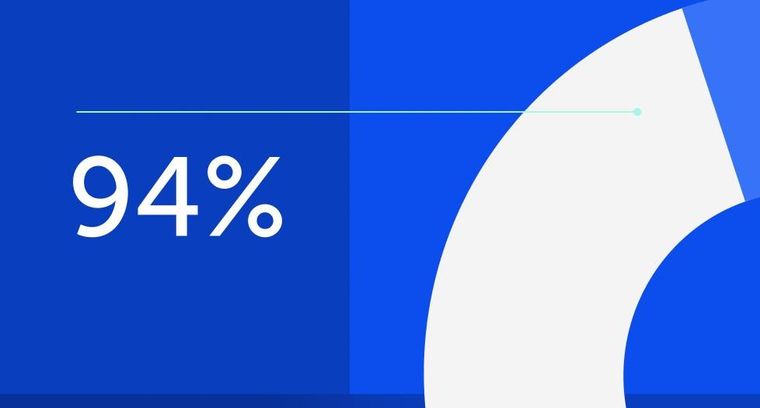
94% of researchers rate our articles as excellent or good
Learn more about the work of our research integrity team to safeguard the quality of each article we publish.
Find out more
ORIGINAL RESEARCH article
Front. Behav. Neurosci., 08 July 2022
Sec. Behavioral Endocrinology
Volume 16 - 2022 | https://doi.org/10.3389/fnbeh.2022.869526
This article is part of the Research TopicNew Insights into the Role of the Vagus Nerve in Health and Disease: Basic and Clinical StudiesView all 11 articles
Background: Vagus nerve is one of the crucial routes in communication between the immune and central nervous systems. The impaired vagal nerve function may intensify peripheral inflammatory processes. This effect subsides along with prolonged recovery after permanent nerve injury. One of the results of such compensation is a normalized plasma concentration of stress hormone corticosterone – a marker of hypothalamic-pituitary-adrenal (HPA) axis activity. In this work, we strive to explain this corticosterone normalization by studying the mechanisms responsible for compensation-related neurochemical alterations in the hypothalamus.
Materials and Methods: Using microarrays and high performance liquid chromatography (HPLC), we measured genome-wide gene expression and major amino acid neurotransmitters content in the hypothalamus of bilaterally vagotomized rats, 1 month after surgery.
Results: Our results show that, in the long term, vagotomy affects hypothalamic amino acids concentration but not mRNA expression of tested genes.
Discussion: We propose an alternative pathway of immune to CNS communication after vagotomy, leading to activation of the HPA axis, by influencing central amino acids and subsequent monoaminergic neurotransmission.
The vagus nerve, due to its extensive peripheral projection range, is a prominent interoceptive pathway (Forsythe et al., 2014). The vagus nerve transmits intraperitoneal immune signals to the CNS (Watkins and Maier, 2005; Quan and Banks, 2007; Dantzer, 2009; D’Mello and Swain, 2016), causing neurotransmission alterations (Wieczorek et al., 2005; Dunn, 2006; Hopkins, 2007), behavioral changes (Wieczorek et al., 2005; Dantzer and Kelley, 2007; Dantzer et al., 2008) and indirect activation of the hypothalamus (Wieczorek and Dunn, 2006; Noori et al., 2017) a first element of the hypothalamic-pituitary-adrenal (HPA) axis of stress. HPA activation results in secretion of glucocorticoids (GCs), which are important regulators of the immune system (Hosoi et al., 2000). Moreover, the parasympathetic part of the vagus nerve can directly influence the activity of immune cells in internal organs exposed to pathogens through the cholinergic anti-inflammatory pathway (Tracey, 2007; Karimi et al., 2010; Pereira and Leite, 2016).
The physiological tonic activity of the vagus nerve (so-called vagal tone) is crucial for maintaining body allostasis. Vagal tone is a critical regulator of the inflammatory response. Low vagal tone results in increased basal plasmatic cortisol, tumor necrosis factor α (TNF-α), and epinephrine levels, which Bonaz et al. (2016) summarized as a pro-inflammatory effect (Pellissier et al., 2014; Bonaz et al., 2016). Vagal tone is also involved in regulating emotional and behavioral responsiveness as well as sickness behaviors – a natural response to ongoing inflammation, which supports the immune system in its fight against the pathogen (Porges et al., 1994; Porges, 1995). The experimental model of abolished vagal tone in context of peritoneal vagal functions is a full subdiaphragmatic truncal vagotomy, which we used in the current experiment.
Vagus nerve integrity is necessary for proper inhibition of inflammatory response. Thus, vagotomy results in highly intensified inflammation and decreased secretion of anti-inflammatory GCs. Surprisingly, these effects diminish after a prolonged period of recovery following vagotomy (Ghia et al., 2007; Mitsui et al., 2014).
Previously, we reported that 30 days after subdiaphragmatic vagotomy LPS-induced increase of plasma corticosterone is similar to the control group (Kobrzycka et al., 2019). At the same time, we reported that monoaminergic neurotransmission is altered in the hypothalamus after a prolonged period of recovery following vagotomy, which might contribute to the restoration of HPA axis activity. These neurotransmission changes may occur due to the presence of compensatory mechanisms for impaired immune sensory and anti-inflammatory functions of the vagus nerve. Wieczorek and Dunn (2006) suggested that function of the damaged vagal nerve is substituted by COX-dependent pathway, where immune signals are carried by blood-borne prostaglandin E2 (PGE2). Corroborating this hypothesis, we previously observed that the concentration of PGE2 under inflammatory conditions is increased in the plasma of vagotomized animals, 30 days after vagotomy procedure (Kobrzycka et al., 2019).
In this work, we present a data set investigated simultaneously with those presented previously (Kobrzycka et al., 2019). Here, we investigate whether transcriptomic changes and/or altered amino acid neurotransmission accompanies monoaminergic alterations in the hypothalamus and, in consequence, may be responsible for the preservation of HPA axis activity after recovery from vagotomy. To test those hypotheses, we performed an RNA microarray with subsequent RT-qPCR analysis and high performance liquid chromatography (HPLC) concentration analysis of excitatory and inhibitory amino acid neurotransmitters.
The studies were performed on 3-month-old male Wistar rats (300 g ± 25 g, N = 75). Animals were individually housed in breeding cages under the following conditions: free access to water and feed (Purina granules), artificial lighting conditions (a 12-h day-night cycle, light on at 7.00 AM), temperature 21–22°C and 60–65% humidity. Before the start of the experiments, the animals were habituated for 7 days to the conditions in the animal facility.
The animals were divided into two main groups: sham operated (SH, n = 31) and subdiaphragmatically vagotomized (VG, n = 33). Animals in the VG group were subjected to surgery under general anesthesia, induced by an intraperitoneal injection of Innovar plus (6 μl/g body weight) and local anesthesia with 2% lidocaine solution (0.5 ml/animal). During operation, a small fragment of gastrointestinal and hepatic branches of the vagal nerve was cut just below the diaphragm. To prevent post-operative infection and to facilitate wound healing, an antibacterial agent (Alu Spray, V.M.D.) was applied to the operation site. Bilateral subdiaphragmatic truncal vagotomy procedure and its validation are described in detail in Kobrzycka et al. (2019). Sham operation (SH) was performed in an analogous way to subdiaphragmatic vagotomy, with exception of cutting the nerves. Following surgeries, the animals were returned to their cages, and after a 30-day-long recovery period, the animals from SH and VG groups were intraperitoneally injected with either saline (0.9% NaCl, 100 μl i.p., SH n = 15, VG n = 15) or LPS (10 μg E. coli 026:B6 in 100 μl 0.9% NaCl, 100 μl i.p., SH n = 16, VG n = 18). After 120 min, the animals were decapitated.
Immediately after decapitation, hypothalamus (HPT) samples, restricted mainly to the medial part containing the paraventricular nucleus (PVN), were taken. Immediately after decapitation, the brain was cut on a glass plate kept on ice. After the section, samples assigned for HPLC analysis were placed in tubes on dry ice, weighed, and processed in homogenization buffer – the whole procedure took up to 5 min. The samples assigned for RNA analysis were placed in RNA-later solution right after dissection (within 2–3 min after decapitation). HPT samples weighted on average 0.05 g (±0.02 g). Stereotactic coordinates used to locate HPT were: AP = −1.44 to −2.04, L = ±1.5, H = −8 to −10 according to the Paxinos and Watson stereotaxic atlas (Paxinos and Watson, 1998).
For the enzyme-linked immunosorbent assay (ELISA) test of pro-inflammatory cytokines, plasma was obtained by centrifuging the trunk blood collected on EDTA (1 ml/100 μl of Na2 EDTA), 4,000 rpm for 10 min at 4°C. The obtained plasma was frozen and stored at −80°C until future analysis. For HPLC analysis of amino acid neurotransmitters, hypothalamuses (SH NaCl n = 6, VG NaCl n = 7, SH LPS n = 7, VG LPS n = 8) were immediately homogenized using an ultrasonic homogenizer (Fisher BioBlock Scientific, France) for 15 s in 150 μl homogenization solution (0.4 mM Na2S2O5, 0.6 mM HClO4), and centrifuged at 12,000 rpm for 15 min at 4°C. At least 100 μl of the supernatant was collected from each sample, transferred to chromatographic tubes and frozen at −80°C.
For microarray and PCR analyses, hypothalamuses (naive control CNT n = 11, SH NaCl n = 9, VG NaCl n = 8, SH LPS n = 9, VG LPS n = 10) were stored in −20°C in 200 μl of RNA-later (Thermo Fisher Scientific, United States). Total RNA was extracted from dissected hypothalamuses using a spin column-based Universal RNA Purification Kit (EURx, Poland) according to the manufacturer’s protocol. RNA samples were subjected to DNase treatment on-column (RNase-free DNase I, EURx, Poland, 15 min, room temperature) as well as in-sample (TURBO DNA-free Kit, Thermo Fisher Scientific, United States, 30 min, 37°C), to remove any residual DNA contamination. The quantity, purity, and quality of RNA samples were estimated using a NanoDrop spectrophotometer (Thermo Fisher Scientific, United States) and a Bioanalyzer 2100 microcapillary electrophoresis device (Agilent, United States) with RNA 6000 Nano Assay Kit (Agilent, United States). Samples were of high quality (RNA Integrity Number >9) and purity (see Supplementary Table 1).
Tumor Necrosis Factor α (TNF-α) concentration (SH NaCl n = 11, VG NaCl n = 10, SH LPS n = 11, VG LPS n = 12) was established as a control variable for confirming ongoing intraperitoneal inflammation. Plasma TNF-α concentration was determined using the Rat TNF-α ELISA Kit, from Diaclone, France (cat. no. 865.000), according to the manufacturer’s instructions. The same statistical assumptions and analytical procedure for HPLC data were used.
The concentration of the amino acid neurotransmitters: aspartate (ASP), glutamate (GLU), glycine (GLY), gamma-aminobutyric acid (GABA), tyrosine (TYR), and tryptophan (TRP) was determined in prepared HPT samples with RP-HPLC-ED gradient method with pre-column derivatization with fresh OPT-thiol reagent (0.1 M Borax, 0.5% OPT, 0.9% mercaptoethanol). The Agilent 1100 chromatographic system with Waters, AccQ-Tag for hydrolysate Amino Acid analysis chromatographic column (3.9 × 150 mm) preceded by a ZORBAX SB-C18 pre-column (4.6 × 12.5 mm) was used. The column temperature was set at 37°C and mobile phase flow at 0.4 ml/min. The carbon working electrode was set at +0.5 V, relative to the Ag/AgCl reference electrode. The gradient elution was performed using phosphate buffer (5.5 pH) containing: at 0.05 M NaH2PO4 × H2O, and addition of methanol; in buffer (A) 20% and (B) 80%. At the start, the mobile phase consisted of 100% buffer A. During the first 10 min of analysis, the concentration of buffer B was raised to 10% (in the 10th min: 90% A and 10% B). In the next 30 min, the content of buffer B in the mobile phase was raised from 10 to 85% (in 40th min: 15% A and 85% B). In the next 5 min, the content of buffer B was decreased to 0% (in the 45th min: 100% A and 0% B). After each sample, the system was re-equilibrated for 10 min. The chromatographic data were analyzed using ChemStation, Revision-B.03.02, Agilent software.
High performance liquid chromatography (HPLC) and TNF-α data were tested for normality (Shapiro–Wilk test) and homogeneity of variance (Levene’s test). Because some data did not meet the assumptions of the parametric test, we performed a Box-Cox transformation. Next, ANOVA with the Bonferroni post hoc test was used. Results of statistical tests and Box-Cox transformation formulas are presented Supplementary Table 5. The p-values lower than 0.05 were considered statistically significant. These statistical analyses were performed using the STATISTICA software, version 13.3 TIBCO Software Inc (2017).
Microarrays were used to analyze hypothalamic gene expression in all four experimental groups. The results of this analysis were validated using real-time qPCR.
A total of 21 samples (3–5 per experimental group, see Supplementary Table 1) were used for microarray analysis. These comprised the entire set of samples available in the experiment at that time. Samples were analyzed individually; 100 ng of the given sample that passed the initial quality control screen (2100 Bioanalyzer, Agilent) was labeled and amplified using GeneChip WT PLUS Reagent Kit and hybridized to an Affymetrix Rat Gene 2.1 ST Array Strip microarray using GeneAtlas Hybridization, Wash, and Stain Kit for WT Array Strips. GeneChip Poly-A RNA Control Kit and GeneChip Hybridization Controls were used to monitor the process of sample preparation. Hybridization to the microarrays was conducted for 20 h at 48°C. Following the hybridization, the microarrays were washed, stained, and scanned on a GeneAtlas™ System for Academic Customers. All the reagents and equipment for the microarray experiment were provided by Affymetrix, a subsidiary of Thermo Fisher Scientific, United States, and the procedures were performed according to the manufacturer’s protocol. The quality of the analyses was verified using Affymetrix Expression Console Software and standard Affymetrix quality metrics.
All Affymetrix.cel files were first imported into Partek® Genomics Suite® software, version 7, build 7.19.1125 (Partek, United States). Prior to any computations, the imported data set was normalized. Background correction was conducted with the use of the RMA method (Bolstad et al., 2003) and was followed by quantile normalization. Probes were logged using base 2, while the median polish (Mosteller and Tukey, 1977) algorithm was applied to probe set summarization. The definition of all contrasts of interest (comparisons of interest) constituted a base for the detection of differentially expressed genes, which was performed with the use of the ANOVA feature of Partek® Genomics Suite®. As a result, every feature’s description contained (among others) p-value for a particular comparison accompanied by its FDR-corrected (Mosteller and Tukey, 1977) value and 95% confidence interval for those. The data set was annotated with the Ensembl Transcripts, release 100, genome assembly Rnor_6.0.
The results of the microarray analysis were validated using SYBR Green-based real-time qPCR. A total of 47 samples (including 21 samples used for microarray experiment, 8–11 samples per each experimental group, see Supplementary Table 1) were used for qPCR analysis. For each sample, 100 ng of total RNA was reverse-transcripted to cDNA using Smart RT-PCR Kit (EURx, Poland). Expression of 7 genes (Actn2, Cxcl14, Gabrg1, Gria1, Oxt, Rab1b, and Vim) was studied. Hmbs was used as a reference gene. It was identified as the most stably expressed out of a set of candidate reference genes (Gapdh, Hmbs, Hprt1, Tbp, and Yhwaz) using the NormFinder tool (see Supplementary Table 3). For details on the method of selecting the reference gene, see our previous work (Stankiewicz et al., 2015). For detailed primers description, see Supplementary Table 2. Rotor-Gene Q (Qiagen, Netherlands) with Fast SG qPCR Master Mix (2x) (EURx, Poland) were used to perform qPCR. qPCR reaction conditions were as follows: (1) denaturation −95°C for 50 s; (2) amplification, 35 cycles – 95°C for 10 s then 10 s in primer-specific annealing temperature then 72°C for 15 s; (3) melting curve analysis – 72–95°C. All assays were executed in triplicate. Each run included five 4-fold serial dilutions for calculating reaction efficiency. A melting curve analysis was performed to verify the presence of one gene-specific peak and the absence of primer-dimer peaks. The Pfaffl model was used to calculate relative expression ratios of studied genes. Detailed data on qPCR analysis are presented in Supplementary Table 2. qPCR was performed according to the Minimum Information for Publication of Quantitative Real-Time PCR Experiments (MIQE) guidelines (Bustin et al., 2009). Shapiro–Wilk test was used to test the normality of qPCR data. As the data were not distributed normally, Mann–Whitney–Wilcoxon test was used to investigate the significance of comparisons, which showed a non-adjusted p-value lower than 0.05 in the microarray experiment. As multiple comparisons were made within each analyzed gene, the Benjamini-Hochberg method (Benjamini and Hochberg, 1995) was used to control False Discovery Rate. The R script used for statistical analysis of the results can be found in Supplementary Presentation 1.
Increased plasma TNF-α (Figure 1) concentration confirms the development of inflammatory conditions in both rat groups injected with LPS [F(3,34) = 28.191, p < 0.001, SH NaCl vs. SH LPS p < 0.001, VG NaCl vs. VG LPS p < 0.001]. However, the inflammatory response of vagotomized animals seems to be stunted in comparison to sham control, which is in line with observations made by Ghia et al. (2007).
Figure 1. Increased plasma tumor necrosis factor α (TNF-α) concentration after intraperitoneal injection of LPS confirm initiation of pro-inflammatory activity of the immune system. Details in text.
Statistical analysis revealed significant differences between groups in concentrations of all measured hypothalamic amino acids concentration (Figure 2) [TRP F(3,21) = 18.292, p < 0.001, TYR F(3,21) = 10.419, p < 0.001, ASP F(3,21) = 15.473, p < 0.001, GLU F(3,21) = 8.019, p < 0.001, GABA F(3,21) = 18.020, p < 0.001, GLY F(3,21) = 20.72, p < 0.001]. Intraperitoneal injection of LPS did not affect hypothalamic TRP, ASP, and GABA, concentrations in SH group (SH NaCl vs. SH LPS: TRP p = 0.077, ASP p = 1, GABA p = 0.604). Subdiaphragmatic vagotomy significantly increased the concentration of these amino acids despite LPS conditions (SH NaCl vs. VG NaCl: TRP p = 0.001, ASP p = 0.011, GABA p < 0.001; SH LPS vs. VG LPS: TRP p < 0.001, ASP p < 0.001, GABA p = 0.041). Worth noting, GABA concentration in VG group is increased after LPS administration (VG NaCl vs. VG LPS p = 0.006). On the other hand, intraperitoneal injection of LPS significantly increased hypothalamic TYR, GLY, and GLU concentrations in SH group (SH NaCl vs. SH LPS: TYR p = 0.024, GLU p = 0.025, GLY p < 0.001). Subdiaphragmatic vagotomy significantly increased TYR, GLY, and GLU concentrations in non-septic conditions (SH NaCl vs. VG NaCl: TYR p = 0.003, GLU p = 0.024, GLY p < 0.001). Also, after LPS administration, TYR, GLY, and GLU concentrations are elevated in similar manner as VG NaCl group – significant increased compare to SH NaCl group conditions (SH NaCl vs. VG LPS: TYR p < 0.001, GLU p < 0.001, GLY p < 0.001) and no differences between vagotomized groups (VG NaCl vs. VG LPS: TYR p = 1, GLU p = 0.886, GLY p = 1). Therefore, no significant differences between SH LPS and VG LPS are observed (SH LPS vs. VG LPS: TYR p = 0.262, GLU p = 0.840, GLY p = 0.619).
Figure 2. Results of high performance liquid chromatography (HPLC) analysis of amino acids in hypothalamus. Concentrations of monoaminergic neurotransmitter precursors (Tyrosine, Tryptophan) and inhibitory amino acid neurotransmitters (GABA, Glycine) are increased in vagotomized group (VG NaCl vs. SH NaCl). Similar effect is absent in case of excitatory amino acid neurotransmitters (Glutamate, Aspartate). During intraperitoneal inflammation changes in tyrosine and tryptophan concentrations in vagotomized group (VG LPS vs. SH LPS) are even more pronounced. Additionally, we observe a significant increase of inhibitory GABA and excitatory aspartate concentration in that group (VG LPS vs. SH LPS). Details in text.
Using microarrays, we have analyzed transcriptomic patterns induced by experimental conditions in the hypothalamus. None of the observed statistically significant changes in expression of analyzed transcripts survived adjustment for multiple comparisons. Nevertheless, we have selected 7 genes characterized by relatively low non-adjusted p-values in some of the comparisons (mean = 0.019, SD = 0.01, also see Supplementary Table 4), and analyzed their expression using qPCR (Figure 3). The qPCR analysis showed that the studied genes (Actn2, Cxcl14, Gabrg1, Gria1, Oxt, Rab1b, and Vim) do not respond to experimental conditions, thus supporting data from microarrays (see Supplementary Table 4).
Figure 3. QPCR analysis confirms that experimental procedures did not affect the hypothalamic gene expression pattern. The whiskers extend from the hinge to the smallest/largest value no further than 1.5 × IQR from the hinge (where IQR is the inter-quartile range, or distance between the first and third quartiles). Data beyond the end of the whiskers are called “outlying” points and are plotted individually.
Vagal denervation attenuates inflammation-induced increase of plasma corticosterone and ACTH concentrations for up to 2 weeks (Fleshner et al., 1995; Wieczorek and Dunn, 2006). Afterward, this effect of vagotomy is diminished. This indicates that the proper functioning of the anti-inflammatory HPA axis is restored in the long run.
However, even after 1 month following a vagotomy, a monoaminergic neurotransmission in the hypothalamus (a first element of the anti-inflammatory HPA axis), as well as in other limbic system structures, is deregulated (Kobrzycka et al., 2019). We confirmed these results in this work by observing an increase in a number of precursors of the monoaminergic neurotransmitters (tyrosine and tryptophan) in the hypothalamus of the vagotomized animals. In fact, a similar tendency to increase tryptophan concentration was also noticed by Wieczorek and Dunn (2006) in many brain areas of vagotomized mice. CNS may require higher amounts of the precursors because they are necessary for reported increased dopamine and serotonin synthesis and turnover in many brain regions after the subdiaphragmatic vagotomy (Wieczorek and Dunn, 2006; Kobrzycka et al., 2019). It is also worth noting that one of the side effects of subdiaphragmatic vagotomy procedure is a slowed movement of food through the digestive system (Martin et al., 1977), which also may affect the content of tyrosine and tryptophan in blood (Fernstrom and Fernstrom, 2007; Kapalka, 2010; Al Mushref and Srinivasan, 2013; Fu et al., 2014).
Hypothalamus is innervated by monoaminergic fibers originating from a number of brain areas directly or indirectly affected by nucleus tractus solitarius (NTS), which distributes the sensory information from the vagus nerve (Rinaman, 2007; Myers et al., 2012; Elson and Simerly, 2015; Miller and Yeh, 2017; Weidenfeld and Ovadia, 2017). Those innervations modulate hypothalamic functions, i.e., by affecting hypothalamic concentrations of amino acid neurotransmitters (e.g., GABA and Glycine) and, subsequently, post-synaptic potentials (Miller and Yeh, 2017). Previously, we observed vagotomy caused changes in monoaminergic neurotransmission in many of those areas (e.g., AM, PAG, and HIP) and the hypothalamus itself. In this work, we show a modest increase in the hypothalamic concentration of amino acid neurotransmitters in full-recovered animals subjected previously to vagotomy as compared to sham procedure. Similar results were reported by Klarer et al. (2014), who studied amino acid neurotransmitters in several other limbic brain structures. Based on these results, we hypothesize that those vagotomy-induced changes in hypothalamic amino acid neurotransmitters may be caused by deregulated monoaminergic signaling originating in limbic structures.
Glutamate, aspartate, and glycine innervation of the hypothalamus originate from the brainstem and internal hypothalamic circuit (Ziegler et al., 2005; Noori et al., 2017; Varga et al., 2019). Glutamate, aspartate, and glycine inhibit CRH release from the hypothalamus in vitro (Buckingham and Hodges, 1977; Patchev et al., 1994). In our experiment, we observed increased levels of all of these neurotransmitters in the vagotomized group regardless of LPS-induced inflammation. Such observation suggests suppression of CRH release and in consequence HPA axis activity. However, such a hypothesis would be in opposition to the previously mentioned restoration of LPS-induced corticosterone increase in long-term recovered vagotomized rats. This apparent incompatibility might be explained by intensified GABA concentration in the hypothalamus.
In basal conditions, the tonic, inter-hypothalamic GABA circuit suppresses the activity of hypothalamic CRH-releasing neurons (Kovács et al., 2004). Our results show that this mechanism is intensified in vagotomized animals compared to sham-operated animals. We propose that this intensification of basal hypothalamic GABA release may underlie reported down-regulation of corticosterone release in animals that underwent vagotomy procedure a few days before measurement (Fleshner et al., 1995; Wieczorek and Dunn, 2006). As we report here, 30 days after vagotomy procedure, hypothalamic GABA concentration is even higher in inflammatory conditions. We think that this may be a result of enhanced external inhibitory signals, especially amygdalic GABA-GABA disinhibitory connections (Herman et al., 2004), which would inhibit the activity of the inter-hypothalamic GABA circuits. Thus, the internal hypothalamic inhibition of CRH release would be suppressed, and in consequence, hypothalamic hormonal activity and further adrenal corticosterone release in response to inflammation would be the same as in sham animals (Kobrzycka et al., 2019). This is indeed what we observe in our studies. However, this hypothesis requires further, more detailed investigation.
Another amino acid crucial for hypothalamic functioning is glycine. Glycine inhibits orexin- mediated arousal, energy homeostasis, and reward-seeking. It also promotes sleep and decreases body temperature during sleep episodes via peripheral vasodilation (Kawai et al., 2015). In conscious rats, glycine acts in PVN to enhance the renal excretion of water and sodium and decrease central sympathetic outflow to the heart and kidneys (Krowicki and Kapusta, 2011). Glycine can act allosterically as an excitatory modulator of the NMDA subtype of ionotropic glutamate receptors and through the activation of NMDA receptors in suprachiasmatic nucleus (SCN). Glycine is also involved in oxytocin and vasopressin synthesis in magnocellular neurosecretory cells (MNCs) in the PVN and SON (supraoptic nucleus) and a significant tonic inhibitory effect is mediated through ionotropic GlyR (Choe et al., 2016).
In the context of HPA axis activity, glycine can inhibit hypothalamic vasopressin synthesis (Hussy et al., 2001; Choe et al., 2016) which synergistically to CRH stimulates ACTH secretion (Mazzocchi et al., 1997; Papadimitriou and Priftis, 2009). Glycine also decreased the spontaneous release of CRH from the hypothalamus but does not affect hypothalamic CRH content (Buckingham and Hodges, 1977). This observation comes, however, from a study carried out on isolated hypothalamic tissue with restrictive controlled neurotransmission alterations – that does not consider simultaneously changes of other neurotransmitters content. In vivo studies showed that intracerebroventricular (Rundgren et al., 1993) or intravenous (Hahn et al., 1991) glycine infusion may increase vasopressin plasma levels. This difference originating from the different routes of glycine administration might be an important factor in the interpretation of our results.
The raphe magnus and the ventrolateral periaqueductal gray were found to be the exclusive sources of the inhibitory glycinergic innervation in the PVN (Varga et al., 2019). Although sufficient amounts of glycine are synthesized de novo, exogenous glycine passively diffuses across the blood-brain barrier and modulates neurotransmission in the CNS (Kawai et al., 2015). Observed after vagotomy increase in hypothalamic glycine concentration might result from altered neurotransmission of other brain structures, but also, as mentioned before, from altered after vagotomy peristalsis.
We do not know how the immune signals reach the CNS in vagotomy conditions in the early stages of inflammation. We think that this may be an effect of an alternative mechanism replacing disrupted vagus-mediated signaling pathway. Wieczorek and Dunn (2006) suggested that this mechanism might be associated with changes in prostaglandin signaling. In fact, Dalli et al. (2017) showed that even unilateral subdiaphragmatic truncal vagotomy significantly alters macrophage lipid profile, which is involved in the metabolism of arachidonic acid during intraperitoneal inflammation. Additionally, they reported that following left trunk vagotomy, the expression of COX2, an enzyme involved in PGE2 synthesis, significantly increased in macrophages (Dalli et al., 2017). We consider that elevation of inflammation-induced PGE2 synthesis may take over immune-to-CNS communication after vagotomy. We think that elevation of inflammation-induced PGE2 synthesis may take over immune-to-CNS communication after vagotomy, as we suggested previously (Kobrzycka et al., 2019). In theory, blood-borne PGE2 reaches the brain via the bloodstream, where it acts upon epithelial cells of blood vessels. These vessels preserve intact prostaglandin-related cellular machinery after vagotomy (Sergeev and Akmaev, 2000). Once at the brain, PGE2 activates CVOs, PVN, and NTS (Sekiyama et al., 1995; Ek et al., 1998; Rocca and FitzGerald, 2002; Cai et al., 2014; Le Maître et al., 2015) and subsequently, other brain areas in a way similar to the now non-functional vagus nerve (Sekiyama et al., 1995; Marty et al., 2008).
Some authors proposed an alternative to the vagal, neural route of immune to CNS communication. Splanchnic sympathetic nerves were shown to control inflammation processes, induced by intravenous administration of LPS (Martelli et al., 2014, 2019). It was proposed that splanchnic sympathetic nerves constitute an efferent part of the inflammatory reflex along with vagal afferents or/and bloodstream as sensory routes of immune to CNS communication (Komegae et al., 2018; McAllen et al., 2022). We cannot exclude that after abdominal vagal denervation, part of immune sensory functions is taken over by other sensory fibers. However, we could not find information about such a process occurring during intraperitoneal inflammation. Until such a process is observed, the vagus nerve is still considered the main immune sensory neural pathway from the abdominal cavity to CNS (Pavlov and Tracey, 2017).
Microarray and subsequent Real-Time qPCR analysis suggest that the transcriptome does not affect the activity of the hypothalamus during inflammation in vagotomized rats after a 30-day-long recovery period. It would be interesting to see whether gene expression changes are more pronounced at earlier stages of recovery while later giving way to other compensatory mechanisms such as neurotransmission alterations. On the other hand, we found that amino acid neurotransmitters are deregulated in the hypothalamus of vagotomized animals after prolonged recovery. This, along with our previous finding on deregulated monoamine systems in brain structures affecting the functioning of the hypothalamus, suggests that vagal role in the regulation of HPA axis after a longer recovery period is replaced by changes in external neurotransmitter modulation of hypothalamic activity rather than alteration of basic hypothalamic functionality. Of course, the presented data do not fully describe the observed phenomena of restored HPA axis activity in recovered vagotomized animals. Previously, we presented changes in CNS monoaminergic neurotransmission. Here, we report changes in amino acid components in the hypothalamus. In neuronal tissue, amino acid neurotransmitters play a critical role in electrophysiological processes not investigated in this study. We also pay attention that observed changes might be evolving in time along with prolonged recovery after vagotomy. For future studies, we would like to extend our research to a few time points that allow us to establish the time dynamic of presented processes. For now, we simply report that hypothalamic amino acid neurotransmission is altered 30 days after vagotomy and it is an interesting issue for discussion and further investigation.
The datasets presented in this study can be found in online repositories. The names of the repository/repositories and accession number(s) can be found below: https://www.ncbi.nlm.nih.gov/geo/query/acc.cgi?acc=GSE199231.
The animal study was reviewed and approved by the Local Ethical Committee for Animal Experiments in Łodz, 73/ŁB582/2012.
AK wrote the manuscript, isolated material for the microarray and qPCR analyses, and performed the qPCR and HPLC analysis as well as statistical analysis of the HPLC and ELISA results. AS wrote the manuscript, and oversaw and participated in isolation of the material for the microarray and qPCR analyses and coordinated them. MW designed the experiment, performed the experimental procedures on living animals, and collected the samples. JG performed the bioinformatic and statistical analyses of microarray data. MG, BB, and RI-N performed the microarray analysis. KP-K performed the ELISA tests. All authors contributed to the article and approved the submitted version.
This work was supported by the Polish National Science Center (NCN, UMO 2012/07/B/NZ4/00205).
The authors declare that the research was conducted in the absence of any commercial or financial relationships that could be construed as a potential conflict of interest.
All claims expressed in this article are solely those of the authors and do not necessarily represent those of their affiliated organizations, or those of the publisher, the editors and the reviewers. Any product that may be evaluated in this article, or claim that may be made by its manufacturer, is not guaranteed or endorsed by the publisher.
The Supplementary Material for this article can be found online at: https://www.frontiersin.org/articles/10.3389/fnbeh.2022.869526/full#supplementary-material
Al Mushref, M., and Srinivasan, S. (2013). Effect of high fat-diet and obesity on gastrointestinal motility. Ann. Transl. Med. 1:14. doi: 10.3978/j.issn.2305-5839.2012.11.01
Benjamini, Y., and Hochberg, Y. (1995). Controlling the false discovery rate: a practical and powerful approach to multiple testing. J. R. Stat. Soc. Ser. B 57, 289–300.
Bolstad, B. M., Irizarry, R. A., Astrand, M., and Speed, T. P. (2003). A comparison of normalization methods for high density oligonucleotide array data based on bias and variance. Bioinformatics 19, 185–193.
Bonaz, B., Sinniger, V., and Pellissier, S. (2016). Vagal tone: effects on sensitivity, motility, and inflammation. Neurogastroenterol. Motil. 28, 455–462. doi: 10.1111/nmo.12817
Buckingham, J. C., and Hodges, J. R. (1977). Production of corticotrophin releasing hormone by the isolated hypothalamus of the rat. J. Physiol. 272, 469–479. doi: 10.1113/jphysiol.1977.sp012054
Bustin, S. A., Benes, V., Garson, J. A., Hellemans, J., Huggett, J., Kubista, M., et al. (2009). The MIQE guidelines: minimum information for publication of quantitative real- time pcr experiments. Clin. Chem. 55, 611–622. doi: 10.1373/clinchem.2008.112797
Cai, P. Y., Bodhit, A., Derequito, R., Ansari, S., Abukhalil, F., Thenkabail, S., et al. (2014). Vagus nerve stimulation in ischemic stroke: old wine in a new bottle. Front. Neurol. 5:107. doi: 10.3389/fneur.2014.00107
Choe, K. Y., Trudel, E., and Bourque, C. W. (2016). Effects of salt loading on the regulation of rat hypothalamic magnocellular neurosecretory cells by ionotropic GABA and glycine receptors. J. Neuroendocrinol. 28, 1–13. doi: 10.1111/jne.12372
D’Mello, C., and Swain, M. G. (2016). “Immune-to-brain communication pathways in inflammation-associated sickness and depression,” in Inflammation-Associated Depression: Evidence, Mechanisms and Implications. Current Topics in Behavioral Neurosciences, Vol. 31, eds R. Dantzer and L. Capuron (Cham: Springer), 76–88. doi: 10.1007/7854_2016_37
Dalli, J., Colas, R. A., Arnardottir, H., and Serhan, C. N. (2017). Vagal regulation of group 3 innate lymphoid cells and the immunoresolvent PCTR1 controls infection resolution. Immunity 46, 92–105. doi: 10.1016/j.immuni.2016.12.009
Dantzer, R. (2009). Cytokine, sickness behavior, and depression. Immunol. Allergy Clin. North Am. 29, 247–264. doi: 10.1016/j.iac.2009.02.002
Dantzer, R., and Kelley, K. W. (2007). Twenty years of research on cytokine-induced sickness behavior. Brain Behav. Immunity 21, 153–160. doi: 10.1016/j.bbi.2006.09.006
Dantzer, R., O’Connor, J. C., Freund, G. G., Johnson, R. W., and Kelley, K. W. (2008). From inflammation to sickness and depression: when the immune system subjugates the brain. Nat. Rev. Neurosci. 9, 46–56. doi: 10.1038/nrn2297
Dunn, A. J. (2006). Effects of cytokines and infections on brain neurochemistry. Clin. Neurosci. Res. 6, 52–68. doi: 10.1016/j.cnr.2006.04.002
Ek, M., Kurosawa, M., Lundeberg, T., and Ericsson, A. (1998). Activation of vagal afferents after intravenous injection of interleukin-1β: role of endogenous prostaglandins. J. Neurosci. 18, 9471–9479. doi: 10.1523/JNEUROSCI.18-22-09471.1998
Elson, A. E., and Simerly, R. B. (2015). Developmental specification of metabolic circuitry. Front. Neuroendocrinol. 39:38–51. doi: 10.1016/j.yfrne.2015.09.003
Fernstrom, J. D., and Fernstrom, M. H. (2007). Tyrosine, phenylalanine, and catecholamine synthesis and function in the brain. J. Nutr. 137, 1539–1547. doi: 10.1093/jn/137.6.1539S
Fleshner, M., Goehler, L. E., Hermann, J., Relton, J. K., Maier, S. F., and Watkins, L. R. (1995). Interleukin-1 beta induced corticosterone elevation and hypothalamic NE depletion is vagally mediated. Brain Res. Bull. 37, 605–610. doi: 10.1016/0361-9230(95)00051-f
Forsythe, P., Bienenstock, J., and Kunze, W. A. (2014). “Vagal pathways for microbiome-brain-gut axis communication,” in Microbial Endocrinology: The Microbiota-Gut-Brain Axis in Health and Disease, Vol. 817, eds J. F. Cryan and M. Lyte (New York, NY: Springer), 115–133. doi: 10.1007/978-1-4939-0897-4_5
Fu, X. Y., Li, Z., Zhang, N., Yu, H. T., Wang, S. R., and Liu, J. R. (2014). Effects of gastrointestinal motility on obesity. Nutr. Metab. 11:3. doi: 10.1186/1743-7075-11-3
Ghia, J. E., Blennerhassett, P., and Collins, S. M. (2007). Vagus nerve integrity and experimental colitis. Am. J. Physiol. Gastrointest. Liver Physiol. 293, 560–567. doi: 10.1152/ajpgi.00098.2007
Hahn, R. G., Stalberg, H. P., and Gustafsson, S. A. (1991). Vasopressin and cortisol levels in response to glycine infusion. Scand. J. Urol. Nephrol. 25, 121–123. doi: 10.3109/00365599109024545
Herman, J. P., Mueller, N. K., and Figueiredo, H. (2004). Role of GABA and glutamate circuitry in hypothalamo-pituitary-adrenocortical stress integration. Ann. N. Y. Acad. Sci. 1018, 35–45. doi: 10.1196/annals.1296.004
Hopkins, S. J. (2007). Central nervous system recognition of peripheral inflammation: a neural, hormonal collaboration. Acta Bio Med. Atenei Parm. 78, 231–247.
Hosoi, T., Okuma, Y., and Nomura, Y. (2000). Electrical stimulation of afferent vagus nerve induces IL-1β expression in the brain and activates HPA axis. Am. J. Physiol. Regul. Integr. Comp. Physiol. 279, 141–147.
Hussy, N., Brès, V., Rochette, M., Duvoid, A., Alonso, G., Dayanithi, G., et al. (2001). Osmoregulation of vasopressin secretion via activation of neurohypophysial nerve terminals glycine receptors by glial taurine. J. Neurosci. 21, 7110–7116. doi: 10.1523/JNEUROSCI.21-18-07110.2001
Kapalka, G. M. (2010). “Substances involved in neurotransmission,” in Nutritional and Herbal Therapies for Children and Adolescents, ed. G. M. Kapalka (Amsterdam: Elsevier Science), 71–99.
Karimi, K., Bienenstock, J., Wang, L. U., and Forsythe, P. (2010). The vagus nerve modulates CD4+ T cell activity. Brain Behav. Immunity 24, 316–323. doi: 10.1016/j.bbi.2009.10.016
Kawai, N., Sakai, N., Okuro, M., Karakawa, S., Tsuneyoshi, Y., Kawasaki, N., et al. (2015). The sleep-promoting and hypothermic effects of glycine are mediated by NMDA receptors in the suprachiasmatic nucleus. Neuropsychopharmacology 40, 1405–1416. doi: 10.1038/npp.2014.326
Klarer, M., Arnold, M., Günther, L., Winter, C., Langhans, W., and Meyer, U. (2014). Gut vagal afferents differentially modulate innate anxiety and learned fear. J. Neurosci. 34, 7067–7076.
Kobrzycka, A., Napora, P., Pearson, B. L., Pierzchała-Koziec, K., Szewczyk, R., and Wieczorek, M. (2019). Peripheral and central compensatory mechanisms for impaired vagus nerve function during peripheral immune activation. J. Neuroinflamm. 16:150. doi: 10.1186/s12974-019-1544-y
Komegae, E. N., Farmer, D., Brooks, V. L., McKinley, M. J., McAllen, R. M., and Martelli, D. (2018). Vagal afferent activation suppresses systemic inflammation via the splanchnic anti-inflammatory pathway. Brain Behav Immunity 73, 441–449. doi: 10.1016/j.bbi.2018.06.005
Kovács, K. J., Miklós, I. H., and Bali, B. (2004). GABAergic mechanisms constraining the activity of the hypothalamo-pituitary-adrenocortical axis. Ann. N. Y. Acad. Sci. 1018, 466–476. doi: 10.1196/annals.1296.057
Krowicki, Z. K., and Kapusta, D. R. (2011). Microinjection of glycine into the hypothalamic paraventricular nucleus produces diuresis, natriuresis, and inhibition of central sympathetic outflow. J. Pharmacol. Exp. Ther. 337, 247–255. doi: 10.1124/jpet.110.175398
Le Maître, E., Revathikumar, P., Idborg, H., Raouf, J., Korotkova, M., Jakobsson, P. J., et al. (2015). Impaired vagus-mediated immunosuppression in microsomal prostaglandin E synthase-1 deficient mice. Prostaglandins Other Lipid Mediators 121, 155–162. doi: 10.1016/j.prostaglandins.2015.05.006
Martelli, D., Farmer, D., McKinley, M. J., Yao, S. T., and McAllen, R. M. (2019). Anti-inflammatory reflex action of splanchnic sympathetic nerves is distributed across abdominal organs. Am. J. Physiol. Regul. Integr. Comp. Physiol. 316, R235–R242. doi: 10.1152/ajpregu.00298.2018
Martelli, D., Yao, S. T., McKinley, M. J., and McAllen, R. M. (2014). Reflex control of inflammation by sympathetic nerves, not the vagus. J. Physiol. 592, 1677–1686. doi: 10.1113/jphysiol.2013.268573
Martin, J. R., Rogers, R. C., Novin, D., and Vander Weele, D. A. (1977). Excessive gastric retention by vagotomized rats and rabbits given a solid diet. Bull. Psychon. Soc. 10, 291–294. doi: 10.3758/BF03329339
Marty, V., El Hachmane, M., and Amédée, T. (2008). Dual modulation of synaptic transmission in the nucleus tractus solitaries by prostaglandin E2 synthesized downstream of IL-1β. Eur. J. Neurosci. 27, 3132–3150. doi: 10.1111/j.1460-9568.2008.06296.x
Mazzocchi, G., Malendowicz, L. K., Rebuffat, P., Tortorella, C., and Nussdorfer, G. G. (1997). Arginine-vasopressin stimulates CRH and ACTH release by rat adrenal medulla, acting via the V1 receptor subtype and a protein kinase C-dependent pathway. Peptides 18, 191–195. doi: 10.1016/s0196-9781(96)00294-x
McAllen, R. M., McKinley, M. J., and Martelli, D. (2022). Reflex regulation of systemic inflammation by the autonomic nervous system. Auton. Neurosci. 237:102926. doi: 10.1016/j.autneu.2021.102926
Miller, S. L., and Yeh, H. H. (2017). “Neurotransmitters and Neurotransmission in the developing and adult nervous system,” in Conn’s Translational Neuroscience, ed. P. Michael Conn (Amsterdam: Elsevier Science), 49–84. doi: 10.1016/b978-0-12-802381-5.00004-x
Mitsui, T., Fukatsu, K., Yanagawa, M., Amenomori, S., Ogawa, E., Fukuda, T., et al. (2014). Truncal vagotomy temporarily decreases the pro- and anti-inflammatory cytokine levels in the small intestine. Surg. Today 44, 1123–1127. doi: 10.1007/s00595-013-0717-z
Mosteller, F., and Tukey, J. W. (1977). Data Analysis and Regression. Cambridge, MA: Addison-Wesley.
Myers, B., McKlveen, J. M., and Herman, J. P. (2012). Neural regulation of the stress response: the many faces of feedback. Cell. Mol. Neurobiol. 32, 683–694. doi: 10.1007/s10571-012-9801-y
Noori, H. R., Schöttler, J., Ercsey-Ravasz, M., Cosa-Linan, A., Varga, M., Toroczkai, Z., et al. (2017). A multiscale cerebral neurochemical connectome of the rat brain. PLoS Biol. 15:e2002612. doi: 10.1371/journal.pbio.2002612
Papadimitriou, A., and Priftis, K. N. (2009). Regulation of the hypothalamic-pituitary-adrenal axis. Neuroimmunomodulation 16, 265–271. doi: 10.1159/000216184
Patchev, V. K., Karalis, K., and Chrousos, G. P. (1994). Effects of excitatory amino acid transmitters on hypothalamic corticotropin-releasing hormone (CRH) and arginine-vasopressin (AVP) release in vitro: implications in pituitary-adrenal regulation. Brain Res. 633, 312–316. doi: 10.1016/0006-8993(94)91554-7
Pavlov, V. A., and Tracey, K. J. (2017). Neural regulation of immunity: molecular mechanisms and clinical translation. Nat. Neurosci. 20, 156–166. doi: 10.1038/nn.4477
Paxinos, G., and Watson, C. H. (1998). The Rat Brain In Stereotaxic Coordinates, 4th Edn. Cambridge, MA: Academic Press.
Pellissier, S., Dantzer, C., Mondillon, L., Trocme, C., Gauchez, A. S., and Ducros, V. (2014). Relationship between vagal tone, cortisol, TNF-alpha, epinephrine and negative affects in Crohn’s disease and irritable bowel syndrome. PLoS One 9:e105328. doi: 10.1371/journal.pone.0105328
Pereira, M. R., and Leite, P. E. C. (2016). The involvement of parasympathetic and sympathetic nerve in the inflammatory reflex. J. Cell. Physiol. 231, 1862–1869. doi: 10.1002/jcp.25307
Porges, S. W. (1995). Cardiac vagal tone: a physiological index of stress. Neurosci. Biobehav. Rev. 19, 225–233. doi: 10.1016/0149-7634(94)00066-a
Porges, S. W., Doussard-Roosevelt, J. A., and Maiti, A. K. (1994). Vagal tone and the physiological regulation of emotion. Monogr. Soc. Res. Child Dev. 59, 167–186. doi: 10.1111/j.1540-5834.1994.tb01283.x
Quan, N., and Banks, W. A. (2007). Brain-immune communication pathways. Brain Behav. Immunity 21, 727–735. doi: 10.1016/j.bbi.2007.05.005
Rinaman, L. (2007). Visceral sensory inputs to the endocrine hypothalamus. Front. Inneuroendocrinol. 28:50–60. doi: 10.1016/j.yfrne.2007.02.002
Rocca, B., and FitzGerald, G. A. (2002). Cyclooxygenases and prostaglandins: shaping up the immune response. Int. Immunopharmacol. 2, 603–630. doi: 10.1016/S1567-5769(01)00204-1
Rundgren, M., Hjelmqvist, H., Gunnarsson, U., and Hahn, R. G. (1993). Intracerebroventricular infusion of glycine stimulates vasopressin release in conscious sheep. Neuroreport 4, 1052–1054. doi: 10.1097/00001756-199308000-00015
Sekiyama, N., Mizuta, S., Hori, A., and Kobayashi, S. (1995). Prostaglandin E2 facilitates excitatory synaptic transmission in the nucleus tractus solitarii of rats. Neurosci. Lett. 188, 101–104. doi: 10.1016/0304-3940(95)11407-n
Sergeev, V. G., and Akmaev, I. G. (2000). Effects of vagotomy and bacterial lipopolysaccharide on food intake and expression of cyclooxygenase-2 mRNA in rat brain vessels. B. Exp. Biol. Med. 129, 553–555. doi: 10.1007/bf02434874
Stankiewicz, A. M., Goscik, J., Dyr, W., Juszczak, G. R., Ryglewicz, D., Swiergiel, A. H., et al. (2015). Novel candidate genes for alcoholism–transcriptomic analysis of prefrontal medial cortex, hippocampus and nucleus accumbens of Warsaw alcohol-preferring and non-preferring rats. Pharmacol. Biochem. Behav. 139(PtA), 27–38. doi: 10.1016/j.pbb.2015.10.003
TIBCO Software Inc (2017). Dedicated analytical and Reporting Systems. Available online at: https://www.statsoft.pl/
Tracey, K. J. (2007). Physiology and immunology of the cholinergic antiinflammatory pathway. J. Clin. Invest. 117, 289–296. doi: 10.1172/JCI30555
Varga, E., Farkas, E., Zséli, G., Kádár, A., Venczel, A., Kõvári, D., et al. (2019). Thyrotropin-releasing-hormone-synthesizing neurons of the hypothalamic paraventricular nucleus are inhibited by glycinergic inputs. Thyroid 29, 1858–1868. doi: 10.1089/thy.2019.0357
Watkins, L. R., and Maier, S. F. (2005). Immune regulation of central nervous system functions: from sickness responses to pathological pain. J. Intern. Med. 257, 139–155. doi: 10.1111/j.1365-2796.2004.01443.x
Weidenfeld, J., and Ovadia, H. (2017). “The role of the amygdala in regulating the hypothalamic-pituitary-adrenal axis,” in The Amygdala: Where Emotions Shape Perception, Learning and Memories, ed. B. Ferry (London: IntechOpen), 173–186. doi: 10.5772/67828
Wieczorek, M., and Dunn, A. J. (2006). Effect of subdiaphragmatic vagotomy on the noradrenergic and HPA axis activation induced by intraperitoneal interleukin-1 administration in rats. Brain Res. 1101, 73–84. doi: 10.1016/j.brainres.2006.04.120
Wieczorek, M., Świergiel, A. H., Pournajafi-Nazarloo, H., and Dunn, A. J. (2005). Physiological and behavioral responses to interleukin-1β and LPS in vagotomized mice. Physiol. Behav. 85, 500–511. doi: 10.1016/j.physbeh.2005.05.012
Keywords: hypothalamus, subdiaphragmatic vagotomy, amino acid neurotransmitters, inflammatory response, microarray, HPA axis
Citation: Kobrzycka AT, Stankiewicz AM, Goscik J, Gora M, Burzynska B, Iwanicka-Nowicka R, Pierzchala-Koziec K and Wieczorek M (2022) Hypothalamic Neurochemical Changes in Long-Term Recovered Bilateral Subdiaphragmatic Vagotomized Rats. Front. Behav. Neurosci. 16:869526. doi: 10.3389/fnbeh.2022.869526
Received: 04 February 2022; Accepted: 10 June 2022;
Published: 08 July 2022.
Edited by:
R. Alberto Travagli, The Pennsylvania State University, United StatesReviewed by:
Andrei V. Derbenev, Tulane University, United StatesCopyright © 2022 Kobrzycka, Stankiewicz, Goscik, Gora, Burzynska, Iwanicka-Nowicka, Pierzchala-Koziec and Wieczorek. This is an open-access article distributed under the terms of the Creative Commons Attribution License (CC BY). The use, distribution or reproduction in other forums is permitted, provided the original author(s) and the copyright owner(s) are credited and that the original publication in this journal is cited, in accordance with accepted academic practice. No use, distribution or reproduction is permitted which does not comply with these terms.
*Correspondence: Anna Teresa Kobrzycka, YW5uYS5rb2Jyenlja2FAYmlvbC51bmkubG9kei5wbA==; Marek Wieczorek, bWFyZWsud2llY3pvcmVrQGJpb2wudW5pLmxvZHoucGw=
Disclaimer: All claims expressed in this article are solely those of the authors and do not necessarily represent those of their affiliated organizations, or those of the publisher, the editors and the reviewers. Any product that may be evaluated in this article or claim that may be made by its manufacturer is not guaranteed or endorsed by the publisher.
Research integrity at Frontiers
Learn more about the work of our research integrity team to safeguard the quality of each article we publish.