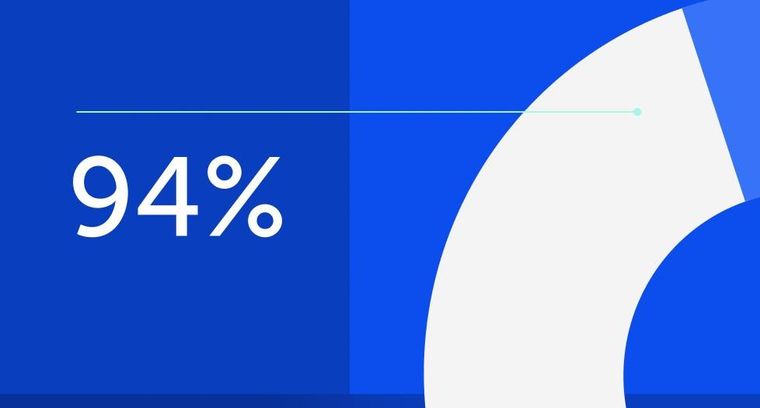
94% of researchers rate our articles as excellent or good
Learn more about the work of our research integrity team to safeguard the quality of each article we publish.
Find out more
ORIGINAL RESEARCH article
Front. Behav. Neurosci., 14 October 2021
Sec. Motivation and Reward
Volume 15 - 2021 | https://doi.org/10.3389/fnbeh.2021.762297
This article is part of the Research TopicBehavior-Driven Changes in Gene ExpressionView all 9 articles
Opioid addiction remains a widespread issue despite continuous attempts by the FDA to help maintain abstinence. Melatonin is a neurohormone considered to be involved only in the neuroendocrine and reproductive systems; however, recent reports have demonstrated its potential to attenuate drug addiction and dependence. Cumulative studies have suggested that melatonin can attenuate the rewarding effects of several drugs of abuse, including opioids. This study aimed to investigate the effect of melatonin (50 mg/kg) on morphine (5 mg/kg) to produce place preference. We also investigated the effect of melatonin and morphine on the expression of GLT-1, BDNF, NF-κB, and CREB within the nucleus accumbens. Male Wistar rats were divided into control, morphine, melatonin, and the morphine + melatonin groups. The study involved a two-phase habituation phase from day 1 to day 3 and an acquisition phase from day 5 to day 14. The conditioned place preference (CPP) score, distance traveled, resting time, ambulatory count, and total activity count were measured for all animals. Rats that received morphine showed a significant increase in CPP score compared to those in the control group. Morphine treatment reduced the mRNA expression of GLT-1, BDNF, and CREB and increased that of NF-κB. However, melatonin treatment administered 30 min before morphine treatment attenuated morphine place preference and reversed GLT-1, BDNF, NF-κB, and CREB expression levels. In conclusion, the study results indicate, for the first time, the new potential targets of melatonin in modulating morphine-induced CPP.
Opioid addiction is a complex phenomenon that heavily impacts economics, health care, and society as a whole (Motaghinejad et al., 2014). Morphine is commonly used in pain management and is considered the gold standard for opioids (Polomano et al., 2008; Jia et al., 2017; Mahshidfar et al., 2017). However, repeated use of morphine has been associated with significant changes in brain function and neural changes, especially in cases of long-term use (Allan et al., 2001; Spiga et al., 2005; Ballantyne, 2018). Several studies have suggested that long-term use of morphine is associated with depression, anxiety, and decline in cognitive function (Compton et al., 2000; Swendsen and Merikangas, 2000; Curran et al., 2001). Its long-term use is also associated with tolerance to its analgesic effect and withdrawal symptoms upon cessation (Mao et al., 2002; Desjardins et al., 2008). These side effects cause users to increase morphine doses, making cessation of its use difficult, as they become physiologically and psychologically dependent on the drug. Thus, there is an urgent need to investigate the molecular effects of morphine addiction and explore potential targets and possible treatments.
Cumulative evidence has established that the glutamatergic system plays an essential role in developing tolerance to morphine and the induction of addiction (Mao et al., 2002; Hao et al., 2005; Haghparast et al., 2007). Repeated administration of morphine can increase extracellular glutamate levels and potentiate glutamate excitotoxicity (Sepulveda et al., 2004; Jacobs et al., 2005). Therefore, several studies have linked the increase in extracellular glutamate levels in several brain areas such as the nucleus accumbens (NAc) with morphine-seeking behavior and withdrawal symptoms (Baharlouei et al., 2015; Yuan et al., 2017; Kim et al., 2018). Furthermore, repeated administration of morphine is associated with a reduction in glutamate clearance and downregulation of the glutamate transporter (Ozawa et al., 2001; Lim et al., 2005). The clearance of extracellular glutamate is mediated through several receptors such as glutamate receptor 1 (GLT-1) and cysteine-glutamate exchanger (Grewer et al., 2014). GLT-1 is considered the major transporter for glutamate clearance in the synaptic cleft, with approximately 90% of the total glutamate being transported into the glia through GLT-1 (Danbolt, 2001). Thus, glutamatergic excitotoxicity and glutamate clearance dysfunction are important therapeutic targets for morphine addiction.
Opioids can alter the immune system and induce neuroinflammation with prolonged morphine use (Roy and Loh, 1996; Sacerdote, 2006). The induced inflammatory responses can facilitate the mediation of neuroinflammatory cytokines and participate in activating transcription factor nuclear factor-kappa B (NF-κB; Roy et al., 1998; Hao et al., 2011; Nennig and Schank, 2017). Furthermore, NF-κB activates other transcription genes, regulates inflammation, and modulates synaptic processes, neurotransmission, and neuroprotection (Kaltschmidt and Kaltschmidt, 2009; Liu et al., 2017). It has been found that chronic morphine can increase NF-κB function in vitro (Sawaya et al., 2009) and elevated NF-κB expression in NAc (Hemby, 2004; Zhang et al., 2011). Therefore, blocking NF-κB in the NAc inhibited morphine-induced conditioned place preference (CPP) in rats (Zhang et al., 2011). Furthermore, morphine can modulate the cAMP response element-binding protein (CREB) transcription, which is involved in many neuronal processes, including neuronal survival, long-term memory, and morphine addiction (Yin and Tully, 1996; Walton and Dragunow, 2000; Martin et al., 2009). Also, it has been found that morphine administration is associated with reduced CREB levels in the NAc (Moron et al., 2010; Tenayuca and Nazarian, 2012), suggesting that behavioral adaptation in response to morphine-associated environmental cues is connected to the CREB signaling pathway. Also, several studies have shown that CREB is involved in morphine addiction (Yin and Tully, 1996; Walton and Dragunow, 2000; Martin et al., 2009). In fact, it has been reported that morphine-induced CPP was associated with lower expression levels of CREB in the NAc (Zhou and Zhu, 2006; Chen et al., 2012) and ventral tegmental area (Rezai et al., 2018). Furthermore, many studies have also described the effects of morphine addiction in the induction of neuroinflammatory regulators, including brain-derived neurotrophic factor (BDNF; Shen et al., 2012; Charkhpour et al., 2015). These changes were observed in morphine-treated animals; these animals showed lower expression levels of BDNF after serious escalating morphine doses (Fatahi et al., 2020). Therefore, the events of neuroinflammation and exploring these genes in the NAc could be a potential target in understanding morphine addiction.
The pineal gland secretes an endogenous indoleamine called melatonin (N-acetyl-5-methoxy-tryptamine; Hardeland et al., 2006; Ma et al., 2020). Melatonin is a neurohormone that activates the melatonin receptors (MTR1 and MTR2), modulating circadian rhythms and many physiological functions in mammals (Hardeland et al., 2006). Melatonin has long been considered to be involved only in the neuroendocrine and reproductive systems; however, several recent reports have suggested that melatonin activity exceeds that of a hormonal modulator. Melatonin has several physiological functions, including effects on mood, sleep, and immunomodulation, as well as antioxidant, and anti-inflammatory effects (Pandi-Perumal et al., 2008; Claustrat and Leston, 2015). In addition, the anti-inflammatory effect of melatonin has been shown to result in reduced NF-κB production, which in turn reduces the activity of several pro-inflammatory cytokines and inflammatory mediators (Beni et al., 2004; Li et al., 2005). Many studies have also claimed that melatonin has a strong neuroprotective effect against glutamate-induced excitotoxicity (Espinar et al., 2000; Lima et al., 2003; Vishnoi et al., 2016). Recently, melatonin has been shown to attenuate the rewarding behavior associated with many drugs of abuse such as cocaine (Sircar, 2000; Barbosa-Mendez and Salazar-Juarez, 2020) and alcohol (Vengeliene et al., 2015).
Cumulative studies have suggested that alcohol addiction is associated with reduced melatonin levels during sleep in humans and rodents (Peres et al., 2011; Crespi, 2012; Vengeliene et al., 2015). In addition, melatonin has been found to modulate the rewarding effects of many drugs of abuse, indicating that it plays an essential role in drug addiction (Onaolapo and Onaolapo, 2018). For example, it has been reported that melatonin attenuates cocaine-induced place preference and decreases both dopamine levels and locomotor sensitization in rats, whereas using luzindole (an MTR blocker) reverses the effects of melatonin (Barbosa-Mendez et al., 2021). Another study has shown that melatonin treatment can block cocaine self-administration and decrease relapse-like behavior (Takahashi et al., 2017). In addition, it has been reported that MTR1 and MTR2 knockout mice do not show place preference for methamphetamine, which further confirms the role of the melatonergic system in drug reward and addiction (Clough et al., 2014).
The NAc is an important brain region known to be critically involved in the learning process and rewards (Day and Carelli, 2007; Gold et al., 2019; Soares-Cunha et al., 2020). The NAc has different projections from and to multiple brain areas, including the prefrontal cortex (PFC), subcortical structures, hippocampus, amygdala, and ventral tegmental area (French and Totterdell, 2002; Xia et al., 2011, 2020; Piantadosi et al., 2017). Therefore, several studies have evaluated the contribution of the NAc in drug-related behaviors. For instance, opioid reinforcing and seeking effects are mediated through the dopaminergic and glutamatergic neurotransmission in the NAc (LaLumiere and Kalivas, 2008; Alshehri et al., 2018; Corre et al., 2018). Moreover, it has been found that morphine injection in the ventral tegmental area showed an augmented increase of the dopamine level in NAc (Leone et al., 1991). Recently, studies have shown that accumbal glutamate homeostasis is a potential target in attenuating cocaine-seeking in both humans (Engeli et al., 2020) and animals (Zhang et al., 2021).
Given the background mentioned above, this study investigated the effect of melatonin on morphine-induced CPP and the effect of melatonin on modulating morphine-induced changes in the expression of GLT-1, BDNF, NF-κB, and CREB in NAc. Thus, we show that the melatonin administration has effects on decreasing morphine-induced CPP and reversing some of the key markers of inflammation in the NAc.
Male Wistar rats (weight 250–280 g) were obtained from the King Fahd Medical Research Center, King Abdulaziz University, Jeddah, and housed in plastic cages with free access to standard feed and water. Rearing conditions for the animals were as follows: 21°C, humidity 50%, and 12/12 light/dark cycle. The study was approved by the Animal Care and Use Committee (ACUC) guidelines of the King Fahd Medical Research Center. In addition, the experiments were approved by the Biomedical Ethics Research Committee (Reference 405-20) at King Abdulaziz University, following the guidelines of ethics and research on living creatures, prepared by the King Abdulaziz City for Science and Technology (KACST), approved by Royal Decree No. M/59 on 24 August 2010. Melatonin was purchased from Sigma-Aldrich (M5250); a fresh stock solution was prepared every day in 0.5% ethanol and diluted with saline (vehicle). Morphine was supplied by King Abdulaziz University Hospital Pharmacy (50 mg/kg, i.p.; Takahashi et al., 2017). The vehicle was composed of 0.5% ethanol and saline.
The CPP apparatus consisted of two chambers made of Plexiglas and one small external chamber in the middle of the two chambers. The apparatus was obtained from Columbus Instruments, Columbus, OH, USA. The white chamber had vertical white stripes with a smooth white floor, whereas the black chamber had a small square of white and black and a small circle drilled in the floor. The apparatus was equipped with infrared sensors to measure the movement and activity of the animals during the test. In addition, an Auto-Track software (OPTO-MAX) was connected to the apparatus to automatically calculate time spent, ambulatory and distant movement, and resting time.
The study consisted of two phases (Figure 1): the habituation phase, wherein from day 1 to day 3, animals explored the apparatus for 20 min with the partition divider raised between the two chambers. The habituation was done by placing the animal in the external chamber and then raising the gate to allow the animal to enter the apparatus. Then, on day 4, the pre-test was conducted for 20 min. The test was conducted by placing the animal in the external chamber and raising the gate to allow the animal to enter the apparatus. Once the animal entered the apparatus, the Auto-Track software (OPTO-MAX) automatically calculated the time spent, total activity count, ambulatory count, resting time, and distance traveled in each chamber. Most animals showed a preference for the black chamber. Therefore, a biased approach was used to avoid excluding many animals. Two animals were excluded due to diarrhea. Next, in the acquisition phase from day 5 to day 14, the partition divider separated the two chambers. Then, the post-test was conducted for 20 min on day 15, in which animals were placed in the apparatus with the partition divider raised, and the CPP score was calculated. On day 16, all the animals were euthanized using isoflurane.
Figure 1. The experiment schedule for the habitation and acquisition of conditioned place preference (CPP). Animals underwent 3 days of habitation (20 min each day), followed by pre-test on day 4 (20 min). During the acquisition phase, the control group received vehicle (1 ml/kg), the morphine group received (5 mg/kg), the melatonin group received (50 mg/kg), and the morphine + melatonin received melatonin (50 mg/kg) 30 min before morphine (5 mg/kg) every other day alternatively between the drug paired chamber and the vehicle paired chamber (45 min each day). Then, at the end of the acquisition phase, which continued for 10 days, the post-test was conducted on day 15 (20 min). Morphine 5 mg/kg (i.p), melatonin 50 mg/kg.
Animals were divided into four groups (n = 7–8) as shown in Table 1. Control group, morphine group, melatonin group, and the morphine + melatonin group. The control group was administered the vehicle during the acquisition phase in the white and black chambers alternately for a total of 10 days. The morphine group received morphine i.p. (5 mg/kg) during the acquisition phase in the white chamber (drug-paired chamber) and the vehicle in the black chamber alternately for a total of 10 days. The melatonin group received melatonin i.p. (50 mg/kg) in the white chamber (drug-paired chamber) and the vehicle in the black chamber alternately during the acquisition phase for a total of 10 days. The morphine + melatonin group received melatonin i.p. (50 mg/kg) 30 min before morphine i.p. (5 mg/kg) in the white chamber (drug-paired chamber) and the vehicle in the black chamber alternately during the acquisition phase for a total of 10 days.
Animal brains were collected on day 16, immediately frozen, and stored at −80°C. The NAc was collected as pooled tissue containing NAc (core and shell) and isolated using a cryostat machine (Leica Biosystems). The NAc region (1.2–3.7 mm from bregma) was identified using the Brain Rat Atlas (Paxinos and Watson, 2006) and as performed previously in Alshehri et al. (2018) and Hammad et al. (2017).
Total RNA was extracted from the NAc using the RNeasy Mini Kit (Qiagen, USA). Complementary DNA strand synthesis was performed using the cDNA synthesis kit (Sigma-Aldrich, UK). The qPCR quantification was done using the relative quantification approach by comparing the Ct value of (GLT-1, NFκB, CREB, and BDNF) to GAPDH, which was used as the reference gene for all mRNA expression analyses. All samples were run as triplicate, and the mean of the Ct value was taken. The relative expression of the tested genes was performed using the 2−ΔΔCT method (Livak and Schmittgen, 2001; Deng et al., 2012). The Real-time qPCR was performed using the following primers to detect GLT-1- forward primer: 5′-GAGCATTGGTGCAGCCAGTATT-3′, reverse primer: 5′-GTTCTCATTCTATCCAGCAGCCAG-3′, NF-κB forward primer: 5′-GTCATCAGGAAGAGGTTTGGCT-3′, reverse primer: 5′-TGATAAGCTTAGCCCTTGCAGC-3′, BDNF forward primer: 5′-TCTACGAGACCAAGTGTAATCC-3′, reverse primer: 5′-TATGAACCGCCAGCCAAT-3′, CREB forward primer: 5′-CCAAACTAGCAGTGGGCAGT-3′, reverse primer: 5′- GAATGGTAGTACCCGGCTGA-3′, GAPDH (house-keeping gene) forward primer: 5′-CCCCCAATGTATCCGTTGTG-3′, reverse primer: 5′-TAGCCCAGGATGCCCTTTAGT-3′.
One-way analysis of variance (ANOVA) followed by Tukey’s post hoc tests was used to analyze the CPP score, distance traveled, resting time, ambulatory count, and total activity count. The CPP score was calculated as follows: (non-preferred chamber)/(total time spent in both chambers; Sun et al., 2018). In addition, one-way ANOVA was used to analyze the mRNA expression of GLT-1, NFκB, CREB, and BDNF. GAPDH was used as the reference gene for all mRNA expression analyses. All data were analyzed using Prism version 9.1.0. (p-value < 0.05).
The effect of melatonin on morphine-induced CPP was measured using the CPP paradigm. The analysis revealed a significant difference between the treatment groups (F(3,26) = 17.10, p < 0.0001, Figure 2A). Further analysis with a Tukey’s post hoc test showed that animals that received morphine during the acquisition phase (morphine group) spent significant time in the drug-paired chamber with a higher CPP score compared to the control group (p = 0.0008) and the melatonin group (p < 0.0001). However, repeated melatonin treatment 30 min before receiving morphine (morphine + melatonin group) attenuated morphine-induced CPP, resulting in a significantly lower amount of time spent in the drug-paired chamber, with a lower CPP score than the morphine alone group (p = 0.0001). The melatonin-treated group did not show any significant change in CPP score compared to the control group (p = 0.1757) or the melatonin + morphine group (p = 0.5120).
Figure 2. Effect of melatonin and morphine on CPP score (A). The morphine group showed a higher CPP score compared to the control group and the melatonin group. Melatonin treatment 30 min before morphine (morphine + melatonin group) significantly lowers the CPP score than the morphine alone group. The melatonin group did not show any change in CPP score compared to the control group or the melatonin + morphine group. No changes were observed in total activity count (B), ambulatory count (C), resting time (D), and distance traveled (E). ***P < 0.001; ****P < 0.0001. MOR, morphine; MEL, melatonin.
Analysis of the total activity count, which calculates any beam break due to animal movement, including stereotypical movement (scratching or grooming behavior), showed that the total travel distance did not differ significantly between the treatment groups (F(3,24) = 0.7659, p = 0.5244, Figure 2B). In addition, the ambulatory count, which is the number of instances when the animal breaks the beams inside both chambers, excluding instances of stereotypic movement, did not differ significantly between the treatment groups (F(3,24) = 0.7460, p = 0.5353, Figure 2C). Moreover, the resting time, representing the time when the animal did not move inside the chambers, did not change significantly between the treatment groups (F(3,27) = 1.336, p = 0.2833, Figure 2D). The total distance traveled inside the chamber, calculated in inches, did not significantly differ between the treatment groups (F(3,23) = 1.280, p = 0.3048, Figure 2E).
The effect of melatonin and morphine on GLT-1 mRNA expression was also measured. Significant difference was found between the treatment groups (F(3,24) = 13.23, p < 0.0001, Figure 3A). Additional analysis with Tukey’s post hoc test showed that animals that received morphine during the acquisition phase (morphine group) had lower GLT-1 expression compared to those in the control group animals (p = 0.0093). However, repeated melatonin treatment 30 min before morphine (morphine + melatonin group), which attenuated morphine-induced CPP, successfully prevented the reduction in GLT-1 expression (p = 0.0001), with no significant difference in GLT-1 levels compared to the control group (p = 0.2914).
Figure 3. Effect of melatonin and morphine on GLT-1 mRNA expression (A). Morphine treatment decreased GLT-1 mRNA expression compared to the control and melatonin group; however, melatonin treatment in (morphine + melatonin) restored GLT-1 mRNA expression. Effect of melatonin and morphine on NF-κB mRNA expression (B). Morphine treatment decreased NF-κB mRNA expression compared to the control and melatonin group; however, melatonin treatment in (morphine + melatonin) reduced NF-κB mRNA expression. Effect of melatonin and morphine on BDNF (C) and CREB (D) mRNA expression. Morphine treatment decreased BDNF and CREB mRNA expression compared to the control and melatonin group; however, melatonin treatment in (morphine + melatonin) restored BDNF and CREB mRNA expression (*P < 0.05; **P < 0.01; ***P < 0.001; ****P < 0.0001). MOR, morphine; MEL, melatonin; GLT-1, glutamate transporter-1; CREB, cAMP response element-binding protein; BDNF, brain-derived neurotrophic factor.
The effect of melatonin and morphine on NF-κB mRNA expression was also analyzed. The results showed a significant difference between the treatment groups (F(3,24) = 12.48, p < 0.0001, Figure 3B) and that animals that received morphine during the acquisition phase (morphine group) had a higher NF-κB expression level compared to the control group (p = 0.0363). However, repeated melatonin treatment 30 min before morphine (morphine + melatonin group) was associated with blocking morphine-induced CPP and reduction in the expression of NF-κB compared to that in the morphine group (p = 0.0032), and restored NF-κB expression compared to that in the control group (p = 0.7331). Moreover, repeated treatment with melatonin alone (melatonin group) significantly reduced NF-κB expression compared to that in the control group (p = 0.0239).
With respect to the mRNA expression of BDNF, a significant difference between the treatment groups was found (F(3,24) = 5.145, p = 0.0069, Figure 3C). The results showed that animals in the morphine group had a lower BDNF expression level than those in the control group (p = 0.0425). However, repeated melatonin treatment 30 min before morphine (morphine + melatonin group) increased the expression of BDNF compared to that in the morphine group (p = 0.0136) and reversed BDNF expression compared to that in the control group (p = 0.9576). Repeated treatment with melatonin alone (melatonin group) did not induce any change in BDNF expression compared to that in the control group (p = 0.9560).
Analysis of the mRNA expression of CREB showed a significant difference between the treatment groups (F(3,24) = 8.080, p = 0.0007, Figure 3D). Further analysis showed that the morphine group had a lower CREB expression level compared to the control group (p = 0.0004). However, repeated melatonin treatment 30 min before morphine (morphine + melatonin group) increased the expression of CREB compared to that in the morphine group (p = 0.0499) and did not affect the CREB expression compared to that in the control group (p = 0.2082). Repeated treatment with melatonin alone (melatonin group) did not induce any change in CREB expression compared to that in the control group (p = 0.5276).
The CPP paradigm is a vital tool used to measure different drug rewards based on Pavlov’s conditioning principles (Bardo and Bevins, 2000; Tzschentke, 2007). Therefore, drugs of abuse, such as opioids, act as conditional stimuli and produce reinforcing effects in humans and rodents (Shen et al., 2017; Alshehri et al., 2018; Zhu et al., 2019). In the CPP paradigm, animals receive an appetitive stimulus (such as morphine) and associate the reward with contexts and cues in the drug-paired chamber inside the CPP apparatus (Kobrin et al., 2016; Meepong and Sooksawate, 2019). Thus, an increase in the time spent in the drug-paired chamber than in the control chamber during the post-test is considered to support the drug’s reinforcing effect (Kobrin et al., 2016; Meepong and Sooksawate, 2019). In this study, we aimed to investigate the effect of melatonin on morphine-induced CPP using the CPP paradigm. Morphine conditioning was established in these animals by repeated administration of morphine in a drug-paired chamber over five sessions. However, melatonin treatment before morphine administration successfully attenuated morphine-induced CPP during the post-test.
The dopaminergic system is involved in drug abuse, reinforcing effects, and addiction (Solinas et al., 2019). It is known that homeostasis between the dopaminergic and DA and glutamatergic is essential in regulating cortico-striatal rhythms, where its imbalance can enhance cognitive dysfunctions and motor deficits (Bamford et al., 2004; Calabresi et al., 2007; Gleich et al., 2015). Repeated use of drugs of abuse could impair the capacity of PFC input to the NAc to regulate drug-seeking behavior in corticostriatal circuitry (Scofield et al., 2016). Cumulative studies have extensively investigated the role of dopaminergic neurotransmission in the rewarding properties of morphine by examining genetic alteration or deletion of dopamine receptors (Smith et al., 2002; Francès et al., 2004) and through using dopamine receptor modulator (Manzanedo et al., 2001; Frances et al., 2004; Assar et al., 2016) in the CPP paradigms. Moreover, several reports have suggested that the glutamatergic system also participates in drug reward and seeking behavior (Kalivas, 2004; Kalivas et al., 2005). Deficiency in glutamate homeostasis leads to drug-induced synaptic plasticity and morphological changes in the dendritic spines in the NAc area (Robinson and Kolb, 1997). Thus, using transferring the GLT-1 gene into the NAc by using recombinant adenoviruses techniques, has attenuated morphine-induced CPP (Fujio et al., 2005). In addition, injecting glutamate receptor antagonists such as NMDA blocker in the NAc was revealed to attenuate morphine-induced CPP in rats (Bespalov and Zvartau, 1996). Altering glutamate homeostasis has also been reported with other abuse drugs such as cocaine (Kalivas, 2004), alcohol (Dodd et al., 2000), nicotine (Lambe et al., 2003), cannabinoids (Brown et al., 2003), and opioids (Fundytus, 2001). Thus, restoring glutamate homeostasis is a potential target for drug reward and addiction. In this study, morphine-induced place preference was associated with a reduction in GLT-1 mRNA expression. Melatonin treatment before morphine reversed GLT-1 expression levels in the NAc. Therefore, the pattern of GLT-1 alteration is similar to that observed in other models of addiction, and thus GLT-1 could be an appropriate target for future functional examination using microdialysis or pharmacological techniques.
Moreover, this study evaluated the effect of morphine-induced CPP on the expression of NF-κB, BDNF, and CREB in the NAc. As a result, morphine was associated with a higher mRNA expression level of NF-κB. Previously, drugs of abuse were known to activate NF-κB, indicating the functional role of NF-κB’s rewarding effects. For example, inhibiting NF-κB in the NAc was shown to attenuate morphine-induced place preference in rats (Zhang et al., 2011). For instance, fear memory inhibiting NF-κB was shown to reduce inhibitory avoidance and memory reconsolidation in mice (Freudenthal et al., 2005; Merlo and Romano, 2008). These studies implied that the NF-κB is involved in mediating morphine-CPP. On the other hand, we found that melatonin treatment reverses the upregulation of NF-κB mRNA expression associated with morphine treatment. Similarly, exploring melatonin effect on D-galactose-induced memory impairment, which was associated with the elevated expression level of NF-κB in the cortex, reverse D-galactose induced memory impairment and neuroinflammation (Ali et al., 2015). Therefore, the NF-κB may act as a contributory factor in the neuronal reward mediating the effects of morphine in CPP. Our results suggest that melatonin administration modulates morphine-induced CPP, which was associated with reversing NF-κB expression in NAc. This effect is more likely due to the anti-inflammatory properties of melatonin to reduce NF-κB and several pro-inflammatory cytokines and inflammatory mediators (Beni et al., 2004; Li et al., 2005).
On the other hand, in this study, morphine was associated with a lower mRNA expression level of CREB. Similarly, several reports have suggested morphine-induced preference in CPP was associated with lower expression levels of CREB in the NAc (Zhou and Zhu, 2006; Chen et al., 2012) and ventral tegmental area (Rezai et al., 2018). Furthermore, several studies have shown that CREB is involved in morphine addiction (Yin and Tully, 1996; Walton and Dragunow, 2000; Martin et al., 2009). In fact, it has been found that morphine is associated with reduced CREB levels in the NAc (Moron et al., 2010; Tenayuca and Nazarian, 2012), suggesting that morphine behavioral adaptation is connected to CREB. Also, several studies have reported that morphine can suppress BDNF signaling in the NAc, which was associated with reducing inhibitory GABAergic and enhanced morphine reward (Koo et al., 2014). Importantly, in this study, melatonin treatment reverses morphine associated lower CREB mRNA expression in NAc. Similarly, melatonin treatment was shown to provide a potent antioxidant and neuroprotectant effect against Polychlorinated Biphenyls (PCBs) treated rats as a model of neurotoxicity with lower CREB gene expression. Thus, melatonin treatment attenuated PCBs effect and elevated CREB gene expression cerebral cortex in these rats (Bavithra et al., 2015). Therefore, our results suggest that the modulatory effect of melatonin attenuating morphine-induced CPP could be partly due to melatonin inducing cellular changes in CREB expression level in the NAc.
This study examined the effect of morphine on lowering mRNA expression level of BDNF. It has been reported that repeated morphine administration can lower BDNF expression in the ventral tegmental area (Koo et al., 2012). A similar finding was also reported by Rezai et al. (2018) after repeated morphine treatment was associated with lower BDNF levels in the ventral tegmental area. Consequently, resulting in attenuating BDNF signaling from the ventral tegmental area to the NAc and participating in morphine rewarding circulatory. In addition, it has been reported that TrκB receptor antagonist’s (BDNF receptor antagonist) systemic injection facilitates morphine dependency and withdrawal effects in rats (Rezamohammadi et al., 2020). In our study, we reported that melatonin treatment reversed morphine associated lower BDNF mRNA expression in NAc. In fact, it has been reported that melatonin treatment attenuated methamphetamine-induced downregulation of BDNF expression levels in mice (Veschsanit et al., 2021). Thus, the ability of melatonin treatment to attenuate morphine-induced CPP could be described through modulating these targets in the NAc.
Moreover, other behavioral parameters such as distance traveled, resting time, ambulatory count, and total activity count were evaluated to provide a more precise idea of animal behavior during the post-test. The results showed that morphine-induced CPP and melatonin treatment did not significantly affect these parameters in the post-test. This is in agreement with previous studies showing that the morphine-induced CPP does not affect locomotion or distance traveled (Farzinpour et al., 2019). In fact, it is essential to understand that melatonin did not affect animal locomotion or induce any behavior that may have a confounding effect, such as sedation or an aversion effect. In fact, animals that received repeated doses of melatonin did not show any significant behavioral changes when evaluated for CPP score, distance traveled, resting time, ambulatory count, and total activity count.
This study was intended to investigate the effect of melatonin on morphine-induced CPP and the effect of melatonin and morphine on the expression of GLT-1, BDNF, NF-κB, and CREB within the NAc brain region. It is essential to note that the changes in the expression of these targets are not only limited to morphine-induced CPP. In fact, It has been suggested that sucrose withdrawal after long-term exposure is associated with the inactivation of CREB in NAc (Kim et al., 2018). Also, repeated variable stress was shown to enhance nicotine-seeking behavior and decreased CREB in the NAc (Leao et al., 2012). Also, cumulative studies have linked reduction of GLT-1 expression to several drugs reward and relpase such as methamphetamine (Siemsen et al., 2019), cocaine (Trantham-Davidson et al., 2012), and alcohol (Das et al., 2015). Thus, giving the GLT-1 in NAc a common target for multiple drugs of abuse. Future studies are warranted to investigate GLT-1, BDNF, NF-κB, and CREB and assess their function in natural reward of other compounds and their involvement in other brain areas.
There is an increasing need to investigate and understand the molecular effects of morphine addiction and explore potential targets and possible treatments. Glutamatergic excitotoxicity, neuroinflammation, and glutamate clearance dysfunction are important therapeutic targets for morphine addiction. Thus, we investigated the effect of melatonin on morphine-induced place preference using the CPP paradigm. Melatonin treatment before morphine administration successfully attenuated morphine-induced CPP. This was associated with reversing the morphine-induced changes in GLT-1, NF-κB, BDNF, and CREB expression in the NAc brain region. This shows that melatonin blocking effect against morphine-induced CPP could be through modulating glutamate transporter, neurotrophins, and neuroinflammatory targets.
The original contributions presented in the study are included in the article, further inquiries can be directed to the corresponding author.
The animal study was reviewed and approved by the Animal Care and Use Committee (ACUC) guidelines of the King Fahd Medical Research Center. In addition, the experiments were approved by the Biomedical Ethics Research Committee (Reference 405-20) at King Abdulaziz University, following the guidelines of ethics and research on living creatures, prepared by the King Abdulaziz City for Science and Technology (KACST), approved by Royal Decree No. M/59 on 24 August 2010.
BA and FA worked on conceptualization, methodology, validation, data analysis, investigation, writing, reviewing, and editing the study. All authors contributed to the article and approved the submitted version.
This project was funded by the Deanship of Scientific Research at King Abdulaziz University, Jeddah, under grant no. G: 621-140-1441.
The authors declare that the research was conducted in the absence of any commercial or financial relationships that could be construed as a potential conflict of interest.
All claims expressed in this article are solely those of the authors and do not necessarily represent those of their affiliated organizations, or those of the publisher, the editors and the reviewers. Any product that may be evaluated in this article, or claim that may be made by its manufacturer, is not guaranteed or endorsed by the publisher.
We thank the Deanship of Scientific Research for their technical and financial support. Special thanks to the Pre-Clinical Research Unit at the King Fahd Medical Research Center for providing the facilities for this work.
NAc, nucleus accumbens; GLT-1, glutamate transporter-1; CREB, cAMP response element-binding protein; BDNF, brain-derived neurotrophic factor.
Ali, T., Badshah, H., Kim, T. H., and Kim, M. O. (2015). Melatonin attenuates D-galactose-induced memory impairment, neuroinflammation and neurodegeneration via RAGE/NF-K B/JNK signaling pathway in aging mouse model. J. Pineal Res. 58, 71–85. doi: 10.1111/jpi.12194
Allan, L., Hays, H., Jensen, N. H., de Waroux, B. L., Bolt, M., Donald, R., et al. (2001). Randomised crossover trial of transdermal fentanyl and sustained release oral morphine for treating chronic non-cancer pain. BSMJ 322, 1154–1158. doi: 10.1136/bmj.322.7295.1154
Alshehri, F. S., Hakami, A. Y., Althobaiti, Y. S., and Sari, Y. (2018). Effects of ceftriaxone on hydrocodone seeking behavior and glial glutamate transporters in P rats. Behav. Brain Res. 347, 368–376. doi: 10.1016/j.bbr.2018.03.043
Assar, N., Mahmoudi, D., Farhoudian, A., Farhadi, M. H., Fatahi, Z., and Haghparast, A. (2016). D1- and D2-like dopamine receptors in the CA1 region of the hippocampus are involved in the acquisition and reinstatement of morphine-induced conditioned place preference. Behav. Brain Res. 312, 394–404. doi: 10.1016/j.bbr.2016.06.061
Baharlouei, N., Sarihi, A., Komaki, A., Shahidi, S., and Haghparast, A. (2015). Blockage of acquisition and expression of morphine-induced conditioned place preference in rats due to activation of glutamate receptors type II/III in nucleus accumbens. Pharmacol. Biochem. Behav. 135, 192–198. doi: 10.1016/j.pbb.2015.06.004
Ballantyne, J. C. (2018). The brain on opioids. Pain 1, S24–S30. doi: 10.1097/j.pain.0000000000001270
Bamford, N. S., Robinson, S., Palmiter, R. D., Joyce, J. A., Moore, C., and Meshul, C. K. (2004). Dopamine modulates release from corticostriatal terminals. J Neurosci 24, 9541–9552. doi: 10.1523/JNEUROSCI.2891-04.2004
Barbosa-Mendez, S., Perez-Sanchez, G., Becerril-Villanueva, E., and Salazar-Juarez, A. (2021). Melatonin decreases cocaine-induced locomotor sensitization and cocaine-conditioned place preference in rats. J. Psychiatr. Res. 132, 97–110. doi: 10.1016/j.jpsychires.2020.09.027
Barbosa-Mendez, S., and Salazar-Juarez, A. (2020). Melatonin decreases cocaine-induced locomotor activity in pinealectomized rats. Braz. J. Psychiatry 42, 295–308. doi: 10.1590/1516-4446-2018-0400
Bardo, M. T., and Bevins, R. A. (2000). Conditioned place preference: what does it add to our preclinical understanding of drug reward? Psychopharmacology (Berl) 153, 31–43. doi: 10.1007/s002130000569
Bavithra, S., Sugantha Priya, E., Selvakumar, K., Krishnamoorthy, G., and Arunakaran, J. (2015). Effect of melatonin on glutamate: BDNF signaling in the cerebral cortex of polychlorinated biphenyls (PCBs)-exposed adult male rats. Neurochem. Res. 40, 1858–1869. doi: 10.1007/s11064-015-1677-z
Beni, S. M., Kohen, R., Reiter, R. J., Tan, D. X., and Shohami, E. (2004). Melatonin-induced neuroprotection after closed head injury is associated with increased brain antioxidants and attenuated late-phase activation of NF-kappaB and AP-1. FASEB J. 18, 149–151. doi: 10.1096/fj.03-0323fje
Bespalov, A. Y., and Zvartau, E. E. (1996). Intraaccumbens administration of NMDA receptor antagonist (+/–)-CPP prevents locomotor activation conditioned by morphine and amphetamine in rats. Pharmacol. Biochem. Behav. 55, 203–207. doi: 10.1016/s0091-3057(96)00065-2
Brown, T. M., Brotchie, J. M., and Fitzjohn, S. M. (2003). Cannabinoids decrease corticostriatal synaptic transmission via an effect on glutamate uptake. J. Neurosci. 23, 11073–11077. doi: 10.1523/JNEUROSCI.23-35-11073.2003
Calabresi, P., Picconi, B., Tozzi, A., and Di Filippo, M. (2007). Dopamine-mediated regulation of corticostriatal synaptic plasticity. Trends Neurosci. 30, 211–219. doi: 10.1016/j.tins.2007.03.001
Charkhpour, M., Ghavimi, H., Ghanbarzadeh, S., Yousefi, B., Khorrami, A., Mesgari, M., et al. (2015). Protective effect of pioglitazone on morphine-induced neuroinflammation in the rat lumbar spinal cord. J. Biomed. Sci. 22:82. doi: 10.1186/s12929-015-0187-2
Chen, S. L., Tao, P. L., Chu, C. H., Chen, S. H., Wu, H. E., Tseng, L. F., et al. (2012). Low-dose memantine attenuated morphine addictive behavior through its anti-inflammation and neurotrophic effects in rats. J. Neuroimmune Pharmacol. 7, 444–453. doi: 10.1007/s11481-011-9337-9
Claustrat, B., and Leston, J. (2015). Melatonin: physiological effects in humans. Neurochirurgie 61, 77–84. doi: 10.1016/j.neuchi.2015.03.002
Clough, S. J., Hutchinson, A. J., Hudson, R. L., and Dubocovich, M. L. (2014). Genetic deletion of the MT1 or MT2 melatonin receptors abrogates methamphetamine-induced reward in C3H/HeN mice. Physiol. Behav. 132, 79–86. doi: 10.1016/j.physbeh.2014.04.049
Compton, W. M. 3rd, Cottler, L. B., Ben Abdallah, A., Phelps, D. L., Spitznagel, E. L., and Horton, J. C. (2000). Substance dependence and other psychiatric disorders among drug dependent subjects: race and gender correlates. Am. J. Addict. 9, 113–125. doi: 10.1080/10550490050173181
Corre, J., van Zessen, R., Loureiro, M., Patriarchi, T., Tian, L., Pascoli, V., et al. (2018). Dopamine neurons projecting to medial shell of the nucleus accumbens drive heroin reinforcement. eLife 7:e39945. doi: 10.7554/eLife.39945
Crespi, F. (2012). Influence of melatonin or its antagonism on alcohol consumption in ethanol drinking rats: a behavioral and in vivo voltammetric study. Brain Res. 1452, 39–46. doi: 10.1016/j.brainres.2011.10.050
Curran, H. V., Kleckham, J., Bearn, J., Strang, J., and Wanigaratne, S. (2001). Effects of methadone on cognition, mood and craving in detoxifying opiate addicts: a dose-response study. Psychopharmacology (Berl) 154, 153–160. doi: 10.1007/s002130000628
Danbolt, N. C. (2001). Glutamate uptake. Prog. Neurobiol. 65, 1–105. doi: 10.1016/s0301-0082(00)00067-8
Das, S. C., Yamamoto, B. K., Hristov, A. M., and Sari, Y. (2015). Ceftriaxone attenuates ethanol drinking and restores extracellular glutamate concentration through normalization of GLT-1 in nucleus accumbens of male alcohol-preferring rats. Neuropharmacology 97, 67–74. doi: 10.1016/j.neuropharm.2015.05.009
Day, J. J., and Carelli, R. M. (2007). The nucleus accumbens and Pavlovian reward learning. Neuroscientist 13, 148–159. doi: 10.1177/1073858406295854
Deng, Y., Xu, Z.-F., Liu, W., Xu, B., Yang, H.-B., and Wei, Y.-G. (2012). Riluzole-triggered GSH synthesis via activation of glutamate transporters to antagonize methylmercury-induced oxidative stress in rat cerebral cortex. Oxid. Med. Cell. Longev. 2012:534705. doi: 10.1155/2012/534705
Desjardins, S., Belkai, E., Crete, D., Cordonnier, L., Scherrmann, J. M., Noble, F., et al. (2008). Effects of chronic morphine and morphine withdrawal on gene expression in rat peripheral blood mononuclear cells. Neuropharmacology 55, 1347–1354. doi: 10.1016/j.neuropharm.2008.08.027
Dodd, P. R., Beckmann, A. M., Davidson, M. S., and Wilce, P. A. (2000). Glutamate-mediated transmission, alcohol and alcoholism. Neurochem. Int. 37, 509–533. doi: 10.1016/s0197-0186(00)00061-9
Engeli, E. J. E., Zoelch, N., Hock, A., Nordt, C., Hulka, L. M., Kirschner, M., et al. (2020). Impaired glutamate homeostasis in the nucleus accumbens in human cocaine addiction. Mol. Psychiatry doi: 10.1038/s41380-020-0828-z [Online ahead of Print].
Espinar, A., Garcia-Oliva, A., Isorna, E. M., Quesada, A., Prada, F. A., and Guerrero, J. M. (2000). Neuroprotection by melatonin from glutamate-induced excitotoxicity during development of the cerebellum in the chick embryo. J. Pineal Res. 28, 81–88. doi: 10.1034/j.1600-079x.2001.280203.x
Farzinpour, Z., Taslimi, Z., Azizbeigi, R., Karimi-Haghighi, S., and Haghparast, A. (2019). Involvement of orexinergic receptors in the nucleus accumbens, in the effect of forced swim stress on the reinstatement of morphine seeking behaviors. Behav. Brain Res. 356, 279–287. doi: 10.1016/j.bbr.2018.08.021
Fatahi, Z., Zeinaddini-Meymand, A., Karimi, S., Khodagholi, F., and Haghparast, A. (2020). Impairment of cost-benefit decision making in morphine-dependent rats is partly mediated via the alteration of BDNF and p-CREB levels in the nucleus accumbens. Pharmacol. Biochem. Behav. 194:172952. doi: 10.1016/j.pbb.2020.172952
Francès, H., Le Foll, B., Diaz, J., Smirnova, M., and Sokoloff, P. (2004). Role of DRD3 in morphine-induced conditioned place preference using drd3-knockout mice. Neuroreport 15, 2245–2249. doi: 10.1097/00001756-200410050-00021
Frances, H., Smirnova, M., Leriche, L., and Sokoloff, P. (2004). Dopamine D3 receptor ligands modulate the acquisition of morphine-conditioned place preference. Psychopharmacology (Berl) 175, 127–133. doi: 10.1007/s00213-004-1807-9
French, S. J., and Totterdell, S. (2002). Hippocampal and prefrontal cortical inputs monosynaptically converge with individual projection neurons of the nucleus accumbens. J. Comp. Neurol. 446, 151–165. doi: 10.1002/cne.10191
Freudenthal, R., Boccia, M. M., Acosta, G. B., Blake, M. G., Merlo, E., Baratti, C. M., et al. (2005). NF-κB transcription factor is required for inhibitory avoidance long-term memory in mice. Eur. J. Neurosci. 21, 2845–2852. doi: 10.1111/j.1460-9568.2005.04126.x
Fujio, M., Nakagawa, T., Sekiya, Y., Ozawa, T., Suzuki, Y., Minami, M., et al. (2005). Gene transfer of GLT-1, a glutamate transporter, into the nucleus accumbens shell attenuates methamphetamine- and morphine-induced conditioned place preference in rats. Eur. J. Neurosci. 22, 2744–2754. doi: 10.1111/j.1460-9568.2005.04467.x
Fundytus, M. E. (2001). Glutamate receptors and nociception: implications for the drug treatment of pain. CNS Drugs 15, 29–58. doi: 10.2165/00023210-200115010-00004
Gleich, T., Deserno, L., Lorenz, R. C., Boehme, R., Pankow, A., Buchert, R., et al. (2015). Prefrontal and striatal glutamate differently relate to striatal dopamine: potential regulatory mechanisms of striatal presynaptic dopamine function? J. Neurosci. 35, 9615–9621. doi: 10.1523/JNEUROSCI.0329-15.2015
Gold, B. P., Mas-Herrero, E., Zeighami, Y., Benovoy, M., Dagher, A., and Zatorre, R. J. (2019). Musical reward prediction errors engage the nucleus accumbens and motivate learning. Proc. Natl. Acad. Sci. U S A 116, 3310–3315. doi: 10.1073/pnas.1809855116
Grewer, C., Gameiro, A., and Rauen, T. (2014). SLC1 glutamate transporters. Pflugers Arch. 466, 3–24. doi: 10.1007/s00424-013-1397-7
Haghparast, A., Gheitasi, I. P., and Lashgari, R. (2007). Involvement of glutamatergic receptors in the nucleus cuneiformis in modulating morphine-induced antinociception in rats. Eur. J. Pain 11, 855–862. doi: 10.1016/j.ejpain.2006.12.010
Hammad, A. M., Alasmari, F., Althobaiti, Y. S., and Sari, Y. (2017). Modulatory effects of ampicillin/sulbactam on glial glutamate transporters and metabotropic glutamate receptor 1 as well as reinstatement to cocaine-seeking behavior. Behav Brain Res 332, 288–298. doi: 10.1016/j.bbr.2017.06.017
Hao, S., Liu, S., Zheng, X., Zheng, W., Ouyang, H., Mata, M., et al. (2011). The role of TNFalpha in the periaqueductal gray during naloxone-precipitated morphine withdrawal in rats. Neuropsychopharmacology 36, 664–676. doi: 10.1038/npp.2010.197
Hao, Y., Yang, J. Y., Guo, M., Wu, C. F., and Wu, M. F. (2005). Morphine decreases extracellular levels of glutamate in the anterior cingulate cortex: an in vivo microdialysis study in freely moving rats. Brain Res 1040, 191–196. doi: 10.1016/j.brainres.2005.01.072
Hardeland, R., Pandi-Perumal, S. R., and Cardinali, D. P. (2006). Melatonin. Int. J. Biochem. Cell. Biol. 38, 313–316. doi: 10.1016/j.biocel.2005.08.020
Hemby, S. E. (2004). Morphine-induced alterations in gene expression of calbindin immunopositive neurons in nucleus accumbens shell and core. Neuroscience 126, 689–703. doi: 10.1016/j.neuroscience.2004.01.056
Jacobs, E. H., Wardeh, G., Smit, A. B., and Schoffelmeer, A. N. (2005). Morphine causes a delayed increase in glutamate receptor functioning in the nucleus accumbens core. Eur. J. Pharmacol. 511, 27–30. doi: 10.1016/j.ejphar.2005.02.009
Jia, X. F., Ji, Y., Huang, G. P., Zhou, Y., and Long, M. (2017). Comparison of intrathecal and local infiltration analgesia by morphine for pain management in total knee and hip arthroplasty: a meta-analysis of randomized controlled trial. Int. J. Surg. 40, 97–108. doi: 10.1016/j.ijsu.2017.02.060
Kalivas, P. W. (2004). Glutamate systems in cocaine addiction. Curr. Opin. Pharmacol. 4, 23–29. doi: 10.1016/j.coph.2003.11.002
Kalivas, P. W., Volkow, N., and Seamans, J. (2005). Unmanageable motivation in addiction: a pathology in prefrontal-accumbens glutamate transmission. Neuron 45, 647–650. doi: 10.1016/j.neuron.2005.02.005
Kaltschmidt, B., and Kaltschmidt, C. (2009). NF-kappaB in the nervous system. Cold Spring Harb. Perspect. Biol. 1:a001271. doi: 10.1101/cshperspect.a001271
Kim, J., Lee, S., Kang, S., Jeon, T. I., Kang, M. J., Lee, T. H., et al. (2018). Regulator of G-protein signaling 4 (RGS4) controls morphine reward by glutamate receptor activation in the nucleus accumbens of mouse brain. Mol. Cells 41, 454–464. doi: 10.14348/molcells.2018.0023
Kim, S., Shou, J., Abera, S., and Ziff, E. B. (2018). Sucrose withdrawal induces depression and anxiety-like behavior by Kir2.1 upregulation in the nucleus accumbens. Neuropharmacology 130, 10–17. doi: 10.1016/j.neuropharm.2017.11.041
Kobrin, K. L., Moody, O., Arena, D. T., Moore, C. F., Heinrichs, S. C., and Kaplan, G. B. (2016). Acquisition of morphine conditioned place preference increases the dendritic complexity of nucleus accumbens core neurons. Addict. Biol. 21, 1086–1096. doi: 10.1111/adb.12273
Koo, J. W., Lobo, M. K., Chaudhury, D., Labonte, B., Friedman, A., Heller, E., et al. (2014). Loss of BDNF signaling in D1R-expressing NAc neurons enhances morphine reward by reducing GABA inhibition. Neuropsychopharmacology 39, 2646–2653. doi: 10.1038/npp.2014.118
Koo, J. W., Mazei-Robison, M. S., Chaudhury, D., Juarez, B., LaPlant, Q., Ferguson, D., et al. (2012). BDNF is a negative modulator of morphine action. Science 338, 124–128. doi: 10.1126/science.1222265
Lambe, E. K., Picciotto, M. R., and Aghajanian, G. K. (2003). Nicotine induces glutamate release from thalamocortical terminals in prefrontal cortex. Neuropsychopharmacology 28, 216–225. doi: 10.1038/sj.npp.1300032
LaLumiere, R. T., and Kalivas, P. W. (2008). Glutamate release in the nucleus accumbens core is necessary for heroin seeking. J. Neurosci. 28, 3170–3177. doi: 10.1523/JNEUROSCI.5129-07.2008
Leao, R. M., Cruz, F. C., Marin, M. T., and Planeta Cda, S. (2012). Stress induces behavioral sensitization, increases nicotine-seeking behavior and leads to a decrease of CREB in the nucleus accumbens. Pharmacol. Biochem. Behav. 101, 434–442. doi: 10.1016/j.pbb.2012.01.025
Leone, P., Pocock, D., and Wise, R. A. (1991). Morphine-dopamine interaction: ventral tegmental morphine increases nucleus accumbens dopamine release. Pharmacol. Biochem. Behav. 39, 469–472. doi: 10.1016/0091-3057(91)90210-s
Li, J. H., Yu, J. P., Yu, H. G., Xu, X. M., Yu, L. L., Liu, J., et al. (2005). Melatonin reduces inflammatory injury through inhibiting NF-kappaB activation in rats with colitis. Mediators Inflamm. 2005, 185–193. doi: 10.1155/MI.2005.185
Lim, G., Wang, S., and Mao, J. (2005). cAMP and protein kinase a contribute to the downregulation of spinal glutamate transporters after chronic morphine. Neurosci. Lett. 376, 9–13. doi: 10.1016/j.neulet.2004.11.016
Lima, A. C. P., Louzada, P. R., De Mello, F. G., and Ferreira, S. T. (2003). Neuroprotection against Aβ and glutamate toxicity by melatonin: are GABA receptors involved? Neurotox. Res. 5, 323–327. doi: 10.1007/BF03033152
Liu, T., Zhang, L., Joo, D., and Sun, S. C. (2017). NF-kappaB signaling in inflammation. Signal Transduct. Target Ther. 2:17023. doi: 10.1038/sigtrans.2017.23
Livak, K. J., and Schmittgen, T. D. (2001). Analysis of relative gene expression data using real-time quantitative PCR and the 2−ΔΔCT method. Methods 25, 402–408. doi: 10.1006/meth.2001.1262
Ma, Q., Reiter, R. J., and Chen, Y. (2020). Role of melatonin in controlling angiogenesis under physiological and pathological conditions. Angiogenesis 23, 91–104. doi: 10.1007/s10456-019-09689-7
Mahshidfar, B., Mofidi, M., Fattahi, M., Farsi, D., Hafezi Moghadam, P., Abbasi, S., et al. (2017). Acute pain management in emergency department, low dose ketamine versus morphine, a randomized clinical trial. Anesth. Pain Med. 7:e60561. doi: 10.5812/aapm.60561
Manzanedo, C., Aguilar, M. A., Rodriguez-Arias, M., and Minarro, J. (2001). Effects of dopamine antagonists with different receptor blockade profiles on morphine-induced place preference in male mice. Behav. Brain Res. 121, 189–197. doi: 10.1016/s0166-4328(01)00164-4
Mao, J., Sung, B., Ji, R. R., and Lim, G. (2002). Chronic morphine induces downregulation of spinal glutamate transporters: implications in morphine tolerance and abnormal pain sensitivity. J. Neurosci. 22, 8312–8323. doi: 10.1523/JNEUROSCI.22-18-08312.2002
Martin, F., Laorden, M. L., and Milanes, M. V. (2009). Morphine withdrawal regulates phosphorylation of cAMP response element binding protein (CREB) through PKC in the nucleus tractus solitarius-A2 catecholaminergic neurons. J. Neurochem. 110, 1422–1432. doi: 10.1111/j.1471-4159.2009.06234.x
Meepong, R., and Sooksawate, T. (2019). Mitragynine reduced morphine-induced conditioned place preference and withdrawal in rodents. Thai J. Pharmaceutical Sci. (TJPS) 43, 21–29. Available online at: www.tjps.pharm.chula.ac.th/ojs/index.php/tjps/article/view/892
Merlo, E., and Romano, A. (2008). Memory extinction entails the inhibition of the transcription factor NF-kappaB. PLoS One 3:e3687. doi: 10.1371/journal.pone.0003687
Moron, J. A., Gullapalli, S., Taylor, C., Gupta, A., Gomes, I., and Devi, L. A. (2010). Modulation of opiate-related signaling molecules in morphine-dependent conditioned behavior: conditioned place preference to morphine induces CREB phosphorylation. Neuropsychopharmacology 35, 955–966. doi: 10.1038/npp.2009.199
Motaghinejad, M., Ebrahimzadeh, A., and Shabab, B. (2014). Preventive effect of central administration of venlafaxine on morphine physical dependence, nociception and blood cortisol level in rat. Int. J. Prev. Med. 5, 1422–1431. Available online at: www.ncbi.nlm.nih.gov/pmc/articles/PMC4274549/.
Nennig, S. E., and Schank, J. R. (2017). The Role of NFKB in Drug Addiction: Beyond Inflammation. Alcohol Alcohol 52, 172–179. doi: 10.1093/alcalc/agw098
Onaolapo, O. J., and Onaolapo, A. Y. (2018). Melatonin in drug addiction and addiction management: exploring an evolving multidimensional relationship. World J. Psychiatry 8, 64–74. doi: 10.5498/wjp.v8.i2.64
Ozawa, T., Nakagawa, T., Shige, K., Minami, M., and Satoh, M. (2001). Changes in the expression of glial glutamate transporters in the rat brain accompanied with morphine dependence and naloxone-precipitated withdrawal. Brain Res. 905, 254–258. doi: 10.1016/s0006-8993(01)02536-7
Pandi-Perumal, S. R., Trakht, I., Srinivasan, V., Spence, D. W., Maestroni, G. J., Zisapel, N., et al. (2008). Physiological effects of melatonin: role of melatonin receptors and signal transduction pathways. Prog. Neurobiol. 85, 335–353. doi: 10.1016/j.pneurobio.2008.04.001
Paxinos, G., and Watson, C. (2006). The Rat Brain in Stereotaxic Coordinates. Cambridge, MA: Academic Press, Elsevier.
Peres, R., do Amaral, F. G., Madrigrano, T. C., Scialfa, J. H., Bordin, S., Afeche, S. C., et al. (2011). Ethanol consumption and pineal melatonin daily profile in rats. Addict. Biol. 16, 580–590. doi: 10.1111/j.1369-1600.2011.00342.x
Piantadosi, P. T., Yeates, D. C. M., Wilkins, M., and Floresco, S. B. (2017). Contributions of basolateral amygdala and nucleus accumbens subregions to mediating motivational conflict during punished reward-seeking. Neurobiol. Learn. Mem. 140, 92–105. doi: 10.1016/j.nlm.2017.02.017
Polomano, R. C., Rathmell, J. P., Krenzischek, D. A., and Dunwoody, C. J. (2008). Emerging trends and new approaches to acute pain management. J. Perianesth. Nurs. 23, S43–S53. doi: 10.1016/j.jopan.2007.11.006
Rezai, M., Mahmoodi, M., Kaeidi, A., Karimabad, M. N., Khoshdel, A., and Hajizadeh, M. R. (2018). Effect of crocin carotenoid on BDNF and CREB gene expression in brain ventral tegmental area of morphine treated rats. Asian Pacific J. Trop. Biomed. 8, 387–393. doi: 10.4103/2221-1691.239426
Rezamohammadi, F., Rahmani, M., Ghanbari, A., Khaleghian, A., and Miladi-Gorji, H. (2020). BDNF receptor antagonism during the induction of morphine dependence exacerbates the severity of physical dependence and ameliorates psychological dependence in rats. Neurosci. Lett. 737:135332. doi: 10.1016/j.neulet.2020.135332
Robinson, T. E., and Kolb, B. (1997). Persistent structural modifications in nucleus accumbens and prefrontal cortex neurons produced by previous experience with amphetamine. J. Neurosci. 17, 8491–8497. doi: 10.1523/JNEUROSCI.17-21-08491.1997
Roy, S., Cain, K. J., Chapin, R. B., Charboneau, R. G., and Barke, R. A. (1998). Morphine modulates NF kappa B activation in macrophages. Biochem. Biophys. Res. Commun. 245, 392–396. doi: 10.1006/bbrc.1998.8415
Roy, S., and Loh, H. H. (1996). Effects of opioids on the immune system. Neurochem. Res. 21, 1375–1386. doi: 10.1007/BF02532379
Sacerdote, P. (2006). Opioids and the immune system. Palliat. Med. 1, s9–s15. doi: 10.1191/0269216306pm1124oa
Sawaya, B. E., Deshmane, S. L., Mukerjee, R., Fan, S., and Khalili, K. (2009). TNF alpha production in morphine-treated human neural cells is NF-κB-dependent. J. Neuroimmune Pharmacol. 4, 140–149. doi: 10.1007/s11481-008-9137-z
Scofield, M. D., Heinsbroek, J. A., Gipson, C. D., Kupchik, Y. M., Spencer, S., Smith, A. C., et al. (2016). The nucleus accumbens: mechanisms of addiction across drug classes reflect the importance of glutamate homeostasis. Pharmacol. Rev. 68, 816–871. doi: 10.1124/pr.116.012484
Sepulveda, J., Oliva, P., and Contreras, E. (2004). Neurochemical changes of the extracellular concentrations of glutamate and aspartate in the nucleus accumbens of rats after chronic administration of morphine. Eur. J. Pharmacol. 483, 249–258. doi: 10.1016/j.ejphar.2003.10.037
Shen, Y., Cao, X., Shan, C., Dai, W., and Yuan, T. F. (2017). Heroin addiction impairs human cortical plasticity. Biol. Psychiatry 81, e49–e50. doi: 10.1016/j.biopsych.2016.06.013
Shen, C. H., Tsai, R. Y., and Wong, C. S. (2012). Role of neuroinflammation in morphine tolerance: effect of tumor necrosis factor-alpha. Acta Anaesthesiol Taiwan 50, 178–182. doi: 10.1016/j.aat.2012.12.004
Siemsen, B. M., Reichel, C. M., Leong, K. C., Garcia-Keller, C., Gipson, C. D., Spencer, S., et al. (2019). Effects of methamphetamine self-administration and extinction on astrocyte structure and function in the nucleus accumbens core. Neuroscience 406, 528–541. doi: 10.1016/j.neuroscience.2019.03.040
Sircar, R. (2000). Effect of melatonin on cocaine-induced behavioral sensitization. Brain Res. 857, 295–299. doi: 10.1016/s0006-8993(99)02460-9
Smith, J. W., Fetsko, L. A., Xu, R., and Wang, Y. (2002). Dopamine D2L receptor knockout mice display deficits in positive and negative reinforcing properties of morphine and in avoidance learning. Neuroscience 113, 755–765. doi: 10.1016/s0306-4522(02)00257-9
Soares-Cunha, C., de Vasconcelos, N. A., Coimbra, B., Domingues, A. V., Silva, J. M., Loureiro-Campos, E., et al. (2020). Nucleus accumbens medium spiny neurons subtypes signal both reward and aversion. Mol. Psychiatry 25, 3241–3255. doi: 10.1038/s41380-019-0484-3
Solinas, M., Belujon, P., Fernagut, P. O., Jaber, M., and Thiriet, N. (2019). Dopamine and addiction: what have we learned from 40 years of research. J. Neural Transm. (Vienna) 126, 481–516. doi: 10.1007/s00702-018-1957-2
Spiga, S., Puddu, M. C., Pisano, M., and Diana, M. (2005). Morphine withdrawal-induced morphological changes in the nucleus accumbens. Eur. J. Neurosci. 22, 2332–2340. doi: 10.1111/j.1460-9568.2005.04416.x
Sun, Y., Chen, G., Zhou, K., and Zhu, Y. (2018). A conditioned place preference protocol for measuring incubation of craving in rats. J. Vis. Exp. doi: 10.3791/58384
Swendsen, J. D., and Merikangas, K. R. (2000). The comorbidity of depression and substance use disorders. Clin. Psychol. Rev. 20, 173–189. doi: 10.1016/s0272-7358(99)00026-4
Takahashi, T. T., Vengeliene, V., and Spanagel, R. (2017). Melatonin reduces motivation for cocaine self-administration and prevents relapse-like behavior in rats. Psychopharmacology (Berl) 234, 1741–1748. doi: 10.1007/s00213-017-4576-y
Tenayuca, J. M., and Nazarian, A. (2012). Hydrocodone and morphine possess similar rewarding effects and reduce ERK and CREB phosphorylation in the nucleus accumbens. Synapse 66, 918–922. doi: 10.1002/syn.21577
Trantham-Davidson, H., LaLumiere, R. T., Reissner, K. J., Kalivas, P. W., and Knackstedt, L. A. (2012). Ceftriaxone normalizes nucleus accumbens synaptic transmission, glutamate transport and export following cocaine self-administration and extinction training. J. Neurosci. 32, 12406–12410. doi: 10.1523/JNEUROSCI.1976-12.2012
Tzschentke, T. M. (2007). Measuring reward with the conditioned place preference (CPP) paradigm: update of the last decade. Addict. Biol. 12, 227–462. doi: 10.1111/j.1369-1600.2007.00070.x
Vengeliene, V., Noori, H. R., and Spanagel, R. (2015). Activation of melatonin receptors reduces relapse-like alcohol consumption. Neuropsychopharmacology 40, 2897–2906. doi: 10.1038/npp.2015.143
Veschsanit, N., Yang, J. L., Ngampramuan, S., Viwatpinyo, K., Pinyomahakul, J., Lwin, T., et al. (2021). Melatonin reverts methamphetamine-induced learning and memory impairments and hippocampal alterations in mice. Life Sci. 265:118844. doi: 10.1016/j.lfs.2020.118844
Vishnoi, S., Raisuddin, S., and Parvez, S. (2016). Glutamate excitotoxicity and oxidative stress in epilepsy: modulatory role of melatonin. J. Environ. Pathol. Toxicol. Oncol. 35, 365–374. doi: 10.1615/JEnvironPatholToxicolOncol.2016016399
Walton, M. R., and Dragunow, M. (2000). Is CREB a key to neuronal survival? Trends Neurosci. 23, 48–53. doi: 10.1016/s0166-2236(99)01500-3
Xia, Y., Driscoll, J. R., Wilbrecht, L., Margolis, E. B., Fields, H. L., and Hjelmstad, G. O. (2011). Nucleus accumbens medium spiny neurons target non-dopaminergic neurons in the ventral tegmental area. J. Neurosci. 31, 7811–7816. doi: 10.1523/JNEUROSCI.1504-11.2011
Xia, S. H., Yu, J., Huang, X., Sesack, S. R., Huang, Y. H., Schluter, O. M., et al. (2020). Cortical and thalamic interaction with amygdala-to-accumbens synapses. J. Neurosci. 40, 7119–7132. doi: 10.1523/JNEUROSCI.1121-20.2020
Yin, J. C., and Tully, T. (1996). CREB and the formation of long-term memory. Curr. Opin. Neurobiol. 6, 264–268. doi: 10.1016/s0959-4388(96)80082-1
Yuan, K., Sheng, H., Song, J., Yang, L., Cui, D., Ma, Q., et al. (2017). Morphine treatment enhances glutamatergic input onto neurons of the nucleus accumbens via both disinhibitory and stimulating effect. Addict. Biol. 22, 1756–1767. doi: 10.1111/adb.12438
Zhang, X., Cui, Y., Jing, J., Cui, Y., Xin, W., and Liu, X. (2011). Involvement of p38/NF-kappaB signaling pathway in the nucleus accumbens in the rewarding effects of morphine in rats. Behav. Brain Res. 218, 184–189. doi: 10.1016/j.bbr.2010.11.049
Zhang, L. Y., Zhou, Y. Q., Yu, Z. P., Zhang, X. Q., Shi, J., and Shen, H. W. (2021). Restoring glutamate homeostasis in the nucleus accumbens via endocannabinoid-mimetic drug prevents relapse to cocaine seeking behavior in rats. Neuropsychopharmacology 46, 970–981. doi: 10.1038/s41386-021-00955-1
Zhou, L. F., and Zhu, Y. P. (2006). Changes of CREB in rat hippocampus, prefrontal cortex and nucleus accumbens during three phases of morphine induced conditioned place preference in rats. J. Zhejiang Univ. Sci. B 7, 107–113. doi: 10.1631/jzus.2006.B0107
Keywords: morphine, addiction, melatonin, GLT-1, BDNF, NF-κB, CREB
Citation: Alghamdi BS and Alshehri FS (2021) Melatonin Blocks Morphine-Induced Place Preference: Involvement of GLT-1, NF-κB, BDNF, and CREB in the Nucleus Accumbens. Front. Behav. Neurosci. 15:762297. doi: 10.3389/fnbeh.2021.762297
Received: 21 August 2021; Accepted: 28 September 2021;
Published: 14 October 2021.
Edited by:
Luis Miguel Tuesta, University of Miami, United StatesReviewed by:
Kathryn J. Reissner, University of North Carolina at Chapel Hill, United StatesCopyright © 2021 Alghamdi and Alshehri. This is an open-access article distributed under the terms of the Creative Commons Attribution License (CC BY). The use, distribution or reproduction in other forums is permitted, provided the original author(s) and the copyright owner(s) are credited and that the original publication in this journal is cited, in accordance with accepted academic practice. No use, distribution or reproduction is permitted which does not comply with theseterms.
*Correspondence: Badrah S. Alghamdi, YmFzYWxnaGFtZGlAa2F1LmVkdS5zYQ==
†ORCID: Badrah S. Alghamdi bWFpbHRvOm9yY2lkLm9yZy8wMDAwLTAwMDEtNjk2Ni0wMTI4 Fahad S. Alshehri b3JjaWQub3JnLzAwMDAtMDAwMS02OTY2LTAxMjg=
Disclaimer: All claims expressed in this article are solely those of the authors and do not necessarily represent those of their affiliated organizations, or those of the publisher, the editors and the reviewers. Any product that may be evaluated in this article or claim that may be made by its manufacturer is not guaranteed or endorsed by the publisher.
Research integrity at Frontiers
Learn more about the work of our research integrity team to safeguard the quality of each article we publish.