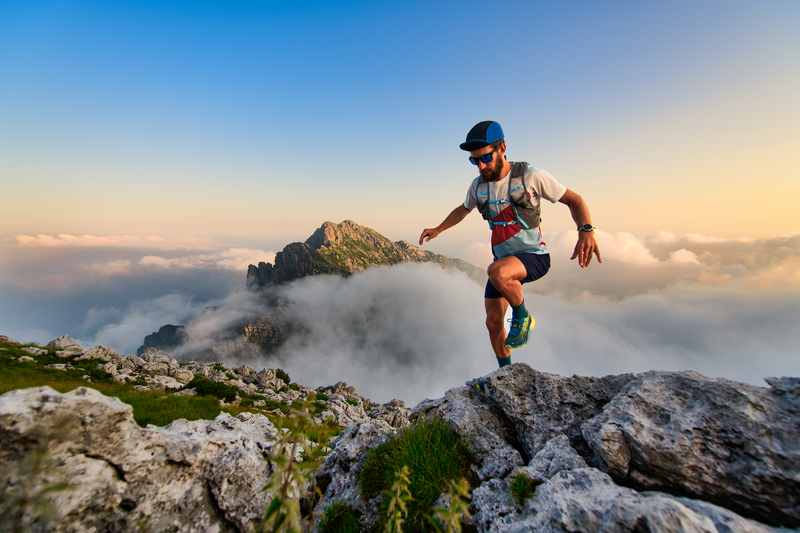
94% of researchers rate our articles as excellent or good
Learn more about the work of our research integrity team to safeguard the quality of each article we publish.
Find out more
ORIGINAL RESEARCH article
Front. Behav. Neurosci. , 23 November 2021
Sec. Learning and Memory
Volume 15 - 2021 | https://doi.org/10.3389/fnbeh.2021.748563
Little is known about the effects of methamphetamine (Meth) and buprenorphine (Bup) on memory and learning in rats. The aim of this investigation was to examine the impact of Meth and Bup on memory and learning. Fourteen male Wistar rats weighing 250–300 g were assigned to four groups: Sham, Meth, Bup, and Meth + Bup and were treated for 1 week. Spatial learning and memory, avoidance learning, and locomotion were assessed using the Morris water maze, passive avoidance learning, and open field tests, respectively. Meth and Bup impaired spatial learning and memory in rats. Co-administration of Meth + Bup did not increase the time spent in the target quadrant compared to Meth alone in the MWM. The Bup and Meh + Bup groups were found with an increase in step-through latency (STLr) and a decrease in the time spent in the dark compartment (TDC). Meth and Bup had no effects on locomotor activity in the open field test. Bup showed a beneficial effect on aversive memory. Since Bup demonstrates fewer side effects than other opioid drugs, it may be preferable for the treatment of avoidance memory deficits in patients with Meth addiction.
Methamphetamine (Meth) is an incredibly addictive psychostimulant with devastating effects on the central nervous system (Mori et al., 2006; Melo et al., 2012; Miladi-Gorji et al., 2015). The prevalence of Meth abuse, as an escalating public health issue (Melo et al., 2012), has increased dramatically, reaching epidemic proportions worldwide (Camarasa et al., 2010) over the last 20 years (Moenk and Matuszewich, 2012). The universality of this drug can be due to its low price and simple production in comparison with cocaine or heroin (Macúchová et al., 2013). Long-term Meth abuse is linked to ventricular hypertrophy, cardiomyopathies, premature atherosclerosis, and kidney failure (Melo et al., 2012). Due to the effects of Meth on neuronal excitability, short-term Meth can cause euphoria and increased alertness and also improve motor activity and awareness (Chen et al., 2012). Meth abuse is associated with significant health problems, including psychosis, psychomotor dysfunction, anxiety, and depression. Clinical investigations have identified impaired functions of the striatal and cortical systems (Krasnova et al., 2010), and several cognitive deficiencies after Meth abuse, even years of drug abstinence (Reichel et al., 2014), leading to deficits in attention, learning, episodic memory, information processing, working memory, decision-making, and impulse control. Meth also causes memory deficits concomitant with reducing hippocampal volume and deleterious structural changes within the hippocampus (Krasnova et al., 2010; Moenk and Matuszewich, 2012; Braren et al., 2014). It diminishes cognition (Melo et al., 2012) and causes significant impairment in the performance of hippocampus-dependent spatial tasks, such as the Morris water maze (MWM) (Vorhees et al., 2000; Ghazvini et al., 2016). Investigations on human fetuses exposed to Meth also have demonstrated cognitive deficits and malformations in different structures of the brain involved in learning (Moenk and Matuszewich, 2012). Some studies have shown that high doses of Meth impaired spatial learning and memory, while lower doses did not (Chen et al., 2012).
Opioids can change the activity of dopaminergic neurons and consequently, alter the pharmacodynamic effect of Meth on the dopaminergic system. It has been reported that the opioid system may be affected by the conditioned cues following Meth administration (Lelong-Boulouard et al., 2006).
Buprenorphine (Bup) is a semi-synthetic partial agonist of the μ-opioid receptor, which has antagonistic effects on the κ- and δ- opioid receptors. It also has poor euphoric impacts and a weak negative effect on respiration (Pournaghash and Riley, 1993; Lelong-Boulouard et al., 2006). Bup causes a limited degree of physical dependency; therefore, it produces better clinical outcomes than morphine and other related agonists (Glover and Davis, 2008). It has been reported that Bup attenuates Meth-induced self-injurious behavior (Mori et al., 2006). Moreover, μ-opioid receptor-mediated pathways may influence the genetic risk for Meth consumption and Bup interferes with the acquisition of Meth intake in mice, potentially due to Bup’s partial agonist activity (Eastwood and Phillips, 2014). Adding Bup to the matrix method resulted in a significant reduction in Meth cravings (Salehi et al., 2015).
Little is known on the effects of Bup on memory function and the reports in this regard are often controversial. The effect of Bup on the improvement or impairment of learning and memory has been evaluated using various tests, experimental species, and doses (Lelong-Boulouard et al., 2006). This investigation was done to examine the efficiency of Bup in inhibiting the learning and memory deficits caused by the administration of Meth in rats.
Adult male Wistar rats weighing 250–300 g were studied for the prevention of sex-dependent factors in the present investigation. The rats were randomly categorized into four groups (10 animals per group with computer-generated random numbers) and kept under 12-h dark/light cycles (lights on at 8:00 AM) in a temperature-controlled (22 ± 2°C) colony room. Three days before the tests, animals were housed in considered cages (one rat per cage). All rats had unrestricted access to tap water and food. The researchers responsible for testing the rats were blind to the treatment groups. The rats used in this investigation were treated according to the Guide for the Care and Use of Laboratory Animals and the investigation protocols were proved by the Institutional Animal Care and Use Committee of Hamadan University of Medical Sciences.
The doses of drugs were chosen based on the most of previous studies. Methamphetamine hydrochloride (received from the Presidency Drug Control Headquarters, Tehran, Iran) was dissolved in 0.9% saline (Polston et al., 2011; Melo et al., 2012) and administered at a dose of 2 mg/kg (Lee et al., 2011; Chiang et al., 2014; Miladi-Gorji et al., 2015; Etaee et al., 2017). Bup (FARAN Shimi, Iran) was dissolved in 0.9% saline (Wala and Holtman, 2011) and administered at the dose of 5 mg/kg (Martin et al., 2001; Thompson et al., 2006; Etaee et al., 2017).
The routes of drugs administration and the number of days for administration were chosen based on previous studies. The times for the administration of drugs before the tests were chosen based on the time to reach the peak plasma concentration. In this study, 40 rats were divided into the four following groups: Bup: Bup was administered intragastrically or by gavage (IG) (Martin et al., 2001; Thompson et al., 2006) at 5 mg/kg once a day for 7 days (Wala and Holtman, 2011); Meth: Meth was administered intraperitoneally (IP) (Chiang et al., 2014) at 2 mg/kg once a day (Chiang et al., 2014; Miladi-Gorji et al., 2015) for 7 days (Jia et al., 2008); Bup + Meth: Meth and Bup were administered once a day for 7 days; and Sham: Saline was given intragastrically (IG) once a day for 7 days. On the 7th day, Meth and Bup were given to the groups 30 (Schutová et al., 2009) and 60 (Wala and Holtman, 2011) min before testing, respectively.
The experimental timeline is demonstrated in Figure 1.
The rats’ learning and spatial memory were assessed using the MWM task, which consisted of a black-colored circular pool (60 cm in height, 180 cm in diameter), filled to a depth of 25 cm with water (22 ± 1°C) (Krasnova et al., 2010; Asadbegi et al., 2017). A dim light was applied to illuminate the area, and the room was soundproof. Several visual cues were present. The pool had four quadrants with four starting lines, named as the north, south, east, and west, and an invisible Plexiglas platform (10 cm in diameter) was positioned centrally 1 cm below the water’s surface in the northwest (target) quadrant (Camarasa et al., 2010).
The training was performed around the same time each day for 4 days, and two blocks of four trials (60 s) were performed per day. A 30-s gap separated the two trials and the rats were allowed to rest for 5 min between the sequential blocks (Jia et al., 2008). During the eight trials, each starting position was randomly used twice. Facing the wall, the rat was positioned in the water at a starting point in one of the four quadrants. Afterward, each rat was allowed to swim in the pool for a period of 60 s to find the hidden platform in the target position. After mounting the platform, the animal was allowed to remain there for 20 s. Therefore, each swim trial took 60 s, the animal was left 20 s on the platform, and 30 s took before the animal was released again.
A video camera, which was positioned above the pool and directly connected to a computer, recorded the rats’ behaviors. The camera recorded the parameters, such as escape latency (the time spent to reach the hidden platform), traveled distance (the length of the swim path to find the hidden platform), and mean speed (the ratio of traveled distance to escape latency) in each trial (Zheng et al., 2002).
One day after the spatial acquisition phase (on day 5), in the retention phase, a probe trial was conducted, in which the platform was removed from the pool, and the rat was allowed to swim for 60 s while we recorded the time spent in the target quadrant (Zarrinkalam et al., 2018). The obtained data were analyzed using Maze Router homemade software.
In the passive avoidance test, the rats’ natural tendency for a dark environment is used. The device consisted of two chambers that each had a steel-rod grid floor (10 mm apart, 3 mm in diameter; Borj Sanat Co.). One of the chambers (20 × 20 × 30 cm) was provided with a 20 W lamp that was placed centrally at the height of 50 cm, while another chamber was dark with identical size. A guillotine gate (20 × 15 cm) connected the chambers. A dark room was used during the trial session. In the training trial, the guillotine door between the light and dark chambers was closed. When each animal was located in the light chamber with its back to the guillotine door, the door was opened and the time until the rat entered the dark chamber (step-through latency, STLa) was measured with a stopwatch. Then, when the rat entered the dark chamber, the door was closed (Barzegar et al., 2015).
This test lasted 2 days. On the initial day, all rats were habituated to the device. Each animal was located in the lighted chamber with its back to the guillotine door and 5 s later, the guillotine door was opened (Shiri et al., 2016). Upon entrance to the dark chamber, the door was closed, and the animal was taken from the dark chamber and transferred to the home cage. The habituation trial was replicated after 30 min. The acquisition trial was conducted 30 min later, in which the guillotine door was closed, and a constant current shock (50 Hz, 1.2 mA) (Moradkhani et al., 2015) was applied for 2 s (Khodamoradi et al., 2015; Ganji et al., 2017) immediately after the rat entered the dark chamber. The rat was remained in the device and experienced a foot shock every time it returned to the dark chamber. The training was stopped when the rat remained in the light chamber for 120 s. The number of trials until acquisition (entries into the dark chamber) was recorded. This training was conducted 6 days after drug administration (Motamedi et al., 2010; Zarrindast et al., 2013).
The retention experiment was conducted 24 h after the passive avoidance training, and the animal was located in the lighted chamber and 15 s later, the guillotine door was raised (Zarrinkalam et al., 2016). The latency to enter the dark chamber (step-through latency, STLr) and the time spent in the dark chamber (TDC) were recorded (Barzegar et al., 2015). This test was conducted 7 days after onset of drug administration (Jia et al., 2008).
Locomotor activity was measured using an open field apparatus, which was made of white acrylic with a size of 50 cm (length) × 50 cm (width) × 38 cm (height). Ambient, low-level, room lights lighted the field. An aloft video camera videotaped the time spent by the animal in the central and peripheral zones, and the data were analyzed with video-tracking software. The rats’ locomotor activity was examined to rule out the probability of differentiation in their baseline activity levels that influenced their performance. The rat was placed in the center of the trial chamber in the open field arena and could explore it for 10 min. The test was conducted for 10 min, 6 days after onset of drug administration (Hollais et al., 2014; Castel et al., 2018).
The analyses were done using the SPSS version 20.0 (SPSS, Chicago, IL, United States). Statistical significance regarding escape latency traveled distance, and speed in the MWM was assessed using two-way analysis of variance (ANOVA), followed by Bonferroni post hoc test. The statistical significance of the results of the open field and passive avoidance tasks and also the time spent in the target quadrant of MWM were compared by the one-way ANOVA, followed by a Tukey’s post hoc test. All results were displayed as the mean ± SEM. A P < 0.05 was considered statistically significant.
The results of two-way ANOVA showed a significant effect of the drug (P < 0.0001), day (P < 0.0001), but not their interaction (P = 0.21) on the escape latency between groups. Post-test comparisons demonstrated a significant increase in the escape latency after the administration of Meth and Bup or their co-administration in comparison with the Sham group. Our results indicated that the co-administration of Meth + Bup increased the escape latency in comparison with the Meth group on the fourth day of training (P < 0.01). It means that the Meth + Bup co-administration accentuated the deteriorating effect of Meth on spatial memory. Moreover, on the third day of training, co-administration of Meth + Bup increased the escape latency in comparison with the Bup group (P < 0.05). There were no significant differences between the other groups (P > 0.05) (Figure 2A).
Figure 2. The effect of Bup (5 mg/kg; IG) and Meth (2 mg/kg; IP) on the escape latency (A), traveled distance (B), and mean speed (C), in consecutive training days in the Morris water maze. *P < 0.05, **P < 0.01, ***P < 0.001, ****P < 0.0001: Sham vs. other three groups; #P < 0.05, ##P < 0.01, ###P < 0.001: Meth + Bup group vs. the Bup group; $$P < 0.01: Meth + Bup group vs. the Meth group.
Rats in the Sham group showed a significant decrease in the escape latency on the third and fourth days compared to the first day of training (one-way ANOVA; P < 0.05 and P < 0.01, respectively). In the Bup group, significant reductions in escape latency were observed between the first day of trials in comparison with the third and fourth days (P < 0.05). No significant changes were observed in the escape latency of the other groups during training days (P > 0.05). Therefore, except for the Sham and Bup groups, other groups did not learn the location of the platform during the training in the MWM (performance was roughly the same during training days).
The results of two-way ANOVA showed the significant effect of the drug (P < 0.0001), day (P = 0.0067), and their interaction (P = 0.0012) on the traveled distance. Post-test comparisons revealed a significant increase in the traveled distance after the administration of Meth and Bup and their co-administration in comparison with the Sham group. Our results indicated that the co-administration of Meth + Bup on the second day of training increased the traveled distance in comparison with the Bup group (P < 0.001). Co-administration of Meth + Bup did not lead to significant changes in the traveled distance in comparison with the Meth group (P > 0.05). There were not any significant differences between the other groups (P > 0.05) (Figure 2B).
Rats in the Sham group showed a significant decrease in the traveled distance during training days (one-way ANOVA; P < 0.001). There was a significant difference in the traveled distance between the first and the second days of training following the co-administration of Meth + Bup (one-way ANOVA; P < 0.05). There were no significant differences between other groups in the traveled distance during training days (P > 0.05).
The results of two-way ANOVA showed the significant effect of the drug (P < 0.001), drug and day interaction (P < 0.0001), but not day (P = 0.11) on mean speed. A significant difference was observed between the Meth and Sham groups on the fourth day of training in terms of mean speed (P < 0.01).
Meth + Bup administration decreased the mean speed in comparison with the Bup group on the second day of training (P < 0.001). No significant changes were observed between the other groups (P > 0.05 for all) (Figure 2C). There was a significant difference between the first and fourth days (P < 0.05). Also, no significant changes were observed between other trial days (P > 0.05 for all).
The effects of Bup, Meth, and Meth + Bup on the time spent in the target quadrant are shown in Figure 3. Tukey’s post hoc test revealed a significant reduction in the time spent in the target quadrant after the administration of Meth (mean ± SEM = 13.24 ± 1.25 s) in comparison with the Sham group (25.22 ± 2.58 s) (P < 0.001). Bup administration (16.95 ± 1.37 s) significantly reduced the time spent in the target quadrant in comparison with the Sham group (P < 0.05). Also, the co-administration of Meth + Bup (16.18 ± 1.09 s) decreased the time spent in the target quadrant in comparison with the Sham group (P < 0.01) (Figure 3).
Figure 3. The effect of Bup (5 mg/kg; IG) and Meth (2 mg/kg; IP) on the time spent in the target quadrant in absence of platform in the Morris water maze. Comparisons were made using one-way ANOVA, followed by Tukey’s post hoc test. This figure shows that spatial memory deteriorated in the Meth, Bup, and Meth + Bup groups in comparison with the Sham group. *P < 0.05: Bup group vs. the Sham group; **P < 0.01: Meth + Bup group vs. the Sham group; ***P < 0.001: Meth group vs. the Sham group.
There were no significant differences in the STLa between the Meth (28.63 ± 12.53 s), Bup (20.67 ± 6.86 s), Meth + Bup (78 ± 24.7 s), and Sham (15.4 ± 5.75 s) group (P > 0.05 for all) (Figure 4A).
Figure 4. The effect of Bup (5 mg/kg; IG) and Meth (2 mg/kg; IP) on step-through latency in the training test (STLa) (A), trials to acquisition (B), step-through latency in the retention test (STLr) (C), the time spent in the dark compartment (TDC) (D), and the number of entries into the dark compartment (E) in the passive avoidance test. *P < 0.05: Bup and Meth + Bup groups in comparison with the Sham and Meth groups; #P < 0.05: Meth + Bup group vs. the Meth group.
Regarding the number of entries into the dark compartment during acquisition and training, there were no significant differences between the Meth (1.38 ± 0.375), Bup (1.11 ± 0.11), Meth + Bup (1.14 ± 0.14), and Sham (1.1 ± 0.1) groups (P > 0.05 for all) (Figure 4B).
Rats in the Bup (206 ± 20.73 s) and Meth + Bup (185.71 ± 21.8 s) groups showed a significant increase in STLr in the retention test in comparison with the Sham group (133.9 ± 23.3 s) (P < 0.05 for both). Co-administration of Meth + Bup significantly increased the STLr in comparison with the Meth group (145.78 ± 21.45 s) (P < 0.05) (Figure 4C). STLr was increased in both Bup and Meth + Bup groups. Therefore, the increase in STLr in the Meth + Bup group may be attributed to the effect of Bup. It can be concluded that Bup showed a beneficial effect on aversive memory.
Animals in the Bup (21.78 ± 5.62 s) and Meth + Bup (34.14 ± 8.14 s) groups showed a significant decrease in TDC in comparison with the Sham group (87.1 ± 15.95 s) (P < 0.05 for both). Co-administration of Meth + Bup significantly decreased the TDC in comparison with the Meth group (72.33 ± 17.82 s) (P < 0.05; Figure 4D). Increased TDC in the Bup and Meth + Bup groups suggested and confirmed that Bup (not its co-administration with Meth) exerted a beneficial effect on avoidance memory.
Regarding the number of entries into the dark compartment, there were no significant differences between the Meth (2.11 ± 1.02), Bup (1 ± 0.5), Meth + Bup (4.43 ± 2.57), and sham (2.4 ± 0.77) groups (P > 0.05 for all) (Figure 4E).
Locomotor activity (traveled distance) was not significantly changed by the administration of Meth (16.28 ± 1.49), Bup (17.62 ± 1.16 m), or Meth + Bup (17.19 ± 1.15 m) in comparison with the sham group (17.70 ± 2.26 m) (P > 0.05) or between the groups (P > 0.05 for all) (Figure 5).
Figure 5. The effect of Bup (5 mg/kg; IG) and Meth (2 mg/kg; IP) on locomotor activity [traveled distance (m)] in the open field test. There were no significant differences between the Meth, Bup, and Meth + Bup groups and the Sham group.
The results of the current study indicated that Meth, Bup, and Meth + Bup increased the escape latency and traveled distance compared to the sham group, indicating deterioration in the spatial memory. Moreover, Bup decreased the escape latency on the third and fourth days compared to the first day of training. Thus, during training Bup administration alone had only a moderate effect on spatial impairment.
Also, STL and TDC were found to be decreased in the Bup and Meth + Bup groups, indicating an enhancement in the aversive memory. This observation suggests that Bup alone and in combination with Meth exert its positive effects on aversive memory. Therefore, the most interesting finding of the passive avoidance task was the effectiveness of Bup in the potentiation of aversive memory.
The role of Meth abuse in cognitive-related deterioration is not well known. Nevertheless, previous investigations have recommended that while the acute use of Meth may enhance memory and attention, chronic use results in reduced memory function (Seminerio et al., 2013). It has been reported that exposure to Meth during preadolescence improves spatial learning in male rats (Macúchová et al., 2013). Surprisingly, clinical studies have also declared that Meth can result in improved learning and memory function, including visuospatial perception and response speed following limited stimulant usage (Braren et al., 2014). Conversely, repeated Meth injection showed learning and mnemonic impairments (Jia et al., 2008). Some studies have shown that single-day regimens produce cognitive deficits (Braren et al., 2014). Furthermore, repeated Meth-provoked behavioral sensitization caused cognitive impairment. In addition, long-term memory deficiencies have been recognized in chronic Meth abusers (Kamei et al., 2006). Acute administration of a high dose of Meth (>2–10 mg/kg) caused impairments in spatial and non-spatial memory functions, caused by the degeneration of serotonergic and dopaminergic nerve terminals in the brain (Lee et al., 2011). Accordingly, animals previously exposed to a 1-day Meth regimen had dysfunctions in an appetitive maze sequential learning task and motor performance tasks and demonstrated mild spatial memory dysfunctions (Marshall et al., 2007). In contrast, low-dose Meth (2 mg/kg) injection caused hyperlocomotion but did not alter memory (Lee et al., 2011). The importance of morphine and other opioids in memory processes has been well described (Lelong-Boulouard et al., 2006).
Our data demonstrated that the co-administration of Meth + Bup increased the escape latency in comparison with the Meth group on the fourth day of training. It means that Bup partially potentiated Meth-induced memory impairment. In agreement with our results, it has been shown that opioid receptor agonists may diminish short- and/or long-term memory processes (Lelong-Boulouard et al., 2006). In this regard, the endogenous opioid system may play a crucial role in stress-induced memory impairment (Cao et al., 2015). In addition, Bup induced deficits in long-term memory in the passive avoidance test and short-term memory deficits in the Y-maze test (Lelong-Boulouard et al., 2006). Stimulation of κ-opioid receptors enhanced memory dysfunction arising from the blockade of muscarinic M1 receptors (Ukai et al., 1997). In contrast, acute administration of Bup improved short-term memory for social reward cues (Syal et al., 2015).
After repeated administration, Meth adheres to the dopamine transporter (DAT) on dopaminergic nerve terminals through a pseudo-transmitter function, which increases dopamine release but represses dopamine reuptake, leading to an increase in the level of dopamine in the synaptic cleft (Chen et al., 2012; Seminerio et al., 2013). As a result, Meth triggers dopamine release from the cytosol within the extracellular space using reverse transport by the DAT (Melo et al., 2012). Consistently, acute treatment with a low dose of Meth (2 mg/kg) enhanced striatal extracellular concentrations of dopamine. Furthermore, Meth reduced the intraneuronal oxidative metabolism of dopamine by the repression of monoamine oxidase (Pereira et al., 2011). Also, acute administration of Meth caused an increase in the dopamine level in the nucleus accumbens (Macúchová et al., 2013).
Meth is neurotoxic and directly damages neurons and memory function (Chen et al., 2012). Dopamine pathways are involved in cognition (Macúchová et al., 2013). Dopamine has been exhibited to change various cognitive functions, including attention, memory, response inhibition, and task switching (Seminerio et al., 2013). Long-term use of Meth damages dopaminergic systems and reduces various indices of dopamine terminal integrity, particularly in the striatum (Camarasa et al., 2010; Braren et al., 2014). Meth affects both the prefrontal cortex and striatum via the dopaminergic system. Dopamine deficiencies in the striatum decrease simple task performance and reaction time while dopamine deficits in the prefrontal cortex contribute to cognitive dysfunction (Seminerio et al., 2013). Changes caused by chronic exposure to Meth in rats include selective damage to dopaminergic terminals within the dorsal striatum and hippocampus, long-lasting decreases in dopamine content, loss of dopamine transporters, and a reduction in the activity of tyrosine hydroxylase and DAT (Camarasa et al., 2010; Braren et al., 2014). Vesicular monoamine transporter 2 has an essential role in Meth-induced toxicity (Melo et al., 2012). Meth reduces long-term potentiation (LTP) and produces synaptic maladaptation through changing excitatory synaptic transmission by the activation of the serotonin and dopamine receptor systems (Chen et al., 2012).
Furthermore, Meth use leads to nerve terminal degeneration by the production of nitrogen and reactive oxygen species. Nerve terminal and axonal degeneration can occur in the nigrostriatal dopaminergic projections, potentially resulting in cognitive impairment (Seminerio et al., 2013). Meth also induces cortical cell degeneration. Specifically, the Meth-induced degeneration of cortical neurons may only express the most apparent consequences of the powerful and enduring effects of Meth on the cerebral cortex (Marshall et al., 2007). This evidence is furthermore confirmed by the reports of the positive association between Meth intake and cell death in the prefrontal cortex and negative association between Meth intake and hippocampal volume in rodents (Krasnova et al., 2010).
Bup causes a progressive increase in extracellular dopamine and enhances basal levels of dopamine in the NAc (Sorge and Stewart, 2006). Also, Bup, like other partial μ-receptor agonists, stimulates the release of dopamine in the mesolimbic system (Nantwi et al., 1998). Moreover, opioid receptor agonists can change the activity of dopamine neurons and alter the pharmacodynamic impacts of Meth on the dopaminergic system (Sorge and Stewart, 2006).
Meth increases glutamate release in various brain regions, such as the cerebral cortex, hippocampus, and striatum (Lee et al., 2011). Conversely, prolonged administration of Bup can decrease glutamate function. Continuous exposure to Bup also results in reduced glutamatergic activity in the NAc and striatum (Sorge and Stewart, 2006). In contrast, Bup treatment increased basal levels of glutamate in the NAc of rats (Placenza et al., 2008). The glutamatergic system is perturbed by Meth exposure, leading to increased glutamate signaling. GluA2 (GluR2) incorporation into AMPA receptors in multiple brain regions is associated with Meth treatment and subsequent reduction in glutamate overflow and associated excitotoxicity (Olsen et al., 2013). In addition, Meth-induced impaired memory function due to alterations of N-Methyl-D-aspartate (NMDA) receptor binding sites in the hippocampus and prefrontal cortex may also underlie the memory and learning deficits correlated with the administration of it (Lee et al., 2011). NMDA receptors are needed for synaptic plasticity associated with learning and memory (Lee et al., 2011; Barzegar et al., 2015).
Other neurotransmitter systems, in addition to the dopaminergic and glutamatergic systems, may be involved in the effects of Meth and Bup on learning and memory. The increased, unchanged, or decreased serotonin (5HT) content after Meth administration has been reported (Melo et al., 2012). Meth may cause a decrease in the concentration of 5HT and its particular metabolites, a reduction in the number of 5HT transporter binding sites, a decline in the activity of tryptophan hydroxylase, and the loss of 5HT transporters in the striatum and hippocampus (Marshall et al., 2007). Conversely, opioids can increase the 5HT release and their analgesic effect depends partly on the consequent activation of postsynaptic 5HT1A receptors (Tao et al., 2003). Gamma-aminobutyric acid (GABA) receptors play a role in Meth-associated rewarding memories (Jiao et al., 2016). Intra-NAc infusion of muscimol (a GABA receptor agonist) reduced Meth-induced enhancement of LTP in the dentate gyrus-, while the infusion of AP5 (an NMDA receptor antagonist) inhibited Meth-induced improvement of LTP (Heysieattalab et al., 2016). The existence of interactions between GABAergic and opiate systems in the brain has been declared. The extensive concentration of opioid receptors in the limbic system (thalamus, amygdala, and NAc), hippocampus, and cerebral cortex suggests that GABA neurons are involved in emotional responses and many other cognitive processes (Lelong-Boulouard et al., 2006). In addition, the opioid-induced release of dopamine is likely a secondary response, owing to the inhibition of GABA interneurons, which results in the disinhibition of dopaminergic neurons (Johnson and North, 1992).
In summary, this study demonstrated that Bup administration accentuates the learning and memory impairment induced by Meth administration in rats. While Bup impairs spatial learning and memory, it potentiates aversive memory. Since Bup exhibits lower side effects compared to other opioids, it may be desirable for the treatment of avoidance memory discrepancies in patients with Meth addiction.
The original contributions presented in the study are included in the article/supplementary material, further inquiries can be directed to the corresponding author/s.
Rats used in this investigation were treated under the Guide for the Care and Use of Laboratory Animals, and the investigation protocols were proved by the Institutional Animal Care and Use Committee at Hamadan University of Medical Sciences (IR.UMSHA.REC.1400.346).
MT, AK, and FE wrote the manuscript and revised it. AR-K, NF, and SK supervised the study and performed the experiment. MA and SR analyzed the data. All authors approved the manuscript.
This research was supported by a grant (Grant No. IR.UMSHA.REC.1396.521) of the Hamadan University of Medical Sciences, Hamadan, Iran.
The authors declare that the research was conducted in the absence of any commercial or financial relationships that could be construed as a potential conflict of interest.
All claims expressed in this article are solely those of the authors and do not necessarily represent those of their affiliated organizations, or those of the publisher, the editors and the reviewers. Any product that may be evaluated in this article, or claim that may be made by its manufacturer, is not guaranteed or endorsed by the publisher.
We would like to express their gratitude to the staff of the Neurophysiology Research Center for helping us to carry out this project.
Asadbegi, M., Yaghmaei, P., Salehi, I., Komaki, A., and Ebrahim-Habibi, A. (2017). Investigation of thymol effect on learning and memory impairment induced by intrahippocampal injection of amyloid beta peptide in high fat diet-fed rats. Metab. Brain Dis. 32, 827–839. doi: 10.1007/s11011-017-9960-0
Barzegar, S., Komaki, A., Shahidi, S., Sarihi, A., Mirazi, N., and Salehi, I. (2015). Effects of cannabinoid and glutamate receptor antagonists and their interactions on learning and memory in male rats. Pharmacol. Biochem. Behav. 131, 87–90. doi: 10.1016/j.pbb.2015.02.005
Braren, S. H., Drapala, D., Tulloch, I. K., and Serrano, P. A. (2014). Methamphetamine-induced short-term increase and long-term decrease in spatial working memory affects protein Kinase M zeta (PKMζ), dopamine, and glutamate receptors. Front. Behav. Neurosci. 8:438. doi: 10.3389/fnbeh.2014.00438
Camarasa, J., Rodrigo, T., Pubill, D., and Escubedo, E. (2010). Memantine is a useful drug to prevent the spatial and non-spatial memory deficits induced by methamphetamine in rats. Pharmacol. Res. 62, 450–456. doi: 10.1016/j.phrs.2010.05.004
Cao, L., Wen, J., and Liu, Z. (2015). [Opioid μ receptors mediate the stress-induced spatial reference memory impairment]. Sheng Li Xue Bao:[Acta physiologica Sinica] 67, 173–180.
Castel, D., Sabbag, I., Nasaev, E., Peng, S., and Meilin, S. (2018). Open field and a behavior score in PNT model for neuropathic pain in pigs. J. Pain Res. 11:2279. doi: 10.2147/JPR.S172300
Chen, Y.-J., Liu, Y.-L., Zhong, Q., Yu, Y.-F., Su, H.-L., Toque, H. A., et al. (2012). Tetrahydropalmatine protects against methamphetamine-induced spatial learning and memory impairment in mice. Neurosci. Bull. 28, 222–232. doi: 10.1007/s12264-012-1236-4
Chiang, Y. C., Hung, T. W., and Ho, I. K. (2014). Development of sensitization to methamphetamine in offspring prenatally exposed to morphine, methadone and buprenorphine. Add. Biol. 19, 676–686. doi: 10.1111/adb.12055
Eastwood, E. C., and Phillips, T. J. (2014). Morphine intake and the effects of naltrexone and buprenorphine on the acquisition of methamphetamine intake. Genes Brain Behav. 13, 226–235. doi: 10.1111/gbb.12100
Etaee, F., Asadbegi, M., Taslimi, Z., Shahidi, S., Sarihi, A., Asl, S. S., et al. (2017). The effects of methamphetamine and buprenorphine, and their interaction on anxiety-like behavior and locomotion in male rats. Neurosci. Lett. 655, 172–178. doi: 10.1016/j.neulet.2017.04.043
Ganji, A., Salehi, I., Nazari, M., Taheri, M., and Komaki, A. (2017). Effects of Hypericum scabrum extract on learning and memory and oxidant/antioxidant status in rats fed a long-term high-fat diet. Metab. Brain Dis. 32, 1255–1265. doi: 10.1007/s11011-017-0022-4
Ghazvini, H., Khaksari, M., Esmaeilpour, K., Shabani, M., Asadi-Shekaari, M., Khodamoradi, M., et al. (2016). Effects of treatment with estrogen and progesterone on the methamphetamine-induced cognitive impairment in ovariectomized rats. Neurosci. Lett. 619, 60–67. doi: 10.1016/j.neulet.2016.02.057
Glover, E. M., and Davis, M. (2008). Anxiolytic-like effects of morphine and buprenorphine in the rat model of fear-potentiated startle: tolerance, cross-tolerance, and blockade by naloxone. Psychopharmacology 198, 167–180. doi: 10.1007/s00213-008-1112-0
Heysieattalab, S., Naghdi, N., Hosseinmardi, N., Zarrindast, M. R., Haghparast, A., and Khoshbouei, H. (2016). Methamphetamine-induced enhancement of hippocampal long-term potentiation is modulated by NMDA and GABA receptors in the shell–accumbens. Synapse 70, 325–335. doi: 10.1002/syn.21905
Hollais, A. W., Patti, C. L., Zanin, K. A., Fukushiro, D. F., Berro, L. F., Carvalho, R. C., et al. (2014). Effects of acute and long-term typical or atypical neuroleptics on morphine-induced behavioural effects in mice. Clin. Exp. Pharmacol. Physiol. 41, 255–263. doi: 10.1111/1440-1681.12203
Jia, F., Mobarakeh, J. I., Dai, H., Kato, M., Xu, A., Okuda, T., et al. (2008). Blocking histamine H1 improves learning and mnemonic dysfunction in mice with social isolation plus repeated methamphetamine injection. J. Pharmacol. Sci. 107, 167–174. doi: 10.1254/jphs.FP0072424
Jiao, D.-L., Liu, Y., Long, J.-D., Du, J., Ju, Y.-Y., Zan, G.-Y., et al. (2016). Involvement of dorsal striatal α1-containing GABA A receptors in methamphetamine-associated rewarding memories. Neuroscience 320, 230–238. doi: 10.1016/j.neuroscience.2016.02.001
Johnson, S., and North, R. (1992). Opioids excite dopamine neurons by hyperpolarization of local interneurons. J. Neurosci. 12, 483–488. doi: 10.1523/JNEUROSCI.12-02-00483.1992
Kamei, H., Nagai, T., Nakano, H., Togan, Y., Takayanagi, M., Takahashi, K., et al. (2006). Repeated methamphetamine treatment impairs recognition memory through a failure of novelty-induced ERK1/2 activation in the prefrontal cortex of mice. Biol. Psychiatry 59, 75–84. doi: 10.1016/j.biopsych.2005.06.006
Khodamoradi, N., Komaki, A., Salehi, I., Shahidi, S., and Sarihi, A. (2015). Effect of vitamin E on lead exposure-induced learning and memory impairment in rats. Physiol. Behav. 144, 90–94. doi: 10.1016/j.physbeh.2015.03.015
Krasnova, I. N., Justinova, Z., Ladenheim, B., Jayanthi, S., McCoy, M. T., Barnes, C., et al. (2010). Methamphetamine self-administration is associated with persistent biochemical alterations in striatal and cortical dopaminergic terminals in the rat. PLoS One 5:e8790. doi: 10.1371/journal.pone.0008790
Lee, K.-W., Kim, H.-C., Lee, S.-Y., and Jang, C.-G. (2011). Methamphetamine-sensitized mice are accompanied by memory impairment and reduction of N-methyl-d-aspartate receptor ligand binding in the prefrontal cortex and hippocampus. Neuroscience 178, 101–107. doi: 10.1016/j.neuroscience.2011.01.025
Lelong-Boulouard, V., Quentin, T., Moreaux, F., Debruyne, D., Boulouard, M., and Coquerel, A. (2006). Interactions of buprenorphine and dipotassium clorazepate on anxiety and memory functions in the mouse. Drug Alcohol Dependence 85, 103–113. doi: 10.1016/j.drugalcdep.2006.03.017
Macúchová, E., Nohejlová-Deykun, K., and Slamberova, R. (2013). Effect of methamphetamine on cognitive functions of adult female rats prenatally exposed to the same drug. Physiol. Res. 62:S89. doi: 10.33549/physiolres.932598
Marshall, J. F., Belcher, A. M., Feinstein, E. M., and O’Dell, S. J. (2007). Methamphetamine-induced neural and cognitive changes in rodents. Addiction 102, 61–69. doi: 10.1111/j.1360-0443.2006.01780.x
Martin, L. B., Thompson, A. C., Martin, T., and Kristal, M. B. (2001). Analgesic efficacy of orally administered buprenorphine in rats. Comp. Med. 51, 43–48.
Melo, P., Magalhães, A., Alves, C. J., Tavares, M. A., de Sousa, L., Summavielle, T., et al. (2012). Methamphetamine mimics the neurochemical profile of aging in rats and impairs recognition memory. Neurotoxicology 33, 491–499. doi: 10.1016/j.neuro.2012.03.002
Miladi-Gorji, H., Fadaei, A., and Bigdeli, I. (2015). Anxiety assessment in methamphetamine-sensitized and withdrawn rats: immediate and delayed effects. Iran. J. Psychiatry 10:150.
Moenk, M. D., and Matuszewich, L. (2012). Juvenile but not adult methamphetamine exposure improves performance in the Morris Water Maze in male rats. Int. J. Dev. Neurosci. 30, 325–331. doi: 10.1016/j.ijdevneu.2012.01.006
Moradkhani, S., Salehi, I., Abdolmaleki, S., and Komaki, A. (2015). Effect of Calendula officinalis hydroalcoholic extract on passive avoidance learning and memory in streptozotocin-induced diabetic rats. Ancient Sci. Life 34:156. doi: 10.4103/0257-7941.157160
Mori, T., Ito, S., Kita, T., Narita, M., Suzuki, T., and Sawaguchi, T. (2006). Effects of μ-, δ-and κ-opioid receptor agonists on methamphetamine-induced self-injurious behavior in mice. Eur. J. Pharmacol. 532, 81–87. doi: 10.1016/j.ejphar.2005.12.035
Motamedi, F., Ghasemi, M., Davoodi, F., and Naghdi, N. (2010). Comparison of learning and memory in morphine dependent rats using different behavioral models. Iran. J. Pharmaceut. Res. 2, 225–230.
Nantwi, K., Hicks, S., Bradley, D., and Schoener, E. (1998). Interactions of buprenorphine and selective dopamine receptor antagonists in the rat nucleus accumbens. General Pharmacol. Vascular System 31, 425–429. doi: 10.1016/S0306-3623(98)00020-2
Olsen, R. H., Allen, C. N., Derkach, V. A., Phillips, T. J., Belknap, J. K., and Raber, J. (2013). Impaired memory and reduced sensitivity to the circadian period lengthening effects of methamphetamine in mice selected for high methamphetamine consumption. Behav. Brain Res. 256, 197–204. doi: 10.1016/j.bbr.2013.08.015
Pereira, F. C., Gough, B., Macedo, T. R., Ribeiro, C. F., Ali, S. F., and Binienda, Z. K. (2011). Buprenorphine modulates methamphetamine-induced dopamine dynamics in the rat caudate nucleus. Neurotoxicity Res. 19, 94–101. doi: 10.1007/s12640-009-9143-9
Placenza, F., Rajabi, H., and Stewart, J. (2008). Effects of chronic buprenorphine treatment on levels of nucleus accumbens glutamate and on the expression of cocaine-induced behavioral sensitization in rats. Psychopharmacology 200, 347–355. doi: 10.1007/s00213-008-1210-z
Polston, J., Rubbinaccio, H., Morra, J., Sell, E., and Glick, S. (2011). Music and methamphetamine: conditioned cue-induced increases in locomotor activity and dopamine release in rats. Pharmacol. Biochem. Behav. 98, 54–61. doi: 10.1016/j.pbb.2010.11.024
Pournaghash, S., and Riley, A. L. (1993). Buprenorphine as a stimulus in drug discrimination learning: an assessment of mu and kappa receptor activity. Pharmacol. Biochem. Behav. 46, 593–604. doi: 10.1016/0091-3057(93)90549-9
Reichel, C. M., Gilstrap, M. G., Ramsey, L. A., and See, R. E. (2014). Modafinil restores methamphetamine induced object-in-place memory deficits in rats independent of glutamate N-methyl-d-aspartate receptor expression. Drug Alcohol Dependence 134, 115–122. doi: 10.1016/j.drugalcdep.2013.09.018
Salehi, M., Emadossadat, A., Kheirabadi, G. R., Maracy, M. R., and Sharbafchi, M. R. (2015). The effect of buprenorphine on methamphetamine cravings. J. Clin. Psychopharmacol. 35, 724–727. doi: 10.1097/JCP.0000000000000408
Schutová, B., Hrubá, L., Pometlová, M., and Šlamberová, R. (2009). Impact of prenatal and acute methamphetamine exposure on behaviour of adult male rats. Prague Med. Rep. 110, 67–78.
Seminerio, M. J., Hansen, R., Kaushal, N., Zhang, H.-T., McCurdy, C. R., and Matsumoto, R. R. (2013). The evaluation of AZ66, an optimized sigma receptor antagonist, against methamphetamine-induced dopaminergic neurotoxicity and memory impairment in mice. Int. J. Neuropsychopharmacol. 16, 1033–1044. doi: 10.1017/S1461145712000831
Shiri, M., Komaki, A., Oryan, S., Taheri, M., Komaki, H., and Etaee, F. (2016). Effects of cannabinoid and vanilloid receptor agonists and their interactions on learning and memory in rats. Can. J. Physiol. Pharmacol. 95, 382–387. doi: 10.1139/cjpp-2016-0274
Sorge, R. E., and Stewart, J. (2006). The effects of chronic buprenorphine on intake of heroin and cocaine in rats and its effects on nucleus accumbens dopamine levels during self-administration. Psychopharmacology 188, 28–41. doi: 10.1007/s00213-006-0485-1
Syal, S., Ipser, J., Terburg, D., Solms, M., Panksepp, J., Malcolm-Smith, S., et al. (2015). Improved memory for reward cues following acute buprenorphine administration in humans. Psychoneuroendocrinology 53, 10–15. doi: 10.1016/j.psyneuen.2014.11.009
Tao, R., Karnik, M., Ma, Z., and Auerbach, S. B. (2003). Effect of fentanyl on 5-HT efflux involves both opioid and 5-HT1A receptors. Br. J. Pharmacol. 139, 1498–1504. doi: 10.1038/sj.bjp.0705378
Thompson, A. C., DiPirro, J. M., Sylvester, A. R., Martin, L. B., and Kristal, M. B. (2006). Lack of analgesic efficacy in female rats of the commonly recommended oral dose of buprenorphine. J. Am. Assoc. Lab. Animal Sci. 45, 13–16.
Ukai, M., Itoh, J., Kobayashi, T., Shinkai, N., and Kameyama, T. (1997). Effects of the κ-opioid dynorphin A (1–13) on learning and memory in mice. Behav. Brain Res. 83, 169–172. doi: 10.1016/S0166-4328(97)86063-9
Vorhees, C. V., Inman-Wood, S. L., Morford, L. L., Broening, H. W., Fukumura, M., and Moran, M. S. (2000). Adult learning deficits after neonatal exposure tod-methamphetamine: selective effects on spatial navigation and memory. J. Neurosci. 20, 4732–4739. doi: 10.1523/JNEUROSCI.20-12-04732.2000
Wala, E. P., and Holtman, J. R. (2011). Buprenorphine-induced hyperalgesia in the rat. Eur. J. Pharmacol. 651, 89–95. doi: 10.1016/j.ejphar.2010.10.083
Zarrindast, M. R., Ardjmand, A., Rezayof, A., and Ahmadi, S. (2013). The time profile of morphine effect on different phases of inhibitory avoidance memory in rat. Arch. Iran. Med. 16, 34–37.
Zarrinkalam, E., Heidarianpour, A., Salehi, I., Ranjbar, K., and Komaki, A. (2016). Effects of endurance, resistance, and concurrent exercise on learning and memory after morphine withdrawal in rats. Life Sci. 157, 19–24. doi: 10.1016/j.lfs.2016.05.034
Zarrinkalam, E., Ranjbar, K., Salehi, I., Kheiripour, N., and Komaki, A. (2018). Resistance training and hawthorn extract ameliorate cognitive deficits in streptozotocin-induced diabetic rats. Biomed. Pharmacotherapy 97, 503–510. doi: 10.1016/j.biopha.2017.10.138
Keywords: methamphetamine, buprenorphine, learning, memory, interaction
Citation: Etaee F, Rezvani-Kamran A, Komaki S, Asadbegi M, Faraji N, Raoufi S, Taheri M, Kourosh-Arami M and Komaki A (2021) Effects of Buprenorphine on the Memory and Learning Deficit Induced by Methamphetamine Administration in Male Rats. Front. Behav. Neurosci. 15:748563. doi: 10.3389/fnbeh.2021.748563
Received: 28 July 2021; Accepted: 29 October 2021;
Published: 23 November 2021.
Edited by:
Nadja Schroder, Federal University of Rio Grande do Sul, BrazilReviewed by:
Jan Svoboda, Academy of Sciences of the Czech Republic (ASCR), CzechiaCopyright © 2021 Etaee, Rezvani-Kamran, Komaki, Asadbegi, Faraji, Raoufi, Taheri, Kourosh-Arami and Komaki. This is an open-access article distributed under the terms of the Creative Commons Attribution License (CC BY). The use, distribution or reproduction in other forums is permitted, provided the original author(s) and the copyright owner(s) are credited and that the original publication in this journal is cited, in accordance with accepted academic practice. No use, distribution or reproduction is permitted which does not comply with these terms.
*Correspondence: Alireza Komaki, YWxpcmV6YWtvbWFraUBnbWFpbC5jb20=; S29tYWtpQHVtc2hhLmFjLmly
Disclaimer: All claims expressed in this article are solely those of the authors and do not necessarily represent those of their affiliated organizations, or those of the publisher, the editors and the reviewers. Any product that may be evaluated in this article or claim that may be made by its manufacturer is not guaranteed or endorsed by the publisher.
Research integrity at Frontiers
Learn more about the work of our research integrity team to safeguard the quality of each article we publish.