- 1Provincial-Level Key Laboratory for Molecular Medicine of Major Diseases and the Prevention and Treatment with Traditional Chinese Medicine Research in Gansu Colleges and University, Gansu University of Chinese Medicine, Lanzhou, China
- 2College of Basic Medicine, Gansu University of Chinese Medicine, Lanzhou, China
- 3Key Laboratory for Transfer of Dunhuang Medicine at the Provincial and Ministerial Level, Gansu University of Traditional Chinese Medicine, Lanzhou, China
- 4College of Public Health, Gansu University of Chinese Medicine, Lanzhou, China
Both sensory and metabolic processes guide food intake. Olfactory inputs help coordinate food appreciation and selection, but their role in food consumption and post-feeding physiology remains poorly understood. In this study, using Drosophila melanogaster as a model system, we investigated the effects of olfactory sensory neurons (OSNs) on food consumption, metabolism, and stress responses. We found that dysfunction of OSNs affects diverse processes, including decreased food consumption, increased triacylglycerol level, enhanced stress resistance to starvation or desiccation, and decreased cold resistance. Decreased neuropeptide F receptor (NPFR) level or increased insulin activity in OSNs inhibited food consumption, while impaired NPF signaling or insulin signaling in OSNs increased resistance to starvation and desiccation. These studies provide insights into the function of the olfactory system in control of feeding behaviors and physiology.
Introduction
The regulation of energy homeostasis depends on a dynamic balance between food consumption and energy expenditure (Casanova et al., 2019). This dynamic balance requires the coordinated integration of the peripheral sensory system inputs with internal response systems. The sensory system directs animals toward food sources, guides preferences and satiety experience after consumption, and promotes dietary learning (McCrickerd and Forde, 2016). Animals must weigh both aversive and attractive sensory inputs to evaluate potential food sources. The sensory system undergoes dramatic functional modifications when animals enter different internal states, such as hunger or fullness (Ko et al., 2015). It is important to reveal the mechanisms by which sensory systems, such as the olfactory system and influence feeding.
Olfaction is an ancient sensory system present in animals ranging from Drosophila to man. Odorant receptors (ORs) expressed in olfactory sensory neurons (OSNs) can detect and distinguish different olfactory cues (Touhara and Vosshall, 2009). Some OSNs initiate an attractive response to odors, while others mediate the aversive response to odors (Semmelhack and Wang, 2009). Sensitive and accurate detection of food-related odors via the olfactory system can guide animals to sources of energy and nutrition. Food-related odors stimulate appetite responses in animals. For instance, the rating of hunger is increased following exposure to food-related odors (Rogers and Hill, 1989). Food-related odors significantly stimulate salivation, insulin release, and gastric acid secretion (Yeomans, 2006). Food odorants also drive animals to consume more food (Reisenman and Scott, 2019). However, the role of the specific OSNs in food intake remains unclear.
The perceptions of olfactory stimuli and behavioral decisions are influenced by internal states. Fasted animals have greater olfactory sensitivity and more active olfactory-driven behaviors than satiated ones (Albrecht et al., 2009; Soria-Gomez et al., 2014), which is due to the decreased secretion of anorexigenic molecules (e.g., insulin, cholecystokinin, and leptin) and increased secretion of orexigenic molecules (e.g., neuropeptide, orexins, and ghrelin) (Soria-Gomez et al., 2014; Peng et al., 2019). Starvation leads to increased behavioral attraction to appetite odors and blunts behavioral avoidance to averse odors (Root et al., 2011; Bracker et al., 2013). The initiation and cessation of feeding behaviors are associated with changes in olfactory sensitivity. Olfactory sensitivity is decreased after the ingestion of food, which is regulated by the satiety signal (Savigner et al., 2009). Insulin receptors are expressed in a subset of OSNs in Drosophila flies and vertebrates (Huang et al., 2019; Slankster et al., 2020). In hungry flies, the lower insulin signaling frees production of the short neuropeptide F receptor (sNPFR) to increase sNPF signaling, enhancing the response to odors (Root et al., 2011). In addition, the olfactory system regulates energy homeostasis. Loss of adult OSNs protects against diet-induced obesity in mice, while ablation of IGF1 signaling in OSNs leads to increased adiposity and insulin resistance (Riera et al., 2017).
To further dissect the interplay between the olfactory system and food intake or metabolism, adult flies were used in these studies. Drosophila is an excellent model animal for unraveling odor-associated behaviors and physiology, as well as the signaling pathways that mediate feeding and metabolism (Graham and Pick, 2017; Koyama et al., 2020). Our studies reveal that dysfunctional OSNs significantly reduced food intake, increased TAG level, and increased the resistance to starvation and desiccation. We show that NPFR signaling and dInR signaling in OSNs affect the food intake and the stress response. Overall, these findings suggest that specific sets of odor channels, which utilize NPFR signaling and dInR signaling in a gain control mechanism, regulate food intake and the stress response in flies, thereby affecting metabolism.
Materials and Methods
Fly Strains and Maintenance
Flies were reared on standard cornmeal dextrose agar food at 25°C, 65% humidity, and 12/12 light/dark cycle. The following fly stocks were obtained from the Bloomington Drosophila stock center (BDSC; IN, United States): w1118 (#5905), orco1 (#23129), Orco-Gal4 (#23292), Orco-Gal4 (#26818), Or42b-Gal4 (#9971), Or85a-Gal4 (#23133), UAS-TNT (#28838), UAS-Orco (#23145), UAS-InRCA (#8263), UAS-InRDN (#8251), UAS-NPFR RNAi (#25939), and UAS-mCD8:GFP (#5137). The strains were backcrossed for at least five generations with w1118 to isogenize the genetic background. Adult female flies (3–5 days old) were collected using light CO2 anesthesia and allowed to recover for 2 days before further experimentation.
Food Consumption
The amount of food eaten by single flies was measured using the Capillary Feeder (CAFE) assay (He et al., 2021). Briefly, eight female flies were distributed into each vial with 1% agar at the bottom. Four 5 μl glass capillaries (BRAND, Germany) were inserted into the vial through the lid. The capillaries were filled with 5% sucrose, 5% yeast extract, or both. For the non-starved condition, the amount of liquid food in the capillaries was measured for 24 h. For the starved condition, flies were starved for 21 h before measuring the amount of liquid food for 3 h. One parallel vial void of flies was used as control to determine the extent of liquid evaporation from the capillaries. The total food intake (μl/fly) was calculated as (food consumption − evaporation loss)/number of flies.
Stress Resistance Assay
Twenty female flies per vial were used to measure the resistance to starvation and desiccation. Replicates (9–10) were set up for each group. Flies were placed in empty vials with 1% agar for starvation resistance and without 1% agar for desiccation resistance. All vials were incubated under constant conditions (25°C, 65% humidity, and 12/12 light/dark cycle). Dead flies were counted every 6–12 h until all flies had died. For resistance to cold stress, flies were placed in empty vials and subjected to cold treatment at 0°C for 4 h, and then, the number of recovered flies was counted every 2 min in a 22°C room.
Triacylglycerol Assay
The level of triacylglycerol (TAG) was measured as described previously (Tennessen et al., 2014). Briefly, 5 female adult flies per vial were weighed and frozen at −80°C until assay. After homogenizing in PBS + 1% Triton-X, the samples were heated at 70°C for 10 min and then cooled to room temperature. An aliquot was added to the triglyceride reagent (T2449; Sigma, United States), incubated at 37°C for 60 min, added to the free glycerol reagent (F6428; Sigma, United States), and incubated for 5 min at 37°C. TAG content was quantified using a microplate spectrophotometer at OD540 using a standard curve and blank well.
Statistical Analysis
The data are expressed as mean ± standard error of the mean (S.E.M). Statistical analysis was performed with Prism 7.03 (GraphPad Software, United States). The differences between two groups or among the three groups were examined using Student’s t-test or one-way ANOVA followed by Fisher’s protected least significant difference (LSD) test. Survivorships among groups were compared and tested for significance with a Log-rank (Mantel-Cox) test. Differences among different groups were considered to be statistically significant at *p < 0.05, **p < 0.01, and ***p < 0.001.
Results
Olfactory Sensory Neurons Are Necessary to Regulate Food Intake in Non-starved Flies
Olfaction is known to play a major role in food choice. Olfactory sensitivity depends on the internal states, in which starvation encourages animals to search for food via increasing olfactory sensitivity (Stafford and Welbeck, 2011; Ko et al., 2015). We wondered whether OSNs regulate the amount of food intake in adult flies. The simple CAFE assay was used to measure food consumption in flies where olfactory signaling was modified (Figure 1A). The results show that total food consumption (5% sucrose + 5% yeast extract) was significantly decreased in Orco1 mutant flies (Figure 1B) in the non-starved state. Odorant co-receptor (Orco) interacts with the odor-specific receptor and boosts the functional expression of odor-specific receptors in OSNs. We used the UAS-Gal4 system to block chemical synaptic transmission in OSNs by expressing tetanus toxin light chain (TNT). Orco-Gal4/UAS-TNT adult flies eat significantly less compared to control flies (Figure 1C). These results indicated that blocking OSNs inhibits food consumption. To confirm that the decreased food intake is indeed due to loss of OSNs function in flies, we restored Orco function in Orco1 mutants using the Orco-Gal4 driver (Figure 1D). Expression of Orco in OSNs restored food intake in Orco1 mutants. To eliminate the development effect of OSNs, OSNs were acutely activated by expressing TrpA1 at 30°C during testing. Orco-Gal4/UAS-TrpA1 adult flies consumed remarkably more food compared to control flies at 30°C, while there was no difference between control flies and experimental flies at 30°C (Supplementary Figure 1). This indicates that acute activation of OSNs promotes food intake. To eliminate the influence of yeast extract odors in food, food was replaced with 5% sucrose or 5% yeast extract (Figures 1E,F). Orco1 mutants also consumed less food than w1118 flies. Next, nutrient preference was detected. w1118 flies and Orco1 mutants displayed a significant preference for food containing 5% sucrose and 5% yeast extract, compared to food with 5% sucrose (Supplementary Figure 2). However, Orco1 mutants had a similar preference as w1118 flies, which suggests that alternation of OSNs plays no role in the regulation of nutrient choice. Taken together, these findings indicate that blocking OSNs inhibits the amount of food consumed in a non-starved state.
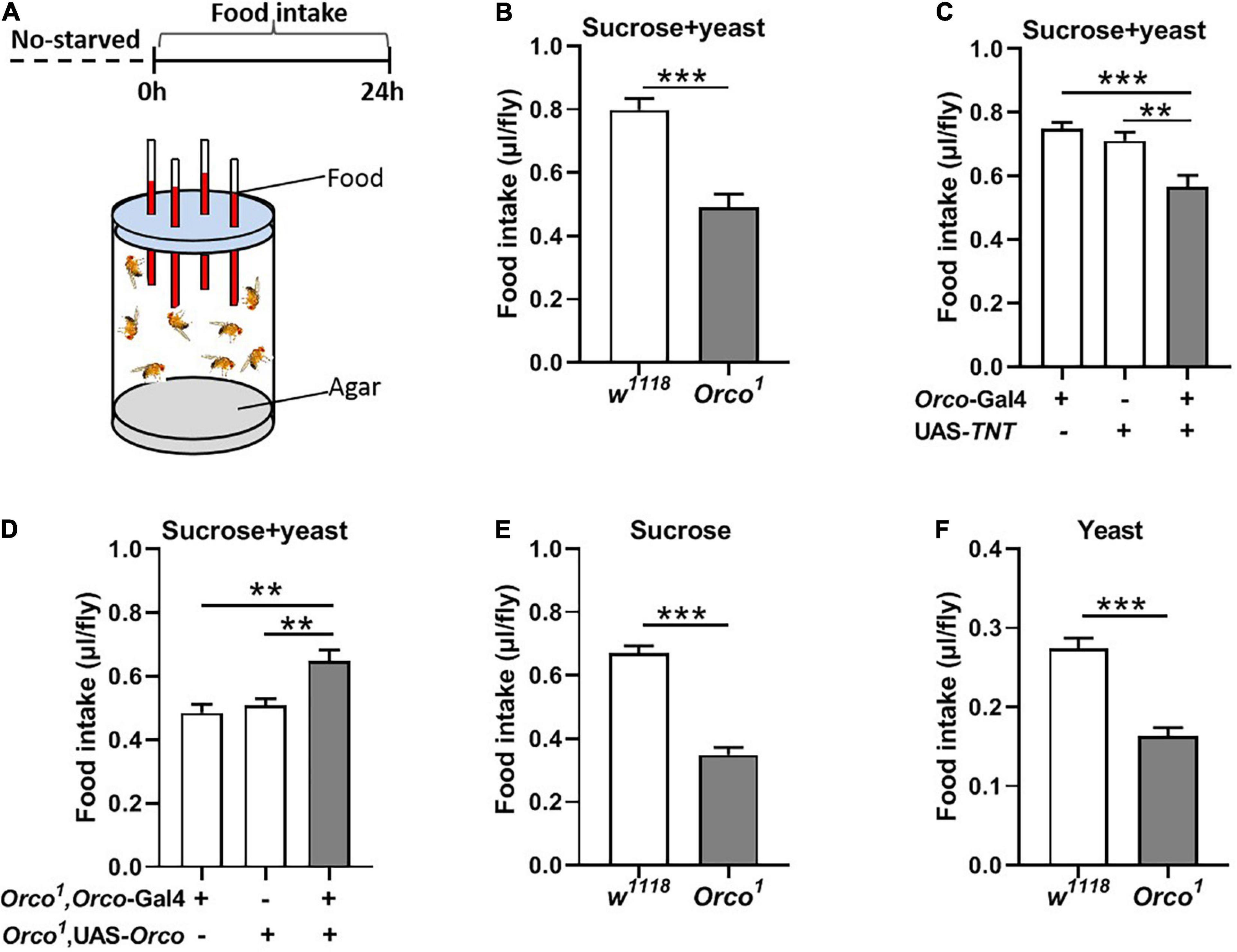
Figure 1. Blocking OSNs decreases the food consumption in non-starved flies. (A) Schematic diagram of the CAFE assay for examining food intake in non-starved flies. (B) Orco1 mutant flies consumed less sucrose and yeast extract food than control w1118 flies (n = 15). (C) Food intake was decreased after blocking OSNs by expressing TNT in Orco-GAL4 driver (n = 21). (D) Expression of Orco in the OSNs in Orco1 mutants rescued food (5% sucrose and 5% yeast extract) intake (n = 15). Orco1 mutant flies consumed less single sucrose food (n = 13) (E) and yeast extract food (n = 17) (F). Data are represented as mean ± S.E.M. One-sample t-test and one-way ANOVA followed by Dunnett’s post hoc test were used to analyze the difference. **p < 0.01, and ***p < 0.001.
Blocking Olfactory Sensory Neurons Decreases Food Intake in Starved Flies
To further explore the function of OSNs in food consumption, we measured food intake in starved flies (Figure 2A). After 21 h starvation, Orco1 mutant flies consumed less food (15% sucrose and 15% yeast extract) than w1118 flies (Figure 2B, p < 0.001). Blocking OSNs by driving the expression of UAS-TNT resulted in decreased food consumption compared to corresponding controls (Figure 2C, p < 0.01). Restored Orco expression in Orco1 mutants using the Orco-Gal4 driver restored food consumption in Orco1 mutants (Figure 2D), indicating that the decreased food consumption was due to loss of orco-related OSNs function. When the food was replaced by single 5% sucrose or 5% yeast extract, starved Orco1 mutants still consumed less food than w1118 control flies (Figures 2E,F). Restored Orco expression in Orco1 mutants using the Orco-Gal4 driver restored food consumption in Orco1 mutants (Figure 3D), indicating that the decreased food consumption was due to loss of orco-related OSNs function. When the food was replaced by single 5% sucrose or 5% yeast extract, starved Orco1 mutants still consumed less food than w1118 control flies, indicating that the odor of yeast extract was not the reason for altered food consumption. Thus, these results suggest that blocking OSNs inhibits the amount of food consumed in the starved state.
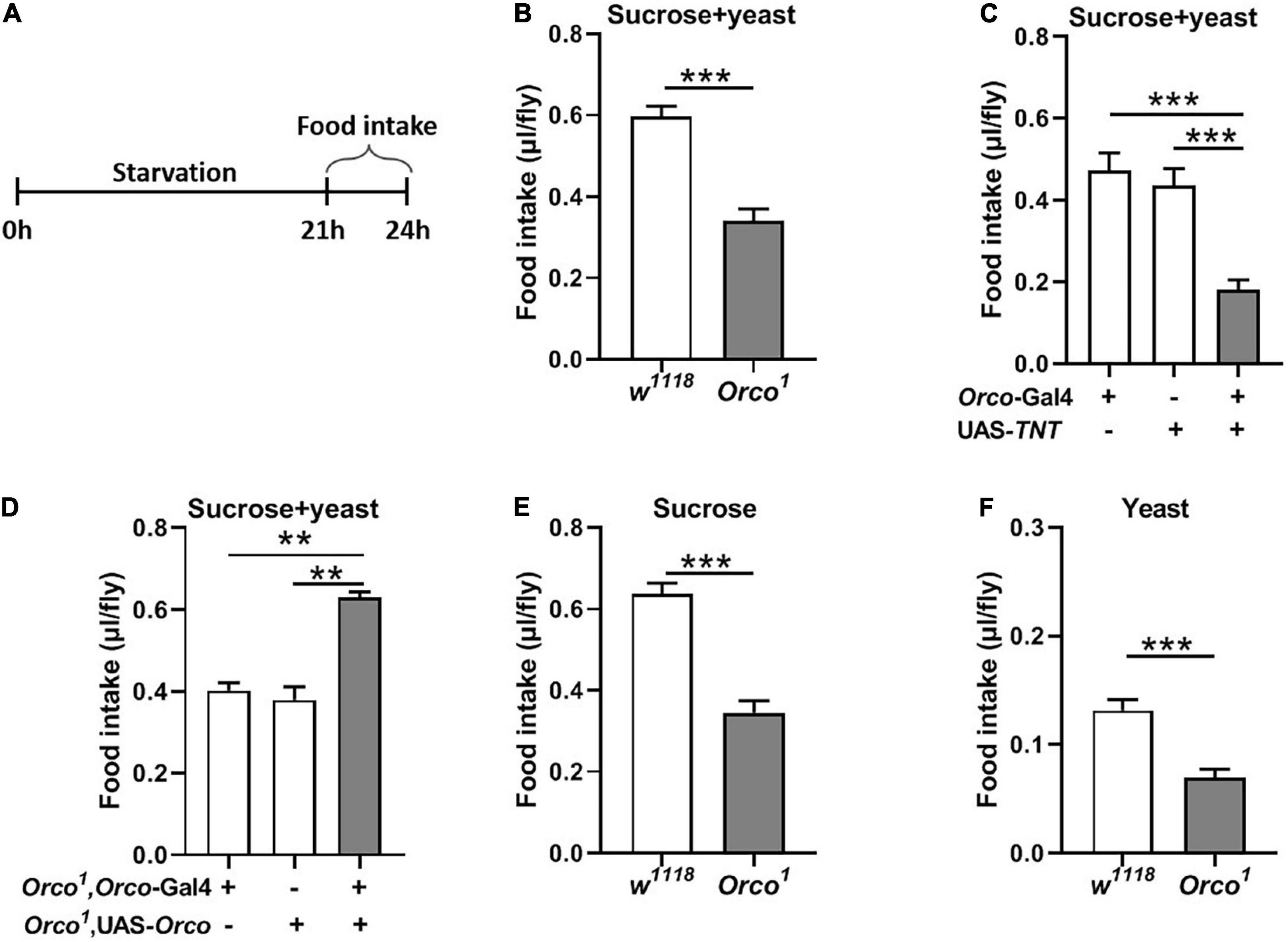
Figure 2. Blocking OSNs decreases the food consumption in starved flies. (A) Schematic for food intake of starved flies. (B) After 21 h starvation, Orco1 mutant flies consumed less liquid food containing 5% sucrose and 5% yeast extract than control w1118 flies (n = 12). (C) Expression TNT in OSNs decreased food consumption (n = 12). (D) Expression of Orco in OSNs of Orco1 mutants flies rescued food intake (n = 15). Starved Orco1 mutant flies consumed less single sucrose food (n = 19–20) (E) or yeast extract food (n = 12) (F). **p < 0.01 and ***p < 0.001.
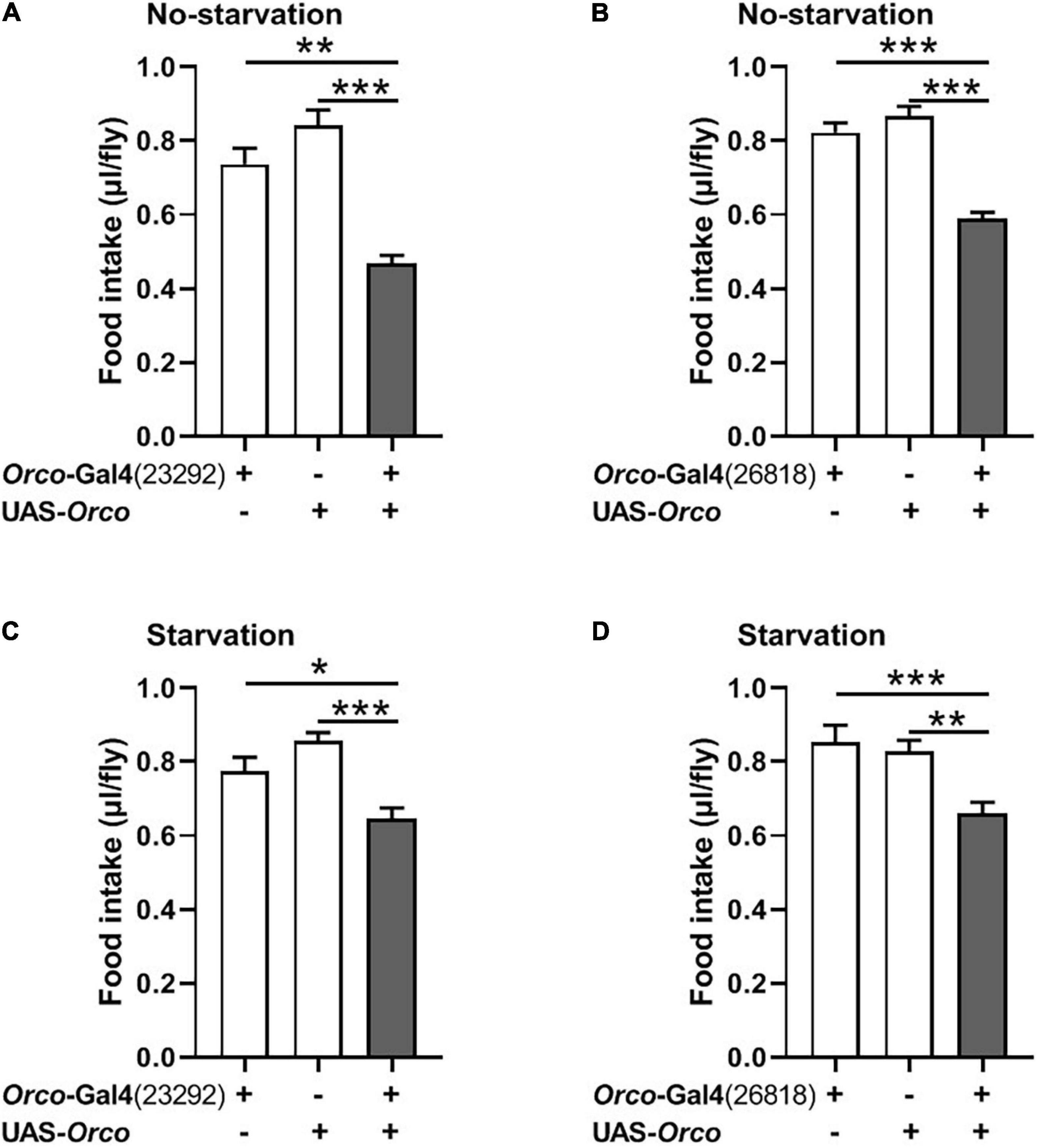
Figure 3. Overexpressing orco in OSNs decreases food consumption. Flies expressing orco in both Orco-GAL4 drive (#23292 and #26818) eaten less food in the non-starved state (A,B) and starved state (C,D), n = 15–21. One-way ANOVA followed by Dunnett’s post hoc test was used to analyze the difference. **p < 0.01 and ***p < 0.001.
Overexpression of Orco in Olfactory Sensory Neurons Inhibits the Food Intake
We next asked whether overexpression of Orco in OSNs would promote food consumption in flies. Expression of Orco in OSNs using the Orco-Gal4 driver (#23292) significantly reduced food intake in non-starved flies (Figure 3A). The same phenotype was found when using another Orco-Gal4 driver (#26818) to overexpress orco in flies (Figure 3B). In addition, after 21 h of starvation, expressing Orco in OSNs remarkably decreased food consumption (Figures 3C,D). Therefore, overexpressing Orco in OSNs leads to decreased food consumption.
Or85a and Or42b OSNs Have No Function in the Regulation of Food Consumption
Previous studies in food search have shown that activation of the DM5 glomerulus, innervated by Or85a OSNs, triggers aversion to odors, while activation of the DM1 glomerulus, innervated by Or42b OSNs, regulates attraction to odors in flies (Semmelhack and Wang, 2009; Ko et al., 2015). Therefore, we next investigated whether modulation of Or85a or Or42b OSN signaling would alter food intake in flies. Visualizing the Gal4 expression domain of the Or85a-Gal4 line using a UAS-mCD8:GFP transgene, Or85a OSNs projected into the DM5 glomerulus (Figure 4A). Blocking Or85a OSNs by expressing TNT in flies did not affect the food consumption (Figure 4B). In addition, we found that the DM1 glomerulus was innervated by Or42b OSNs (Figure 4C). When Or42b OSNs were blocked by expressing TNT, the food consumption was not affected in flies (Figure 4D). Therefore, these results suggest that Or85a and Or42b OSNs play no role in the regulation of food intake.
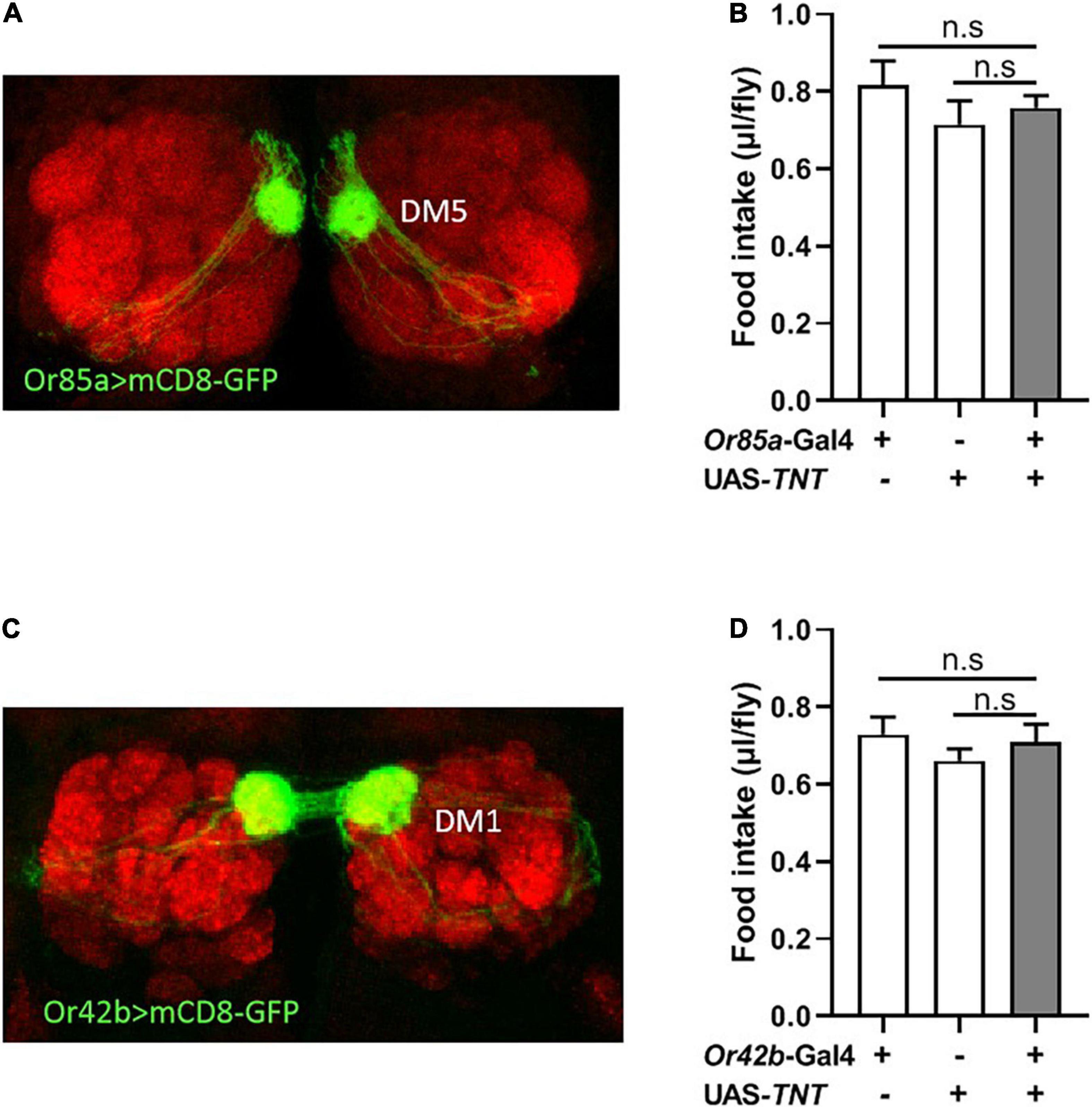
Figure 4. Or85a OSNs regulates food consumption. (A) Expression of Or85a OSNs in DM5 of antennal lobe. Or85a-GAL4 drives UAS-mCD8-GFP expression (Green). (B) The food consumption was not affect after expression TNT in Or85a OSNs (n = 21). (C) Expression of Or42b OSNs in DM1 of antennal lobe. Or42b-GAL4 drives UAS-mCD8-GFP expression (Green). (D) No effect on food intake after expression TNT in Or42b OSNs (n = 15). One-way ANOVA followed by Dunnett’s post-hoc test were used to analyze the difference.
Insulin and NPF Signaling in Olfactory Sensory Neurons Participate in Food Consumption
InR expression and NPFR expression have been clearly demonstrated at terminals of adult fly OSNs (Lee et al., 2017; Slankster et al., 2020). Previous studies found that insulin signaling plays important roles during both starvation and olfactory behavior in flies and mammals (Enell et al., 2010; Slankster et al., 2020). NPF enhances the olfactory sensitivity of OSNs and increases appetite behavior in flies (Wang et al., 2013; Lee et al., 2017). We, therefore, investigated the role of insulin and NPF signaling in OSNs on food consumption. We used the UAS-Gal4 system to manipulate InR and NPFR levels in OSNs. InR levels were increased or reduced in OSNs by driving a UAS-InRCA or a UAS-InRDN. Increasing InR activity in OSNs resulted in decreased food consumption compared to corresponding controls (Figure 5A). Decreased InR level did not affect consumption in flies (Figure 5B). Next, NPFR levels were reduced in OSNs by driving a UAS-NPFR-RNAi. Reducing NPFR levels in OSNs remarkably decreased food consumption (Figure 5C). These results suggest that insulin and NPF signaling in OSNs have an important role in the regulation of food consumption in flies.
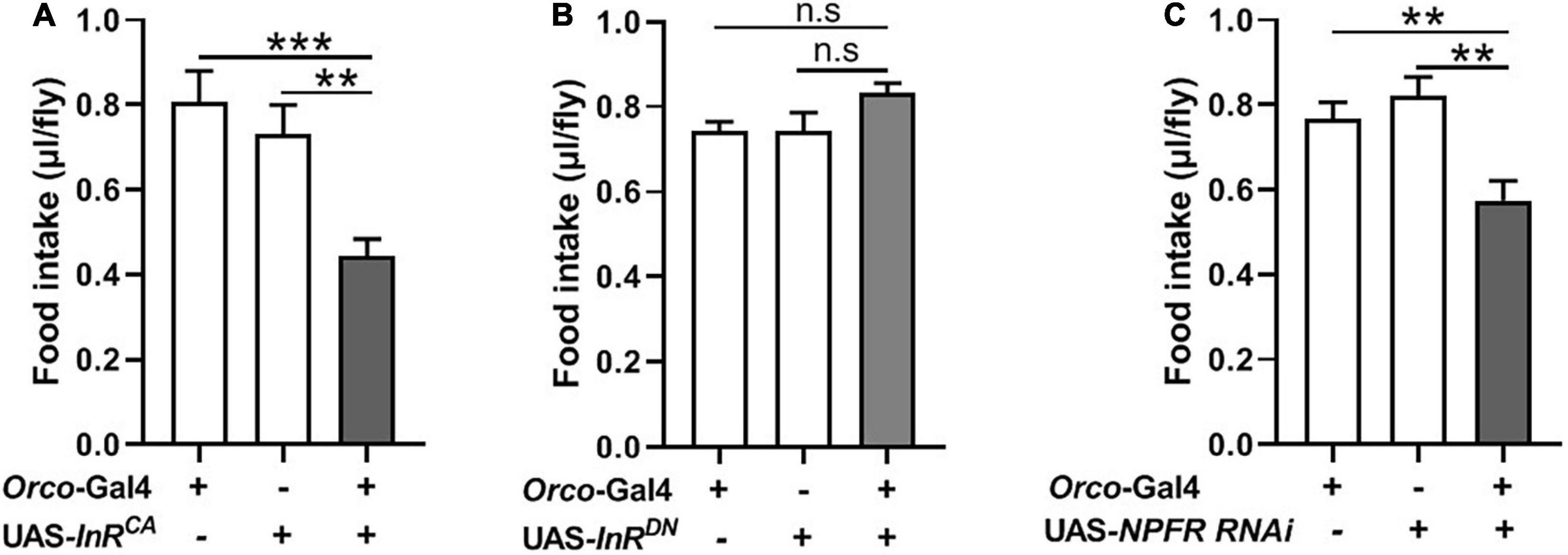
Figure 5. Modulating expression of InR and NPFR in OSNs affect food intake. (A) Increasing InR activity in OSNs by driving a UAS-InRCA decreased food consumption (n = 20). (B) No effect on food intake after decreasing InR activity (n = 12). (C) Decreasing NPFR expression in OSNs inhibited food intake (n = 18). One-way ANOVA followed by Dunnett’s post-hoc test were used to analyze the difference. **p < 0.01 and ***p < 0.001.
Abnormal Olfactory Sensory Neurons Signaling Enhances Starvation Resistance
Given that manipulation of OSNs signaling can modulate food intake in flies, we next tested whether they also affect starvation resistance and lipid metabolism. Orco1 mutants exhibited a significant increase in survival rate compared with w1118 flies (Figure 6A). Blocking OSNs signaling also extended survival time (Figure 6B). Unexpectedly, overexpression of Orco in OSNs remarkably increased the survival rate (Figure 6C). Reducing InR levels in OSNs resulted in an increased survival rate compared to corresponding controls (Figure 6D). Decreasing NPFR levels in OSNs also increased the survival rate under starvation conditions (Figure 6E). These results suggest that abnormal OSNs signaling in flies leads to increased resistance to starvation, which is related to insulin and NPF signaling. The observation of enhanced starvation resistance further prompted us to investigate whether body fat deposition was affected by OSNs. TAG levels in Orco1 mutants were significantly elevated when compared to the w1118 flies (Figure 6F). Flies in which orco was overexpressed in OSNs had similar TAG levels with flies having normal OSNs function (Figure 6G). Reducing InR levels in OSNs slightly increased the TAG levels, but the difference was not significant (Figure 6H). Therefore, altering OSNs signaling or reducing InR levels in OSNs can enhance the resistance to starvation, likely by modulating lipid metabolism.
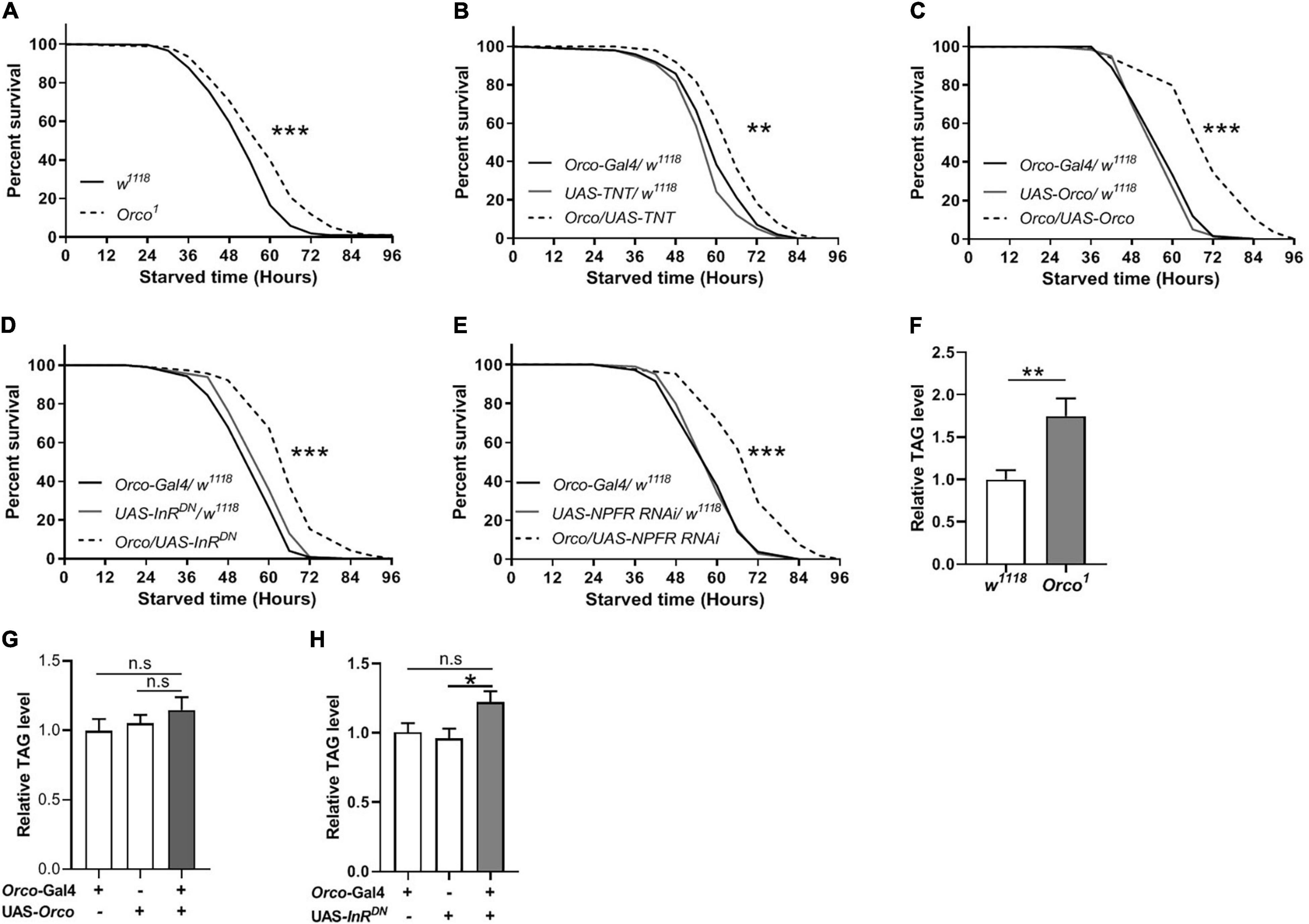
Figure 6. OSNs regulate starvation resistance and lipid metabolism. (A) Orco1 mutant flies had longer survival lifespan under starved condition than w1118 flies (n = 9 vials/group). (B) Blocking OSNs by expressing TNT enhanced the starvation resistance (n = 9 vials/group). The resistance to starvation was increased after expressing Orco in OSNs (C) (n = 11–12). Decreasing InR activity (D) or decreasing NPFR level (E) in OSNs extended the survival lifespan under starved condition (n = 10). (F) Orco1 mutant flies had higher triacylglycerol (TAG) level than w1118 flies (n = 9). The level of TAG did not affect after expressing Orco (G) and decreasing InR activity (H) (n = 9–10). *p < 0.05, **p < 0.01, and ***p < 0.001.
Abnormal Olfactory Sensory Neurons Signaling Alters Desiccation and Cold Resistance
To further establish a role for OSNs in the regulation of stress resistance, we investigated whether disorder in olfactory function is sufficient to alter the stress response to desiccation. Compared with w1118 flies, Orco1 mutants had a higher survival rate in desiccated conditions (Figure 7A). Flies in which OSNs were blocked lived longer than their parental controls in desiccated conditions (Figure 7B). Expressing Orco in OSNs significantly extended the survival time (Figure 7C). When InR or NPFR signaling was reduced in OSNs, flies showed an increased survival rate (Figures 7D,E). In addition, the stress response to cold was detected in flies. Orco1 mutants took more time to recover after cold stress as compared to w1118 flies (Figure 7F). Blocking OSNs by expressing TNT in flies also extended the recovery time after 4 h of cold stress (Figure 7G). Therefore, altering OSNs signaling or reducing InR levels in OSNs enhances the resistance to desiccant, and blocking OSNs decreases the resistance to cold stress.
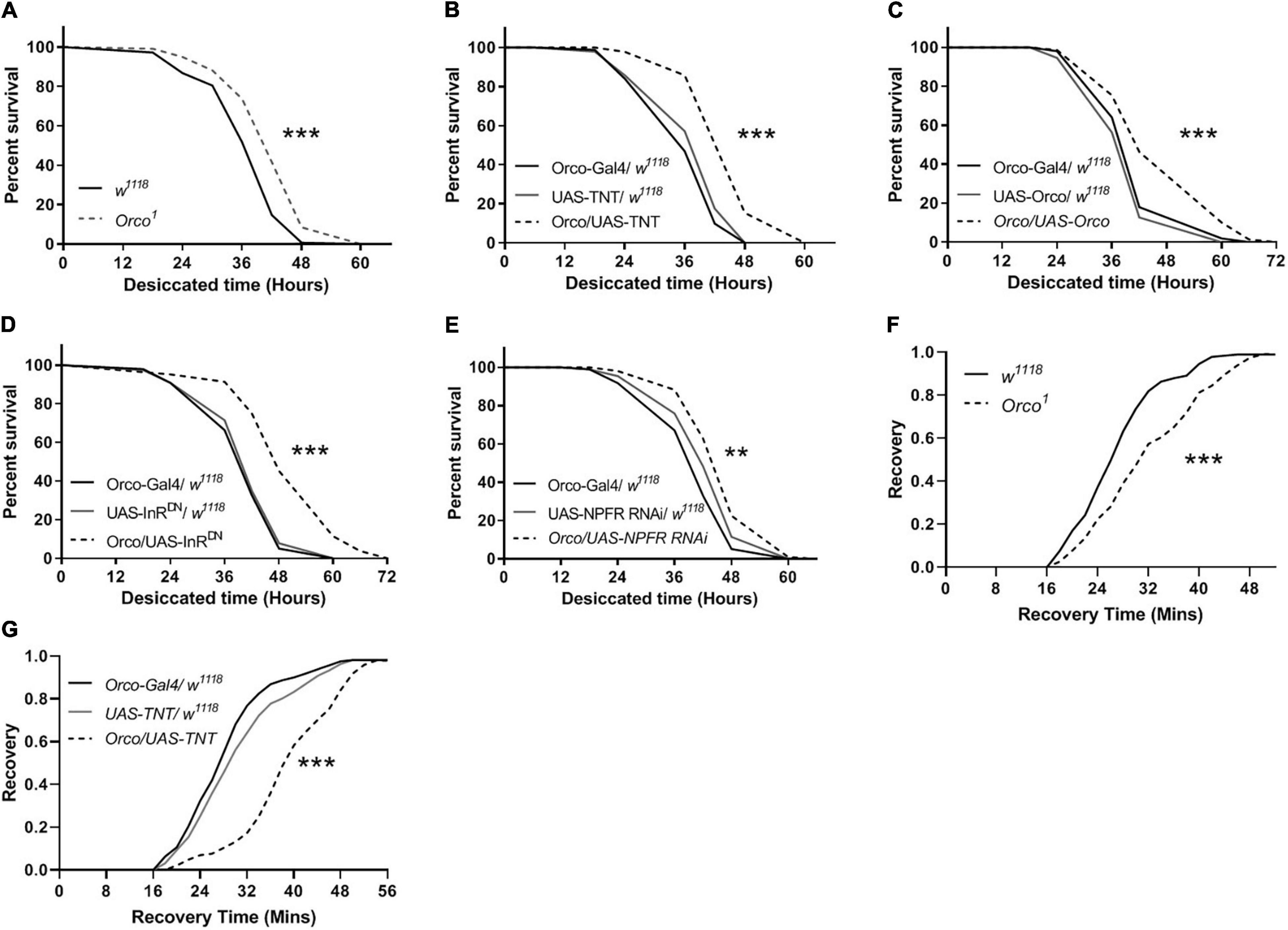
Figure 7. OSNs mediate desiccation and cold resistances. The survival lifespan under desiccated condition was extended in Orco1 mutant flies (A), after expressing TNT in OSNs (B), after expressing Orco in OSNs (C) (n = 9–10 vials/group). Decreasing InR activity (D) or decreasing NPFR level (E) in OSNs enhanced the desiccation resistance (n = 8–9). The recovery time after cold stimulation was extended in Orco1 mutant flies (F), or after expressing TNT in OSNs (G) (n = 9–12). Log-rank (Mantel-Cox) test were used to determine the significant differences. **p < 0.01 and ***p < 0.001.
Discussion
In this study, we investigated the roles of OSNs in food consumption and stress responses in Drosophila melanogaster. We found that defects in OSNs decreased food consumption, increased the TAG level, increased resistance to starvation and desiccation, and decreased cold stress. Or85a and Or42b OSNs played no role in the regulation of food intake. In addition, altering NPFR or insulin signaling in OSNs also modulated food consumption and stress response to starvation or desiccation. Overall, these findings imply a potential relationship between olfactory sensory signaling and animal physiology.
Hunger increases olfactory performance to facilitate the retrieval and ingestion of food in animals (Soria-Gomez et al., 2014). Our results show that flies with loss of functional OSNs consumed less food containing sucrose and yeast extract than control flies and were consistent with the results in which blocking OSNs decreased the consumption of single sucrose food or yeast extract food. This suggests that the odor of yeast extract did not affect the food intake regulated by OSNs. In addition, restored OSNs function in Orco1 mutants rescued the decreased food intake. Previous studies indicated that food-related odors act as one of the complex environmental influences on appetite and lead to short-term overeating in human and Drosophila (Soria-Gomez et al., 2014). The rating of hunger is increased following exposure to food-related odors (Rogers and Hill, 1989). Recent studies found that loss of olfactory input dramatically increases taste sensitivity, which is starvation-independent (Junca et al., 2021). Thus, blocking olfactory signaling inhibits food intake, which may be due to inhibiting appetite behavior. It also may be possible that when OSNs are blocked in flies, the information of food-related odors may not be transferred to the center region of the brain that regulates feeding, resulting in decreased food consumption. Surprisingly, overexpression of Orco in all OSNs that express the olfactory co-receptor Orco decreased food consumption. This unexpected result raises the possibility that some OSNs that regulate aversive response are activated and may override the function of other OSNs that mediate the attractive response; second, the increase of OSNs signaling would lead to sensory-specific satiety. Previous studies have shown that prolonged sensory exposure to foods contributes to meal termination and is associated with lower food intake in Drosophila and mammals (de Graaf and Kok, 2010; Lushchak et al., 2015); finally, overexpression of Orco in OSNs may lead to dysfunction of OSNs, resulting in decreased food consumption. When we activated OSNs by expressing TrpA1 at 30°C, the amount of food was remarkably increased. These results suggest that overexpression of Orco in OSNs does not activate OSNs, but results in dysfunction of OSNs. In starved flies, the sensitivity is increased in Or42b OSNs and is reduced in Or85a OSNs (Ko et al., 2015). Activation of Or85a OSNs induces an aversive response to odors, while activation of Or42b OSNs triggers attraction to odors (Semmelhack and Wang, 2009). However, we found that blocking Or85a or Or42b OSNs does not affect the amount of food consumed. The specific OSNs that regulate feeding enhancement need to be further determined.
Previous studies found that NPF enhances the olfactory sensitivity of OSNs and promotes appetitive odor-induced feeding in Drosophila larvae (Wang et al., 2013; Lee et al., 2017). In this study, decreasing NPF signaling by knocking down NPFR in adult flies leads to a reduction in food intake. This result is in line with several other studies in flies and mammals. Overexpression of NPF in flies and NPY injection in the hypothalamus of rats resulted in increased food consumption (Wahlestedt et al., 1993; Lee et al., 2004). Together, these studies indicate that NPF signaling in OSNs positively regulates foraging and feeding behavior in flies. In addition, lower insulin signaling enhances responses to odors by increasing sNPF signaling in OSNs of starved flies (Wahlestedt et al., 1993; Lee et al., 2004). InR is expressed in a subset of OSNs in Drosophila flies and vertebrates (Huang et al., 2019; Slankster et al., 2020). We found that expressing an active dInR in OSNs resulted in decreased food consumption in adult flies, which was consistent with findings with larvae flies (Huang et al., 2019; Slankster et al., 2020). It is reasonable to speculate that NPFR and InR levels in OSNs could contribute to differential modulation of OSNs based on the animal’s internal state, which in turn impacts food consumption. NPF signaling and insulin signaling in OSNs positively or negatively regulate feeding in flies.
To increase body fat, either energy has to increase or energy expenditure has to decrease (Spiegelman and Flier, 2001). The loss function of OSNs significantly decreased food intake and elevated the levels of TAG in flies, which suggests that increased TAG appears to occur mainly by the reduced energy expenditure. We also found that the loss function of OSNs extended the resistance to starvation. Therefore, blocking OSNs signaling leads to decreased lipid metabolism. Previous studies have shown that loss of olfactory function significantly extended the lifespan of Drosophila (Libert et al., 2007). Dietary restriction has been shown to extend lifespan in almost all model organisms, including Drosophila (Libert et al., 2007). Thus, it is possible that decreased lipid metabolism induced by decreasing OSNs signaling promotes flies to live longer. Interestingly, overexpression of Orco in OSNs remarkably extended the resistance to starvation or desiccation and had no effect on regulating TAG levels, suggesting that dysfunction of OSNs mainly decreases energy output. Inactivated insulin signaling remarkably increased resistance to starvation in flies (Broughton et al., 2005). Consistently, we found that reducing InR levels in OSNs resulted in increased lifespan in flies exposed to starvation and slightly increased the TAG level in flies, which indicates that insulin signaling in OSNs regulates lipid metabolism. In Drosophila, knockdown of NPF in dorsolateral peptidergic neurons (DLPs) or NPF mutants resulted in increased starvation resistance (Broughton et al., 2005). Knockdown of NPFR in OSNs significantly increased starvation resistance. Additionally, dietary restriction (DR) has been shown to increase stress resistance, which may lead to improved health (Wan et al., 2003; Bross et al., 2005). We also found that abnormal OSNs signaling enhanced the desiccation resistance, indicating that OSNs play roles in the regulation of water homeostasis in flies. Blocking OSNs signaling decreased the resistance to cold stress in flies. These results support the conclusion that the olfactory system serves a critical function as a detector for the external environment and as an early warning system to trigger defense responses that are essential for survival (Koike et al., 2021). Therefore, the olfactory system helps flies to sense and adapt to the external environment.
In conclusion, our study demonstrates that stable olfactory signaling input is necessary to regulate feeding behavior and is related to lipid metabolism. NPF signaling and insulin signaling in OSNs have an important role in maintaining the internal state. These studies can help us better understand how animals adapt to their external environment by modulating olfactory sensory input.
Data Availability Statement
The original contributions presented in the study are included in the article/Supplementary Material, further inquiries can be directed to the corresponding author/s.
Author Contributions
JH, YL, and MX conceived and designed the study. WT, JH, MF, TZ, XZ, and YD performed the experiments and analyzed the data. JH and MX wrote the manuscript. YL reviewed and edited the manuscript. All authors contributed to the article and approved the submitted version.
Funding
This work was supported by the National Natural Science Foundation of China (No. 81960793), Provincial University-Industry Support Project in Gansu (No. 2020C-15), Gansu Natural Science Foundation (No. 20JR5RA184), the Talent Introduction Project from Gansu University of Chinese Medicine (No. 2018YJRC-11).
Conflict of Interest
The authors declare that the research was conducted in the absence of any commercial or financial relationships that could be construed as a potential conflict of interest.
Publisher’s Note
All claims expressed in this article are solely those of the authors and do not necessarily represent those of their affiliated organizations, or those of the publisher, the editors and the reviewers. Any product that may be evaluated in this article, or claim that may be made by its manufacturer, is not guaranteed or endorsed by the publisher.
Acknowledgments
We would like to thank the Bloomington Stock Center (BSC) for generously providing fly stocks. We are grateful to the members of the JH’s laboratory for providing useful comments on the manuscript.
Supplementary Material
The Supplementary Material for this article can be found online at: https://www.frontiersin.org/articles/10.3389/fnbeh.2022.788633/full#supplementary-material
Supplementary Figure 1 | Activating OSNs promote the food consumption in non-starved flies. Food intake was increased when activating OSNs by expressing TrpA1 in Orco-GAL4 driver at 30°C (n = 12–15).
Supplementary Figure 2 | Blocking OSNs has no function in nutrient preference. Orco1 mutant flies and w1118 flies all prefer 5% sucrose with 5% yeast extract diet than 5% sucrose diet.
References
Albrecht, J., Schreder, T., Kleemann, A. M., Schopf, V., Kopietz, R., Anzinger, A., et al. (2009). Olfactory detection thresholds and pleasantness of a food-related and a non-food odour in hunger and satiety. Rhinology 47, 160–165. doi: 10.1093/chemse/bjaa045
Bracker, L. B., Siju, K. P., Varela, N., Aso, Y., Zhang, M., Hein, I., et al. (2013). Essential role of the mushroom body in context-dependent CO(2) avoidance in Drosophila. Curr. Biol. 23, 1228–1234. doi: 10.1016/j.cub.2013.05.029
Bross, T. G., Rogina, B., and Helfand, S. L. (2005). Behavioral, physical, and demographic changes in Drosophila populations through dietary restriction. Aging Cell 4, 309–317. doi: 10.1111/j.1474-9726.2005.00181.x
Broughton, S. J., Piper, M. D., Ikeya, T., Bass, T. M., Jacobson, J., Driege, Y., et al. (2005). Longer lifespan, altered metabolism, and stress resistance in Drosophila from ablation of cells making insulin-like ligands. Proc. Natl. Acad. Sci. U.S.A. 102, 3105–3110. doi: 10.1073/pnas.0405775102
Casanova, N., Beaulieu, K., Finlayson, G., and Hopkins, M. (2019). Metabolic adaptations during negative energy balance and their potential impact on appetite and food intake. Proc. Nutr. Soc. 78, 279–289. doi: 10.1017/S0029665118002811
de Graaf, C., and Kok, F. J. (2010). Slow food, fast food and the control of food intake. Nat. Rev. Endocrinol. 6, 290–293. doi: 10.1038/nrendo.2010.41
Enell, L. E., Kapan, N., Soderberg, J. A., Kahsai, L., and Nassel, D. R. (2010). Insulin signaling, lifespan and stress resistance are modulated by metabotropic GABA receptors on insulin producing cells in the brain of Drosophila. PLoS One 5:e15780. doi: 10.1371/journal.pone.0015780
Graham, P., and Pick, L. (2017). Drosophila as a model for diabetes and diseases of insulin resistance. Curr. Top. Dev. Biol. 121, 397–419. doi: 10.1016/bs.ctdb.2016.07.011
He, J., Tan, A., Ng, S. Y., Rui, M., and Yu, F. (2021). Cannabinoids modulate food preference and consumption in Drosophila melanogaster. Sci. Rep. 11:4709. doi: 10.1038/s41598-021-84180-2
Huang, T. W., Li, S. T., Wang, Y. H., and Young, T. H. (2019). Regulation of chitosan-mediated differentiation of human olfactory receptor neurons by insulin-like growth factor binding protein-2. Acta Biomater. 97, 399–408. doi: 10.1016/j.actbio.2019.08.022
Junca, P., Stanley, M., Musso, P.-Y., and Gordon Michael, D. (2021). Modulation of taste sensitivity by the olfactory system in Drosophila. bioRxiv [Preprint]. doi: 10.1101/2021.03.30.437740
Ko, K. I., Root, C. M., Lindsay, S. A., Zaninovich, O. A., Shepherd, A. K., Wasserman, S. A., et al. (2015). Starvation promotes concerted modulation of appetitive olfactory behavior via parallel neuromodulatory circuits. Elife 4:e08298. doi: 10.7554/eLife.08298
Koike, K., Yoo, S.-J., Bleymehl, K., Omura, M., Zapiec, B., Pyrski, M., et al. (2021). Danger perception and stress response through an olfactory sensor for the bacterial metabolite hydrogen sulfide. Neuron 109, 2469–2484.e7. doi: 10.1016/j.neuron.2021.05.032
Koyama, T., Texada, M. J., Halberg, K. A., and Rewitz, K. (2020). Metabolism and growth adaptation to environmental conditions in Drosophila. Cell. Mol. Life Sci. 77, 4523–4551. doi: 10.1007/s00018-020-03547-2
Lee, K. S., You, K. H., Choo, J. K., Han, Y. M., and Yu, K. (2004). Drosophila short neuropeptide F regulates food intake and body size. J. Biol. Chem. 279, 50781–50789. doi: 10.1074/jbc.M407842200
Lee, S., Kim, Y. J., and Jones, W. D. (2017). Central peptidergic modulation of peripheral olfactory responses. BMC Biol. 15:35. doi: 10.1186/s12915-017-0374-6
Libert, S., Zwiener, J., Chu, X., Vanvoorhies, W., Roman, G., and Pletcher, S. D. (2007). Regulation of Drosophila life span by olfaction and food-derived odors. Science 315, 1133–1137. doi: 10.1126/science.1136610
Lushchak, O. V., Carlsson, M. A., and Nassel, D. R. (2015). Food odors trigger an endocrine response that affects food ingestion and metabolism. Cell. Mol. Life Sci. 72, 3143–3155. doi: 10.1007/s00018-015-1884-4
McCrickerd, K., and Forde, C. G. (2016). Sensory influences on food intake control: moving beyond palatability. Obes. Rev. 17, 18–29. doi: 10.1111/obr.12340
Peng, M., Coutts, D., Wang, T., and Cakmak, Y. O. (2019). Systematic review of olfactory shifts related to obesity. Obes. Rev. 20, 325–338. doi: 10.1111/obr.12800
Reisenman, C. E., and Scott, K. (2019). Food-derived volatiles enhance consumption in Drosophila melanogaster. J. Exp. Biol. 222:jeb202762. doi: 10.1242/jeb.202762
Riera, C. E., Tsaousidou, E., Halloran, J., Follett, P., Hahn, O., Pereira, M., et al. (2017). The sense of smell impacts metabolic health and obesity. Cell Metab. 26, 198–211. doi: 10.1016/j.cmet.2017.06.015
Rogers, P. J., and Hill, A. J. (1989). Breakdown of dietary restraint following mere exposure to food stimuli: interrelationships between restraint, hunger, salivation, and food intake. Addict. Behav. 14, 387–397. doi: 10.1016/0306-4603(89)90026-9
Root, C. M., Ko, K. I., Jafari, A., and Wang, J. W. (2011). Presynaptic facilitation by neuropeptide signaling mediates odor-driven food search. Cell 145, 133–144. doi: 10.1016/j.cell.2011.02.008
Savigner, A., Duchamp-Viret, P., Grosmaitre, X., Chaput, M., Garcia, S., Ma, M., et al. (2009). Modulation of spontaneous and odorant-evoked activity of rat olfactory sensory neurons by two anorectic peptides, insulin and leptin. J. Neurophysiol. 101, 2898–2906. doi: 10.1152/jn.91169.2008
Semmelhack, J. L., and Wang, J. W. (2009). Select Drosophila glomeruli mediate innate olfactory attraction and aversion. Nature 459, 218–223. doi: 10.1038/nature07983
Slankster, E., Kollala, S., Baria, D., Dailey-Krempel, B., Jain, R., Odell, S. R., et al. (2020). Mechanism underlying starvation-dependent modulation of olfactory behavior in Drosophila larva. Sci. Rep. 10:3119. doi: 10.1038/s41598-020-60098-z
Soria-Gomez, E., Bellocchio, L., Reguero, L., Lepousez, G., Martin, C., Bendahmane, M., et al. (2014). The endocannabinoid system controls food intake via olfactory processes. Nat. Neurosci. 17, 407–415. doi: 10.1038/nn.3647
Spiegelman, B. M., and Flier, J. S. (2001). Obesity and the regulation of energy balance. Cell 104, 531–543. doi: 10.1016/s0092-8674(01)00240-9
Stafford, L. D., and Welbeck, K. (2011). High hunger state increases olfactory sensitivity to neutral but not food odors. Chem. Senses 36, 189–198. doi: 10.1093/chemse/bjq114
Tennessen, J. M., Barry, W. E., Cox, J., and Thummel, C. S. (2014). Methods for studying metabolism in Drosophila. Methods 68, 105–115. doi: 10.1016/j.ymeth.2014.02.034
Touhara, K., and Vosshall, L. B. (2009). Sensing odorants and pheromones with chemosensory receptors. Annu. Rev. Physiol. 71, 307–332. doi: 10.1146/annurev.physiol.010908.163209
Wahlestedt, C., Pich, E. M., Koob, G. F., Yee, F., and Heilig, M. (1993). Modulation of anxiety and neuropeptide Y-Y1 receptors by antisense oligodeoxynucleotides. Science 259, 528–531. doi: 10.1126/science.8380941
Wan, R., Camandola, S., and Mattson, M. P. (2003). Intermittent food deprivation improves cardiovascular and neuroendocrine responses to stress in rats. J. Nutr. 133, 1921–1929. doi: 10.1093/jn/133.6.1921
Wang, Y. H., Pu, Y. H., and Shen, P. (2013). Neuropeptide-gated perception of appetitive olfactory inputs in Drosophila larvae. Cell Rep. 3, 820–830. doi: 10.1016/j.celrep.2013.02.003
Keywords: olfaction, food consumption, physiology, stress resistance, Drosophila melanogaster
Citation: He J, Tuo W, Zhang X, Dai Y, Fang M, Zhou T, Xiu M and Liu Y (2022) Olfactory Senses Modulate Food Consumption and Physiology in Drosophila melanogaster. Front. Behav. Neurosci. 16:788633. doi: 10.3389/fnbeh.2022.788633
Received: 03 October 2021; Accepted: 22 February 2022;
Published: 01 April 2022.
Edited by:
Osborne F. X. Almeida, Max Planck Institute of Psychiatry (MPI), GermanyReviewed by:
Oleh Lushchak, Vasyl Stefanyk Precarpathian National University, UkraineKatrin Vogt, University of Konstanz, Germany
Copyright © 2022 He, Tuo, Zhang, Dai, Fang, Zhou, Xiu and Liu. This is an open-access article distributed under the terms of the Creative Commons Attribution License (CC BY). The use, distribution or reproduction in other forums is permitted, provided the original author(s) and the copyright owner(s) are credited and that the original publication in this journal is cited, in accordance with accepted academic practice. No use, distribution or reproduction is permitted which does not comply with these terms.
*Correspondence: Minghui Xiu, Z3N6eW5reEAxNjMuY29t; Yongqi Liu, bGl1eW9uZ3FpNzNAMTYzLmNvbQ==