- 1Department of Neurosurgery, Clinical Neuroscience Research Center, Tulane Brain Institute, Tulane University School of Medicine, New Orleans, LA, United States
- 2Department of Neurology, Tulane University School of Medicine, New Orleans, LA, United States
Major depression is a significant medical issue impacting millions of individuals worldwide. Identifying factors contributing to its manifestation has been a subject of intense investigation for decades and several targets have emerged including sex hormones and the immune system. Indeed, an extensive body of literature has demonstrated that sex hormones play a critical role in modulating brain function and impacting mental health, especially among female organisms. Emerging findings also indicate an inflammatory etiology of major depression, revealing new opportunities to supplement, or even supersede, currently available pharmacological interventions in some patient populations. Given the established sex differences in immunity and the profound impact of fluctuations of sex hormone levels on the immune system within the female, interrogating how the endocrine, nervous, and immune systems converge to impact women’s mental health is warranted. Here, we review the impacts of endogenous estrogens as well as exogenously administered estrogen-containing therapies on affect and immunity and discuss these observations in the context of distinct reproductive milestones across the female lifespan. A theoretical framework and important considerations for additional study in regards to mental health and major depression are provided.
Introduction
Mood disorders, including major depressive disorder (MDD), are a significant global health issue (Krishnan and Nestler, 2008). Worldwide lifetime prevalence of mood disorders has been reported to be nearly 10% (Steel et al., 2014), and in 2010, the nearly 300 million global cases of MDD accounted for 8.2% of all disease-induced years lived with disability (Ferrari et al., 2013a). In the US alone, one in six adults will receive an MDD diagnosis in their lifetime and more than 13 million Americans experience a major depressive episode with severe impairment each year (Kessler et al., 2005; Ferrari et al., 2013b; Brody et al., 2018). Annual costs associated with this condition are estimated at ∼$210 billion (Greenberg et al., 2015). MDD is generally considered a brain-targeted disease associated with persistent sadness, guilt, anhedonia (reduced interest in rewarding stimuli), despair, and in some cases, suicide (Krishnan and Nestler, 2008). Due to a happenstance discovery of psychiatric patients showing improved mood when treated with monoamine oxidase inhibitors (Schildkraut, 1965), MDD has historically been associated with deficiencies in serotonergic (5-HT), dopaminergic, and noradrenergic signaling within limbic, reward, and brainstem structures (Krishnan and Nestler, 2008). Problematically, available pharmacologic treatments targeting these presumed dysregulated monoamine systems are associated with delayed and inadequate symptom alleviation in a large proportion of patients (Trivedi et al., 2006; Al-Harbi, 2012; Akil et al., 2018). This led the field to conclude that the pathology of MDD is more complex than previously appreciated, that the neurotransmitters thought to underlie MDD-associated brain pathology may not be the sole contributors to its presentation, and that the therapeutic interventions targeting these systems will likely remain insufficient at imparting symptomatic relief.
Emerging data strongly implicate additional mechanisms in the manifestation of mood disorders. As a result, many researchers have begun considering biological factors that could significantly contribute to the development and persistence of MDD. One of these factors is that of genetic sex and the accompanying differences in sex hormone secretion across the lifespan. A substantial amount of research attention has been paid to the role of sex hormones, especially the steroid hormone estrogen, in driving development of MDD in women (Wharton et al., 2012; Eid et al., 2019). In addition to sex hormones, converging data amassed over the past few decades also support significant immune contributions to brain function and mood (Leonard, 2010; Dantzer, 2018). Indeed, it is now accepted that inflammatory cascades mediated by innate and adaptive arms of the immune system significantly contribute to MDD, at least in some patient subsets (Maes, 2011; Wohleb et al., 2016; Herkenham and Kigar, 2017). Sex differences in the susceptibility to certain infections, the presence of sex hormone receptors on immune cells, and shifts in the function of the immune system during distinct periods of the reproductive lifespan all point to a critical role of sex hormones in modulating immunity (Pennell et al., 2012; Klein and Flanagan, 2016).
Given the known sex differences in the prevalence of mood disorders, emerging support for the immune system’s role in mediating susceptibility or resilience to psychosocial stress, and the potentially profound impacts of sex hormones (especially estrogens) on impacting immunity, the consideration of neuro-immuno-endocrine interactions in the context of mood and MDD across the female lifespan, is warranted. Here, we will review evidence regarding the mood impacts of these factors individually, describe shifts in immune responses during key reproductive milestones, highlight a few examples of potential autoimmune consequences of estrogenic stimulation in females, and summarize the small but growing collection of findings exploring the convergence of sex, sex hormones and immune function in the context of mood and MDD. Finally, we present important experimental considerations when the convergence of these factors is investigated.
Manifestation of Disordered Mood Across the Female Lifespan: Role for Estrogens
Women shoulder a disproportionate burden of mood disorders and the role of estrogen in modulating mood has been well studied. Estrogens are generally thought to improve mood in many, but not all, circumstances. Below, we highlight major observations driving this conclusion. Though a thorough discussion of this extensive literature is beyond the scope of the current review, the reader is directed to several excellent reviews specifically addressing this topic (Wharton et al., 2012; Altemus et al., 2014; Eid et al., 2019; LeGates et al., 2019).
Sex Differences in Depression
Differences in the prevalence of MDD, phenotypic manifestations of depression, and the efficacy of antidepressant therapy between the sexes are well established (Altemus et al., 2014; LeGates et al., 2019). Rates of MDD are substantially higher among females compared to males (Weissman and Klerman, 1977), though this sex difference appears to be critically dependent on age. Prior to puberty, boys are more likely to have a mood disorder than girls (Faravelli et al., 2013). This incidence shifts during the pubertal transition as girls display depression at a rate double that of boys between the ages of 15 to 19 (Faravelli et al., 2013). MDD is nearly twice as prevalent in adult women than men, at rates of 10.4 and 5.5%, respectively (Brody et al., 2018). However, following reproductive senescence during the fifth decade of life, aging men and women tend to have similar prevalence rates of mood disorders (Faravelli et al., 2013).
Throughout life, men and women may also differ in their MDD endophenotypes. Results of several studies, including the large-scale Sequenced Treatment Alternatives to Relieve Depression (STAR*D) trial, indicate that women display higher rates of atypical and anxious depressive phenotypes. These are characterized by increased appetite, weight gain, comorbid eating disorder, rumination, hypersomnia, gastrointestinal complaints, and a higher rate of past suicide attempts relative to male patients (Marcus et al., 2008; Shors et al., 2017). Men are more likely to display comorbid substance use coping strategies and have higher rates of successful suicide, likely due to their use of more lethal means (e.g., firearms). Reports of irritability and the melancholic depressive subtype are similar in both men and women (Marcus et al., 2008).
Finally, though MDD treatments are available, barriers to treatment access as well as intervention type playing a role in the realization of symptom relief (LeGates et al., 2019) leaves the affective symptoms of many patients poorly controlled. Indeed, a recent study assessing nearly 250,000 depressed adults noted that only about 30% of MDD patients obtained pharmacological antidepressant treatment within three months of diagnosis (Waitzfelder et al., 2018), and of those, antidepressant efficacy is often delayed and highly variable (Trivedi et al., 2006; Al-Harbi, 2012; Akil et al., 2018). Sex may account for some of this variability as women appear to experience better symptom remission from selective serotonin (SSRI) or norepinephrine reuptake inhibitors, while men respond better when treated with tricyclic antidepressants (LeGates et al., 2019). Sex differences were not readily observed among adult patients with refractory bipolar/MDD undergoing repetitive transcranial magnetic stimulation (Huang et al., 2008). This observation appears to be age- and hormone status-dependent. Older women may display a poor response to rTMS (Huang et al., 2008) or the SSRI, venlafaxine (Thase et al., 2005), and this effect was reversed by estrogen supplementation. Sex differences in response to newly developed, fast-acting, glutamatergic-modulating, antidepressant interventions such as ketamine, are only beginning to be assessed. Emerging findings suggests conflicting results. Some groups have reported needing lower ketamine doses in female rats to impact affective behaviors under basal conditions while others report that male mice may be more responsive than females following exposure to stress (Saland et al., 2017; Okine et al., 2020). This is noteworthy given the recent Federal Drug Administration approval of nasally-administered esketamine for MDD patients treatment-resistant to traditional antidepressant interventions FDA (2019). It is also important to note that these sex-specific antidepressant treatment responses could also be explained, at least in part, by differences in the observed MDD endophenotypes in men versus women described above; further interrogation of this possibility is needed.
Hormone Effects on Depression and Mood During and After the Reproductive Years in Women
These observations along with the dynamic shifts in reproductive capacity that take place across the female lifespan implicate ovarian hormones in modulating mood and mood disorders in women. Indeed, between 10 and 80% of women experience mood disruptions that are related to their menstrual cycle, (Baker and Driver, 2007), and 3-8% of women can experience premenstrual dysphoric disorder, characterized by extreme premenstrual anxiety, decreased mood, and irritability (Robakis et al., 2019). These observations have been reported for the past several decades in both human and preclinical populations, though not all studies have consistently found an association between cycle stage and affect (Moos et al., 1969; Laessle et al., 1990; Jenkins et al., 2001; D’Souza and Sadananda, 2017; Sundström-Poromaa, 2018; Zhao et al., 2021). As well, the peripartum period is associated with dynamic shifts in sex hormone levels, and one of the most common complications of pregnancy, observed to impact one in seven mothers, are postpartum mood and anxiety disorders (Wenzel, 2016; Luca et al., 2019). Among menopausal women, (Maartens et al., 2002; Bekku et al., 2006; Gordon et al., 2016; Soares, 2017; Gracia and Freeman, 2018) and ovariectomized rodents (de Chaves et al., 2009; Li et al., 2014; Schoenrock et al., 2016), in whom levels of key sex hormones are substantially lower, increased anxiety and depressive behaviors have been noted.
Effects of Exogenous Estrogen Therapies on Mood
Estrogen-containing treatments have been shown to improve mood or attenuate depressive symptoms in humans (Schmidt et al., 2000; Soares et al., 2001; Poromaa and Segebladh, 2012; Maki et al., 2019) and to reverse at least some ovariectomy-induced pro-depressive changes in rodents (Bernardi et al., 1989; Galea et al., 2001; Walf and Frye, 2009; Schiller et al., 2013; Li et al., 2014; Hiroi et al., 2016), suggesting pro-resilience benefits. Estrogens, especially the most potent naturally circulating estrogen 17β-estradiol (E2), are known to induce dendritic spine plasticity and neuronal complexity, facilitate neurogenesis, regulate brain region volume and activity levels, and impact key neurotransmitter and growth factor systems implicated in depression, to name just a few examples (Galea et al., 2001; Maki and Resnick, 2001; Brinton, 2009; Walf and Frye, 2009; Wharton et al., 2012; Marrocco and McEwen, 2016; Engler-Chiurazzi et al., 2017). Yet, not all studies report beneficial impacts of exogenously administered estrogens on mood. Several studies have noted increased depression among women taking hormonal contraceptives (Duke et al., 2007; Skovlund et al., 2016; de Wit et al., 2020) though collective findings generally suggest that contraception exerts minimal effects on mood (Robakis et al., 2019). The realization of neurobiological and behavioral effects of estrogen-containing treatments depends on a number of factors including, but not limited to, age of the organism, etiology and duration of hormone depletion, type of estrogen, treatment route of administration, treatment regimen, and functional domain targeted (Engler-Chiurazzi et al., 2017). Consideration of these factors is of key importance when assessing mood-impacting effects of this hormone.
Evidence of Immune Impacts on the Development and Persistence of Depression
The immune system supports the body’s response against infection, injury, and disease. This complex network of intercommunicating, interactive cells and their secretory factors coordinates across multiple organs to mount a rapid and appropriate response to a threat to homeostasis through complex signaling cascades and activation/regulation sequences; the reader is directed to several excellent reviews that thoroughly describe the complexities of this system in detail (Chaplin, 2010; Marshall et al., 2018). Understanding of the complexity of neuroimmune mechanisms within the central nervous system (CNS) has grown rapidly in recent years. Although once considered “immune privileged”, a compelling body of literature indicates that the CNS and the peripheral immune systems engage in bidirectional communication, profoundly influencing one another during homeostasis and in pathological/diseased states (Lucas et al., 2006; Pavlov et al., 2018), including those associated with chronic stress and MDD (Dantzer, 2018). Microglial cells, the resident immune cells of the CNS, represent a particularly well-studied neuroimmune cascade mediator. Their actions as well as the contributions of other key CNS components (i.e., astrocytes, oligodendrocytes, perivascular macrophages, neurons, and endothelial cells) to the local neuroinflammatory cascade in response to CNS perturbation have been extensively described elsewhere (Ousman and Kubes, 2012; Ransohoff et al., 2015; Morimoto and Nakajima, 2019). Therefore, we will focus our discussion on the contributions of peripheral immune components to mood and MDD.
The peripheral innate immune response is characterized by rapid and non-specific activation of pattern/danger recognition receptors on innate immune cells to initiate phagocytosis of non-self antigens, secrete a variety of signaling factors including cytokines and chemokines, and/or function as antigen presenting cells to trigger adaptive immune activation (Chaplin, 2010; Marshall et al., 2018). Inflammation driven by innate immune system components, particularly macrophages, in modulating mood is now well established (Adzic et al., 2018). Chronic inflammation is implicated in a variety of mood disorders, leading to the emergence of the “macrophage/monokine theory of depression” (Dey and Hankey Giblin, 2018). For instance, depressive phenotypes have been consistently reported both among patients receiving proinflammatory cytokine treatment regimens and in preclinical models (Pryce and Fontana, 2017). As well, elevated levels of circulating cytokines, principally tumor necrosis factor (TNF)-α, interleukin (IL)-1β and IL-6, have been repeatedly reported among some subsets of depressed clinical populations (Dowlati et al., 2010; Köhler et al., 2017). Elevated levels of these inflammatory biomarkers are often associated with poor responsiveness to 5-HT targeting interventions (Arteaga-Henríquez et al., 2019), and anti-depressant treatment has been shown to reduce proinflammatory cytokine levels among treatment-responders or in preclinical models of immune challenge (Roumestan et al., 2007; Arteaga-Henríquez et al., 2019). Finally, compared to placebo, antidepressant treatment with co-administration of agents of anti-inflammatory action, such as non-steroidal anti-inflammatory drugs, statins, or cytokine inhibitors, improved depressive symptoms and MDD remission rates (Köhler-Forsberg et al., 2019). Mood benefits among depressed patients were even realized when these anti-inflammatory agents were administered as monotherapies (Köhler-Forsberg et al., 2019).
The complement system, an innate Immune arm that amplifies the recruitment signals initiated by other innate immune players, labels non-self antigens to facilitate immune-induced attack on these cells and mitigates the spread of the infection via membrane dysfunction-induced cell death (Rus et al., 2005), is also impacted by stress and depression. Indeed, levels of C3c and C4 complement as well as several other positive acute phase proteins including α1-antitrypsin and haptoglobin are elevated in depressed populations, while negative acute phase proteins like albumin are reduced (Kronfol and House, 1989; Maes et al., 1992b; Song et al., 1994).
The adaptive immune arm represents a delayed, antigen-specific response that targets intracellular infection/damage, amplifies and also resolves inflammatory cascade responses, and facilitates antigen memory (Chaplin, 2010; Marshall et al., 2018). Though evidence supporting a role for the peripheral adaptive immune system in modulating mood was slower to evolve due in part to the historical perception that lymphocytes are largely absent from brain parenchyma, T and B cells have also been implicated in response to CNS injury and disease, in the control of some normal brain functions and more recently, in MDD (Maes, 2011; Herkenham and Kigar, 2017; Dantzer, 2018). Indeed, many adaptive immune cells express the cellular machinery to respond to stimulation by the stress hormone, cortisol, and elevated cortisol levels, like those associated with a host of mood disorders, tend to be immunosuppressive (Gruver-Yates et al., 2014; Kovacs, 2014). Importantly, chronic stress is known to affect lymphocyte numbers/function in both humans suffering from mood disorders and in preclinical populations exposed to stressful conditions (Yin et al., 2000; Domínguez-Gerpe and Rey-Méndez, 2001; Frick et al., 2009; Scheinert et al., 2016). Lymphocytes are also profoundly impacted by 5-HT, at least in the periphery (Herr et al., 2017).
That peripherally derived T cells are now appreciated to be present in healthy brain parenchyma and can also infiltrate CNS tissue in response to injury or autoimmune disease has fostered major interest in the role of antigen-specific adaptive immunity in normal and abnormal brain function, including within the context of chronic stress and depression (Fletcher, 2010; Maes, 2011; Filiano et al., 2017; Herkenham and Kigar, 2017; Rayasam et al., 2018). Several seminal observations among depressed patient populations reported increased numbers of T helper/inducer cells and shifted ratios of CD4+/CD8+ T cells (Darko et al., 1988; Schleifer et al., 1989; Maes et al., 1990). Further, studies in lymphocyte-deficient mice (nude, scid or Rag–/– mice) have noted deficits in adaptability to stress and reconstitution with lymphocyte populations generally implicated the absence of T cells in mediating these deficits in a subset-specific way (Cohen et al., 2006; Beurel et al., 2013; Rattazzi et al., 2013; Brachman et al., 2015; Clark et al., 2016). For example, (primarily) T lymphocytes from stress-exposed mice can modify the behavioral response to stress when adoptively transferred into lymphocyte deficient subjects (Brachman et al., 2015). T cells also robustly respond to glutamatergic signaling (Ganor and Levite, 2012), a neurotransmitter system that is emerging as a key contributor to MDD and a principle target for novel, fast acting antidepressants (Wang Y. T. et al., 2021).
The B cell component of the adaptive immune system may also play an important role in modulating both normal CNS function as well as the response to stress. Historically there were inconsistencies with regards to whether B cells were changed in depressed populations. However, methodological advances in measurement of these populations has revealed blood B cell number alterations in the context of mood disorders, including chronic academic stress, MDD, bipolar disorder, and panic disorder (Darko et al., 1988; Maes et al., 1992b; Schleifer et al., 2002; Robertson et al., 2005; Pavón et al., 2006; McGregor et al., 2016; Ahmetspahic et al., 2018). Further, some studies have reported B cell responsiveness among MDD patients given monoamine-modulating antidepressant interventions (Hernandez et al., 2010; Ahmetspahic et al., 2018). These observations have been successfully recapitulated in a recently published preclinical study leveraging the chronic social defeat stress model (Lynall et al., 2021). Indeed, pioneering work from the Clathworhy group (Lynall et al., 2021) reported that chronic stress increased splenic B cell activation and increased meningeal monocytes, while meningeal B cell counts were reduced. From a mechanistic perspective, like T cells, B cells have been shown to express 5-HT receptors and the 5-HT transporter, indicating that these cells may even take up this key MDD-associated neurotransmitter and transport it to distant sites (Meredith et al., 2005; Herr et al., 2017). Whether the brain is one of these is yet to be determined. As well, growth factors, such as brain derived neurotrophic factor, have been implicated in the manifestation of MDD (Yang et al., 2020), and their stimulation is critical for B cell development (Schuhmann et al., 2005; Fauchais et al., 2008). Given the crucial role of B cells in antigen presentation to T cells, their ability to facilitate T cell activation, and emerging understanding of their immunoregulatory impacts, additional exploration of their role in the response to stress is warranted.
Key functional activities of B cells, such as antibody secretion, may also be altered by stress in an antibody subclass-specific way (Kronfol and House, 1989; Joyce et al., 1992; Song et al., 1994; Gold et al., 2012). For example, relative to mentally healthy control subjects, Gold and colleagues (Gold et al., 2012) noted that depressed populations displayed reductions in serum IgA, but not IgM or IgG levels, while Joyce et al. (Joyce et al., 1992) reported increased IgA. Methodological differences between sample populations and measurement approaches may account for some of the discrepancy between these studies. The critical role of hypothalamic-pituitary-adrenal axis dysregulation and altered cortisol secretion in the manifestation of MDD is well established (Krishnan and Nestler, 2008). Physiological states associated with high levels of circulating cortisol, such as hypercortisolism (Sarcevic et al., 2020) or treatment of patients with corticosteroid-based interventions, shifts serum antibody profiles relative to healthy controls (Griggs et al., 1972; Settipane et al., 1978). As well, neuronal surface autoantibody expression has been implicated in a number of neuropsychiatric conditions, MDD included (Zong et al., 2017).
Estrogenic Impacts on Immune Function During Distinct Reproductive Milestones Across the Female Lifespan
Sex differences in immunity are well documented, and hormone influences, including those of estrogens, have been shown to impact immune function throughout adulthood (Pennell et al., 2012; Klein and Flanagan, 2016). Immunological impacts of genetic sex and of estrogenic stimulation across key reproductive milestones are described in the following sections and have been summarized in Figure 1.
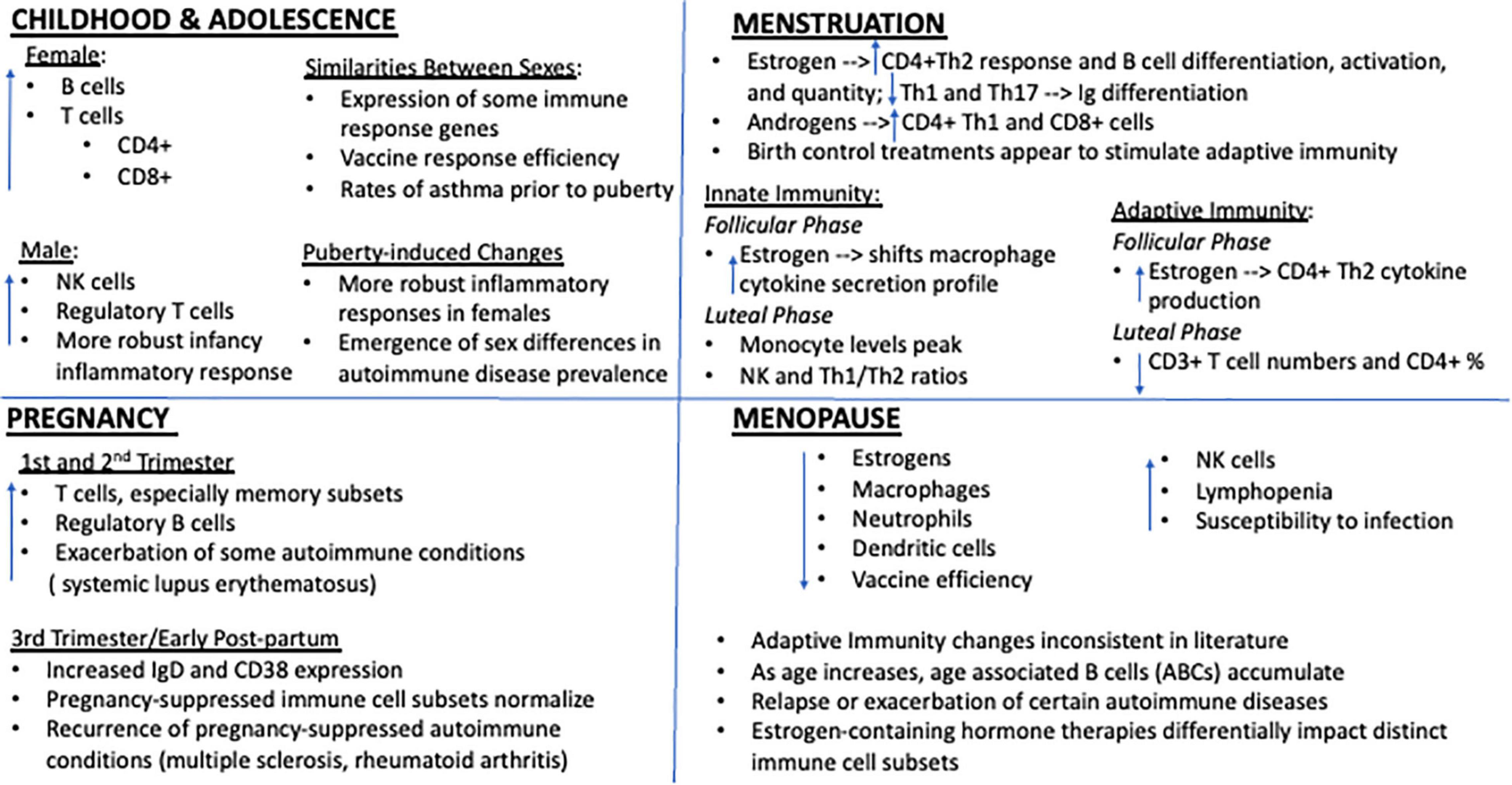
Figure 1. Key immune system impacts of estrogen at distinct female reproductive milestones. Immune function is profoundly impacted by genetic sex and variations in estrogen. Though a few subtle differences have been reported during childhood, prior to puberty onset immune cell counts are generally similar between males and females and any differences appear to have little functional impact on overall immunity. However, beginning with adolescence and the onset of menstruation, marked sex differences in immune cell ratios and response profiles emerge. Generally, females display a more robust inflammatory response to immune challenge, rendering them potentially more resilient to the negative consequences of infection but also more susceptible to certain autoimmune conditions. High concentrations of estrogen, whether they be due to natural shifts in circulating levels across the cycle or via administration of estrogen containing exogenous treatments, appear to exert cell-type specific effects with regards to key immune players, generally potentiating adaptive immunity. Falling estrogen levels with the transition to reproductive and immunosenescence also imparts profound consequences for immunity and is associated with dramatic shifts in peripheral immune cell profiles, autoimmune disease manifestation, and susceptibility to immune challenge.
Mechanisms of Estrogen Regulation of Immunity
Estrogenic signaling is regulated by two nuclear estrogen receptors (ER), ERα and ERβ, both of which are expressed on a variety of immune cell types and tissues. For instance, ERα is widely expressed in bone marrow thymocytes and hematopoietic cells, while ERβ expression appears to be limited to the thymus, lymphocytes in lymph nodes, and the spleen in mid-gestational fetuses (Khan and Ansar Ahmed, 2015; Moulton, 2018; Rubinow, 2018; Zhang et al., 2020). Estrogens regulate immune cell number and function likely via an ER-dependent mechanism. When human lymphocytes were administered 17β-E2, CD45 and CD45RO isoform RNA expression were increased, an effect that was blocked with co-treatment of ER antagonists (Zhang et al., 2020). Less potent naturally circulating estrogens also appear to exert similar regulatory effects on immune cells. For example, estriol, at levels similar to the first trimester of pregnancy (2 ng/mL), increased levels of venous blood CD4+FoxP3+ T regulatory cells and decreased levels of CD4+RORC+ Th17 lymphocytes were seen in women of reproductive age (Shirshev et al., 2019).
Sex Differences in Immunity During Early Life and Puberty
Some subtle sex differences in childhood immunity have been reported. For example, splenocyte response to cell surface-receptor-independent mitogenic combination of phorbol ester and ionomycin was greater in female mice at 3 weeks old, but was greater for 4-6 week old male mice (Rosen et al., 1999). Furthermore, a study on healthy Asian children noted that male babies showed 8% more natural killer (NK) cells at birth than females, while female newborns showed higher levels of CD3+ T cells (Lee et al., 1996). Between 1 and 6 years of age, girls had somewhat higher numbers of lymphocytes, B cells, and CD3+, CD4+, and CD8+ T cells, while boys had higher NK, activated T cells, and CD4+ T cell counts (Lee et al., 1996). In contrast, Lisse et al. found that West African boys show higher levels of CD8+ cells and lower CD4+/CD8+ ratios than girls (Lisse et al., 1997). Despite the discrepancy between these studies, prior to the onset of puberty, it is generally thought that the immune systems of male and female organisms exhibit few robust sex differences in immune cell counts or function (Robinson et al., 2014; Sharma et al., 2019). Indeed, splenic expression of some innate immune response genes was greater in pre-pubescent male mice, though the differences were not statistically significant and expression of adaptive immune response genes was generally similar between the sexes (Lamason et al., 2006). There also appear to be no sex differences in the vaccine response during childhood (Vom Steeg et al., 2019). This variability in the literature warrants future study to clarify the extent to which these observations replicate across study populations and translate to impact immunity overall during childhood.
The pubertal transition to reproductive capacity and the associated dramatic increases in sex hormone levels marks a period of substantial change in the immune system, changes that may exert functionally significant effects with regard to immune function in developing children. For instance, studies have noted increased numbers of circulating NK cells, CD4+ T cells, and B cells among girls, but higher CD8+ T cell numbers among adolescent boys as well as distinct response profiles of cultured peripheral blood mononuclear cells derived from male vs. female donors to phytohaemagglutinin stimulation (Lee et al., 1996; Uppal et al., 2003; Abdullah et al., 2012). There are numerous functional consequences of these puberty-induced sex differences in response to antigen challenge. Inflammatory responses to infection or toll-like receptor stimulation appear to be stronger in females than in males (Seillet et al., 2012; Robinson et al., 2014), with females showing increased gene expression of interferon-gamma, lymphotoxin beta granzyme A, IL-12 receptor beta2, and granulysin (Hewagama et al., 2009). Similar findings have been found preclinically where, in post-pubertal mice, following stimulation with ovalbumin and anti-CD3/CD28 antibodies, IL-4, IL-5, IL-13 were all significantly higher in female bronchial lymph node cells than in male cells (Okuyama et al., 2013). Additionally, IL-5 production from stimulated CD4+ T cells was significantly increased in females compared to males. Viral challenge with the mimetic polyinosinic:polycytidylic acid induced greater sickness behavior in post-pubertal males than females (Sharma et al., 2019). However, changes in body temperature and central c-fos expression were more prevalent in female mice, and gonadectomy both worsened sickness behavior and altered temperature in both sexes. Efficiency of vaccination has also been tested in murine models with adult female mice having greater antibody response to the vaccination and an increased number of antigen-specific hepatic CD8+ T cells compared to young mice (Vom Steeg et al., 2019). Another functional consequence relates to the prevalence of immune-associated diseases, especially asthma. Indeed, despite having similar numbers during childhood, adult females exhibit a 6.2% prevalence of asthma while males exhibit a 4.3% prevalence (Vink et al., 2010). Evidence supports that asthma responses and estrogen are largely correlated (Melgert et al., 2007) and that estrogen contributes to the innate macrophage polarization, thus leading to greater allergy response (Keselman et al., 2017).
Immune Variation Across the Ovulatory Cycle of Reproductively Capable Organisms
Innate immune cell number and function display a complex pattern throughout the menstrual cycle. For example, peripheral levels of NK cells along with their cytotoxic potential became heightened during the luteal phase, when estrogen levels begin to decline but progesterone levels tend to be high (Lee et al., 2010). Monocyte numbers also appear to peak during the luteal phase while circulating neutrophil levels decline during menstruation (Pennell et al., 2012). Overall, estrogen seems to enhance, while progesterone and androgens tend to suppress proinflammatory innate immune responses (Roberts et al., 2001; Musabak et al., 2003; Arruvito et al., 2008; Shepherd et al., 2020).
Adaptive immunity also displays dynamic changes across the menstrual cycle. Estrogens generally have stimulatory effects on lymphocyte presence, concentration, and function (Lee et al., 2010; Oertelt-Prigione, 2012; Pennell et al., 2012; Rodriguez-Garcia et al., 2013; Moulton, 2018) though cell type and tissue-specific effects of estrogen stimulation have also been suggested (Pung et al., 1985; Chen et al., 2015). For instance, increased levels of estrogen are thought to stimulate overall CD4+ Th2 cytokine production in females (Ackerman, 2006; Pennell et al., 2012; Shah, 2012). Peripheral regulatory T cell counts were shown to be higher during the follicular phase when estrogen levels are typically highest (Moulton, 2018). Wegienka et al. (2011) noted that blood levels of the less potent naturally circulating estrogen, estrone, were positively correlated with regulatory T cell counts in asthmatic women. Peripheral blood CD3+ and CD4+ T cell percentages decrease in the luteal phase, when estrogen levels are low relative to those of progesterone (Lee et al., 2010). Inhibitory effects of estrogens have also been noted within certain immune cell subtypes. Indeed, estrogen exposure inhibits Th1 cytokine proliferation and Th17 differentiation (Chen et al., 2015).
Though information regarding B cell changes across the menstrual cycle is more limited, converging evidence suggests that estrogen stimulates B cell differentiation and activation, increases B cell numbers, and enhances their function (Verthelyi, 2001; Oertelt-Prigione, 2012; Moulton, 2018). For example, B cell activation by 17β-E2 generally induces higher levels of Ig synthesis (Franklin and Kutteh, 1999; Pennell et al., 2012) specifically in B cells found in bone marrow and the spleen (Moulton, 2018). In mice treated with sustained slow-release 17β-E2-containing silastic implants (4-6 mg) resulting in levels comparable to those achieved during murine pregnancy, numbers of antibody-secreting plasma cell numbers increased dramatically, and secretion of various immunoglobulins and autoantibodies increased (Verthelyi and Ahmed, 1998). This estrogen-driven B cell hyperactivity may contribute to the development of autoimmune diseases (Pennell et al., 2012).
Sex hormone type also appears to influence female immunity in a cell subtype-specific way. Indeed, while it is widely accepted that estrogens usually correspond with an increased CD4+ Th2 cell response, androgens promote CD4+ Th1 and CD8+ cell responses (Ackerman, 2006; Pennell et al., 2012; Guven Yorgun and Ozakbas, 2019). As for progesterone, increased levels during the luteal phase sometimes correspond to increased NK cell levels, unchanged Th1/Th2 ratios, decreased CD3+ and CD4+ T cell percentages, and increased serum levels of the anti-inflammatory IL-1 receptor antagonist (Lee et al., 2010; Vetrano et al., 2020). Despite this, in one study, serum CD4+/IL10+ regulatory T cells displayed heightened responses when progesterone levels were elevated in the late follicular and luteal phases (Weinberg et al., 2011). These findings reveal the significance of distinguishing between the different immune cell subtypes in how they react to steroid hormone stimulation in distinct target tissues and in response to various immunological challenges.
Pregnancy-Associated Impacts to the Immune System
Tight regulation of the maternal immune response are key contributors to pregnancy success; historically, immune responses during pregnancy were thought to be suppressed to allow for a semi-allogeneic fetus (Racicot et al., 2014). However, this previously held notion has been reevaluated as additional findings implicating sex hormone regulation of immune responses have emerged in recent years (Mor and Cardenas, 2010). Indeed, immune contributions to the development of the decidua and placenta and the maintenance of the maternal-fetal interface is required for a successful pregnancy (Hsu and Nanan, 2014). Nair et al. (2017), and there is growing appreciation that dynamic shifts in maternal sex hormone levels may, at least in part, contribute to observed shifts in gestational immunity (Robinson and Klein, 2012). Uterine immune cells, including NK cells, macrophages, T cells, dendritic cells, mast cells, and B cells, are necessary for the normal formation of placenta beds and appear to play a key role in converting high-resistance, low-flow vessels to low-resistance, high-flowing vessels in spiral arteries in the placental bed (Faas and De Vos, 2018). Maternal monocytes and macrophages obtain a unique phenotype throughout pregnancy that allows them to retain immunological tolerance and permit hormone–immune cell interactions, both of which are required for progression of the fetus inside the uterus (Mendoza-Cabrera et al., 2020).
It is thought that the increase in steroid hormone levels throughout pregnancy modulates inflammatory responses at the maternal fetal interface, and E2, estriol, and progesterone influence the transcriptional signaling of those responses (Robinson and Klein, 2012). During the first trimester, levels of placental-derived estrogen increase sharply and contribute significantly to the development of organs and other bodily systems in the fetus. T cell subsets are profoundly affected by these changes. Early in pregnancy, the increase of regulatory T cells supports the development of a semi-allogeneic fetus protected from maternal immune rejection by restraining inflammation during the shift from proinflammatory to anti-inflammatory immunity (Krop et al., 2020). CD25+/CD4+ T regulatory cell numbers reach a peak during the second trimester, and it is thought that these cells allow the maternal immune system to respond to the developing fetal organs within the uterus (Somerset et al., 2004; Lima et al., 2017). Further, appropriately titrated T cell populations early in pregnancy may contribute to fetal viability. Indeed, when Lissauer and colleagues (Lissauer et al., 2014) evaluated circulating T cell subsets across distinct pregnancy stages, they observed that about 60% of Th17 cells in the body during pregnancy were found during the first trimester of pregnancy, though no changes in Th1 or Th2 T cell subsets were noted across the gestational and postpartum period. Th1 and Th17 cells numbers were elevated among women with recurrent miscarriage, suggesting that these cell types may serve as important targets to improve gestational success (Lissauer et al., 2014). Memory T cells are also increased during the first trimester and promote fetal-maternal tolerance (Kieffer et al., 2019). It is thought that insufficient numbers of memory CD4+ T cells contribute to pregnancy complications such as preeclampsia, gestational diabetes, and premature labor (Lim et al., 2019). Following pregnancy, it can take three to four months for cells to return to normal function after delivery, and inhibition of helper T cells and NK cells appears to last for the first few months.
Like T cells, B cells also support the semi-allogeneic fetus while protecting the mother and fetus against infection (Muzzio et al., 2013). Regulatory B cell numbers similarly increase during the first trimester, limiting proinflammatory responses (Esteve-Solé et al., 2018). B cell activation factors, which facilitate the inflammatory response, are also increased, likely in support immune tolerance of the semi-allogeneic fetus (Wang L. et al., 2021). B cells also appear to impact immunity during later stages of pregnancy and following parturition. Lima and colleagues examined healthy pregnancies and determined the degree of activation of different B cell subsets, reporting increases in CD38+ and IgD markers of B cell activation during the third trimester of pregnancy and postpartum period (Lima et al., 2016).
Immune Shifts During Female Reproductive Senescence and Aging
Aging plays a significant role in modulating the function of the immune system and is associated with deterioration of immunity seen in the elderly (Fulop et al., 2017). Immunosenescence cascades have been reviewed elsewhere (Xu et al., 2020) but in brief, key immunosenescence characteristics include inflammaging, lymphopenia, higher susceptibility to infection and poor vaccine response (Ghosh et al., 2014). Within the adaptive immune system, aging is associated with an increase in differentiated memory T cells, effector T cells, senescent CD8+CD28– T cells, and age-associated innate-like B cells, but a decrease in most B cell subsets and the ratio of CD4:CD8 T cells, to name just a few examples (Weyand and Goronzy, 2016). Collectively, these and other senescence-related changes diminish the ability of the immune system to protect against certain infections and cancers and may accelerate the development of certain diseases, rendering older populations at-risk for a host of immunological challenges. It was historically presumed that this senescence-associated shift in immunity occurred at a similar rate and manner, regardless of sex (Aiello et al., 2019). However, rising life expectancies have revealed that men and women experience these consequences along different trajectories; emerging evidence suggests sex-specific and potentially profound consequences of immunosenescence (Gubbels Bupp et al., 2018; Márquez et al., 2020). Indeed, it is now appreciated that female innate immune systems appear to age at a faster rate, whereas the adaptive immune systems of men age at a faster rate (Ghosh et al., 2014).
Age-related shifts in reproductive function likely influence the function of the immune system during aging, and the sex-specific nature of this transition period may account for differences in male and female immunosenescence. Indeed, while men experience andropause, a gradual reduction in circulating testosterone over the course of several decades (Kevorkian, 2007), women experience a more accelerated transition. Menopause marks a period of reproductive senescence in a woman’s life when the ovarian oocytes have become depleted, and sex hormones are no longer produced by the ovaries (Keppel and Wickens, 2004). As a result, the menstrual cycle becomes irregular and eventually terminates while levels of estrogens and progestins drastically decline. Natural menopause is a normal part of aging that typically occurs in the fourth and fifth decade of life and can take place over the course of only a few years. Still others undergo surgical menopause to remove the ovaries when there is an increased likelihood of cancer, infection or endometriosis (“Medical Causes of Menopause”), resulting in an accelerated reproductive senescence.
The distinct trajectory of female reproductive senescence has important impacts with regard to immune function during aging. In comparison to men of a similar age or to reproductively capable women, post-menopausal women are disproportionately affected by certain autoimmune disorders and have an increased susceptibility to infection with aging (Fairweather et al., 2008; Gubbels Bupp et al., 2018; Maglione et al., 2019). Estrogen deficiency has been implicated in many senescence-associated changes seen in the immune cells, such as the increase in proinflammatory markers IL-1, IL-6 and TNF-α (Gameiro et al., 2010), and low levels of estrogen are linked to higher levels of IL-17 produced by Th17 cells (Molnár et al., 2014). Following menopause, women undergo various changes in the levels of innate immune cells. Whereas the number of NK cells increases, their cytotoxic capacity is diminished (Albrecht et al., 1996; Ghosh et al., 2014; Toniolo et al., 2015). Further, the number of macrophages, neutrophils and dendritic cells decreases (Ghosh et al., 2014; Toniolo et al., 2015). Macrophages are vital, as they aid in the conversion of proinflammatory phenotypes to anti-inflammatory phenotypes, and estrogens help to prevent the effects of proinflammatory agents on the functions of macrophages by accelerating the resolution phase of inflammation in these cells (Toniolo et al., 2015; Villa et al., 2015). E2 also seems to decrease the rate of apoptosis in neutrophils as following menopause, neutrophils numbers have been shown to decrease as the rate of apoptosis increases (Chen et al., 2016).
Menopause is also associated with significant shifts in adaptive immunity. As reviewed in Gubbels Bupp et al. (2018), some studies report a decrease in total lymphocyte counts in postmenopausal women (Giglio et al., 1994; Kamada et al., 2000), while other studies have shown that numbers of some lymphocyte subsets are significantly higher in postmenopausal women (Chen et al., 2016; Abildgaard et al., 2020). Further, levels of functioning CD4+ T and B cells decrease, while numbers of exhausted and senescent cells rise, whether the etiology of menopause is surgical or transitional (Giglio et al., 1994; Gameiro et al., 2010; Gubbels Bupp et al., 2018; Maglione et al., 2019; Abildgaard et al., 2020; Vrachnis et al., 2021). However, it has also been shown that thirty days after surgical menopause via total abdominal hysterectomy and bilateral salpingo-oopherectomy, patients displayed increased levels of CD8+, but decreased levels of B cells and a reduced CD4+/CD8+ T cell ratio (Kumru et al., 2004). Other conflicting literature has noted a decrease in naïve CD8+ T cells, but an increase in memory or activated T cells in postmenopausal women compared to pre-menopausal women or women taking hormone replacement therapy (HRT) (Kamada et al., 2000; Engelmann et al., 2016; Vrachnis et al., 2021). In regards to the function of B cells, E2 enhances certain aspects of humoral immunity (Gameiro et al., 2010). Aged women tend to accumulate more innate-like age-associated B cells (ABCs) than young women and men of any age, and there is a relationship between ABCs, viral infections, autoimmunity and a proinflammatory state (Rubtsova et al., 2015; Gubbels Bupp et al., 2018). ABCs are known to originate from follicular B cells and show a bias in females, due to hormones and X chromosome-encoded genes, but the mechanisms that cause the production and accumulation of ABCs are still unknown and need to be further investigated (Rubtsova et al., 2015).
Effects of Exogenous Estrogen-Containing Treatments on Immune Function
Immune cells not only respond to endogenously secreted estrogens; estrogen-containing contraceptives, commonly used for pregnancy prevention, hormonal imbalances, and menstrual cycle regulation, also impact immunity. For example, compared to untreated women, women taking the oral contraceptive pill, Ortho Novum 777 (containing ethinyl estradiol and norethindrone), had higher Ig levels, implicating these hormones in promoting B cell activity (Franklin and Kutteh, 1999). In another small study evaluating respiratory performances of thirteen asthmatic women, blood regulatory T cell counts were higher among contraceptive treated women, and this was associated with less intense asthmatic symptoms (Wegienka et al., 2011; Vélez-Ortega et al., 2013). Estrogen-containing contraceptives administered vaginally also impact the local immune environment. Indeed, Hughes et al. noted that the NuvaRing® (0.12 mg etonogestrel/0.015 mg E2 per day) was associated with increased T cell- related proteins, granulysin and granzyme B in cervicovaginal fluid, indicating that, similar to during phases of heightened estrogen in the menstrual cycle, estrogen has a stimulatory effect on vaginal T cell response when locally administered. Yet in mice, when the synthetic estrogen, diethylstilbestrol, was administered subcutaneously for five consecutive days, T cell proliferation and IL-2 production in the spleen both declined (Pung et al., 1985), implicating species or estrogen subtype differences with regards to exogenous estrogen impacts to immunity.
Though menopausal HRT is commonly prescribed to attenuate the negative vasomotor and vaginal symptoms of menopause, it may also be a potential therapeutic option to modify menopause-related shifts in immune system function (Ghosh et al., 2014); the complexities associated with HRT impacts to the brain and immunity have been extensively reviewed elsewhere (Abdi et al., 2016). As an example, postmenopausal women taking estrogen and progestin-containing HRT have been reported to have higher numbers of lymphocytes and B cells specifically, but maintain low levels of CD4+ T cells, and exhibit a decrease in CD8+ cells resulting in an increase in the ratio of CD4+/CD8+ T cells; naïve and memory/activated T cell numbers generally remained consistent (Kamada et al., 2000; Yang et al., 2000; Porter et al., 2001; Kumru et al., 2004). These HRT-induced immune cell impacts may be effective in alleviating the symptoms associated with menopause or autoimmune disease, as well as the risk for developing certain disorders, especially when used within 10 years of experiencing symptoms if the woman is under 60 years old (Stopińska-Głuszak et al., 2006; Cagnacci and Venier, 2019). Taken together, these data indicate that exogenous estrogen treatment has significant immunological consequences, which may in turn impact women’s susceptibility to systemic infection or autoimmune disease (see below); additional investigation in this research domain is clearly warranted.
Sex, Estrogen and Autoimmunity – Consequences of Estrogenic Impacts to the Female Immune System
Though the evidence described above reveals robust sex-specific differences in immune responses, may at times, provide some advantages to infection for female organisms, maladaptive consequences have also been indicated. Indeed, women shoulder a disproportionate burden of some autoimmune diseases and several reviews extensively explore the topic of sex differences in the prevalence of autoimmunity (Lateef and Petri, 2012; Pennell et al., 2012; Ortona et al., 2016; Moulton, 2018; Keestra et al., 2021). For instance, with a typical age of disease onset occurring during puberty, the prevalence of systemic lupus erythematosus (SLE), an autoimmune condition associated with widespread inflammation, in prepubertal girls is only double that of boys; by adulthood, the ratio of female to male patients has increased to 9:1 (Ngo et al., 2014; Moulton, 2018). Further, SLE-associated flare-ups during pregnancy are common (Petri, 2020). That estrogen stimulation promotes a Th2 immune phenotype may further contribute to the increased prevalence of Th2-mediated autoimmune diseases such as SLE (Ackerman, 2006). For instance, regulatory CD4+ T cells from female SLE patients showed reduced FoxP3 expression when incubated with physiological levels of E2, suggesting that high E2 levels may place women at an increased risk due to the presence of fewer immune regulatory cells (Singh and Bischoff, 2021). Among SLE patients, HRT has been found to increase the amount of mild, but not severe, flares (Lateef and Petri, 2012).
A sex-specific burden of multiple sclerosis (MS), a chronic, progressive, demyelinating inflammatory autoimmune disease associated with a myriad of degenerative sensori/locomotor and cognitive deficits, has also been documented (Goldenberg, 2012). Indeed, a woman’s risk for developing MS increases after the pubertal transition, an effect linked to increasing levels of estrogens given that MS symptomology appears to decrease in intensity during the luteal phase of the menstrual cycle, when estrogen levels are low (Moulton, 2018; Keestra et al., 2021). Additional clarity regarding the contributions of sex hormones alone and in combination is warranted as perplexingly, some studies note greater MS symptomology and worsened cognitive function in the premenstrual phase when sex hormones are generally at their lowest levels (Guven Yorgun and Ozakbas, 2019; Keestra et al., 2021). As well, though relapse rates increase significantly by three months post-partum, pregnancy is typically associated with symptom remission (Confavreux et al., 1998). Short-term corticosteroid treatment to manage MS symptoms during late pregnancy is considered safe with regards to fetal outcomes such as risk of pre-term birth and low birth weight (Ramo-Tello et al., 2021). Whether this treatment impacts affective outcomes in the pregnant or post-partum mother is not clear and represents an important area of investigation, given that corticosteroid treatments are known to induce psychiatric symptoms such as mania, depression, psychosis, and cognitive changes (Brown and Chandler, 2001).
Rheumatoid arthritis (RA) is an autoimmune disease characterized by joint pain, painful swelling, fatigue and fever due to the immune system attacking its own healthy tissue (Bullock et al., 2018). RA is both more common and may be more severe in women than men (Walker, 2011; Pennell et al., 2012). Like MS patients, women with RA experience symptom remission during pregnancy but these effects are short-lived as women often experience disease aggravation following parturition (Ostensen et al., 1983). The typical age of RA onset in women is during the menopausal transition, and an early age at menopause is associated with an increased likelihood of RA (Goemaere et al., 1990; Desai and Brinton, 2019). This observation may be attributed to the loss of endogenous estrogen that women experience during menopause. Menopausal RA patients taking HRT do not appear to display increased flare-ups and may even experience improved disease symptomology (Holroyd and Edwards, 2009). Similar effects have been demonstrated among pre-menopausal women, where oral contraceptive use did not prevent emergence of new disease but did reduce transformation of cases from mild to severe, suggesting beneficial effects of exogenously-administered estrogen-containing therapies against disease progression.
Convergence of Sex, Estrogen and Immunity in Stress and Depression
The complexities of how biological sex or sex hormones and peripheral immunity converge to impact mood are beginning to be revealed. Sex-specific affective responses to peripheral inflammatory challenge generally suggest that female organisms may respond more robustly to immune activation (Bekhbat and Neigh, 2018). Indeed, inflammatory challenge with lipopolysaccharide (LPS; bacterial infection mimic) was associated with mood disruptions in women but not men (Moieni et al., 2015) and intranasal LPS administration induced depressive-like behavior and elevated hippocampal proinflammatory cytokine expression only in female rodents (Tonelli et al., 2008). However, this effect has not consistently been observed. For instance, following LPS challenge, while women displayed greater increases in proinflammatory IL-6 and TNF-α levels than men and men displayed higher levels of the typically anti-inflammatory cytokine IL-10, surprisingly affective consequences were similar among both sex groups (Engler et al., 2016). Similar observations were noted in preclinical studies where male and female rodents displayed similar depressive-like behavioral phenotypes despite robust sex-distinct effects on inflammatory and growth factor cascades in response to peripheral immune stimulation (Adzic et al., 2015; Brkic et al., 2017). Still, other reports suggest that males may be more susceptible to affective impacts of peripheral immune activation. Indeed, in male mice exposed to a LPS challenge, depressive-like behavioral changes along with altered brain proinflammatory cytokine mRNA levels were observed at 24 h, and hippocampal apoptosis was shown at 28 days later, effects not observed in female mice (Millett et al., 2019; Rossetti et al., 2019).
Sex differences in peripheral circulating cytokine levels among clinically depressed populations or in preclinical models have also been reported. For instance, higher levels of C-reactive protein were associated with an increased risk of depressive transformation, and increased psychopathology among depressed women was associated with elevated levels of C-reactive protein where no such association was noted in depressed men (Köhler-Forsberg et al., 2017; Kim et al., 2021; Zainal and Newman, 2021). Genetic predispositions related to the immune system also appear to induce sex-specific risk factors for development of a depressive phenotype as IL-18 haplotype in women, but not men, is associated with increased threat-induced central amygdala reactivity (Swartz et al., 2017). Other cytokines that are associated with depressive phenotypes in females, or the responsiveness of depressed patients to antidepressant treatment, include IL-1β, and IL-6 (Carboni et al., 2019; Kim et al., 2021; Zainal and Newman, 2021). However, some inconsistencies regarding sex-specific differences in peripheral inflammation among depressed populations have been reported. For example, while Piantella and colleagues (Piantella et al., 2021) agreed with other literature that IL-6 was associated with higher depressive symptoms in women exposed to workplace stress, they observed that higher C-reactive protein levels were associated with depression only in men. Further, in a study of more than 1,800 patient samples, C-reactive protein was associated with MDD state only in men (Ramsey et al., 2016). The experimental heterogeneity associated with the study population and sample size, the stressor nature and severity being evaluated, the approach to measure cytokine levels, the post-stress measurement timeframe, etc., among evaluations reported in the literature indicate that additional work is needed to discern the utility of sex-specific cytokine biomarkers for depression.
Taken together, it appears that immune activation cascades in response to psychosocial stress differ between males and females, though whether the consequences of these distinct trajectories reliably manifest in differential mood-related disruptions between the sexes is not altogether clear. Further clarification of the parameters in which sex-specific mood impacts may be realized in the context of antigen-driven or sterile immune challenges is needed.
Discussion: Challenges in Exploring Neuro-Immuno-Endocrine Interactions in the Context of Mood
As summarized above, genetic sex, estrogen, and the immune system significantly contribute to mood and mood disorders both individually and as converging, interactive factors (Figure 2). As this exciting field further develops, consideration of a number of limitations and challenges to probing these complex interactions in the context of mental health is warranted.
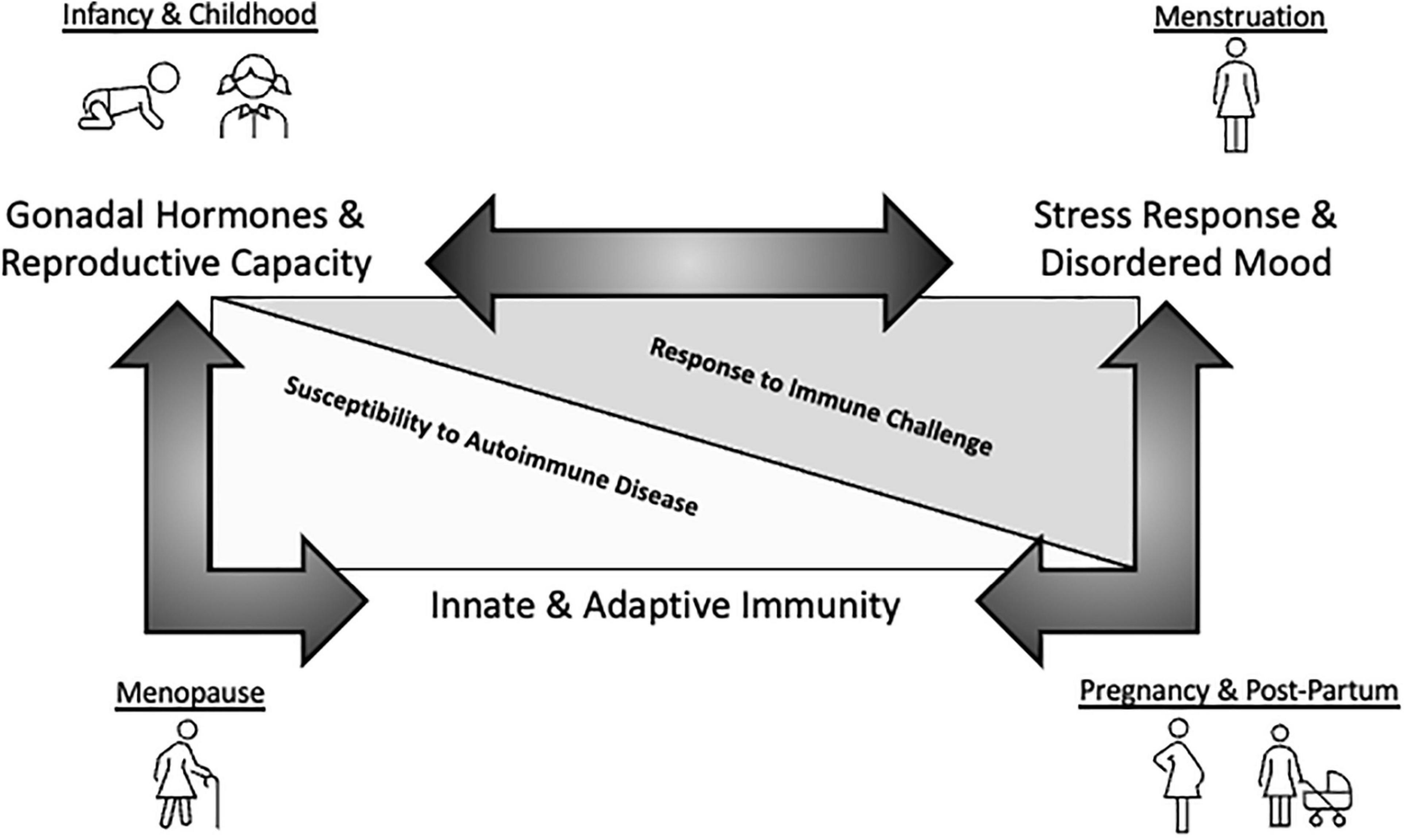
Figure 2. Schematic representation of mood-immune convergence across the female reproductive lifespan. A substantial amount of research has been dedicated to exploring how endocrine and immune factors impact mood separately. For instance, neuroprotective effects of estrogen in regards to MDD are well-established. Further, inflammatory insult and immune dysfunction are emerging as key contributors to disordered mood. Finally, genetic sex and estrogen clearly modulate immune system components, having important functional consequences for immunity across the reproductive lifespan. However, insight regarding how these two systems converge to impact mental health, especially during aging, is currently limited. This knowledge gap may be driven by experimental challenges associated with exploring these complicated interactions including, but not limited to, heterogeneity associated with the study population and sample size, the species used, the stressor nature and severity being evaluated, the approach to measure cytokine levels, the post-stress measurement timeframe, to name a few examples. Whether estrogenic influences on inflammatory activation cascades in the context of ‘sterile’ psychosocial stress-induced immune challenges result in sex-specific susceptibility to MDD during key reproductive milestones remains to be further interrogated and represents an exciting area of study.
Consideration of Relevant Biological Variables
First, though historical representation of both sexes in biomedical research has been lacking, there is increasing awareness among researchers regarding the need to consider sex as a biological variable and moreover consider how biological phenomena change as reproductive capacity shifts across the lifespan (Arnegard et al., 2020). Indeed, in 2015, the NIH (2015). announced requirements for the appropriate consideration of sex as a biological variable, incorporating this as a review criteria for all proposals submitted shortly thereafter. This policy change included requirements for the use of both sexes within study populations unless strong justification is provided as to why research questions being assessed could only be evaluated in one sex (e.g., exploration of ovarian function would preclude the use of only female organisms) as well as disaggregation of data analyses to observe sex-related trends and accurate reporting of data based on sex As part of NIH’s larger initiative to improve experimental rigor and reproducibility (Price and Duman, 2020), due consideration of other relevant biological variables is now also strongly advised (Lauer, 2016).
At present, the majority of research addressing the convergence of immune cells and sex or sex hormones on mood outcomes does not regularly factor in cyclicity stage, parturition experience, nor circulating levels of steroid hormones. As well, even when females are included in experimental designs, the majority of work in this area is conducted in young adult subjects prior to initiation of age-related immunosenescence cascades, potentially limiting translatability of the findings to older cohorts. While due consideration of key biological variables is not without its methodological challenges, there exists numerous aging or sex-based research centers of excellence around the United States (e.g., Nathan Shock Centers of Excellence in the Basic Biology of Aging, Tulane Center for Excellence In Sex-Based Biology and Medicine, and several workshops (e.g., International Symposium on the Neurobiology and Neuroendocrinology of Aging) providing training in the conduct of aging and/or sex-based research have been developed in recent years. In addition to informal laboratory based training, several publications laying out strategies are readily available (Bale and Epperson, 2017; Joel and McCarthy, 2017; Clayton, 2018). Especially given the profound age-related shifts in immune function, there exist numerous opportunities for productive research collaborations between immunologists, neuroendocrinologists, and biostatisticians to thoroughly address the convergence of sex, sex hormones, age, immune function, and stress responses. Continued progress is still needed (Woitowich and Woodruff, 2019; Arnegard et al., 2020), and additional incentivization of research specifically aimed at systematically addressing sex differences and the influence of sex hormones within the scope of mental health research will likely benefit the field.
Complexities of Evaluating Mood and Modeling Human Mental Health Disorders Preclinically
Another significant challenge facing this area of study is that effectively modeling complex mood disorders such as MDD in a rodent is difficult (Krishnan and Nestler, 2008; Nestler and Hyman, 2010; Wang et al., 2017). Whereas the etiology of MDD can be varied in humans, ‘depressive-like’ states in rodents are typically experimentally induced via environmental, experiential, genetic, pharmacological, physical, social, or surgical manipulations. Many of the classic induction approaches, developed at the height of the monoamine hypothesis of depression, were aimed at revealing antidepressant efficacy novel drugs (Nestler and Hyman, 2010; Wang et al., 2017). Unfortunately, no single stress-induction approach fully recapitulates the heterogeneity of disease susceptibility and no one readout fully captures the behavioral and neurobiological pathology seen in human populations, though some newer paradigms have been developed that display better translational validity. For instance, the long-leveraged forced swim stressor results in near ubiquitous floating behavior, thought to be an indicator of behavioral despair/learned helplessness, while the chronic social defeat paradigm can effectively discriminate susceptible from resilient populations (Golden et al., 2011; Bogdanova et al., 2013).
As well, MDD is a psychiatric disorder associated with a variety of phenotypes, and many symptoms experienced by human patients (i.e., sadness, guilt, suicide ideation) cannot be directly evaluated in rodents. When MDD symptomology can be more effectively recapitulated preclinically (anhedonia, behavioral despair), the available murine tests of depressive-like behavior generally only probe one dimension of this heterogeneity. Evaluating depression phenotypes through the assessment of other impacted functions, such as cognitive domains, may provide additional insights (Hales et al., 2014; Price and Duman, 2020). Further, in contrast with the delayed response of antidepressants prescribed to patients in the clinic, acute treatment of mice with antidepressants is sufficient to alleviate depressive-like behavior in some of the commonly employed preclinical tests, though again some paradigms show response timing profiles similar to those observed in clinical populations (Golden et al., 2011; Willner, 2017).
Finally, methods used to induce stress phenotypes in rodents may confound readouts. For instance, a common behavioral readout of the chronic variable stress paradigm is sucrose preference, a measure of anhedonia, the results of which may be profoundly impacted by metabolic changes associated with brief food restriction, a commonly leveraged component of that stress-induction approach (Willner, 2017). As such, no single preclinical stressor paradigm or test for ‘depression’ fully recapitulates the complexity of the MDD phenotype nor the response profile to typically prescribed treatments given to alleviate symptomology.
Selection of the method to induce stress as well as the approach to determine behavioral, physiological, and neurobiological responses require careful consideration of the research question being posed. In alignment with recent guidance from the NIMH (2019) and in pursuit of current Research Domain Criteria (RDoC) recommendations (Maes, 2011), it is advisable to leverage tests where the underlying neurobiological circuitry is well understood rather than on the basis of “presumed congruence to human symptoms of mental illness”. Homological validity, that is capturing behavioral readouts that are species-relevant, should also be prioritized. The use of a behavioral battery of readouts within affective domains rather than a single assessment is also highly recommended to accurately capture the breadth and depth of a phenomenon, though test order should be an important consideration in their deployment (Powell et al., 2012). Composite behavioral battery z-scores should also be leveraged to capture overall impacts of stress on an organism as there is often substantial individual performance variability on unique readouts, especially among controls (Johnson et al., 2021). Behavioral readouts are best coupled with physiological readouts of stress, such as circulating corticosterone levels, metabolic alterations (such as attenuated weight gain), reduced self-care and health metrics, and shifts in circadian activity. There are also logistical complexities in preclinically modeling depression specifically in female organisms, including whether to consider stage of estrous cycle and reproductive capacity. Rigorous research portends the quantification of vaginal lavage to determine cyclicity and inclusion of Cycle Stage as an additional factor in statistical assessment of stress responses. Importantly, Johnson et al. (2021) did not identify a predicitive relationship between cycle stage and the stress response of their experimental and control animals. Further, though ‘non-brain’ measures showed more female-associated variability, a recent metanalysis of 311 articles did not report large-scale sex differences in neuroscience outcome measures and failed to identify increased variability in female rodents due to estrous cycle (Becker et al., 2016), suggesting that the impact of cycle stage on stress/neuroscience readouts may be relatively small. It is also important to consider that some stress paradigms are extremely difficult to apply to females and may need significant modification to be applied to appropriately. For example, chromogenic activation of the ventromedial hypothalamus was required to induce male aggressors to attack female test mice (Takahashi et al., 2017). When addressing age interactions in response to stress within females, careful consideration of species differences in the trajectory of reproductive senescence as well as the biological consequences of surgical hormone depletion is also warrented (Engler-Chiurazzi et al., 2017). Of note, there are potentially independent cognitive contributions of ovaries versus uterus (Koebele et al., 2019) and consideration of the entire reproducitve system is necessary to comprehensively discern immune-sex hormone interactions with mood. Finally, investigators should not limit themselves to studying only populations that display maladaptive stress response profiles but should also consider exploration of subjects that display stress resiliency; important understanding in this domain is actively being advanced (Russo et al., 2012; Faye et al., 2018).
Sick as a Mouse: Can Rodent Models Effectively Recapitulate Human Immunity?
From an immunological perspective, consideration of the limitations of the experimental model leveraged to study the convergence of endocrine-immune factors within mental health is of paramount importance. First, species differences among humans vs. rodents in the development, total numbers, and functional ability of a variety of immune cell subsets have been long established and comprehensively discussed (Mestas and Hughes, 2004). For example, notable differences in innate immune responses including neutrophil defensin expression, toll-like receptor distribution, and macrophage function as it relates to nitric oxide, and natural killer cell inhibitor receptors for major histocompatibility complex I molecules between humans and rodents have been observed (Mestas and Hughes, 2004). Peripheral leukocyte profiles also vary by species such that up to 70% of immune cells in human blood are granulocytes (such as neutrophils) while lymphocytes make up approximately 30% of cells; monocytes are up to 10%, and other cell populations are more rare (Mestas and Hughes, 2004; Olin et al., 2018). In contrast, rodents display some sex differences in total blood leukocyte counts though importantly in both sexes lymphocytes, at between ∼75-90% for males and females, respectively, were the dominant immune cell type in circulation while neutrophil counts ranged between 24 and 8% (Doeing et al., 2003). Species differences in adaptive immune responses have also been reported, including variations in Fc receptor and Ig isotype expression, the regulation of T and B cell development, and the functional response of lymphocytes to antigen challenge (Mestas and Hughes, 2004). The consequences of these variations may be significant when attempting to address consequences of immunogens that exhibit host-specific patterns of infection, such as cytomegalovirus (Mestas and Hughes, 2004; Masopust et al., 2017), leading some researchers to suggest that rodents poorly recapitulate human injury or disease-associated inflammatory cascades and to advocate caution in the utilization of rodent models for immune-focused research questions (Seok et al., 2013).
As well, research mice are raised in specific pathogen free vivarium facilities that abide guidelines for cleanliness from regulatory organizations such as the United States Department of Agriculture and Association for the Assessment and Accreditation of Laboratory Animal Care accrediting bodies. While such practices support the health and welfare of laboratory rodents and promote reproducibility of data generated in a variety of fields, it is now recognized that pathogen free mice have immature immune systems that are functionally distinct from laboratory mice deliberately exposed to pathogens, from wild caught or pet-store reared mice, and from the human populations they are meant to model (Abolins et al., 2017; Masopust et al., 2017; Tao and Reese, 2017). For instance, adult humans have differentiated memory CD8+ T cell subsets that are not observed in laboratory mice raised in typical pathogen-free conditions; co-housing mice with more antigen experienced pet-store mice can “humanize” their immune profiles, potentially improving their translational validity (Beura et al., 2016). Importantly, antigen exposure history shapes the function of the immune system (Beura et al., 2016; Tao and Reese, 2017), an important consideration given that emerging evidence implicates a higher infection burden with several negative neurological and cognitive consequences across the aging trajectory. Whether these factors manifest in functionally significant impacts for immunity as it relates to mental health is not yet clear and will be an important area of future study as the field evolves. Increased interest among both scientists and funding organizations in the rethinking of the research pipeline, the utilization of “dirty” mice, and the deploying of novel sequencing methods that capture the complexity of immune responses to explore key research questions may reveal more translational insights in the coming years (Shultz, 2016; Tao and Reese, 2017; Wagar et al., 2018).
Challenges Investigating Mood and Immunity in Human Populations
Many factors contribute to challenges in successful translation of preclinical findings to human populations. Here we will highlight variability in human immune profiles (Brodin and Davis, 2017) as well as mood disorder manifestations (Altemus et al., 2014). Immune profiles in middle aged adults evaluated longitudinally over the course of one year display some intra-individual variability that varies in magnitude from subject to subject and may be predictive of overall health (Lakshmikanth et al., 2020). Immune variability is also prevalent across individuals as immune profiles of the very young (Olin et al., 2018) and the very old (Kaczorowski et al., 2017) exhibit more heterogenous composition than do those of adults. As immune composition of monozygotic twins become increasingly distinct with time, the shaping of individual immune profiles is likely due to a combination of heritable and environmental influences (Brodin et al., 2015). Further, the numerous and sometimes vague or opposing diagnostic criteria used to identify clinically depressed patients leads to a potentially highly variable subject pool that likely reflects distinct MDD sub-phenotypes (Zimmerman et al., 2015; LeGates et al., 2019). To account for this variability when evaluating variables of interest, a straightforward statistical solution is to increase sample size (Keppel and Wickens, 2004). However, many of the seminal papers exploring immune profile variations among depressed and mentally healthy populations had stressed/depressed participant numbers of less than 50 (Darko et al., 1988; Maes et al., 1990, 1992a; Petitto et al., 1993; McGregor et al., 2016; Ahmetspahic et al., 2018). This potential under-sampling not only presents challenges to replicability of the significant differences of immune readouts revealed in each study, but could also indicate a lack of statistical power to detect more subtle differences (Keppel and Wickens, 2004). However, many studies do not report observed power nor effect sizes, limiting the ability to make such determinations. These collective factors may contribute to potentially large intra-individual differences that make evaluation of the convergence of mood and immune function a significant logistical challenge. Robust assessment of immune-mood-sex interactions with statistically powerful meta-analysis approaches will become more feasible as additional investigations are conducted.
Conclusion
In summary, collective evidence addressing the unique affective contributions of genetic sex, sex hormones, reproductive capacity, and immunity has already expanded the prevailing ‘monoamine theory of depression’ and yielded improved understanding of the mechanisms driving disordered mood. Given the complex interactions that take place across the female lifespan between these systems, due consideration of how these factors acting in concert may converge to modulate mood is necessary. This will be made possible by adherence to new policies in the consideration of key biological variables, the inclusion of diverse subject populations and the reporting of findings based on population factors such as sex, reproductive experience, and age. The goal of this expanded appreciation for neuro-endo-immune factors in modulating mood is an increased appreciation for the mechanisms driving the manifestation of MDD and other mood disorders and revelation of novel, potentially sex or age-specific therapeutic interventions; we look forward to this outcome.
Author Contributions
All authors listed have made a substantial, direct, and intellectual contribution to the work, and approved it for publication.
Funding
This work was supported by funds awarded to EE-C including NIH K01 MH117343, NIH P20 GM109036, the Tulane Brain Institute Research Fund Award and start-up funds provided by Tulane University.
Conflict of Interest
The authors declare that the research was conducted in the absence of any commercial or financial relationships that could be construed as a potential conflict of interest.
Publisher’s Note
All claims expressed in this article are solely those of the authors and do not necessarily represent those of their affiliated organizations, or those of the publisher, the editors and the reviewers. Any product that may be evaluated in this article, or claim that may be made by its manufacturer, is not guaranteed or endorsed by the publisher.
Abbreviations
5-HT, serotonin; E2, estradiol; ABC, age-associated B cell; CNS, central nervous system; ER, estrogen receptor; HRT, hormone replacement therapy; IL, interleukin; Ig, Immunoglobulin; LPS, lipopolysaccharide; MDD, major depressive disorder; NK, natural killer (cell); rTMS, repetitive transcranial magnetic stimulation; SSRI, selective serotonin reuptake inhibitor; TNF, tumor necrosis factor.
References
Abdi, F., Mobedi, H., Mosaffa, N., Dolatian, M., and Ramezani Tehrani, F. (2016). Effects of hormone replacement therapy on immunological factors in the postmenopausal period. Climacteric 19, 234–239. doi: 10.3109/13697137.2016.1164136
Abdullah, M., Chai, P. S., Chong, M. Y., Tohit, E. R., Ramasamy, R., Pei, C. P., et al. (2012). Gender effect on in vitro lymphocyte subset levels of healthy individuals. Cell Immunol. 272, 214–219. doi: 10.1016/j.cellimm.2011.10.009
Abildgaard, J., Tingstedt, J., Zhao, Y., Hartling, H. J., Pedersen, A. T., Lindegaard, B., et al. (2020). Increased systemic inflammation and altered distribution of T-cell subsets in postmenopausal women. PLoS One 15:e0235174. doi: 10.1371/journal.pone.0235174
Abolins, S., King, E. C., Lazarou, L., Weldon, L., Hughes, L., Drescher, P., et al. (2017). The comparative immunology of wild and laboratory mice, Mus musculus domesticus. Nat. Commun. 8:14811. doi: 10.1038/ncomms14811
Ackerman, L. S. (2006). Sex hormones and the genesis of autoimmunity. Arch. Dermatol. 142, 371–376. doi: 10.1001/archderm.142.3.371
Adzic, M., Brkic, Z., Mitic, M., Francija, E., Jovicic, M. J., Radulovic, J., et al. (2018). Therapeutic strategies for treatment of inflammation-related depression. Curr. Neuropharmacol. 16, 176–209. doi: 10.2174/1570159x15666170828163048
Adzic, M., Djordjevic, J., Mitic, M., Brkic, Z., Lukic, I., and Radojcic, M. (2015). The contribution of hypothalamic neuroendocrine, neuroplastic and neuroinflammatory processes to lipopolysaccharide-induced depressive-like behaviour in female and male rats: involvement of glucocorticoid receptor and C/EBP-β. Behav. Brain Res. 291, 130–139. doi: 10.1016/j.bbr.2015.05.029
Ahmetspahic, D., Schwarte, K., Ambrée, O., Bürger, C., Falcone, V., Seiler, K., et al. (2018). Altered B cell homeostasis in patients with major depressive disorder and normalization of CD5 surface expression on regulatory B cells in treatment responders. J. Neuroimmune Pharmacol. 13, 90–99. doi: 10.1007/s11481-017-9763-4
Aiello, A., Farzaneh, F., Candore, G., Caruso, C., Davinelli, S., Gambino, C. M., et al. (2019). Immunosenescence and its hallmarks: how to oppose aging strategically? A review of potential options for therapeutic intervention. Front. Immunol. 10:2247. doi: 10.3389/fimmu.2019.02247
Akil, H., Gordon, J., Hen, R., Javitch, J., Mayberg, H., McEwen, B., et al. (2018). Treatment resistant depression: a multi-scale, systems biology approach. Neurosci. Biobehav. Rev. 84, 272–288. doi: 10.1016/j.neubiorev.2017.08.019
Albrecht, A. E., Hartmann, B. W., Scholten, C., Huber, J. C., Kalinowska, W., and Zielinski, C. C. (1996). Effect of estrogen replacement therapy on natural killer cell activity in postmenopausal women. Maturitas 25, 217–222. doi: 10.1016/s0378-5122(96)01063-8
Al-Harbi, K. S. (2012). Treatment-resistant depression: therapeutic trends, challenges, and future directions. Patient Prefer Adherence 6, 369–388. doi: 10.2147/ppa.S29716
Altemus, M., Sarvaiya, N., and Neill Epperson, C. (2014). Sex differences in anxiety and depression clinical perspectives. Front. Neuroendocrinol. 35:320–330. doi: 10.1016/j.yfrne.2014.05.004
Arnegard, M. E., Whitten, L. A., Hunter, C., and Clayton, J. A. (2020). Sex as a biological variable: a 5-year progress report and call to action. J. Womens Health (Larchmt) 29, 858–864. doi: 10.1089/jwh.2019.8247
Arruvito, L., Giulianelli, S., Flores, A. C., Paladino, N., Barboza, M., Lanari, C., et al. (2008). NK cells expressing a progesterone receptor are susceptible to progesterone-induced apoptosis. J. Immunol. 180, 5746–5753. doi: 10.4049/jimmunol.180.8.5746
Arteaga-Henríquez, G., Simon, M. S., Burger, B., Weidinger, E., Wijkhuijs, A., Arolt, V., et al. (2019). Low-grade inflammation as a predictor of antidepressant and anti-inflammatory therapy response in mdd patients: a systematic review of the literature in combination with an analysis of experimental data collected in the EU-MOODINFLAME Consortium. Front. Psychiatry 10:458. doi: 10.3389/fpsyt.2019.00458
Baker, F. C., and Driver, H. S. (2007). Circadian rhythms, sleep, and the menstrual cycle. Sleep Med. 8, 613–622. doi: 10.1016/j.sleep.2006.09.011
Bale, T. L., and Epperson, C. N. (2017). Sex as a biological variable: who, what, when, why, and how. Neuropsychopharmacology 42, 386–396. doi: 10.1038/npp.2016.215
Becker, J. B., Prendergast, B. J., and Liang, J. W. (2016). Female rats are not more variable than male rats: a meta-analysis of neuroscience studies. Biol. Sex Differ. 7, 34. doi: 10.1186/s13293-016-0087-5
Bekhbat, M., and Neigh, G. N. (2018). Sex differences in the neuro-immune consequences of stress: focus on depression and anxiety. Brain Behav. Immun. 67, 1–12. doi: 10.1016/j.bbi.2017.02.006
Bekku, N., Yoshimura, H., and Araki, H. (2006). Factors producing a menopausal depressive-like state in mice following ovariectomy. Psychopharmacology (Berl) 187, 170–180. doi: 10.1007/s00213-006-0395-2
Bernardi, M., Vergoni, A. V., Sandrini, M., Tagliavini, S., and Bertolini, A. (1989). Influence of ovariectomy, estradiol and progesterone on the behavior of mice in an experimental model of depression. Physiol. Behav. 45, 1067–1068. doi: 10.1016/0031-9384(89)90238-2
Beura, L. K., Hamilton, S. E., Bi, K., Schenkel, J. M., Odumade, O. A., Casey, K. A., et al. (2016). Normalizing the environment recapitulates adult human immune traits in laboratory mice. Nature 532, 512–516. doi: 10.1038/nature17655
Beurel, E., Harrington, L. E., and Jope, R. S. (2013). Inflammatory T helper 17 cells promote depression-like behavior in mice. Biol. Psychiatry 73, 622–630. doi: 10.1016/j.biopsych.2012.09.021
Bogdanova, O. V., Kanekar, S., D’Anci, K. E., and Renshaw, P. F. (2013). Factors influencing behavior in the forced swim test. Physiol. Behav. 118, 227–239. doi: 10.1016/j.physbeh.2013.05.012
Brachman, R. A., Lehmann, M. L., Maric, D., and Herkenham, M. (2015). Lymphocytes from chronically stressed mice confer antidepressant-like effects to naive mice. J. Neurosci. 35, 1530–1538. doi: 10.1523/jneurosci.2278-14.2015
Brinton, R. D. (2009). Estrogen-induced plasticity from cells to circuits: predictions for cognitive function. Trends Pharmacol. Sci. 30, 212–222. doi: 10.1016/j.tips.2008.12.006
Brkic, Z., Francija, E., Petrovic, Z., Franic, D., Lukic, I., Mitic, M., et al. (2017). Distinct modifications of hippocampal glucocorticoid receptor phosphorylation and FKBPs by lipopolysaccharide in depressive female and male rats. J. Psychopharmacol. 31, 1234–1249. doi: 10.1177/0269881117725914
Brodin, P., and Davis, M. M. (2017). Human immune system variation. Nat. Rev. Immunol 17, 21–29. doi: 10.1038/nri.2016.125
Brodin, P., Jojic, V., Gao, T., Bhattacharya, S., Angel, C. J., Furman, D., et al. (2015). Variation in the human immune system is largely driven by non-heritable influences. Cell 160, 37–47. doi: 10.1016/j.cell.2014.12.020
Brody, D. J., Pratt, L. A., and Hughes, J. P. (2018). Prevalence of depression among adults aged 20 and Over: United States, 2013-2016. NCHS Data Brief. 303, 1–8.
Brown, E. S., and Chandler, P. A. (2001). Mood and cognitive changes during systemic corticosteroid therapy. Prim. Care Companion J. Clin. Psychiatry 3, 17–21. doi: 10.4088/pcc.v03n0104
Bullock, J., Rizvi, S. A. A., Saleh, A. M., Ahmed, S. S., Do, D. P., Ansari, R. A., et al. (2018). Rheumatoid arthritis: a brief overview of the treatment. Med. Princ. Pract. 27, 501–507. doi: 10.1159/000493390
Cagnacci, A., and Venier, M. (2019). The controversial history of hormone replacement therapy. Medicina (Kaunas) 55, 602. doi: 10.3390/medicina55090602
Carboni, L., McCarthy, D. J., Delafont, B., Filosi, M., Ivanchenko, E., Ratti, E., et al. (2019). Biomarkers for response in major depression: comparing paroxetine and venlafaxine from two randomised placebo-controlled clinical studies. Transl. Psychiatry 9, 182. doi: 10.1038/s41398-019-0521-7
Chaplin, D. D. (2010). Overview of the immune response. J. Allergy Clin. Immunol. 125(2 Suppl. 2), S3–S23. doi: 10.1016/j.jaci.2009.12.980
Chen, R. Y., Fan, Y. M., Zhang, Q., Liu, S., Li, Q., Ke, G. L., et al. (2015). Estradiol inhibits Th17 cell differentiation through inhibition of RORγT transcription by recruiting the ERα/REA complex to estrogen response elements of the RORγT promoter. J. Immunol. 194, 4019–4028. doi: 10.4049/jimmunol.1400806
Chen, Y., Zhang, Y., Zhao, G., Chen, C., Yang, P., Ye, S., et al. (2016). Difference in leukocyte composition between women before and after menopausal age, and distinct sexual dimorphism. PLoS One 11:e0162953. doi: 10.1371/journal.pone.0162953
Clark, S. M., Soroka, J. A., Song, C., Li, X., and Tonelli, L. H. (2016). CD4(+) T cells confer anxiolytic and antidepressant-like effects, but enhance fear memory processes in Rag2(-/-) mice. Stress 19, 303–311. doi: 10.1080/10253890.2016.1191466
Clayton, J. A. (2018). Applying the new SABV (sex as a biological variable) policy to research and clinical care. Physiol. Behav. 187, 2–5. doi: 10.1016/j.physbeh.2017.08.012
Cohen, H., Ziv, Y., Cardon, M., Kaplan, Z., Matar, M. A., Gidron, Y., et al. (2006). Maladaptation to mental stress mitigated by the adaptive immune system via depletion of naturally occurring regulatory CD4+CD25+ cells. J. Neurobiol. 66, 552–563. doi: 10.1002/neu.20249
Confavreux, C., Hutchinson, M., Hours, M. M., Cortinovis-Tourniaire, P., and Moreau, T. (1998). Rate of pregnancy-related relapse in multiple sclerosis. Pregnancy in Multiple Sclerosis Group. N. Engl. J. Med. 339, 285–291. doi: 10.1056/NEJM199807303390501
Dantzer, R. (2018). Neuroimmune Interactions: from the Brain to the Immune System and Vice Versa. Physiol. Rev. 98, 477–504. doi: 10.1152/physrev.00039.2016
Darko, D. F., Lucas, A. H., Gillin, J. C., Risch, S. C., Golshan, S., Hamburger, R. N., et al. (1988). Cellular immunity and the hypothalamic-pituitary axis in major affective disorder: a preliminary study. Psychiatry Res. 25, 1–9. doi: 10.1016/0165-1781(88)90152-7
de Chaves, G., Moretti, M., Castro, A. A., Dagostin, W., da Silva, G. G., Boeck, C. R., et al. (2009). Effects of long-term ovariectomy on anxiety and behavioral despair in rats. Physiol. Behav. 97, 420–425. doi: 10.1016/j.physbeh.2009.03.016
de Wit, A. E., Booij, S. H., Giltay, E. J., Joffe, H., Schoevers, R. A., and Oldehinkel, A. J. (2020). Association of use of oral contraceptives with depressive symptoms among adolescents and young women. JAMA Psychiatry 77, 52–59. doi: 10.1001/jamapsychiatry.2019.2838
Desai, M. K., and Brinton, R. D. (2019). Autoimmune disease in women: endocrine transition and risk across the lifespan. Front. Endocrinol. (Lausanne) 10:265. doi: 10.3389/fendo.2019.00265
Dey, A., and Hankey Giblin, P. A. (2018). Insights into macrophage heterogeneity and cytokine-induced neuroinflammation in major depressive disorder. Pharmaceuticals (Basel) 11:64. doi: 10.3390/ph11030064
Doeing, D. C., Borowicz, J. L., and Crockett, E. T. (2003). Gender dimorphism in differential peripheral blood leukocyte counts in mice using cardiac, tail, foot, and saphenous vein puncture methods. BMC Clin Pathol 3:3. doi: 10.1186/1472-6890-3-3
Domínguez-Gerpe, L., and Rey-Méndez, M. (2001). Alterations induced by chronic stress in lymphocyte subsets of blood and primary and secondary immune organs of mice. BMC Immunol 2:7. doi: 10.1186/1471-2172-2-7
Dowlati, Y., Herrmann, N., Swardfager, W., Liu, H., Sham, L., Reim, E. K., et al. (2010). A meta-analysis of cytokines in major depression. Biol. Psychiatry 67, 446–457. doi: 10.1016/j.biopsych.2009.09.033
D’Souza, D., and Sadananda, M. (2017). Estrous cycle phase-dependent changes in anxiety- and depression-like profiles in the late adolescent wistar-kyoto rat. Ann. Neurosci. 24, 136–145. doi: 10.1159/000477151
Duke, J. M., Sibbritt, D. W., and Young, A. F. (2007). Is there an association between the use of oral contraception and depressive symptoms in young Australian women? Contraception 75, 27–31. doi: 10.1016/j.contraception.2006.08.002
Eid, R. S., Gobinath, A. R., and Galea, L. A. M. (2019). Sex differences in depression: insights from clinical and preclinical studies. Prog. Neurobiol. 176, 86–102. doi: 10.1016/j.pneurobio.2019.01.006
Engelmann, F., Rivera, A., Park, B., Messerle-Forbes, M., Jensen, J. T., and Messaoudi, I. (2016). Impact of estrogen therapy on lymphocyte homeostasis and the response to seasonal influenza vaccine in post-menopausal women. PLoS One 11:e0149045. doi: 10.1371/journal.pone.0149045
Engler, H., Benson, S., Wegner, A., Spreitzer, I., Schedlowski, M., and Elsenbruch, S. (2016). Men and women differ in inflammatory and neuroendocrine responses to endotoxin but not in the severity of sickness symptoms. Brain Behav. Immun. 52, 18–26. doi: 10.1016/j.bbi.2015.08.013
Engler-Chiurazzi, E. B., Brown, C. M., Povroznik, J. M., and Simpkins, J. W. (2017). Estrogens as neuroprotectants: estrogenic actions in the context of cognitive aging and brain injury. Prog. Neurobiol. 157, 188–211. doi: 10.1016/j.pneurobio.2015.12.008
Esteve-Solé, A., Luo, Y., Vlagea, A., Deyà-Martínez, Á, Yagüe, J., Plaza-Martín, A. M., et al. (2018). B regulatory cells: players in pregnancy and early life. Int. J. Mol. Sci. 19:2099. doi: 10.3390/ijms19072099
Faas, M. M., and De Vos, P. (2018). Innate immune cells in the placental bed in healthy pregnancy and preeclampsia. Placenta 69, 125–133. doi: 10.1016/j.placenta.2018.04.012
Fairweather, D., Frisancho-Kiss, S., and Rose, N. R. (2008). Sex differences in autoimmune disease from a pathological perspective. Am. J. Pathol. 173, 600–609. doi: 10.2353/ajpath.2008.071008
Faravelli, C., Alessandra Scarpato, M., Castellini, G., and Lo Sauro, C. (2013). Gender differences in depression and anxiety: the role of age. Psychiatry Res. 210, 1301–1303. doi: 10.1016/j.psychres.2013.09.027
Fauchais, A. L., Lalloué, F., Lise, M. C., Boumediene, A., Preud’homme, J. L., Vidal, E., et al. (2008). Role of endogenous brain-derived neurotrophic factor and sortilin in B cell survival. J. Immunol. 181, 3027–3038. doi: 10.4049/jimmunol.181.5.3027
Faye, C., McGowan, J. C., Denny, C. A., and David, D. J. (2018). Neurobiological mechanisms of stress resilience and implications for the aged population. Curr. Neuropharmacol. 16, 234–270. doi: 10.2174/1570159x15666170818095105
FDA (2019). FDA Approves New Nasal Spray Medication for Treatment-Resistant Depression. Silver Spring, MD: FDA.
Ferrari, A. J., Charlson, F. J., Norman, R. E., Flaxman, A. D., Patten, S. B., Vos, T., et al. (2013a). The epidemiological modelling of major depressive disorder: application for the Global Burden of Disease Study 2010. PLoS One 8:e69637. doi: 10.1371/journal.pone.0069637
Ferrari, A. J., Charlson, F. J., Norman, R. E., Patten, S. B., Freedman, G., Murray, C. J., et al. (2013b). Burden of depressive disorders by country, sex, age, and year: findings from the global burden of disease study 2010. PLoS Med. 10:e1001547. doi: 10.1371/journal.pmed.1001547
Filiano, A. J., Gadani, S. P., and Kipnis, J. (2017). How and why do T cells and their derived cytokines affect the injured and healthy brain? Nat. Rev. Neurosci. 18, 375–384. doi: 10.1038/nrn.2017.39
Fletcher, H. (2010). Han JY, Choi JS, Chun JM, Park HD, Lee SY, Kim CH, et al. 2009. Pregnancy outcome of women transfused during pregnancy with blood products inadvertently obtained from donors treated with acitretin. Journal of Obstetric and Gynaecology. 29:694-697. J. Obstet. Gynaecol. 30, 333; author reply 334. doi: 10.3109/01443610903506198
Franklin, R. D., and Kutteh, W. H. (1999). Characterization of immunoglobulins and cytokines in human cervical mucus: influence of exogenous and endogenous hormones. J. Reprod. Immunol. 42, 93–106. doi: 10.1016/s0165-0378(98)00086-2
Frick, L. R., Arcos, M. L., Rapanelli, M., Zappia, M. P., Brocco, M., Mongini, C., et al. (2009). Chronic restraint stress impairs T-cell immunity and promotes tumor progression in mice. Stress 12, 134–143. doi: 10.1080/10253890802137437
Fulop, T., Larbi, A., Dupuis, G., Le Page, A., Frost, E. H., Cohen, A. A., et al. (2017). Immunosenescence and inflamm-aging as two sides of the same coin: friends or foes? Front. Immunol. 8:1960. doi: 10.3389/fimmu.2017.01960
Galea, L. A., Wide, J. K., and Barr, A. M. (2001). Estradiol alleviates depressive-like symptoms in a novel animal model of post-partum depression. Behav. Brain Res. 122, 1–9. doi: 10.1016/s0166-4328(01)00170-x
Gameiro, C. M., Romão, F., and Castelo-Branco, C. (2010). Menopause and aging: changes in the immune system–a review. Maturitas 67, 316–320. doi: 10.1016/j.maturitas.2010.08.003
Ganor, Y., and Levite, M. (2012). “Glutamate in the immune system: glutamate receptors in immune cells, potent effects, endogenous production and involvement in disease,” in Nerve-Driven Immunity: Neurotransmitters and Neuropeptides in the Immune System, ed. M. Levite (Vienna: Springer), 121–161. doi: 10.1007/978-3-7091-0888-8_4
Ghosh, M., Rodriguez-Garcia, M., and Wira, C. R. (2014). The immune system in menopause: pros and cons of hormone therapy. J. Steroid Biochem. Mol. Biol. 142, 171–175. doi: 10.1016/j.jsbmb.2013.09.003
Giglio, T., Imro, M. A., Filaci, G., Scudeletti, M., Puppo, F., De Cecco, L., et al. (1994). Immune cell circulating subsets are affected by gonadal function. Life Sci. 54, 1305–1312. doi: 10.1016/0024-3205(94)00508-7
Goemaere, S., Ackerman, C., Goethals, K., De Keyser, F., Van der Straeten, C., Verbruggen, G., et al. (1990). Onset of symptoms of rheumatoid arthritis in relation to age, sex and menopausal transition. J. Rheumatol. 17, 1620–1622.
Gold, P. W., Pavlatou, M. G., Carlson, P. J., Luckenbaugh, D. A., Costello, R., Bonne, O., et al. (2012). Unmedicated, remitted patients with major depression have decreased serum immunoglobulin A. Neurosci. Lett. 520, 1–5. doi: 10.1016/j.neulet.2012.04.072
Golden, S. A., Covington, H. E. III., Berton, O., and Russo, S. J. (2011). A standardized protocol for repeated social defeat stress in mice. Nat. Protoc. 6, 1183–1191. doi: 10.1038/nprot.2011.361
Gordon, J. L., Rubinow, D. R., Eisenlohr-Moul, T. A., Leserman, J., and Girdler, S. S. (2016). Estradiol variability, stressful life events, and the emergence of depressive symptomatology during the menopausal transition. Menopause 23, 257–266. doi: 10.1097/gme.0000000000000528
Gracia, C. R., and Freeman, E. W. (2018). Onset of the menopause transition: the earliest signs and symptoms. Obstet. Gynecol. Clin. North Am. 45, 585–597. doi: 10.1016/j.ogc.2018.07.002
Greenberg, P. E., Fournier, A. A., Sisitsky, T., Pike, C. T., and Kessler, R. C. (2015). The economic burden of adults with major depressive disorder in the United States (2005 and 2010). J. Clin. Psychiatry 76, 155–162. doi: 10.4088/JCP.14m09298
Griggs, R. C., Condemi, J. J., and Vaughan, J. H. (1972). Effect of therapeutic dosages of prednisone on human immunoglobulin G metabolism. J. Allergy Clin. Immunol. 49, 267–273. doi: 10.1016/0091-6749(72)90094-2
Gruver-Yates, A. L., Quinn, M. A., and Cidlowski, J. A. (2014). Analysis of glucocorticoid receptors and their apoptotic response to dexamethasone in male murine B cells during development. Endocrinology 155, 463–474. doi: 10.1210/en.2013-1473
Gubbels Bupp, M. R., Potluri, T., Fink, A. L., and Klein, S. L. (2018). The confluence of sex hormones and aging on immunity. Front. Immunol. 9:1269. doi: 10.3389/fimmu.2018.01269
Guven Yorgun, Y., and Ozakbas, S. (2019). Effect of hormonal changes on the neurological status in the menstrual cycle of patient with multiple sclerosis. Clin. Neurol. Neurosurg. 186:105499. doi: 10.1016/j.clineuro.2019.105499
Hales, C. A., Stuart, S. A., Anderson, M. H., and Robinson, E. S. (2014). Modelling cognitive affective biases in major depressive disorder using rodents. Br. J. Pharmacol. 171, 4524–4538. doi: 10.1111/bph.12603
Herkenham, M., and Kigar, S. L. (2017). Contributions of the adaptive immune system to mood regulation: mechanisms and pathways of neuroimmune interactions. Prog. Neuropsychopharmacol. Biol. Psychiatry 79(Pt A), 49–57. doi: 10.1016/j.pnpbp.2016.09.003
Hernandez, M. E., Martinez-Fong, D., Perez-Tapia, M., Estrada-Garcia, I., Estrada-Parra, S., and Pavón, L. (2010). Evaluation of the effect of selective serotonin-reuptake inhibitors on lymphocyte subsets in patients with a major depressive disorder. Eur. Neuropsychopharmacol. 20, 88–95. doi: 10.1016/j.euroneuro.2009.11.005
Herr, N., Bode, C., and Duerschmied, D. (2017). The effects of serotonin in immune cells. Front. Cardiovasc. Med. 4:48. doi: 10.3389/fcvm.2017.00048
Hewagama, A., Patel, D., Yarlagadda, S., Strickland, F. M., and Richardson, B. C. (2009). Stronger inflammatory/cytotoxic T-cell response in women identified by microarray analysis. Genes Immun. 10, 509–516. doi: 10.1038/gene.2009.12
Hiroi, R., Weyrich, G., Koebele, S. V., Mennenga, S. E., Talboom, J. S., Hewitt, L. T., et al. (2016). Benefits of hormone therapy estrogens depend on estrogen type: 17β-estradiol and conjugated equine estrogens have differential effects on cognitive, anxiety-like, and depressive-like behaviors and increase tryptophan hydroxylase-2 MRNA levels in dorsal raphe nucleus subregions. Front. Neurosci. 10:517. doi: 10.3389/fnins.2016.00517
Holroyd, C. R., and Edwards, C. J. (2009). The effects of hormone replacement therapy on autoimmune disease: rheumatoid arthritis and systemic lupus erythematosus. Climacteric 12, 378–386. doi: 10.1080/13697130903025449
Hsu, P., and Nanan, R. K. (2014). Innate and adaptive immune interactions at the fetal-maternal interface in healthy human pregnancy and pre-eclampsia. Front. Immunol. 5:125. doi: 10.3389/fimmu.2014.00125
Huang, C. C., Wei, I. H., Chou, Y. H., and Su, T. P. (2008). Effect of age, gender, menopausal status, and ovarian hormonal level on rTMS in treatment-resistant depression. Psychoneuroendocrinology 33, 821–831. doi: 10.1016/j.psyneuen.2008.03.006
Jenkins, J. A., Williams, P., Kramer, G. L., Davis, L. L., and Petty, F. (2001). The influence of gender and the estrous cycle on learned helplessness in the rat. Biol. Psychol. 58, 147–158. doi: 10.1016/s0301-0511(01)00111-9
Joel, D., and McCarthy, M. M. (2017). Incorporating sex as a biological variable in neuropsychiatric research: where are we now and where should we be? Neuropsychopharmacology 42, 379–385. doi: 10.1038/npp.2016.79
Johnson, A., Rainville, J. R., Rivero-Ballon, G. N., Dhimitri, K., and Hodes, G. E. (2021). Testing the limits of sex differences using variable stress. Neuroscience 454, 72–84. doi: 10.1016/j.neuroscience.2019.12.034
Joyce, P. R., Hawes, C. R., Mulder, R. T., Sellman, J. D., Wilson, D. A., and Boswell, D. R. (1992). Elevated levels of acute phase plasma proteins in major depression. Biol. Psychiatry 32, 1035–1041. doi: 10.1016/0006-3223(92)90065-8
Kaczorowski, K. J., Shekhar, K., Nkulikiyimfura, D., Dekker, C. L., Maecker, H., Davis, M. M., et al. (2017). Continuous immunotypes describe human immune variation and predict diverse responses. Proc. Natl. Acad. Sci. U.S.A. 114, E6097–E6106. doi: 10.1073/pnas.1705065114
Kamada, M., Irahara, M., Maegawa, M., Yasui, T., Takeji, T., Yamada, M., et al. (2000). Effect of hormone replacement therapy on post-menopausal changes of lymphocytes and T cell subsets. J. Endocrinol. Invest. 23, 376–382. doi: 10.1007/bf03343741
Keestra, S. M., Male, V., and Salali, G. D. (2021). Out of balance: the role of evolutionary mismatches in the sex disparity in autoimmune disease. Med. Hypotheses 151, 110558. doi: 10.1016/j.mehy.2021.110558
Keppel, G. W., and Wickens, T. D. (2004). Design and Analysis. Saddle River, NJ: Pearson/Prentice Hall.
Keselman, A., Fang, X., White, P. B., and Heller, N. M. (2017). Estrogen signaling contributes to sex differences in macrophage polarization during asthma. J. Immunol. 199, 1573–1583. doi: 10.4049/jimmunol.1601975
Kessler, R. C., Chiu, W. T., Demler, O., Merikangas, K. R., and Walters, E. E. (2005). Prevalence, severity, and comorbidity of 12-month DSM-IV disorders in the National Comorbidity Survey Replication. Arch. Gen. Psychiatry 62, 617–627. doi: 10.1001/archpsyc.62.6.617
Khan, D., and Ansar Ahmed, S. (2015). The Immune system is a natural target for estrogen action: opposing effects of estrogen in two prototypical autoimmune diseases. Front. Immunol. 6:635. doi: 10.3389/fimmu.2015.00635
Kieffer, T. E. C., Laskewitz, A., Scherjon, S. A., Faas, M. M., and Prins, J. R. (2019). Memory T cells in pregnancy. Front. Immunol. 10:625. doi: 10.3389/fimmu.2019.00625
Kim, J., Kim, J. H., and Chang, K. A. (2021). Sex difference in peripheral inflammatory biomarkers in drug-naïve patients with major depression in young adulthood. Biomedicines 9:708. doi: 10.3390/biomedicines9070708
Klein, S. L., and Flanagan, K. L. (2016). Sex differences in immune responses. Nat. Rev. Immunol. 16, 626–638. doi: 10.1038/nri.2016.90
Koebele, S. V., Palmer, J. M., Hadder, B., Melikian, R., Fox, C., Strouse, I. M., et al. (2019). Hysterectomy uniquely impacts spatial memory in a rat model: a role for the nonpregnant uterus in cognitive processes. Endocrinology 160, 1–19. doi: 10.1210/en.2018-00709
Köhler, C. A., Freitas, T. H., Maes, M., de Andrade, N. Q., Liu, C. S., Fernandes, B. S., et al. (2017). Peripheral cytokine and chemokine alterations in depression: a meta-analysis of 82 studies. Acta Psychiatr. Scand. 135, 373–387. doi: 10.1111/acps.12698
Köhler-Forsberg, O., Buttenschøn, H. N., Tansey, K. E., Maier, W., Hauser, J., Dernovsek, M. Z., et al. (2017). Association between C-reactive protein (CRP) with depression symptom severity and specific depressive symptoms in major depression. Brain Behav. Immun. 62, 344–350. doi: 10.1016/j.bbi.2017.02.020
Köhler-Forsberg, O., Lydholm, C. N., Hjorthøj, C., Nordentoft, M., Mors, O., and Benros, M. E. (2019). Efficacy of anti-inflammatory treatment on major depressive disorder or depressive symptoms: meta-analysis of clinical trials. Acta Psychiatr. Scand. 139, 404–419. doi: 10.1111/acps.13016
Kovacs, W. J. (2014). To B or not to B? Glucocorticoid impact on B lymphocyte fate and function. Endocrinology 155, 339–342. doi: 10.1210/en.2013-2085
Krishnan, V., and Nestler, E. J. (2008). The molecular neurobiology of depression. Nature 455, 894–902. doi: 10.1038/nature07455
Kronfol, Z., and House, J. D. (1989). Lymphocyte mitogenesis, immunoglobulin and complement levels in depressed patients and normal controls. Acta Psychiatr. Scand. 80, 142–147. doi: 10.1111/j.1600-0447.1989.tb01316.x
Krop, J., Heidt, S., Claas, F. H. J., and Eikmans, M. (2020). Regulatory T cells in pregnancy: it is not all about foxP3. Front. Immunol. 11:1182. doi: 10.3389/fimmu.2020.01182
Kumru, S., Godekmerdan, A., and Yilmaz, B. (2004). Immune effects of surgical menopause and estrogen replacement therapy in peri-menopausal women. J. Reprod. Immunol. 63, 31–38. doi: 10.1016/j.jri.2004.02.001
Laessle, R. G., Tuschl, R. J., Schweiger, U., and Pirke, K. M. (1990). Mood changes and physical complaints during the normal menstrual cycle in healthy young women. Psychoneuroendocrinology 15, 131–138. doi: 10.1016/0306-4530(90)90021-z
Lakshmikanth, T., Muhammad, S. A., Olin, A., Chen, Y., Mikes, J., Fagerberg, L., et al. (2020). Human Immune System Variation during 1 Year. Cell Rep. 32:107923. doi: 10.1016/j.celrep.2020.107923
Lamason, R., Zhao, P., Rawat, R., Davis, A., Hall, J. C., Chae, J. J., et al. (2006). Sexual dimorphism in immune response genes as a function of puberty. BMC Immunol. 7:2. doi: 10.1186/1471-2172-7-2
Lateef, A., and Petri, M. (2012). Hormone replacement and contraceptive therapy in autoimmune diseases. J. Autoimmun. 38, J170–J176. doi: 10.1016/j.jaut.2011.11.002
Lauer, M. (2016). Consideration of Relevant Biological Variables in NIH Grant Applications [Online]. Bethesda, MD: NIH.
Lee, B. W., Yap, H. K., Chew, F. T., Quah, T. C., Prabhakaran, K., Chan, G. S., et al. (1996). Age- and sex-related changes in lymphocyte subpopulations of healthy Asian subjects: from birth to adulthood. Cytometry 26, 8–15. doi: 10.1002/(SICI)1097-0320(19960315)26:1<8::AID-CYTO2>3.0.CO;2-E
Lee, S., Kim, J., Jang, B., Hur, S., Jung, U., Kil, K., et al. (2010). Fluctuation of peripheral blood T, B, and NK cells during a menstrual cycle of normal healthy women. J. Immunol. 185, 756–762. doi: 10.4049/jimmunol.0904192
LeGates, T. A., Kvarta, M. D., and Thompson, S. M. (2019). Sex differences in antidepressant efficacy. Neuropsychopharmacology 44, 140–154. doi: 10.1038/s41386-018-0156-z
Leonard, B. E. (2010). The concept of depression as a dysfunction of the immune system. Curr. Immunol. Rev. 6, 205–212. doi: 10.2174/157339510791823835
Li, L. H., Wang, Z. C., Yu, J., and Zhang, Y. Q. (2014). Ovariectomy results in variable changes in nociception, mood and depression in adult female rats. PLoS One 9:e94312. doi: 10.1371/journal.pone.0094312
Lim, A. I., Harrison, O. J., and Belkaid, Y. (2019). Pre-birth memory. Nat. Immunol. 20, 254–256. doi: 10.1038/s41590-019-0326-0
Lima, J., Martins, C., Leandro, M. J., Nunes, G., Sousa, M. J., Branco, J. C., et al. (2016). Characterization of B cells in healthy pregnant women from late pregnancy to post-partum: a prospective observational study. BMC Pregnancy Childbirth 16:139. doi: 10.1186/s12884-016-0927-7
Lima, J., Martins, C., Nunes, G., Sousa, M. J., Branco, J. C., and Borrego, L. M. (2017). Regulatory T cells show dynamic behavior during late pregnancy, delivery, and the postpartum period. Reprod. Sci. 24, 1025–1032. doi: 10.1177/1933719116676395
Lissauer, D., Goodyear, O., Khanum, R., Moss, P. A., and Kilby, M. D. (2014). Profile of maternal CD4 T-cell effector function during normal pregnancy and in women with a history of recurrent miscarriage. Clin. Sci. (Lond) 126, 347–354. doi: 10.1042/cs20130247
Lisse, I. M., Aaby, P., Whittle, H., Jensen, H., Engelmann, M., and Christensen, L. B. (1997). T-lymphocyte subsets in West African children: impact of age, sex, and season. J Pediatr 130, 77–85. doi: 10.1016/s0022-3476(97)70313-5
Luca, D. L., Garlow, N., Staatz, C., Margiotta, C., and Zivin, K. (2019). Societal Costs of Untreated Perinatal Mood and Anxiety Disorders in the United States. Princeton, NJ: Mathematica Policy Research.
Lucas, S. M., Rothwell, N. J., and Gibson, R. M. (2006). The role of inflammation in CNS injury and disease. Br. J. Pharmacol. 147 Suppl 1(Suppl 1), S232–S240. doi: 10.1038/sj.bjp.0706400
Lynall, M. E., Kigar, S. L., Lehmann, M. L., DePuyt, A. E., Tuong, Z. K., Listwak, S. J., et al. (2021). B-cells are abnormal in psychosocial stress and regulate meningeal myeloid cell activation. Brain Behav. Immun. 97, 226–238. doi: 10.1016/j.bbi.2021.08.002
Maartens, L. W., Knottnerus, J. A., and Pop, V. J. (2002). Menopausal transition and increased depressive symptomatology: a community based prospective study. Maturitas 42, 195–200. doi: 10.1016/s0378-5122(02)00038-5
Maes, M. (2011). Depression is an inflammatory disease, but cell-mediated immune activation is the key component of depression. Prog. Neuropsychopharmacol. Biol. Psychiatry 35, 664–675. doi: 10.1016/j.pnpbp.2010.06.014
Maes, M., Bosmans, E., Suy, E., Vandervorst, C., De Jonckheere, C., and Raus, J. (1990). Immune disturbances during major depression: upregulated expression of interleukin-2 receptors. Neuropsychobiology 24, 115–120. doi: 10.1159/000119472
Maes, M., Scharpé, S., Van Grootel, L., Uyttenbroeck, W., Cooreman, W., Cosyns, P., et al. (1992b). Higher alpha 1-antitrypsin, haptoglobin, ceruloplasmin and lower retinol binding protein plasma levels during depression: further evidence for the existence of an inflammatory response during that illness. J. Affect. Disord. 24, 183–192. doi: 10.1016/0165-0327(92)90066-f
Maes, M., Lambrechts, J., Bosmans, E., Jacobs, J., Suy, E., Vandervorst, C., et al. (1992a). Evidence for a systemic immune activation during depression: results of leukocyte enumeration by flow cytometry in conjunction with monoclonal antibody staining. Psychol. Med. 22, 45–53. doi: 10.1017/s0033291700032712
Maglione, A., Rolla, S., Mercanti, S. F., Cutrupi, S., and Clerico, M. (2019). The adaptive immune system in multiple sclerosis: an estrogen-mediated point of view. Cells 8:280. doi: 10.3390/cells8101280
Maki, P. M., Kornstein, S. G., Joffe, H., Bromberger, J. T., Freeman, E. W., Athappilly, G., et al. (2019). Guidelines for the evaluation and treatment of perimenopausal depression: summary and recommendations. J. Womens Health (Larchmt) 28, 117–134. doi: 10.1089/jwh.2018.27099.mensocrec
Maki, P. M., and Resnick, S. M. (2001). Effects of estrogen on patterns of brain activity at rest and during cognitive activity: a review of neuroimaging studies. Neuroimage 14, 789–801. doi: 10.1006/nimg.2001.0887
Marcus, S. M., Kerber, K. B., Rush, A. J., Wisniewski, S. R., Nierenberg, A., Balasubramani, G. K., et al. (2008). Sex differences in depression symptoms in treatment-seeking adults: confirmatory analyses from the Sequenced Treatment Alternatives to Relieve Depression study. Compr. Psychiatry 49, 238–246. doi: 10.1016/j.comppsych.2007.06.012
Márquez, E. J., Chung, C. H., Marches, R., Rossi, R. J., Nehar-Belaid, D., Eroglu, A., et al. (2020). Sexual-dimorphism in human immune system aging. Nat. Commun. 11:751. doi: 10.1038/s41467-020-14396-9
Marrocco, J., and McEwen, B. S. (2016). Sex in the brain: hormones and sex differences. Dialogues Clin. Neurosci. 18, 373–383. doi: 10.31887/DCNS.2016.18.4/jmarrocco
Marshall, J. S., Warrington, R., Watson, W., and Kim, H. L. (2018). An introduction to immunology and immunopathology. Allergy Asthma. Clin. Immunol. 14(Suppl. 2), 49. doi: 10.1186/s13223-018-0278-1
Masopust, D., Sivula, C. P., and Jameson, S. C. (2017). Of mice, dirty mice, and men: using mice to understand human immunology. J. Immunol. 199, 383–388. doi: 10.4049/jimmunol.1700453
McGregor, B. A., Murphy, K. M., Albano, D. L., and Ceballos, R. M. (2016). Stress, cortisol, and B lymphocytes: a novel approach to understanding academic stress and immune function. Stress 19, 185–191. doi: 10.3109/10253890.2015.1127913
Melgert, B. N., Ray, A., Hylkema, M. N., Timens, W., and Postma, D. S. (2007). Are there reasons why adult asthma is more common in females? Curr. Allergy Asthma Rep. 7, 143–150. doi: 10.1007/s11882-007-0012-4
Mendoza-Cabrera, M. I., Navarro-Hernández, R. E., Santerre, A., Ortiz-Lazareno, P. C., Pereira-Suárez, A. L., and Estrada-Chávez, C. (2020). Effect of pregnancy hormone mixtures on cytokine production and surface marker expression in naïve and LPS-activated THP-1 differentiated monocytes/macrophages. Innate Immun. 26, 84–96. doi: 10.1177/1753425919864658
Meredith, E. J., Holder, M. J., Chamba, A., Challa, A., Drake-Lee, A., Bunce, C. M., et al. (2005). The serotonin transporter (SLC6A4) is present in B-cell clones of diverse malignant origin: probing a potential anti-tumor target for psychotropics. FASEB J. 19, 1187–1189. doi: 10.1096/fj.04-3477fje
Mestas, J., and Hughes, C. C. (2004). Of mice and not men: differences between mouse and human immunology. J. Immunol. 172, 2731–2738. doi: 10.4049/jimmunol.172.5.2731
Millett, C. E., Phillips, B. E., and Saunders, E. F. H. (2019). The sex-specific effects of lps on depressive-like behavior and oxidative stress in the hippocampus of the mouse. Neuroscience 399, 77–88. doi: 10.1016/j.neuroscience.2018.12.008
Moieni, M., Irwin, M. R., Jevtic, I., Olmstead, R., Breen, E. C., and Eisenberger, N. I. (2015). Sex differences in depressive and socioemotional responses to an inflammatory challenge: implications for sex differences in depression. Neuropsychopharmacology 40, 1709–1716. doi: 10.1038/npp.2015.17
Molnár, I., Bohaty, I., and Somogyiné-Vári, É (2014). High prevalence of increased interleukin-17A serum levels in postmenopausal estrogen deficiency. Menopause 21, 749–752. doi: 10.1097/gme.0000000000000125
Moos, R. H., Kopell, B. S., Melges, F. T., Yalom, I. D., Lunde, D. T., Clayton, R. B., et al. (1969). Fluctuations in symptoms and moods during the menstrual cycle. J Psychosom. Res. 13, 37–44. doi: 10.1016/0022-3999(69)90017-8
Mor, G., and Cardenas, I. (2010). The immune system in pregnancy: a unique complexity. Am. J. Reprod. Immunol. 63, 425–433. doi: 10.1111/j.1600-0897.2010.00836.x
Morimoto, K., and Nakajima, K. (2019). Role of the immune system in the development of the central nervous system. Front. Neurosci. 13:916. doi: 10.3389/fnins.2019.00916
Moulton, V. R. (2018). Sex hormones in acquired immunity and autoimmune disease. Front. Immunol. 9:2279. doi: 10.3389/fimmu.2018.02279
Musabak, U., Bolu, E., Ozata, M., Oktenli, C., Sengul, A., Inal, A., et al. (2003). Gonadotropin treatment restores in vitro interleukin-1beta and tumour necrosis factor-alpha production by stimulated peripheral blood mononuclear cells from patients with idiopathic hypogonadotropic hypogonadism. Clin. Exp. Immunol. 132, 265–270. doi: 10.1046/j.1365-2249.2003.02141.x
Muzzio, D., Zenclussen, A. C., and Jensen, F. (2013). The role of B cells in pregnancy: the good and the bad. Am. J. Reprod. Immunol. 69, 408–412. doi: 10.1111/aji.12079
Nair, R. R., Verma, P., and Singh, K. (2017). Immune-endocrine crosstalk during pregnancy. Gen. Comp. Endocrinol. 242, 18–23. doi: 10.1016/j.ygcen.2016.03.003
Nestler, E. J., and Hyman, S. E. (2010). Animal models of neuropsychiatric disorders. Nat. Neurosci. 13, 1161–1169. doi: 10.1038/nn.2647
Ngo, S. T., Steyn, F. J., and McCombe, P. A. (2014). Gender differences in autoimmune disease. Front. Neuroendocrinol. 35:347–369. doi: 10.1016/j.yfrne.2014.04.004
NIH (2015). Consideration of Sex as a Biological Variable in NIH-funded Research [Online]. Bethesda, MD: NIH.
NIMH (2019). Notice of NIMH’s Considerations Regarding the Use of Animal Neurobehavioral Approaches in Basic and Pre-Clinical Studies [Online]. Bethesda, MD: NIMH.
Oertelt-Prigione, S. (2012). Immunology and the menstrual cycle. Autoimmun. Rev. 11, A486–A492. doi: 10.1016/j.autrev.2011.11.023
Okine, T., Shepard, R., Lemanski, E., and Coutellier, L. (2020). Sex differences in the sustained effects of ketamine on resilience to chronic stress. Front. Behav. Neurosci. 14:581360. doi: 10.3389/fnbeh.2020.581360
Okuyama, K., Suenaga, M., Furuki, S., Kawano, T., Ohkawara, Y., Takayanagi, M., et al. (2013). Contribution of CD4+ T cells and dendritic cells to female-dominant antigen-induced T helper type 2 cytokine production by bronchial lymph node cells. Int. Arch. Allergy Immunol. 161(Suppl. 2), 58–65. doi: 10.1159/000350426
Olin, A., Henckel, E., Chen, Y., Lakshmikanth, T., Pou, C., Mikes, J., et al. (2018). Stereotypic Immune System Development in Newborn Children. Cell 174(5) 127, e1214. doi: 10.1016/j.cell.2018.06.045
Ortona, E., Pierdominici, M., Maselli, A., Veroni, C., Aloisi, F., and Shoenfeld, Y. (2016). Sex-based differences in autoimmune diseases. Ann. Ist Super Sanita 52, 205–212. doi: 10.4415/ann_16_02_12
Ostensen, M., Aune, B., and Husby, G. (1983). Effect of pregnancy and hormonal changes on the activity of rheumatoid arthritis. Scand. J. Rheumatol. 12, 69–72. doi: 10.3109/03009748309102886
Ousman, S. S., and Kubes, P. (2012). Immune surveillance in the central nervous system. Nat. Neurosci. 15, 1096–1101. doi: 10.1038/nn.3161
Pavlov, V. A., Chavan, S. S., and Tracey, K. J. (2018). Molecular and functional neuroscience in immunity. Annu. Rev. Immunol. 36, 783–812. doi: 10.1146/annurev-immunol-042617-053158
Pavón, L., Sandoval-López, G., Eugenia Hernández, M., Loría, F., Estrada, I., Pérez, M., et al. (2006). Th2 cytokine response in Major Depressive Disorder patients before treatment. J. Neuroimmunol. 172, 156–165. doi: 10.1016/j.jneuroim.2005.08.014
Pennell, L. M., Galligan, C. L., and Fish, E. N. (2012). Sex affects immunity. J. Autoimmun. 38, J282–J291. doi: 10.1016/j.jaut.2011.11.013
Petitto, J. M., Folds, J. D., and Evans, D. L. (1993). Abnormal diurnal variation of B lymphocyte circulation patterns in major depression. Biol. Psychiatry 34, 268–270. doi: 10.1016/0006-3223(93)90082-o
Petri, M. (2020). Pregnancy and systemic lupus erythematosus. Best Pract. Res. Clin. Obstet Gynaecol 64, 24–30. doi: 10.1016/j.bpobgyn.2019.09.002
Piantella, S., Dragano, N., McDonald, S. J., and Wright, B. J. (2021). Depression symptoms mediate the association between workplace stress and interleukin 6 in women, but not men: the Whitehall II study. Brain Behav. Immun. Health 12:100215. doi: 10.1016/j.bbih.2021.100215
Poromaa, I. S., and Segebladh, B. (2012). Adverse mood symptoms with oral contraceptives. Acta Obstet. Gynecol. Scand. 91, 420–427. doi: 10.1111/j.1600-0412.2011.01333.x
Porter, V. R., Greendale, G. A., Schocken, M., Zhu, X., and Effros, R. B. (2001). Immune effects of hormone replacement therapy in post-menopausal women. Exp. Gerontol. 36, 311–326. doi: 10.1016/s0531-5565(00)00195-9
Powell, T. R., Fernandes, C., and Schalkwyk, L. C. (2012). Depression-related behavioral tests. Curr. Protoc. Mouse Biol. 2, 119–127. doi: 10.1002/9780470942390.mo110176
Price, R. B., and Duman, R. (2020). Neuroplasticity in cognitive and psychological mechanisms of depression: an integrative model. Mol. Psychiatry 25, 530–543. doi: 10.1038/s41380-019-0615-x
Pryce, C. R., and Fontana, A. (2017). Depression in autoimmune diseases. Curr. Top. Behav. Neurosci. 31, 139–154. doi: 10.1007/7854_2016_7
Pung, O. J., Tucker, A. N., Vore, S. J., and Luster, M. I. (1985). Influence of estrogen on host resistance: increased susceptibility of mice to Listeria monocytogenes correlates with depressed production of interleukin 2. Infect. Immun. 50, 91–96. doi: 10.1128/iai.50.1.91-96.1985
Racicot, K., Kwon, J. Y., Aldo, P., Silasi, M., and Mor, G. (2014). Understanding the complexity of the immune system during pregnancy. Am. J. Reprod. Immunol. 72, 107–116. doi: 10.1111/aji.12289
Ramo-Tello, C., Blanco, Y., Brieva, L., Casanova, B., Martinez-Caceres, E., Ontaneda, D., et al. (2021). Recommendations for the diagnosis and treatment of multiple sclerosis relapses. J. Pers. Med. 12:6. doi: 10.3390/jpm12010006
Ramsey, J. M., Cooper, J. D., Bot, M., Guest, P. C., Lamers, F., Weickert, C. S., et al. (2016). Sex differences in serum markers of major depressive disorder in the Netherlands Study of Depression and Anxiety (NESDA). PLoS One 11:e0156624. doi: 10.1371/journal.pone.0156624
Ransohoff, R. M., Schafer, D., Vincent, A., Blachère, N. E., and Bar-Or, A. (2015). Neuroinflammation: ways in which the immune system affects the brain. Neurotherapeutics 12, 896–909. doi: 10.1007/s13311-015-0385-3
Rattazzi, L., Piras, G., Ono, M., Deacon, R., Pariante, C. M., and D’Acquisto, F. (2013). CD4+ but not CD8+ T cells revert the impaired emotional behavior of immunocompromised RAG-1-deficient mice. Transl. Psychiatry 3:e280. doi: 10.1038/tp.2013.54
Rayasam, A., Kijak, J. A., Dallmann, M., Hsu, M., Zindl, N., Lindstedt, A., et al. (2018). Regional distribution of cns antigens differentially determines T-Cell mediated neuroinflammation in a CX3CR1-dependent manner. J. Neurosci. 38, 7058–7071. doi: 10.1523/jneurosci.0366-18.2018
Robakis, T., Williams, K. E., Nutkiewicz, L., and Rasgon, N. L. (2019). Hormonal contraceptives and mood: review of the literature and implications for future research. Curr. Psychiatry Rep. 21:57. doi: 10.1007/s11920-019-1034-z
Roberts, C. W., Walker, W., and Alexander, J. (2001). Sex-associated hormones and immunity to protozoan parasites. Clin. Microbiol. Rev. 14, 476–488. doi: 10.1128/cmr.14.3.476-488.2001
Robertson, M. J., Schacterle, R. S., Mackin, G. A., Wilson, S. N., Bloomingdale, K. L., Ritz, J., et al. (2005). Lymphocyte subset differences in patients with chronic fatigue syndrome, multiple sclerosis and major depression. Clin. Exp. Immunol. 141, 326–332. doi: 10.1111/j.1365-2249.2005.02833.x
Robinson, D. P., Hall, O. J., Nilles, T. L., Bream, J. H., and Klein, S. L. (2014). 17β-estradiol protects females against influenza by recruiting neutrophils and increasing virus-specific CD8 T cell responses in the lungs. J. Virol. 88, 4711–4720. doi: 10.1128/jvi.02081-13
Robinson, D. P., and Klein, S. L. (2012). Pregnancy and pregnancy-associated hormones alter immune responses and disease pathogenesis. Horm. Behav. 62, 263–271. doi: 10.1016/j.yhbeh.2012.02.023
Rodriguez-Garcia, M., Biswas, N., Patel, M. V., Barr, F. D., Crist, S. G., Ochsenbauer, C., et al. (2013). Estradiol reduces susceptibility of CD4+ T cells and macrophages to HIV-infection. PLoS One 8:e62069. doi: 10.1371/journal.pone.0062069
Rosen, J. L., Tran, H. T., Lackey, A., and Viselli, S. M. (1999). Sex-related immune changes in young mice. Immunol. Invest. 28, 247–256. doi: 10.3109/08820139909060859
Rossetti, A. C., Paladini, M. S., Trepci, A., Mallien, A., Riva, M. A., Gass, P., et al. (2019). Differential neuroinflammatory response in male and female mice: a role for BDNF. Front. Mol. Neurosci. 12:166. doi: 10.3389/fnmol.2019.00166
Roumestan, C., Michel, A., Bichon, F., Portet, K., Detoc, M., Henriquet, C., et al. (2007). Anti-inflammatory properties of desipramine and fluoxetine. Respir. Res. 8:35. doi: 10.1186/1465-9921-8-35
Rubinow, K. B. (2018). An intracrine view of sex steroids, immunity, and metabolic regulation. Mol. Metab. 15, 92–103. doi: 10.1016/j.molmet.2018.03.001
Rubtsova, K., Rubtsov, A. V., Cancro, M. P., and Marrack, P. (2015). Age-associated b cells: a T-bet-dependent effector with roles in protective and pathogenic immunity. J. Immunol. 195, 1933–1937. doi: 10.4049/jimmunol.1501209
Rus, H., Cudrici, C., and Niculescu, F. (2005). The role of the complement system in innate immunity. Immunol. Res. 33, 103–112. doi: 10.1385/ir:33:2:103
Russo, S. J., Murrough, J. W., Han, M. H., Charney, D. S., and Nestler, E. J. (2012). Neurobiology of resilience. Nat. Neurosci. 15, 1475–1484. doi: 10.1038/nn.3234
Saland, S. K., Duclot, F., and Kabbaj, M. (2017). Integrative analysis of sex differences in the rapid antidepressant effects of ketamine in preclinical models for individualized clinical outcomes. Curr. Opin. Behav. Sci. 14, 19–26. doi: 10.1016/j.cobeha.2016.11.002
Sarcevic, J., Cavelti-Weder, C., Berger, C. T., and Trendelenburg, M. (2020). Case report-secondary antibody deficiency due to endogenous hypercortisolism. Front. Immunol. 11:1435. doi: 10.3389/fimmu.2020.01435
Scheinert, R. B., Haeri, M. H., Lehmann, M. L., and Herkenham, M. (2016). Therapeutic effects of stress-programmed lymphocytes transferred to chronically stressed mice. Prog. Neuropsychopharmacol. Biol. Psychiatry 70, 1–7. doi: 10.1016/j.pnpbp.2016.04.010
Schildkraut, J. J. (1965). The catecholamine hypothesis of affective disorders: a review of supporting evidence. Am. J. Psychiatry 122, 509–522. doi: 10.1176/ajp.122.5.509
Schiller, C. E., O’Hara, M. W., Rubinow, D. R., and Johnson, A. K. (2013). Estradiol modulates anhedonia and behavioral despair in rats and negative affect in a subgroup of women at high risk for postpartum depression. Physiol. Behav. 119, 137–144. doi: 10.1016/j.physbeh.2013.06.009
Schleifer, S. J., Bartlett, J. A., Keller, S. E., Eckholdt, H. M., Shiflett, S. C., and Delaney, B. R. (2002). Immunity in adolescents with major depression. J. Am. Acad. Child. Adolesc. Psychiatry 41, 1054–1060. doi: 10.1097/00004583-200209000-00005
Schleifer, S. J., Keller, S. E., Bond, R. N., Cohen, J., and Stein, M. (1989). Major depressive disorder and immunity. Role of age, sex, severity, and hospitalization. Arch. Gen. Psychiatry 46, 81–87. doi: 10.1001/archpsyc.1989.01810010083011
Schmidt, P. J., Nieman, L., Danaceau, M. A., Tobin, M. B., Roca, C. A., Murphy, J. H., et al. (2000). Estrogen replacement in perimenopause-related depression: a preliminary report. Am. J. Obstet. Gynecol. 183, 414–420. doi: 10.1067/mob.2000.106004
Schoenrock, S. A., Oreper, D., Young, N., Ervin, R. B., Bogue, M. A., Valdar, W., et al. (2016). Ovariectomy results in inbred strain-specific increases in anxiety-like behavior in mice. Physiol. Behav. 167, 404–412. doi: 10.1016/j.physbeh.2016.09.026
Schuhmann, B., Dietrich, A., Sel, S., Hahn, C., Klingenspor, M., Lommatzsch, M., et al. (2005). A role for brain-derived neurotrophic factor in B cell development. J. Neuroimmunol. 163, 15–23. doi: 10.1016/j.jneuroim.2005.01.023
Seillet, C., Laffont, S., Trémollières, F., Rouquié, N., Ribot, C., Arnal, J. F., et al. (2012). The TLR-mediated response of plasmacytoid dendritic cells is positively regulated by estradiol in vivo through cell-intrinsic estrogen receptor α signaling. Blood 119, 454–464. doi: 10.1182/blood-2011-08-371831
Seok, J., Warren, H. S., Cuenca, A. G., Mindrinos, M. N., Baker, H. V., Xu, W., et al. (2013). Genomic responses in mouse models poorly mimic human inflammatory diseases. Proc. Natl. Acad. Sci. U.S.A. 110, 3507–3512. doi: 10.1073/pnas.1222878110
Settipane, G. A., Pudupakkam, R. K., and McGowan, J. H. (1978). Corticosteroid effect on immunoglobulins. J. Allergy Clin. Immunol. 62, 162–166. doi: 10.1016/0091-6749(78)90101-x
Shah, S. (2012). Hormonal link to autoimmune allergies. ISRN Allergy 2012:910437. doi: 10.5402/2012/910437
Sharma, R., Kearns, M. M., Sarr, F., and Ismail, N. (2019). The adaptive immune and stress responses of adult female CD1 mice following exposure to a viral mimetic. Immunol. Lett. 208, 30–38. doi: 10.1016/j.imlet.2019.03.005
Shepherd, R., Cheung, A. S., Pang, K., Saffery, R., and Novakovic, B. (2020). Sexual dimorphism in innate immunity: the role of sex hormones and epigenetics. Front. Immunol. 11:604000. doi: 10.3389/fimmu.2020.604000
Shirshev, S. V., Nekrasova, I. V., Gorbunova, O. L., and Orlova, E. G. (2019). Effect of estriol, chorionic gonadotropin, and oncostatin M on the expression of recombinase RAG-1 in regulatory T lymphocyte subpopulations. Bull. Exp. Biol. Med. 167, 57–61. doi: 10.1007/s10517-019-04460-w
Shors, T. J., Millon, E. M., Chang, H. Y., Olson, R. L., and Alderman, B. L. (2017). Do sex differences in rumination explain sex differences in depression? J. Neurosci. Res. 95, 711–718. doi: 10.1002/jnr.23976
Shultz, D. (2016). Can ‘Dirty Mice’ Save Animal Research?. Available online at: https://www.science.org/content/article/can-dirty-mice-save-animal-research (accessed February 25, 2022).
Singh, R. P., and Bischoff, D. S. (2021). Sex Hormones and Gender Influence the Expression of Markers of Regulatory T Cells in SLE Patients. Front Immunol 12:619268. doi: 10.3389/fimmu.2021.619268
Skovlund, C. W., Morch, L. S., Kessing, L. V., and Lidegaard, O. (2016). Association of hormonal contraception with depression. JAMA Psychiatry 73, 1154–1162. doi: 10.1001/jamapsychiatry.2016.2387
Soares, C. N. (2017). Depression and menopause: current knowledge and clinical recommendations for a critical window. Psychiatr. Clin. North Am. 40, 239–254. doi: 10.1016/j.psc.2017.01.007
Soares, C. N., Almeida, O. P., Joffe, H., and Cohen, L. S. (2001). Efficacy of estradiol for the treatment of depressive disorders in perimenopausal women: a double-blind, randomized, placebo-controlled trial. Arch. Gen. Psychiatry 58, 529–534. doi: 10.1001/archpsyc.58.6.529
Somerset, D. A., Zheng, Y., Kilby, M. D., Sansom, D. M., and Drayson, M. T. (2004). Normal human pregnancy is associated with an elevation in the immune suppressive CD25+ CD4+ regulatory T-cell subset. Immunology 112, 38–43. doi: 10.1111/j.1365-2567.2004.01869.x
Song, C., Dinan, T., and Leonard, B. E. (1994). Changes in immunoglobulin, complement and acute phase protein levels in the depressed patients and normal controls. J. Affect. Disord. 30, 283–288. doi: 10.1016/0165-0327(94)90135-x
Steel, Z., Marnane, C., Iranpour, C., Chey, T., Jackson, J. W., Patel, V., et al. (2014). The global prevalence of common mental disorders: a systematic review and meta-analysis 1980-2013. Int. J. Epidemiol. 43, 476–493. doi: 10.1093/ije/dyu038
Stopińska-Głuszak, U., Waligóra, J., Grzela, T., Głuszak, M., Jóźwiak, J., Radomski, D., et al. (2006). Effect of estrogen/progesterone hormone replacement therapy on natural killer cell cytotoxicity and immunoregulatory cytokine release by peripheral blood mononuclear cells of postmenopausal women. J. Reprod. Immunol. 69, 65–75. doi: 10.1016/j.jri.2005.07.006
Sundström-Poromaa, I. (2018). The menstrual cycle influences emotion but has limited effect on cognitive function. Vitam Horm 107, 349–376. doi: 10.1016/bs.vh.2018.01.016
Swartz, J. R., Prather, A. A., Di Iorio, C. R., Bogdan, R., and Hariri, A. R. (2017). A Functional interleukin-18 haplotype predicts depression and anxiety through increased threat-related amygdala reactivity in women but not men. Neuropsychopharmacology 42, 419–426. doi: 10.1038/npp.2016.129
Takahashi, A., Chung, J. R., Zhang, S., Zhang, H., Grossman, Y., Aleyasin, H., et al. (2017). Establishment of a repeated social defeat stress model in female mice. Sci. Rep. 7:12838. doi: 10.1038/s41598-017-12811-8
Tao, L., and Reese, T. A. (2017). making mouse models that reflect human immune responses. Trends Immunol. 38, 181–193. doi: 10.1016/j.it.2016.12.007
Thase, M. E., Entsuah, R., Cantillon, M., and Kornstein, S. G. (2005). Relative antidepressant efficacy of venlafaxine and SSRIs: sex-age interactions. J. Womens Health (Larchmt) 14, 609–616. doi: 10.1089/jwh.2005.14.609
Tonelli, L. H., Holmes, A., and Postolache, T. T. (2008). Intranasal immune challenge induces sex-dependent depressive-like behavior and cytokine expression in the brain. Neuropsychopharmacology 33, 1038–1048. doi: 10.1038/sj.npp.1301488
Toniolo, A., Fadini, G. P., Tedesco, S., Cappellari, R., Vegeto, E., Maggi, A., et al. (2015). Alternative activation of human macrophages is rescued by estrogen treatment in vitro and impaired by menopausal status. J. Clin. Endocrinol. Metab. 100, E50–E58. doi: 10.1210/jc.2014-2751
Trivedi, M. H., Rush, A. J., Wisniewski, S. R., Nierenberg, A. A., Warden, D., Ritz, L., et al. (2006). Evaluation of outcomes with citalopram for depression using measurement-based care in STAR*D: implications for clinical practice. Am. J. Psychiatry 163, 28–40. doi: 10.1176/appi.ajp.163.1.28
Uppal, S. S., Verma, S., and Dhot, P. S. (2003). Normal values of CD4 and CD8 lymphocyte subsets in healthy indian adults and the effects of sex, age, ethnicity, and smoking. Cytometry B Clin. Cytom. 52, 32–36. doi: 10.1002/cyto.b.10011
Vélez-Ortega, A. C., Temprano, J., Reneer, M. C., Ellis, G. I., McCool, A., Gardner, T., et al. (2013). Enhanced generation of suppressor T cells in patients with asthma taking oral contraceptives. J. Asthma 50, 223–230. doi: 10.3109/02770903.2012.761231
Verthelyi, D. (2001). Sex hormones as immunomodulators in health and disease. Int. Immunopharmacol. 1, 983–993. doi: 10.1016/s1567-5769(01)00044-3
Verthelyi, D. I., and Ahmed, S. A. (1998). Estrogen increases the number of plasma cells and enhances their autoantibody production in nonautoimmune C57BL/6 mice. Cell Immunol. 189, 125–134. doi: 10.1006/cimm.1998.1372
Vetrano, M., Wegman, A., Koes, B., Mehta, S., and King, C. A. (2020). Serum IL-1RA levels increase from follicular to luteal phase of the ovarian cycle: a pilot study on human female immune responses. PLoS One 15:e0238520. doi: 10.1371/journal.pone.0238520
Villa, A., Rizzi, N., Vegeto, E., Ciana, P., and Maggi, A. (2015). Estrogen accelerates the resolution of inflammation in macrophagic cells. Sci. Rep. 5:15224. doi: 10.1038/srep15224
Vink, N. M., Postma, D. S., Schouten, J. P., Rosmalen, J. G., and Boezen, H. M. (2010). Gender differences in asthma development and remission during transition through puberty: the TRacking Adolescents’ Individual Lives Survey (TRAILS) study. J. Allergy Clin. Immunol. 126, 498–504.e1–6. doi: 10.1016/j.jaci.2010.06.018
Vom Steeg, L. G., Flores-Garcia, Y., Zavala, F., and Klein, S. L. (2019). Irradiated sporozoite vaccination induces sex-specific immune responses and protection against malaria in mice. Vaccine 37, 4468–4476. doi: 10.1016/j.vaccine.2019.06.075
Vrachnis, N., Zygouris, D., Vrachnis, D., Antonakopoulos, N., Fotiou, A., Panagopoulos, P., et al. (2021). Effects of hormone therapy and flavonoids capable on reversal of menopausal immune senescence. Nutrients 13:2363. doi: 10.3390/nu13072363
Wagar, L. E., DiFazio, R. M., and Davis, M. M. (2018). Advanced model systems and tools for basic and translational human immunology. Genome Med. 10:73. doi: 10.1186/s13073-018-0584-8
Waitzfelder, B., Stewart, C., Coleman, K. J., Rossom, R., Ahmedani, B. K., Beck, A., et al. (2018). Treatment initiation for new episodes of depression in primary care settings. J. Gen. Intern. Med. 33, 1283–1291. doi: 10.1007/s11606-017-4297-2
Walf, A. A., and Frye, C. A. (2009). Effects of two estradiol regimens on anxiety and depressive behaviors and trophic effects in peripheral tissues in a rodent model. Gend. Med. 6, 300–311. doi: 10.1016/j.genm.2009.04.004
Walker, S. E. (2011). Estrogen and autoimmune disease. Clin. Rev Allergy Immunol. 40, 60–65. doi: 10.1007/s12016-010-8199-x
Wang, L., Jiang, P., Zhao, S., Liu, H., Liu, L., Mor, G., et al. (2021). The dynamic profile and potential function of B-cell subsets during pregnancy. Cell Mol. Immunol. 18, 1082–1084. doi: 10.1038/s41423-020-00535-1
Wang, Q., Timberlake, M. A. II, Prall, K., and Dwivedi, Y. (2017). The recent progress in animal models of depression. Prog. Neuropsychopharmacol. Biol. Psychiatry 77, 99–109. doi: 10.1016/j.pnpbp.2017.04.008
Wang, Y. T., Wang, X. L., Feng, S. T., Chen, N. H., Wang, Z. Z., and Zhang, Y. (2021). Novel rapid-acting glutamatergic modulators: targeting the synaptic plasticity in depression. Pharmacol. Res. 171:105761. doi: 10.1016/j.phrs.2021.105761
Wegienka, G., Bobbitt, K. R., Woodcroft, K. J., and Havstad, S. (2011). Regulatory T cells vary over bleeding segments in asthmatic and non-asthmatic women. J. Reprod. Immunol. 89, 192–198. doi: 10.1016/j.jri.2011.03.002
Weinberg, A., Enomoto, L., Marcus, R., and Canniff, J. (2011). Effect of menstrual cycle variation in female sex hormones on cellular immunity and regulation. J. Reprod. Immunol. 89, 70–77. doi: 10.1016/j.jri.2010.11.009
Weissman, M. M., and Klerman, G. L. (1977). Sex differences and the epidemiology of depression. Arch. Gen. Psychiatry 34, 98–111. doi: 10.1001/archpsyc.1977.01770130100011
Weyand, C. M., and Goronzy, J. J. (2016). Aging of the immune system. mechanisms and therapeutic targets. Ann. Am. Thorac. Soc. 13 Suppl 5(Suppl 5), S422–S428. doi: 10.1513/AnnalsATS.201602-095AW
Wharton, W., Gleason, C. E., Olson, S. R., Carlsson, C. M., and Asthana, S. (2012). Neurobiological underpinnings of the estrogen – mood relationship. Curr. Psychiatry Rev. 8, 247–256. doi: 10.2174/157340012800792957
Willner, P. (2017). The chronic mild stress (CMS) model of depression: history, evaluation and usage. Neurobiol. Stress 6, 78–93. doi: 10.1016/j.ynstr.2016.08.002
Wohleb, E. S., Franklin, T., Iwata, M., and Duman, R. S. (2016). Integrating neuroimmune systems in the neurobiology of depression. Nat. Rev. Neurosci. 17, 497–511. doi: 10.1038/nrn.2016.69
Woitowich, N. C., and Woodruff, T. K. (2019). Opinion: research community needs to better appreciate the value of sex-based research. Proc. Natl. Acad. Sci. U.S.A. 116, 7154–7156. doi: 10.1073/pnas.1903586116
Xu, W., Wong, G., Hwang, Y. Y., and Larbi, A. (2020). The untwining of immunosenescence and aging. Semin. Immunopathol. 42, 559–572. doi: 10.1007/s00281-020-00824-x
Yang, J. H., Chen, C. D., Wu, M. Y., Chao, K. H., Yang, Y. S., and Ho, H. N. (2000). Hormone replacement therapy reverses the decrease in natural killer cytotoxicity but does not reverse the decreases in the T-cell subpopulation or interferon-gamma production in postmenopausal women. Fertil Steril 74, 261–267. doi: 10.1016/s0015-0282(00)00622-1
Yang, T., Nie, Z., Shu, H., Kuang, Y., Chen, X., Cheng, J., et al. (2020). The role of BDNF on neural plasticity in depression. Front. Cell Neurosci. 14:82. doi: 10.3389/fncel.2020.00082
Yin, D., Tuthill, D., Mufson, R. A., and Shi, Y. (2000). Chronic restraint stress promotes lymphocyte apoptosis by modulating CD95 expression. J. Exp. Med. 191, 1423–1428. doi: 10.1084/jem.191.8.1423
Zainal, N. H., and Newman, M. G. (2021). Increased inflammation predicts nine-year change in major depressive disorder diagnostic status. J. Abnorm Psychol. 130, 829–840. doi: 10.1037/abn0000716
Zhang, T., Dong, Z., Cai, H., Rong, J., and Su, Z. (2020). Estradiol regulates the expression of CD45 splicing isoforms in lymphocytes. Mol. Biol. Rep. 47, 3025–3030. doi: 10.1007/s11033-020-05373-y
Zhao, W., Li, Q., Ma, Y., Wang, Z., Fan, B., Zhai, X., et al. (2021). Behaviors related to psychiatric disorders and pain perception in C57BL/6J mice during different phases of estrous cycle. Front. Neurosci. 15:650793. doi: 10.3389/fnins.2021.650793
Zimmerman, M., Ellison, W., Young, D., Chelminski, I., and Dalrymple, K. (2015). How many different ways do patients meet the diagnostic criteria for major depressive disorder? Compr Psychiatry 56, 29–34. doi: 10.1016/j.comppsych.2014.09.007
Keywords: estrogen, sex differences, major depressive disorder, peripheral immune system, mood
Citation: Engler-Chiurazzi EB, Chastain WH, Citron KK, Lambert LE, Kikkeri DN and Shrestha SS (2022) Estrogen, the Peripheral Immune System and Major Depression – A Reproductive Lifespan Perspective. Front. Behav. Neurosci. 16:850623. doi: 10.3389/fnbeh.2022.850623
Received: 07 January 2022; Accepted: 17 March 2022;
Published: 15 April 2022.
Edited by:
Stephanie V. Koebele, Arizona State University, United StatesReviewed by:
Benedetta Leuner, The Ohio State University, United StatesAngela Garcia, Arizona State University, United States
Copyright © 2022 Engler-Chiurazzi, Chastain, Citron, Lambert, Kikkeri and Shrestha. This is an open-access article distributed under the terms of the Creative Commons Attribution License (CC BY). The use, distribution or reproduction in other forums is permitted, provided the original author(s) and the copyright owner(s) are credited and that the original publication in this journal is cited, in accordance with accepted academic practice. No use, distribution or reproduction is permitted which does not comply with these terms.
*Correspondence: Elizabeth B. Engler-Chiurazzi, ZWVuZ2xlcmNoaXVyYXp6aUB0dWxhbmUuZWR1
†These authors have contributed equally to this work