakis, 2017).
Nematodes grown under abundant food conditions show positive taxis (i.e., attraction behavior) to NaCl, whereas they show negative taxis (i.e., an avoidance behavior) to NaCl when grown under conditions of starvation but in the presence of NaCl. This is called salt chemotaxis learning (Figure 2; Saeki et al., 2001). This behavioral change does not occur in the case of starvation in the absence of NaCl or in the case of satiation in the presence of NaCl. In other words, when nematodes approach salt and fail to obtain food, they learn to avoid salt. In this learning, INS-1, DAF-2, and AGE-1 in the IIS pathway play important roles. These molecules function during the adult stage of C. elegans development after completion of the neural circuit formation. Nematodes deficient in INS-1 do not acquire this learning (Kodama et al., 2006; Oda et al., 2011). Ohno et al. (2014) reported that activation of the INS-1/DAF-2/PI3K pathway in a chemosensory neuron, ASER, altered the neuronal characteristics; e.g., a change in synaptic vesicle release from ASER. This associative learning was enhanced by IIS. DAF-2 has two splicing isoforms (DAF-2a and DAF-2c), and DAF-2c was found to function in taste-avoidance learning (Tomioka et al., 2022). Nagashima et al. (2019) demonstrated that the DAF-2c-dependent pathway acts in parallel with DAF-16.
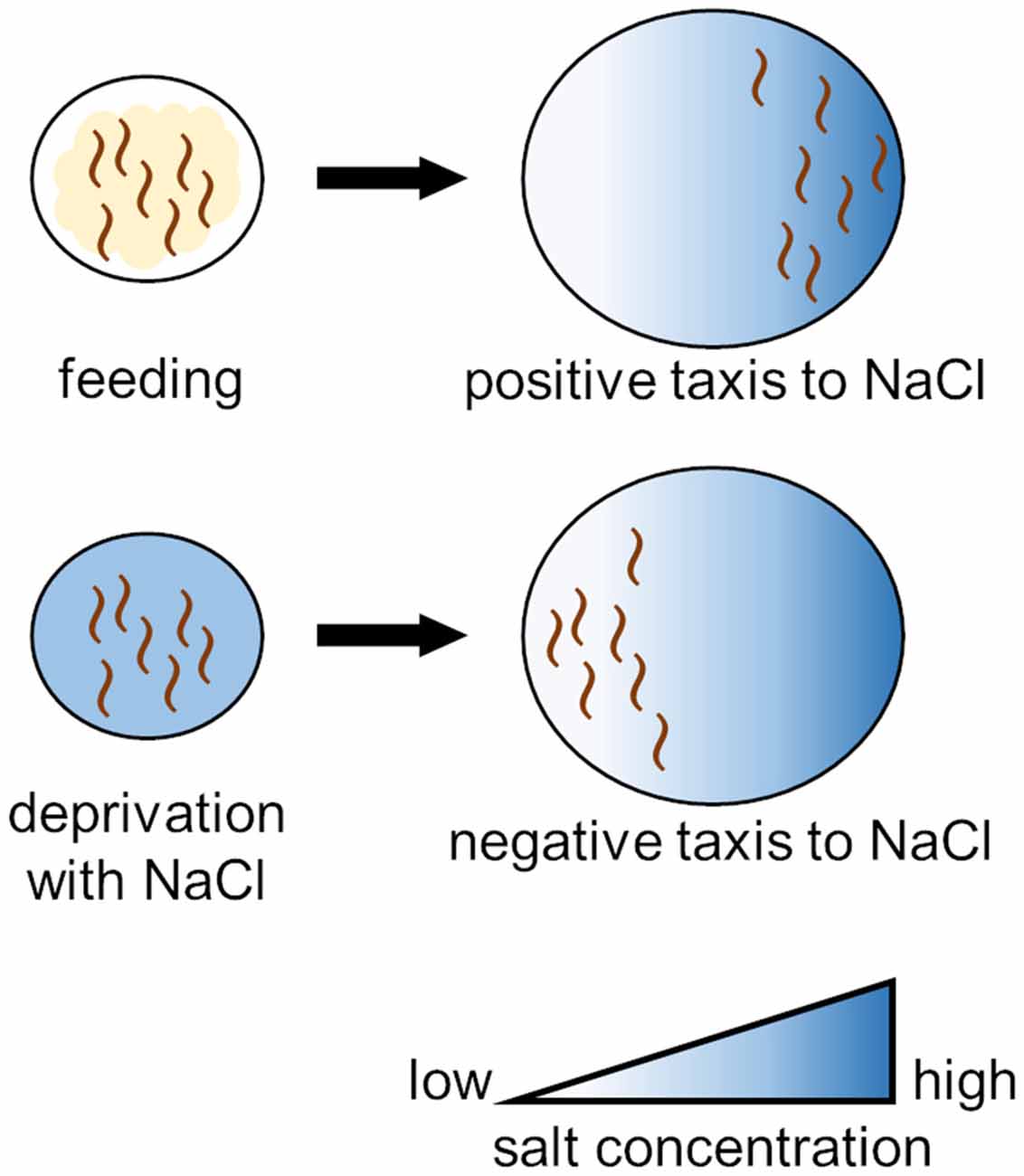
Figure 2. Training protocol for salt chemotaxis learning in C. elegans. Worms exhibit positive chemotaxis (attraction behavior) to NaCl under normal conditions, whereas worms subjected to prolonged exposure to NaCl under starvation conditions exhibit negative chemotaxis (avoidance behavior) to NaCl.
IIS is important not only for learning by NaCl stimulation but also for learning by odor (benzaldehyde) stimulation (Lin et al., 2010). The dramatic change in odorant preference of a nematode after exposure to a high concentration of benzaldehyde in the absence of food is a result of associative learning in which the nematode forms an association between benzaldehyde and starvation (Nuttley et al., 2002). This behavioral plasticity is called benzaldehyde–starvation associative plasticity. Lin et al. (2010) demonstrated that nematodes with mutations in components of IIS pathways are defective in benzaldehyde-starvation associative plasticity and that IIS plays a more significant role in memory retrieval than in memory acquisition. INS-1 can act on multiple neurons and AGE-1 acts on benzaldehyde-sensing amphid wing C (AWC) sensory neurons to direct benzaldehyde–starvation associative plasticity. That is, these findings dissociate the behavioral roles of IIS in the regulation of learning vs. memory recall and help to elucidate the molecular mechanisms involved in this associative plasticity in C. elegans.
On the other hand, in associative learning between presentations of temperature and starvation, INS-1 acts on an interneuron involved in isothermal tracking behavior, antagonizes DAF-2 and AGE-1 and evokes food-related thermotactic plasticity (Murakami et al., 2005, Murakami, 2007). This learning ability declines with age. The age-related decay in age-1 and daf-2 mutants, both of which exhibit increased DAF-16 activity, is delayed. These effects are counteracted in daf-16 mutants. These results suggest that age mutations (age-1 and daf-2 mutations) influence learning behavior in several ways.
Transcriptome analysis using adult C. elegans revealed IIS/FOXO and CREB transcriptional targets required specifically for memory and their tissue-specific expressions (Lakhina et al., 2015; Kaletsky et al., 2018). A nematode CREB homolog, crh-1, is required for long-term memory, but not for learning or short-term memory. The molecular mechanisms involving CREB were clarified in studies of positive butanone associative learning in C. elegans (Kauffman et al., 2011). Nematodes integrate the signals of butanone and food to enhance chemotaxis to butanone (Torayama et al., 2007). Expression of crh-1 decreases with age and its expression and activation correlate with memory performance. The relationship between this crh-1 pathway and the IIS pathway, however, is intricate. Insulin receptor daf-2 mutants exhibit improved memory capacity in early adulthood and maintain learning ability with age. On the other hand, the eat-2 mutant, which is a model of dietary restriction, exhibits impaired long-term memory in young adulthood and this low capability level is maintained with age. That is, rather than changes in the expression and activation of learning-related genes, it is a change in the expression and activation of crh-1 that influences the IIS pathway and dietary restriction pathway in the decreased learning and memory observed with aging (Kauffman et al., 2010).
IIS and Memory in Lymnaea stagnalis
Although the molecular tools available for use in gastropod mollusks are not as advanced as those for application in Drosophila and C. elegans, the contribution of the studies using mollusks to the elucidation of learning and memory mechanisms is significant (Kandel, 2001; Alkon et al., 2005). For example, Aplysia and Limax are well-known to be capable of associative learning (Yamanaka et al., 2021; Momohara et al., 2022). Furthermore, the pond snail Lymnaea stagnalis can be classically or operantly conditioned for many different types of behaviors (e.g., feeding and aerial respiratory behaviors; Sunada et al., 2017a; Crossley et al., 2019; Fodor et al., 2020; Itoh et al., 2021; Pirger et al., 2021; Rivi et al., 2021a, b). As described in the “Introduction” Section, ILP in Lymnaea was recognized as the first ILP with its DNA sequence revealed in invertebrates (Smit et al., 1988; Thorpe and Duve, 1988), leading to extensive studies of the relationship between insulin function and memory formation in Lymnaea (Pirger et al., 2014; Kojima et al., 2015; Benjamin and Kemenes, 2020).
In particular, Lymnaea has the ability to learn a conditioned taste aversion (CTA), which is formed by pairing the application of a sucrose solution as a conditioned stimulus (CS) and the application of a KCl solution or an electric shock as an unconditioned stimulus (US; Figure 3; Kojima et al., 1997; Nakai et al., 2020a). The sucrose solution evokes an innate feeding response, whereas the KCl solution or electric shock induces a withdrawal response. After repeated paired CS-US presentations, the sucrose solution (CS) no longer evokes the feeding response (Totani et al., 2020a). This CTA lasts longer than a month (Kojima et al., 1996). Because temporal changes in memory are generally classified into short-term memory, intermediate-term memory, and long-term memory (Rosenzweig et al., 1993), Lymnaea CTA is considered to be consolidated into long-term memory because of pharmacologic evidence requiring a de novo protein-synthesis processes (Nakai et al., 2020b).
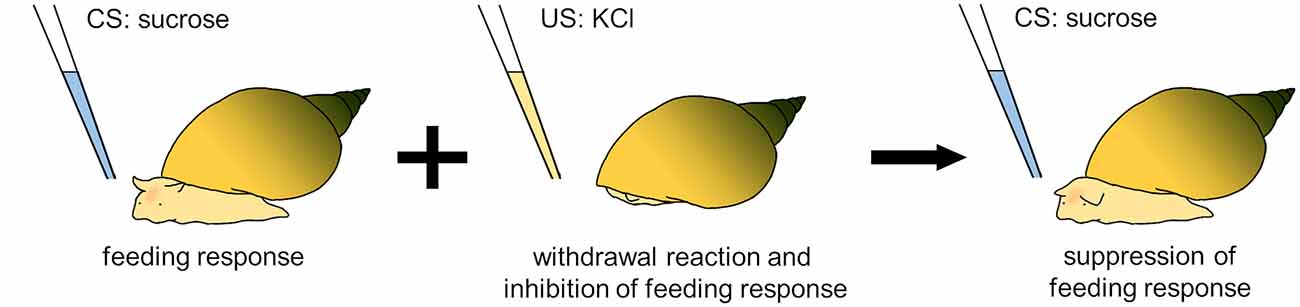
Figure 3. Training protocol for conditioned taste aversion (CTA) in Lymnaea. After a sucrose solution (CS) is paired with a KCl solution (US), the sucrose solution does not elicit a feeding response in snails.
Studies of the relationship between ILPs and memory in Lymnaea began with Azami’s work in 2006, which demonstrated that the expression of some genes was upregulated or downregulated following the formation of CTA and its long-term memory (Azami et al., 2006). In particular, upregulation of MIPs was observed in the CNS isolated from snails exhibiting CTA. Murakami et al. (2013a, b) then hypothesized that MIPs play an important role in altering the activity of feeding neural circuits that result in CTA (Hatakeyama et al., 2013). Applying MIPs or mammalian insulin to the isolated CNS induces long-lasting changes in synaptic efficacy (i.e., enhancement) between a key neuron for CTA (the cerebral giant cell: CGC) and a follower neuron (the B1 motor neuron). The B1 motor neuron innervates the salivary gland and plays a role in radula protraction (McCrohan and Benjamin, 1980a, b; Straub and Benjamin, 2001). These synaptic efficacy changes correlate with the consolidation of CTA into long-term memory in the snail CNS. This synaptic enhancement is blocked by the application of a human insulin receptor antibody, which is considered to block the binding between insulin (or MIPs) and human insulin receptors (or MIP receptors; Taylor et al., 1987). Injection of this human insulin receptor antibody into the snail abdomen before CTA learning blocks long-term memory formation, but not learning (Murakami et al., 2013a). A difference between the neurons critical for learning and those critical for long-term memory was also found in a CGC ablation study (Sunada et al., 2017b). The somata of the CGCs are not necessary for learning acquisition, whereas theyare necessary for long-term memory formation. Thus, both MIPs and the CGC somata play key roles in the memory consolidation of CTA.
Peptide purification of MIP I–III and V and the additional finding of a MIP VII transcript indicate that five types of MIPs function in Lymnaea (Smit et al., 1991, 1993, 1996; Li et al., 1992a, b, c). Their expression is observed in the growth-controlling neuroendocrine light green cells (LGCs) and canopy cells of the cerebral ganglia (Meester et al., 1992; Smit et al., 1992, 1998; Hatakeyama et al., 2000). The cDNA structure of a putative tyrosine kinase receptor for MIPs has also been clarified (Roovers et al., 1995). Many of the typical insulin receptor features, including a cysteine-rich domain, a single transmembrane domain, and two cytoplasmic domains for trans-phosphorylation to induce tyrosine kinase activity, are also conserved in the predicted 1607-amino acid protein in Lymnaea. Extensive screening of cDNA and genomic libraries together with Southern blot analyses have revealed the presence of a single putative MIP receptor gene and suggest that different MIPs bind to the same receptor (Smit et al., 1996).
As described earlier, MIPs reduce the hemolymph glucose concentration in Lymnaea (Mita et al., 2014a), and thus the relationship among the actions of MIPs, nutritional status, and CTA learning ability should be examined (Totani et al., 2020b). Mild starvation (i.e., 1-day food deprivation) results in the best learning and memory for CTA, whereas heavy starvation (i.e., 5-day food deprivation) prevents snails from learning or remembering (Aonuma et al., 2018a). Injecting the snails with mammalian insulin to reduce the hemolymph glucose concentration results in better learning and memory in 5-day food-deprived snails, but injecting glucose into 5-day food-deprived snails does not alter their inability to learn and remember (Mita et al., 2014a, b). On the basis of these observations, the “insulin spike hypothesis” (i.e., a rise in the insulin concentration in the CNS of snails) was proposed for the formation of CTA and its long-term memory (Mita et al., 2014a, b; Kojima et al., 2015).
Evidence to support this hypothesis was obtained in 2020 (Totani et al., 2020c). As mentioned above, 1-day food-deprived snails exhibit the best CTA learning and memory, whereas 5-day food-deprived snails do not express good memory. CTA and its long-term memory, however, are indeed formed in 5-day food-deprived snails, but memory recall for the CTA is prevented by the effects of food deprivation. Long-term memory recall in 5-day food-deprived snails is expressed following 7 days of feeding and then 1 day of food deprivation. Totani et al. (2020c) revealed that this 1 day of food deprivation before the memory test in snails increased the mRNA levels of MIP II, a major MIP, in the CNS. Instead of this 1-day food deprivation, injection of insulin into snails activates CTA neurons and mimics the food deprivation state before a memory test. Together, these results suggest that a spike in insulin release recapitulates the optimal internal state for long-term memory recall following CTA training in snails (Totani et al., 2020c).
The neurocircuit involved in CTA in Lymnaea has been examined from the viewpoint of some neurotransmitters, such as monoamines (Yamanaka et al., 2000; Kawai et al., 2011), and the relationship between monoamines and insulin in CTA learning ability has been examined (Aonuma et al., 2016, 2017; Totani et al., 2019). One monoamine, serotonin (5-hydroxytryptamin; 5-HT), is known to be involved in decision-making in Lymnaea (Aonuma et al., 2020). Although the snails with 1-day food deprivation exhibit the best learning and memory for CTA, immersion of 1-day food-deprived snails in 5-HT impairs CTA learning and memory by increasing the 5-HT content. Furthermore, injection of mammalian insulin into these snails reverses this impairment (Aonuma et al., 2018b). That is, insulin rescues the CTA deficit, which may be due to a decrease in the 5-HT content in the CNS. Totani et al. (2022) further examined why CTA learning ability is worse in 5-day food-deprived snails than in 1-day food-deprived snails and how the CNS 5-HT concentration increases in 5-day food-deprived snails (returns to basal levels). They measured the concentration of tryptophan (i.e., 5-HT precursor) in the hemolymph and CNS, and demonstrated that the CNS tryptophan concentration was higher in 5-day food-deprived snails than in 1-day food-deprived snails, whereas the hemolymph tryptophan concentration was not affected by the duration of food deprivation. This finding suggests the existence of a mediator of the CNS tryptophan concentration independent of food deprivation. This mediator was then identified as “autophagic flux” in the CNS under different food deprivation conditions. The tryptophan concentration in the hemolymph and the autophagic flux in the CNS cooperatively regulate CTA learning ability affected by different durations of food deprivation.
Perspective and Conclusion
These findings together provide a perspective of the deduced scheme for involvement of the insulin pathway in cognitive function in invertebrates (Figure 4). We note two kinds of transcription factors in IIS: CREB and FOXO, which are well analyzed in the above-mentioned three species of invertebrates. ILP/IGF binding to its receptor tyrosine kinase drives the phosphoinositide-3-kinase (PI3K)-Akt/protein kinase B (PKB) pathway (Belfiore et al., 2009; Hay, 2011). The PI3K-Akt/PKB pathway regulates CREB for gene expression (Du and Montminy, 1998; Thiel et al., 2021). In Lymnaea, activation of CREB plays a critical role in the formation of long-term memory (Ribeiro et al., 2003; Sadamoto et al., 2004, 2010), and at the mRNA level, the amount of suppressor CREB (e.g., Lymnaea CREB2) is more abundant than the amount of activator CREB (e.g., Lymnaea CREB1; Wagatsuma et al., 2005). The other transcription factor, FOXO, is “inactivated” by the PI3K-Akt/PKB pathway (Lin et al., 2001; Hay, 2011). FOXO, however, is involved in learning and memory formation in a starved state (Nagashima et al., 2019). That is, the function of FOXO remains a mystery, and likely has two different roles. Akt translocates GLUT4 storage vesicles (GSVs) to the membrane, resulting in the expression of GLUT4. GLUT4 is glucose transporter isoform 4 and is suggested to be involved in memory formation (Grillo et al., 2009).
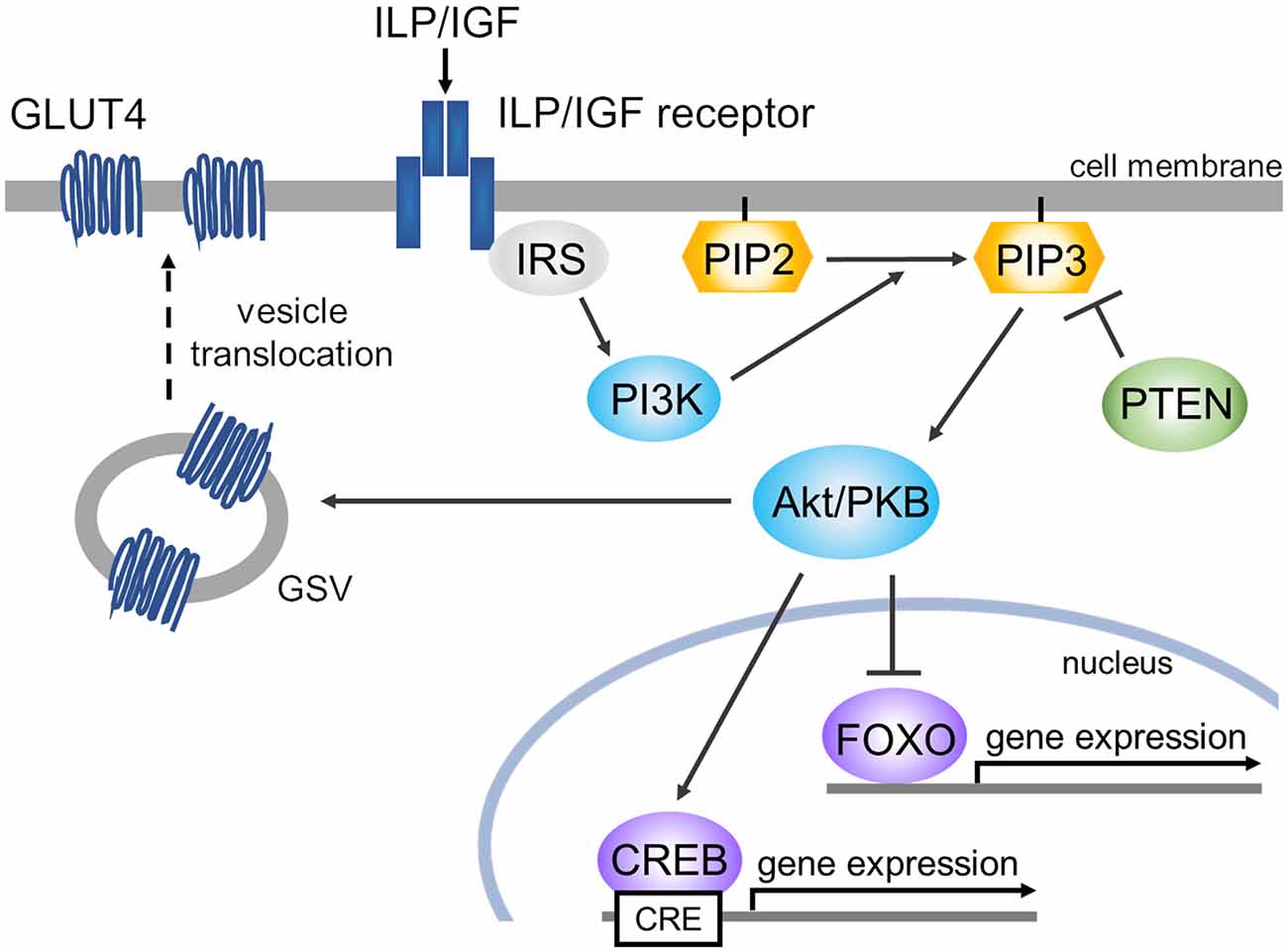
Figure 4. Scheme of deduced ILP/IGF signaling cascades for memory enhancement. There are three main pathways: (1) Akt/PKB phosphorylates CREB (e.g., CREB1 in Lymnaea), resulting in gene expression. (2) Akt/PKB phosphorylates FOXO, inducing its secession from DNA. (3) Akt translocates GSV to the membrane, resulting in the expression of GLUT4. The ILP/IGF receptors are called InR in Drosophila, DAF-2/IGFR (insulin/IGF-1 transmembrane receptor) in C. elegans, and MIPR in Lymnaea. Abbreviations: Akt/PKB, Akt/protein kinase B; CREB, cAMP response element-binding protein; FOXO, forkhead box protein O; GLUT4, glucose transporter isoform 4; GSV, GLUT4 storage vesicle; IRS, insulin receptor substrate; PI3K, phosphoinositide-3-kinase; PIP2, phosphatidylinositol 4,5-bisphosphate; PIP3, phosphatidylinositol 3,4,5-trisphosphate; PTEN, phosphatase and tensin homolog deleted on chromosome 10 (i.e., PIP3 phosphatase).
In conclusion, here we have reviewed the relationship between insulin and memory in Drosophila, C. elegans, and Lymnaea. We propose that the mechanisms underlying the involvement of insulin in cognition in invertebrates occur across phyla, including humans, although findings of the molecular mechanisms in mammals are scant. It is important to note that learning ability depends on the state of starvation in invertebrates. Short-term fasting in mammals also enhances cognition, including memory consolidation (Dias et al., 2021), whereas obesity impairs cognition and increases the risk of dementia in humans (Shinjyo and Kita, 2021). Extensive studies using invertebrates can provide important insight into the advantages of short-term fasting in humans. In addition, by using invertebrates, it is easier to investigate the complex interplay between the specific roles of insulin and not only cognitive abilities but also longevity and stress tolerance. This insight is also important for translational medical studies.
Author Contributions
EI conceptualized the study. JN drew the figures. All authors (JN, NC, KF, YT, DH, VED, and EI) participated in the study design and manuscript preparation, and approved the submitted manuscript and final manuscript. All authors contributed to the article and approved the submitted version.
Funding
This work was supported by the following funding sources: the Japan Science and Technology Agency SPRING Grant number JPMJSP2128 to JN, the Koltzov Institute of Developmental Biology Russian Academy of Sciences Basic Research Program RSF 22-24-00318 to VED, and the Waseda University Grant for Special Research Projects 2018K-141 to EI.
Conflict of Interest
The authors declare that the research was conducted in the absence of any commercial or financial relationships that could be construed as a potential conflict of interest.
Publisher’s Note
All claims expressed in this article are solely those of the authors and do not necessarily represent those of their affiliated organizations, or those of the publisher, the editors and the reviewers. Any product that may be evaluated in this article, or claim that may be made by its manufacturer, is not guaranteed or endorsed by the publisher.
References
Akinola, O. B. (2016). Sweet old memories: a review of the experimental models of the association between diabetes, senility and dementia. Metab. Brain Dis. 31, 1003–1010. doi: 10.1007/s11011-016-9876-0
Alkon, D. L., Epstein, H., Kuzirian, A., Bennett, M. C., and Nelson, T. J. (2005). Protein synthesis required for long-term memory is induced by PKC activation on days before associative learning. Proc. Natl. Acad. Sci. U S A 102, 16432–16437. doi: 10.1073/pnas.0508001102
Andoh, T. (2021). “28A: Insulin,” in Handbook of Hormones (Vol. 1), 2nd Edn., eds H. Ando, K. Ukena, and S. Nagata (London: Academic Press), 279–283.
Aonuma, H., Kaneda, M., Hatakeyama, D., Watanabe, T., Lukowiak, K., and Ito, E. (2016). Relationship between the grades of a learned aversive-feeding response and the dopamine contents in Lymnaea. Biol. Open 5, 1869–1873. doi: 10.1242/bio.021634
Aonuma, H., Kaneda, M., Hatakeyama, D., Watanabe, T., Lukowiak, K., and Ito, E. (2017). Weak involvement of octopamine in aversive taste learning in a snail. Neurobiol. Learn. Mem. 141, 189–198. doi: 10.1016/j.nlm.2017.04.010
Aonuma, H., Mezheritskiy, M., Boldyshev, B., Totani, Y., Vorontsov, D., Zakharov, I., et al. (2020). The role of serotonin in the influence of intense locomotion on the behavior under uncertainty in the mollusk Lymnaea stagnalis. Front. Physiol. 11:221. doi: 10.3389/fphys.2020.00221
Aonuma, H., Totani, Y., Sakakibara, M., Lukowiak, K., and Ito, E. (2018a). Comparison of brain monoamine content in three populations of Lymnaea that correlates with taste-aversive learning ability. Biophys. Physicobiol. 15, 129–135. doi: 10.2142/biophysico.15.0_129
Aonuma, H., Totani, Y., Kaneda, M., Nakamura, R., Watanabe, T., Hatakeyama, D., et al. (2018b). Effects of 5-HT and insulin on learning and memory formation in food-deprived snails. Neurobiol. Learn. Mem. 148, 20–29. doi: 10.1016/j.nlm.2017.12.010
Arnold, S. E., Arvanitakis, Z., Macauley-Rambach, S. L., Koenig, A. M., Wang, H. Y., Ahima, R. S., et al. (2018). Brain insulin resistance in type 2 diabetes and Alzheimer disease: concepts and conundrums. Nat. Rev. Neurol. 14, 168–181. doi: 10.1038/nrneurol.2017.185
Azami, S., Wagatsuma, A., Sadamoto, H., Hatakeyama, D., Usami, T., Fujie, M., et al. (2006). Altered gene activity correlated with long-term memory formation of conditioned taste aversion in Lymnaea. J. Neurosci. Res. 84, 1610–1620. doi: 10.1002/jnr.21045
Barber, A. F., Erion, R., Holmes, T. C., and Sehgal, A. (2016). Circadian and feeding cues integrate to drive rhythms of physiology in Drosophila insulin-producing cells. Genes Dev. 30, 2596–2606. doi: 10.1101/gad.288258.116
Belfiore, A., Frasca, F., Pandini, G., Sciacca, L., and Vigneri, R. (2009). Insulin receptor isoforms and insulin receptor/insulin-like growth factor receptor hybrids in physiology and disease. Endocr. Rev. 30, 586–623. doi: 10.1210/er.2008-0047
Benjamin, P. R., and Kemenes, I. (2020). “Peptidergic systems in the pond snail Lymnaea: from genes to hormones and behavior,” in Advances in Invertebrate (Neuro) Endocrinology, eds S. Saleuddin, A. B. Lange, and I. Orchard (Palm Bay, FL: Apple Academic Press), 213–254. doi: 10.1201/9781003029854-7
Blundell, T. L., Bedarkar, S., Rinderknecht, E., and Humbel, R. E. (1978). Insulin-like growth factor: a model for tertiary structure accounting for immunoreactivity and receptor binding. Proc. Natl. Acad. Sci. U S A 75, 180–184. doi: 10.1073/pnas.75.1.180
Brogiolo, W., Stocker, H., Ikeya, T., Rintelen, F., Fernandez, R., and Hafen, E. (2001). An evolutionarily conserved function of the Drosophila insulin receptor and insulin-like peptides in growth control. Curr. Biol. 11, 213–221. doi: 10.1016/s0960-9822(01)00068-9
Chambers, D. B., Androschuk, A., Rosenfelt, C., Langer, S., Harding, M., and Bolduc, F. V. (2015). Insulin signaling is acutely required for long-term memory in Drosophila. Front. Neural Circuits 9:8. doi: 10.3389/fncir.2015.00008
Chen, D. Y., Stern, S. A., Garcia-Osta, A., Saunier-Rebori, B., Pollonini, G., Bambah-Mukku, D., et al. (2011). A critical role for IGF-II in memory consolidation and enhancement. Nature. 469, 491–497. doi: 10.1038/nature09667
Colombani, J., Andersen, D. S., Boulan, L., Boone, E., Romero, N., Virolle, V., et al. (2015). Drosophila Lgr3 couples organ growth with maturation and ensures developmental stability. Curr. Biol. 25, 2723–2729. doi: 10.1016/j.cub.2015.09.020
Craft, S., Baker, L. D., Montine, T. J., Minoshima, S., Watson, G. S., Claxton, A., et al. (2012). Intranasal insulin therapy for Alzheimer disease and amnestic mild cognitive impairment: a pilot clinical trial. Arch. Neurol. 69, 29–38. doi: 10.1001/archneurol.2011.233
Crossley, M., Lorenzetti, F. D., Naskar, S., O’Shea, M., Kemenes, G., Benjamin, P. R., et al. (2019). Proactive and retroactive interference with associative memory consolidation in the snail Lymnaea is time and circuit dependent. Commun. Biol. 2:242. doi: 10.1038/s42003-019-0470-y
Dias, G. P., Murphy, T., Stangl, D., Ahmet, S., Morisse, B., Nix, A., et al. (2021). Intermittent fasting enhances long-term memory consolidation, adult hippocampal neurogenesis and expression of longevity gene Klotho. Mol. Psychiatry. 26, 6365–6379. doi: 10.1038/s41380-021-01102-4
Du, K., and Montminy, M. (1998). CREB is a regulatory target for the protein kinase Akt/PKB. J. Biol. Chem. 273, 32377–32379. doi: 10.1074/jbc.273.49.32377
Ebberink, R. H. M., Smit, A. B., and Van Minnen, J. (1989). The insulin family: evolution of structure and function in vertebrates and invertebrates. Biol. Bull. 177, 176–182. doi: 10.2307/1541928
Egenriether, S. M., Chow, E. S., Krauth, N., and Giebultowicz, J. M. (2015). Accelerated food source location in aging Drosophila. Aging Cell 14, 916–918. doi: 10.1111/acel.12361
Eschment, M., Franz, H. R., Güllü, N., Hölscher, L. G., Huh, K. E., and Widmann, A. (2020). Insulin signaling represents a gating mechanism between different memory phases in Drosophila larvae. PLoS Genet. 16:e1009064. doi: 10.1371/journal.pgen.1009064
Fernandez, R., Tabarini, D., Azpiazu, N., Frasch, M., and Schlessinger, J. (1995). The Drosophila insulin receptor homolog: a gene essential for embryonic development encodes two receptor isoforms with different signaling potential. EMBO J. 14, 3373–3384. doi: 10.1002/j.1460-2075.1995.tb07343.x
Fodor, I., Hussein, A. A., Benjamin, P. R., Koene, J. M., and Pirger, Z. (2020). The unlimited potential of the great pond snail, Lymnaea stagnalis. eLife 9:e56962. doi: 10.7554/eLife.56962
Garelli, A., Gontijo, A. M., Miguela, V., Caparros, E., and Dominguez, M. (2012). Imaginal discs secrete insulin-like peptide 8 to mediate plasticity of growth and maturation. Science 336, 579–582. doi: 10.1126/science.1216735
Gontijo, A. M., and Garelli, A. (2018). The biology and evolution of the Dilp8-Lgr3 pathway: a relaxin-like pathway coupling tissue growth and developmental timing control. Mech. Dev. 154, 44–50. doi: 10.1016/j.mod.2018.04.005
Grillo, C. A., Piroli, G. G., Hendry, R. M., and Reagan, L. P. (2009). Insulin-stimulated translocation of GLUT4 to the plasma membrane in rat hippocampus is PI3-kinase dependent. Brain Res. 1296, 35–45. doi: 10.1016/j.brainres.2009.08.005
Grillo, C. A., Piroli, G. G., Lawrence, R. C., Wrighten, S. A., Green, A. J., Wilson, S. P., et al. (2015). Hippocampal insulin resistance impairs spatial learning and synaptic plasticity. Diabetes 64, 3927–3936. doi: 10.2337/db15-0596
Hatakeyama, D., Ito, I., Kojima, S., Fujito, Y., and Ito, E. (2000). Complement receptor 3-like immunoreactivity in the light green cells and the canopy cells of the pond snail, Lymnaea stagnalis. Brain Res. 865, 102–106. doi: 10.1016/s0006-8993(00)02203-4
Hatakeyama, D., Okuta, A., Otsuka, E., Lukowiak, K., and Ito, E. (2013). Consolidation of long-term memory by insulin in Lymnaea is not brought about by changing the number of insulin receptors. Commun. Integr. Biol. 6:e23955. doi: 10.4161/cib.23955
Hay, N. (2011). Interplay between FOXO, TOR and Akt. Biochim. Biophys. Acta 1813, 1965–1970. doi: 10.1016/j.bbamcr.2011.03.013
Hirano, Y., Masuda, T., Naganos, S., Matsuno, M., Ueno, K., Miyashita, T., et al. (2013). Fasting launches CRTC to facilitate long-term memory formation in Drosophila. Science 339, 443–446. doi: 10.1126/science.1227170
Hirano, Y., and Saitoe, M. (2013). Hunger and memory; CRTC coordinates long-term memory with the physiological state, hunger. Commun. Integr. Biol. 6:e25152. doi: 10.4161/cib.25152
Itoh, A., Komatsuzaki, Y., Lukowiak, K., and Saito, M. (2021). Epicatechin increases the persistence of long-term memory formed by conditioned taste aversion in Lymnaea. J. Exp. Biol. 224:jeb238055. doi: 10.1242/jeb.238055
Kaletsky, R., Lakhina, V., Arey, R., Williams, A., Landis, J., Ashraf, J., et al. (2016). C. elegans adult neuronal IIS/FOXO transcriptome reveals adult phenotype regulators. Nature 529, 92–96. doi: 10.1038/nature16483
Kaletsky, R., Yao, V., Williams, A., Runnels, A. M., Tadych, A., Zhou, S., et al. (2018). Transcriptome analysis of adult Caenorhabditis elegans cells reveals tissue-specific gene and isoform expression. PLoS Genet. 14:e1007559. doi: 10.1371/journal.pgen.1007559
Kandel, E. R. (2001). The molecular biology of memory storage: a dialog between genes and synapses. Biosci. Rep. 21, 565–611. doi: 10.1023/a:1014775008533
Kauffman, A. L., Ashraf, J. M., Corces-Zimmerman, M. R., Landis, J. N., and Murphy, C. T. (2010). Insulin signaling and dietary restriction differentially influence the decline of learning and memory with age. PLoS Biol. 8:e1000372. doi: 10.1371/journal.pbio.1000372
Kauffman, A., Parsons, L., Stein, G., Wills, A., Kaletsky, R., and Murphy, C. (2011). C. elegans positive butanone learning, short-term and long-term associative memory assays. J. Vis. Exp. 49:e2490. doi: 10.3791/2490
Kawai, R., Kobayashi, S., Fujito, Y., and Ito, E. (2011). Multiple subtypes of serotonin receptors in the feeding circuit of a pond snail. Zoolog. Sci. 28, 517–525. doi: 10.2108/zsj.28.517
Kenyon, C., Chang, J., Gensch, E., Rudner, A., and Tabtiang, R. (1993). A C. elegans mutant that lives twice as long as wild type. Nature 366, 461–464. doi: 10.1038/366461a0
Kim, S. Y., and Webb, A. E. (2017). Neuronal functions of FOXO/DAF-16. Nutr. Healthy Aging 4, 113–126. doi: 10.3233/NHA-160009
Kimura, K. D., Tissenbaum, H. A., Liu, Y., and Ruvkun, G. (1997). daf-2, an insulin receptor-like gene that regulates longevity and diapause in Caenorhabditis elegans. Science 277, 942–946. doi: 10.1126/science.277.5328.942
Kodama, E., Kuhara, A., Mohri-Shiomi, A., Kimura, K. D., Okumura, M., Tomioka, M., et al. (2006). Insulin-like signaling and the neural circuit for integrative behavior in C. elegans. Genes Dev. 20, 2955–2960. doi: 10.1101/gad.1479906
Kojima, S., Nakamura, H., Nagayama, S., Fujito, Y., and Ito, E. (1997). Enhancement of an inhibitory input to the feeding central pattern generator in Lymnaea stagnalis during conditioned taste-aversion learning. Neurosci. Lett. 23, 179–182. doi: 10.1016/s0304-3940(97)00507-7
Kojima, S., Sunada, H., Mita, K., Sakakibara, M., Lukowiak, K., and Ito, E. (2015). Function of insulin in snail brain in associative learning. J. Comp. Physiol. A Neuroethol. Sens. Neural Behav. Physiol. 201, 969–981. doi: 10.1007/s00359-015-1032-5
Kojima, S., Yamanaka, M., Fujito, Y., and Ito, E. (1996). Differential neuroethological effects of aversive and appetitive reinforcing stimuli on associative learning in Lymnaea stagnalis. Zool. Sci. 13, 803–812. doi: 10.2108/zsj.13.803
Lakhina, V., Arey, R. N., Kaletsky, R., Kauffman, A., Stein, G., Keyes, W., et al. (2015). Genome-wide functional analysis of CREB/long-term memory-dependent transcription reveals distinct basal and memory gene expression programs. Neuron 85, 330–345. doi: 10.1016/j.neuron.2014.12.029
Lee, K., and Mylonakis, E. (2017). An intestine-derived neuropeptide controls avoidance behavior in Caenorhabditis elegans. Cell Rep. 20, 2501–2512. doi: 10.1016/j.celrep.2017.08.053
Li, K. W., Geraerts, W. P., Ebberink, R. H., and Joosse, J. (1992a). Purification and sequencing of molluscan insulin-related peptide I (MIP I) from the neuroendocrine light green cells of Lymnaea stagnalis. Mol. Cell. Endocrinol. 85, 141–150. doi: 10.1016/0303-7207(92)90133-q
Li, K. W., Geraerts, W. P., and Joosse, J. (1992b). Purification and sequencing of molluscan insulin-related peptide II from the neuroendocrine light green cells in Lymnaea stagnalis. Endocrinology 130, 3427–3432. doi: 10.1210/endo.130.6.1350761
Li, K. W., Geraerts, W. P., van Loenhout, H., and Joosse, J. (1992c). Biosynthesis and axonal transport of multiple molluscan insulin-related peptides by the neuroendocrine light green cells of Lymnaea stagnalis. Gen. Comp. Endocrinol. 87, 79–86. doi: 10.1016/0016-6480(92)90152-a
Li, W., Kennedy, S. G., and Ruvkun, G. (2003). daf-28 encodes a C. elegans insulin superfamily member that is regulated by environmental cues and acts in the DAF-2 signaling pathway. Genes Dev. 17, 844–858. doi: 10.1101/gad.1066503
Lin, K., Hsin, H., Libina, N., and Kenyon, C. (2001). Regulation of the Caenorhabditis elegans longevity protein DAF-16 by insulin/IGF-1 and germline signaling. Nat. Genet. 28, 139–145. doi: 10.1038/88850
Lin, C. H., Tomioka, M., Pereira, S., Sellings, L., Iino, Y., and van der Kooy, D. (2010). Insulin signaling plays a dual role in Caenorhabditis elegans memory acquisition and memory retrieval. J. Neurosci. 30, 8001–8011. doi: 10.1523/JNEUROSCI.4636-09.2010
McCrohan, C. R., and Benjamin, P. R. (1980a). Patterns of activity and axonal projections of the cerebral giant cells of the snail, Lymnaea stagnalis. J. Exp. Biol. 85, 149–168. doi: 10.1242/jeb.85.1.149
McCrohan, C. R., and Benjamin, P. R. (1980b). Synaptic relationships of the cerebral giant cells with motoneurones in the feeding system of Lymnaea stagnalis. J. Exp. Biol. 85, 169–186. doi: 10.1242/jeb.85.1.169
Meester, I., Ramkema, M. D., van Minnen, J., and Boer, H. H. (1992). Differential expression of four genes encoding molluscan insulin-related peptides in the central nervous system of the pond snail Lymnaea stagnalis. Cell Tissue Res. 269, 183–188. doi: 10.1007/BF00384739
Mita, M. (2019). Starfish gonadotropic hormone: from gamete-shedding substance to relaxin-like gonad-stimulating peptide. Front. Endocrinol. (Lausanne) 10:182. doi: 10.3389/fendo.2019.00182
Mita, K., Okuta, A., Okada, R., Hatakeyama, D., Otsuka, E., Yamagishi, M., et al. (2014a). What are the elements of motivation for acquisition of conditioned taste aversion? Neurobiol. Learn. Mem. 107, 1–12. doi: 10.1016/j.nlm.2013.10.013
Mita, K., Yamagishi, M., Fujito, Y., Lukowiak, K., and Ito, E. (2014b). An increase in insulin is important for the acquisition conditioned taste aversion in Lymnaea. Neurobiol. Learn. Mem. 116, 132–138. doi: 10.1016/j.nlm.2014.10.006
Momohara, Y., Neveu, C. L., Chen, H. M., Baxter, D. A., and Byrne, J. H. (2022). Specific plasticity loci and their synergism mediate operant conditioning. J. Neurosci. 42, 1211–1223. doi: 10.1523/JNEUROSCI.1722-21.2021
Murakami, H., Bessinger, K., Hellmann, J., and Murakami, S. (2005). Aging-dependent and -independent modulation of associative learning behavior by insulin/insulin-like growth factor-1 signal in Caenorhabditis elegans. J. Neurosci. 25, 10894–10904. doi: 10.1523/JNEUROSCI.3600-04.2005
Murakami, J., Okada, R., Sadamoto, H., Kobayashi, S., Mita, K., Sakamoto, Y., et al. (2013a). Involvement of insulin-like peptide in long-term synaptic plasticity and long-term memory of the pond snail Lymnaea stagnalis. J. Neurosci. 33, 371–383. doi: 10.1523/JNEUROSCI.0679-12.2013
Murakami, J., Okada, R., Fujito, Y., Sakakibara, M., Lukowiak, K., and Ito, E. (2013b). Paired pulse ratio analysis of insulin-induced synaptic plasticity in the snail brain. J. Exp. Biol. 216, 1771–1773. doi: 10.1242/jeb.083469
Murakami, S. (2007). Caenorhabditis elegans as a model system to study aging of learning and memory. Mol. Neurobiol. 35, 85–94. doi: 10.1007/BF02700625
Murphy, C. T., and Hu, P. J. (2013). “Insulin/insulin-like growth factor signaling in C. elegans”. WormBook, ed The C. elegans Research Community (WormBook.org), 1–43. doi: 10.1895/wormbook.1.164.1
Naganos, S., Horiuchi, J., and Saitoe, M. (2012). Mutations in the Drosophila insulin receptor substrate, CHICO, impair olfactory associative learning. Neurosci. Res. 73, 49–55. doi: 10.1016/j.neures.2012.02.001
Naganos, S., Ueno, K., Horiuchi, J., and Saitoe, M. (2016). Learning defects in Drosophila growth restricted chico mutants are caused by attenuated adenylyl cyclase activity. Mol. Brain 9:37. doi: 10.1186/s13041-016-0217-3
Nagasawa, H., Kataoka, H., Isogai, A., Tamura, S., Suzuki, A., Mizoguchi, A., et al. (1986). Amino acid sequence of a prothoracicotropic hormone of the silkworm Bombyx mori. Proc. Natl. Acad. Sci. U S A 83, 5840–5843. doi: 10.1073/pnas.83.16.5840
Nagashima, T., Iino, Y., and Tomioka, M. (2019). DAF-16/FOXO promotes taste avoidance learning independently of axonal insulin-like signaling. PLoS Genet. 15:e1008297. doi: 10.1371/journal.pgen.1008297
Nakai, J., Totani, Y., Hatakeyama, D., Dyakonova, V. E., and Ito, E. (2020a). Another example of conditioned taste aversion: case of snails. Biology (Basel) 9:422. doi: 10.3390/biology9120422
Nakai, J., Totani, Y., Kojima, S., Sakakibara, M., and Ito, E. (2020b). Features of behavioral changes underlying conditioned taste aversion in the pond snail Lymnaea stagnalis. Invert. Neurosci. 20:8. doi: 10.1007/s10158-020-00241-7
Nässel, D. R., Kubrak, O. I., Liu, Y., Luo, J., and Lushchak, O. V. (2013). Factors that regulate insulin producing cells and their output in Drosophila. Front. Physiol. 4:252. doi: 10.3389/fphys.2013.00252
Nässel, D. R., Liu, Y., and Luo, J. (2015). Insulin/IGF signaling and its regulation in Drosophila. Gen. Comp. Endocrinol. 221, 255–266. doi: 10.1016/j.ygcen.2014.11.021
Nässel, D. R., and Vanden Broeck, J. (2016). Insulin/IGF signaling in Drosophila and other insects: factors that regulate production, release and post-release action of the insulin-like peptides. Cell. Mol. Life Sci. 73, 271–290. doi: 10.1007/s00018-015-2063-3
Nuttley, W. M., Atkinson-Leadbeater, K. P., and Van Der Kooy, D. (2002). Serotonin mediates food-odor associative learning in the nematode Caenorhabditis elegans. Proc. Natl. Acad. Sci. U S A 99, 12449–112454. doi: 10.1073/pnas.192101699
Oda, S., Tomioka, M., and Iino, Y. (2011). Neuronal plasticity regulated by the insulin-like signaling pathway underlies salt chemotaxis learning in Caenorhabditis elegans. J. Neurophysiol. 106, 301–308. doi: 10.1152/jn.01029.2010
Ohno, H., Kato, S., Naito, Y., Kunitomo, H., Tomioka, M., and Iino, Y. (2014). Role of synaptic phosphatidylinositol 3-kinase in a behavioral learning response in C. elegans. Science 345, 313–317. doi: 10.1126/science.1250709
Paradis, S., Ailion, M., Toker, A., Thomas, J. H., and Ruvkun, G. (1999). A PDK1 homolog is necessary and sufficient to transduce AGE-1 PI3 kinase signals that regulate diapause in Caenorhabditis elegans. Genes Dev. 13, 1438–1452. doi: 10.1101/gad.13.11.1438
Pierce, S. B., Costa, M., Wisotzkey, R., Devadhar, S., Homburger, S. A., Buchman, A. R., et al. (2001). Regulation of DAF-2 receptor signaling by human insulin and ins-1, a member of the unusually large and diverse C. elegans insulin gene family. Genes Dev. 15, 672–686. doi: 10.1101/gad.867301
Pirger, Z., László, Z., Naskar, S., Crossley, M., O’Shea, M., Benjamin, P. R., et al. (2021). Interneuronal mechanisms for learning-induced switch in a sensory response that anticipates changes in behavioral outcomes. Curr. Biol. 31, 1754–1761.e3. doi: 10.1016/j.cub.2021.01.072
Pirger, Z., Naskar, S., László, Z., Kemenes, G., Reglődi, D., and Kemenes, I. (2014). Reversal of age-related learning deficiency by the vertebrate PACAP and IGF-1 in a novel invertebrate model of aging: the pond snail (Lymnaea stagnalis). J. Gerontol. A Biol. Sci. Med. Sci. 69, 1331–1338. doi: 10.1093/gerona/glu068
Reger, M. A., Watson, G. S., Green, P. S., Wilkinson, C. W., Baker, L. D., Cholerton, B., et al. (2008). Intranasal insulin improves cognition and modulates beta-amyloid in early AD. Neurology 70, 440–448. doi: 10.1212/01.wnl.0000265401.62434.36
Rhea, E. M., and Banks, W. A. (2019). Role of the blood-brain barrier in central nervous system insulin resistance. Front. Neurosci. 13:521. doi: 10.3389/fnins.2019.00521
Ribeiro, M. J., Serfozo, Z., Papp, A., Kemenes, I., O’Shea, M., Yin, J. C., et al. (2003). Cyclic AMP response element-binding (CREB)-like proteins in a molluscan brain: cellular localization and learning-induced phosphorylation. Eur. J. Neurosci. 18, 1223–1234. doi: 10.1046/j.1460-9568.2003.02856.x
Rinderknecht, E., and Humbel, R. E. (1978). The amino acid sequence of human insulin-like growth factor I and its structural homology with proinsulin. J. Biol. Chem. 253, 2769–2776. doi: 10.1016/S0021-9258(17)40889-1
Rivi, V., Batabyal, A., Benatti, C., Blom, J. M., Tascedda, F., and Lukowiak, K. (2021a). A flavonoid, quercetin, is capable of enhancing long-term memory formation if encountered at different times in the learning, memory formation and memory recall continuum. J. Comp. Physiol. 208, 253–265. doi: 10.1007/s00359-021-01522-1
Rivi, V., Benatti, C., Lukowiak, K., Colliva, C., Alboni, S., Tascedda, F., et al. (2021b). What can we teach Lymnaea and what can Lymnaea teach us? Biol. Rev. Camb. Philos. Soc. 96, 1590–1602. doi: 10.1111/brv.12716
Roovers, E., Vincent, M. E., van Kesteren, E., Geraerts, W. P., Planta, R. J., Vreugdenhil, E., et al. (1995). Characterization of a putative molluscan insulin-related peptide receptor. Gene 162, 181–188. doi: 10.1016/0378-1119(95)00323-x
Rosenzweig, M. R., Bennett, E. L., Colombo, P. J., Lee, D. W., and Serrano, P. A. (1993). Short-term, intermediate-term and long-term memories. Behav. Brain Res. 57, 193–198. doi: 10.1016/0166-4328(93)90135-d
Sadamoto, H., Kitahashi, T., Fujito, Y., and Ito, E. (2010). Learning-dependent gene expression of CREB1 isoforms in the molluscan brain. Front. Behav. Neurosci. 4:25. doi: 10.3389/fnbeh.2010.00025
Sadamoto, H., Sato, H., Kobayashi, S., Murakami, J., Aonuma, H., Ando, H., et al. (2004). CREB in the pond snail Lymnaea stagnalis: cloning, gene expression and function in identifiable neurons of the central nervous system. J. Neurobiol. 58, 455–466. doi: 10.1002/neu.10296
Saeki, S., Yamamoto, M., and Iino, Y. (2001). Plasticity of chemotaxis revealed by paired presentation of a chemoattractant and starvation in the nematode Caenorhabditis elegans. J. Exp. Biol. 204, 1757–1764. doi: 10.1242/jeb.204.10.1757
Saltiel, A. R., and Kahn, C. R. (2001). Insulin signalling and the regulation of glucose and lipid metabolism. Nature 414, 799–806. doi: 10.1038/414799a
Sasakura, H., and Mori, I. (2013). Behavioral plasticity, learning and memory in C. elegans. Curr. Opin. Neurobiol. 23, 92–99. doi: 10.1016/j.conb.2012.09.005
Semaniuk, U., Piskovatska, V., Strilbytska, O., Strutynska, T., Burdyliuk, N., Vaiserman, A., et al. (2021). Drosophila insulin-like peptides: from expression to functions–a review. Entomol. Exp. Appl. 169, 195–208. doi: 10.1111/eea.12981
Shimizu, M. (2021). “28B: Insulin-like growth factor-1,” in Handbook of Hormones (Vol. 1), 2nd Edn., eds H. Ando, K. Ukena, and S. Nagata (London: Academic Press), 285–288.
Shinjyo, N., and Kita, K. (2021). Infection and immunometabolism in the central nervous system: a possible mechanistic link between metabolic imbalance and dementia. Front. Cell. Neurosci. 15:765217. doi: 10.3389/fncel.2021.765217
Smit, A. B., Geraerts, P. M., Meester, I., van Heerikhuizen, H., and Joosse, J. (1991). Characterization of a cDNA clone encoding molluscan insulin-related peptide II of Lymnaea stagnalis. Eur. J. Biochem. 199, 699–703. doi: 10.1111/j.1432-1033.1991.tb16173.x
Smit, A. B., Spijker, S., Van Minnen, J., Burke, J. F., De Winter, F., Van Elk, R., et al. (1996). Expression and characterization of molluscan insulinrelated peptide VII from the mollusc Lymnaea stagnalis. Neuroscience 70, 589–596. doi: 10.1016/0306-4522(95)00378-9
Smit, A. B., Thijsen, S. F., Geraerts, W. P., Meester, I., van Heerikhuizen, H., and Joosse, J. (1992). Characterization of a cDNA clone encoding molluscan insulin-related peptide V of Lymnaea stagnalis. Brain Res. Mol. Brain Res. 14, 7–12. doi: 10.1016/0169-328x(92)90003-t
Smit, A. B., van Kesteren, R. E., Li, K. W., Van Minnen, J., Spijker, S., Van Heerikhuizen, H., et al. (1998). Towards understanding the role of insulin in the brain: lessons from insulin-related signaling systems in the invertebrate brain. Prog. Neurobiol. 54, 35–54. doi: 10.1016/s0301-0082(97)00063-4
Smit, A. B., van Marle, A., van Elk, R., Bogerd, J., van Heerikhuizen, H., and Geraerts, W. P. (1993). Evolutionary conservation of the insulin gene structure in invertebrates: cloning of the gene encoding molluscan insulin-related peptide III from Lymnaea stagnalis. J. Mol. Endocrinol. 11, 103–113. doi: 10.1677/jme.0.0110103
Smit, A. B., Vreugdenhil, E., Ebberink, R. H., Geraerts, W. P., Klootwijk, J., and Joosse, J. (1988). Growth-controlling molluscan neurons produce the precursor of an insulin-related peptide. Nature 331, 535–538. doi: 10.1038/331535a0
Steen, E., Terry, B. M., Rivera, E. J., Cannon, J. L., Neely, T. R., Tavares, R., et al. (2005). Impaired insulin and insulin-like growth factor expression and signaling mechanisms in Alzheimer’s disease – is this type 3 diabetes? J. Alzheimers Dis. 7, 63–80. doi: 10.3233/jad-2005-7107
Stein, G. M., and Murphy, C. T. (2012). The intersection of aging, longevity pathways and learning and memory in C. elegans. Front. Genet. 3:259. doi: 10.3389/fgene.2012.00259
Straub, V. A., and Benjamin, P. R. (2001). Extrinsic modulation and motor pattern generation in a feeding network: a cellular study. J. Neurosci. 21, 1767–1778. doi: 10.1523/JNEUROSCI.21-05-01767.2001
Sunada, H., Totani, Y., Nakamura, R., Sakakibara, M., Lukowiak, K., and Ito, E. (2017a). Two strains of Lymnaea stagnalis and the progeny from their mating display differential memory-forming ability on associative learning tasks. Front. Behav. Neurosci. 11:161. doi: 10.3389/fnbeh.2017.00161
Sunada, H., Lukowiak, K., and Ito, E. (2017b). Cerebral giant cells are necessary for the formation and recall of memory of conditioned taste aversion in Lymnaea. Zoolog. Sci. 34, 72–80. doi: 10.2108/zs160152
Tanabe, K., Itoh, M., and Tonoki, A. (2017). Age-related changes in insulin-like signaling lead to intermediate-term memory impairment in Drosophila. Cell Rep. 18, 1598–1605. doi: 10.1016/j.celrep.2017.01.053
Taylor, R., Soos, M. A., Wells, A., Argyraki, M., and Siddle, K. (1987). Insulin-like and insulin-inhibitory effects of monoclonal antibodies for different epitopes on the human insulin receptor. Biochem. J. 242, 123–129. doi: 10.1042/bj2420123
Thiel, G., Wagner, L., Ulrich, M., and Rössler, O. G. (2021). Immediate-early transcriptional response to insulin receptor stimulation. Biochem. Pharmacol. 192:114696. doi: 10.1016/j.bcp.2021.114696
Thorpe, A., and Duve, H. (1988). Invertebrate neuroendocrinology. Insulin found at last? Nature 331, 483–484. doi: 10.1038/331483a0
Tomioka, M., Adachi, T., Suzuki, H., Kunitomo, H., Schafer, W. R., and Iino, Y. (2006). The insulin/PI 3-kinase pathway regulates salt chemotaxis learning in Caenorhabditis elegans. Neuron 51, 613–625. doi: 10.1016/j.neuron.2006.07.024
Tomioka, M., Jang, M. S., and Iino, Y. (2022). DAF-2c signaling promotes taste avoidance after starvation in Caenorhabditis elegans by controlling distinct phospholipase C isozymes. Commun. Biol. 5:30. doi: 10.1038/s42003-021-02956-8
Torayama, I., Ishihara, T., and Katsura, I. (2007). Caenorhabditis elegans integrates the signals of butanone and food to enhance chemotaxis to butanone. J. Neurosci. 27, 741–750. doi: 10.1523/JNEUROSCI.4312-06.2007
Totani, Y., Aonuma, H., Oike, A., Watanabe, T., Hatakeyama, D., Sakakibara, M., et al. (2019). Monoamines, insulin and the roles they play in associative learning in pond snails. Front. Behav. Neurosci. 13:65. doi: 10.3389/fnbeh.2019.00065
Totani, Y., Kotani, S., Odai, K., Ito, E., and Sakakibara, M. (2020a). Real-time analysis of animal feeding behavior with a low-calculation-power CPU. IEEE Trans. Biomed. Eng. 67, 1197–1205. doi: 10.1109/TBME.2019.2933243
Totani, Y., Nakai, J., Hatakeyama, D., and Ito, E. (2020b). Memory-enhancing effects of short-term fasting. Eur. Zoolog. J. 87, 597–602. doi: 10.1080/24750263.2020.1827053
Totani, Y., Nakai, J., Dyakonova, V. E., Lukowiak, K., Sakakibara, M., and Ito, E. (2020c). Induction of LTM following an insulin injection. eNeuro 7: ENEURO.0088-20.2020. doi: 10.1523/ENEURO.0088-20.2020
Totani, Y., Nakai, J., Hatakeyama, D., Dyakonova, V. E., Lukowiak, K., and Ito, E. (2022). CNS serotonin content mediating food deprivation-enhanced learning is regulated by hemolymph tryptophan concentration and autophagic flux in the pond snail. Nutr. Neurosci. . [Online ahead of print]. doi: 10.1080/1028415X.2022.2033045
Veenstra, J. A. (2021). Ambulacrarian insulin-related peptides and their putative receptors suggest how insulin and similar peptides may have evolved from insulin-like growth factor. PeerJ 9:e11799. doi: 10.7717/peerj.11799
Veenstra, J. A., Leyria, J., Orchard, I., and Lange, A. B. (2021). Identification of gonadulin and insulin-like growth factor from migratory locusts and their importance in reproduction in Locusta migratoria. Front. Endocrinol. (Lausanne) 12:693068. doi: 10.3389/fendo.2021.693068
Wagatsuma, A., Sadamoto, H., Kitahashi, T., Lukowiak, K., Urano, A., and Ito, E. (2005). Determination of the exact copy numbers of particular mRNAs in a single cell by quantitative real-time RT-PCR. J. Exp. Biol. 208, 2389–2398. doi: 10.1242/jeb.01625
Wakabayashi, T., Yamaguchi, K., Matsui, K., Sano, T., Kubota, T., Hashimoto, T., et al. (2019). Differential effects of diet- and genetically-induced brain insulin resistance on amyloid pathology in a mouse model of Alzheimer’s disease. Mol. Neurodegener. 14:15. doi: 10.1186/s13024-019-0315-7
Wang, B., Goode, J., Best, J., Meltzer, J., Schilman, P. E., Chen, J., et al. (2008). The insulin-regulated CREB coactivator TORC promotes stress resistance in Drosophila. Cell Metab. 7, 434–444. doi: 10.1016/j.cmet.2008.02.010
Yamanaka, M., Hatakeyama, D., Sadamoto, H., Kimura, T., and Ito, E. (2000). Development of key neurons for learning stimulates learning ability in Lymnaea stagnalis. Neurosci. Lett. 278, 113–116. doi: 10.1016/s0304-3940(99)00916-7
Yamanaka, A., Kobayashi, S., Matsuo, Y., and Matsuo, R. (2021). FxRIamide regulates the oscillatory activity in the olfactory center of the terrestrial slug Limax. Peptides 141:170541. doi: 10.1016/j.peptides.2021.170541
Yin, J. C., Del Vecchio, M., Zhou, H., and Tully, T. (1995). CREB as a memory modulator: induced expression of a dCREB2 activator isoform enhances long-term memory in Drosophila. Cell 81, 107–115. doi: 10.1016/0092-8674(95)90375-5
Keywords: Caenorhabditis elegans, classical conditioning, Drosophila, insulin-like growth factor, memory, Lymnaea, starvation, insulin
Citation: Nakai J, Chikamoto N, Fujimoto K, Totani Y, Hatakeyama D, Dyakonova VE and Ito E (2022) Insulin and Memory in Invertebrates. Front. Behav. Neurosci. 16:882932. doi: 10.3389/fnbeh.2022.882932
Received: 24 February 2022; Accepted: 04 April 2022;
Published: 26 April 2022.
Edited by:
Makoto Mizunami, Hokkaido University, JapanReviewed by:
Ildikó Kemenes, University of Sussex, United KingdomIstván Fodor, Eötvös Loránd Research Network, Hungary
Copyright © 2022 Nakai, Chikamoto, Fujimoto, Totani, Hatakeyama, Dyakonova and Ito. This is an open-access article distributed under the terms of the Creative Commons Attribution License (CC BY). The use, distribution or reproduction in other forums is permitted, provided the original author(s) and the copyright owner(s) are credited and that the original publication in this journal is cited, in accordance with accepted academic practice. No use, distribution or reproduction is permitted which does not comply with these terms.
*Correspondence: Etsuro Ito, ZWl0b0B3YXNlZGEuanA=