- 1Preclinical Behavior and Models Core, F. Edward Hébert School of Medicine, Uniformed Services University of the Health Sciences, Bethesda, MD, United States
- 2Department of Anatomy, Physiology and Genetics, F. Edward Hébert School of Medicine, Uniformed Services University of the Health Sciences, Bethesda, MD, United States
Anxiety is a common complaint following acquired traumatic brain injury (TBI). However, the measurement of dysfunctional anxiety behavioral states following experimental TBI in rodents is complex. Some studies report increased anxiety after TBI, whereas others find a decreased anxiety-like state, often described as increased risk-taking behavior or impulsivity. These inconsistencies may reflect a lack of standardization of experimental injury models or of behavioral testing techniques. Here, we review the most commonly employed unconditioned tests of anxiety and discuss them in a context of experimental TBI. Special attention is given to the effects of repeated testing, and consideration of potential sensory and motor confounds in injured rodents. The use of multiple tests and alternative data analysis methods are discussed, as well as the potential for the application of common data elements (CDEs) as a means of providing a format for documentation of experimental details and procedures of each published research report. CDEs may improve the rigor, reproducibility, as well as endpoint for better relating findings with clinical TBI phenotypes and the final goal of translation. While this may not resolve all incongruities in findings across laboratories, it is seen as a way forward for standardized and universal data collection for improvement of data quality and sharing, and advance therapies for neuropsychiatric symptoms that often present for decades following TBI.
Introduction
Anxiety disorders are characterized by the DSM-V (Diagnostic and Statistical Manual of Mental Disorders, version 5) as excessive fear and anxiety with related behavioral disturbances (American Psychiatric Association, 2013), and are associated with comorbid conditions such as cardiovascular disease, migraine, hypertension, and gastrointestinal disease. Psychiatric disturbances including anxiety often persist for many years following traumatic brain injury (TBI) (Koponen et al., 2002, 2006; Scholten et al., 2016), a worldwide growing healthcare burden (James et al., 2019). A recent review pooled long-term prevalence of anxiety following TBI to be 36% (Scholten et al., 2016), and the presence of anxiety 10 years post-TBI has been found to be a strong predictor of poor psychosocial function (Draper et al., 2007).
Animal models have long played a role in the study of behavioral symptoms and pathophysiology following traumatic brain injury, as well as in the development of therapeutic agents for those maladies, albeit with limited success. Behavioral testing following experimental TBI in rodents has provided extensive data in multiple domains of behavior, including motor, cognitive, and neuropsychiatric function (File, 2001; Fujimoto et al., 2004; Malkesman et al., 2013; Osier et al., 2015). Although less attention is given to neuropsychiatric function than to motor and cognitive issues in translational research, the study of anxiety-like behaviors in animal models of TBI is popular due to their high clinical relevance (Scholten et al., 2016). The purpose of this review is to introduce the reader to the most common tests for anxiety employed following experimental TBI, followed by a discussion of the shortcomings and inconclusive results obtained from anxiety testing in animal models of TBI to date. Further attention will be given to potential testing confounds, such as sensory deficits and differences in overall activity levels. We conclude with an emphasis on suggestions for alternative methods of data analysis and the promise of common data elements (CDEs) as a means of improving preclinical studies translation.
Unconditioned Tests of Anxiety in Rodents
Anxiety has been described in animals as a generalized psychological, physiological, and behavioral state induced by exposure to an unknown threat or internal conflict (Steimer, 2002, 2011). There are numerous tests for measuring anxiety-like behaviors in rodent models, and these assays are most often classified as “conditioned” and “unconditioned” tests (Bourin et al., 2007). Conditioned tests depend on the development of a conditioned response to an aversive stimulus, such as fear potentiated startle and conditioned defensive burying. It is important to note that performance on conditioned tests is often dependent on intact cognitive and sensory function, and as such may not be appropriate in a TBI setting if significant deficits are present. Unconditioned tests rely on spontaneous, natural responses to ethologically relevant, stressful situations, which take advantage of the conflict inherent in approach-avoidance situations. The conflict results in a competition between spontaneous exploratory behavior and the innate aversion of open, illuminated areas (Lister, 1990). Although they are not without criticism, unconditioned tests are the most frequently used assays in anxiety and TBI research, largely due to their ease of use. In this section we review the most popular unconditioned tests of anxiety; the reader is also directed to several comprehensive reviews (Lister, 1990; Belzung and Griebel, 2001; Castanheira et al., 2018; Harro, 2018).
Open Field Test
The open field test (OFT) (Figure 1A) was developed by Hall in 1934 and has become one of the most popular behavioral tests in multiple species. It is simple to perform and requires only basic equipment (Hall, 1934; Lister, 1990; Prut and Belzung, 2003). In the OFT, animals are individually placed in the center of a circular or rectangular/square arena [e.g., 40 cm × 40 cm for mice (Yu et al., 2012; Tucker et al., 2017); 100 cm × 100 cm for rats (McAteer et al., 2016; Tsuda et al., 2020)] with walls high enough to prevent escape, and behavior is recorded, typically with automated software. Trial lengths are as short as 2 min and up to an hour (Castanheira et al., 2018). When placed in the OF environment, rodents typically display thigmotactic behavior, staying near the walls of the apparatus and spending less time in the center, more exposed region. The most common measures analyzed include total horizontal distance traveled, number of rearing episodes [see (Sturman et al., 2018) for discussion], freezing, number of fecal boli, and proportion of time spent in a software-defined center zone of the apparatus (Crawley, 2007).
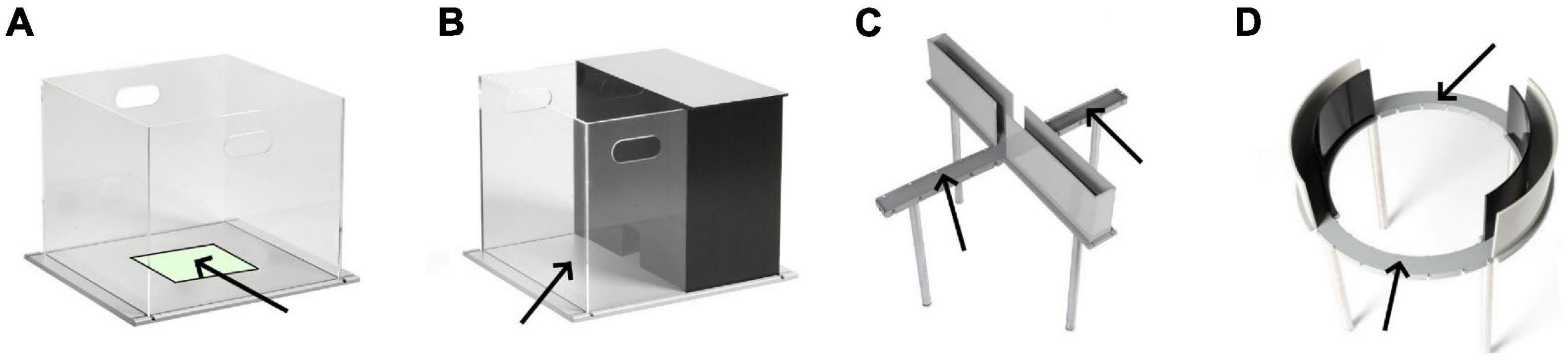
Figure 1. Common apparatuses for measuring unconditioned anxiety-like behaviors in rodents: (A) Open field, (B) Light-dark box, (C) Elevated plus maze, (D) Elevated zero maze. Arrows point to anxiogenic (lit and exposed) regions of the apparatus. Images from Stoelting, Co.
There has been a historical assumption that lower levels of ambulation in the OFT represent increased “emotionality” or anxiety (Hall, 1934; Lister, 1990). However, non-anxiolytic pharmacological agents can increase activity in the arena (Cunha and Masur, 1978), and general locomotor activity has been suggested to be “an unsuitable index of anxiety in psychopharmacological research” (Lister, 1990). Presently, the most commonly employed measure of anxiety is thigmotaxis (Seibenhener and Wooten, 2015), or alternatively, activity in the center region of the OF (either time spent in the center or distance traveled (McAteer et al., 2016; Tucker et al., 2017). Distance traveled in the center should be expressed as a percent of the total distance traveled if there are baseline differences in activity between experimental groups. Greater time spent or distance traveled in the center is interpreted as reduced or less anxiety. However, common anxiolytic drugs do not increase the amount of time spent in the center (Thompson et al., 2015), and the test has been criticized for its inability to dissociate locomotion, exploration, and anxiety (File, 2001). Thus, it has been suggested that conclusions regarding anxiety states in the OFT be considered only preliminary, and followed up with more specific anxiety tests such as the light-dark box or elevated plus maze (Crawley, 2007).
Light-Dark Box
The light-dark box (LDB) (Figure 1A) is a modification of the OFT in which approximately two thirds of the apparatus is open, uncovered and brightly illuminated (e.g., ∼400 Lux), and the remaining one third is enclosed with darkened walls, and covered (Crawley and Goodwin, 1980; Bourin and Hascoet, 2003). An opening in the wall separating the two chambers allows the animal to move freely within the apparatus, and also allows a small amount of light to enter the darkened chamber resulting in a more natural setting. As rodents typically live in small tunnels, they will prefer the smaller darkened chamber, but are also driven by an urge to explore and thus also spend time in the larger brightened portion of the apparatus. The animal is placed in the illuminated section of the apparatus and monitored for 10 min; time spent in each chamber is recorded as well as the number of transitions between the dark and light compartments (as an index of activity). Increases in the time spent in the brightened compartment are suggested to reflect anxiolytic activity. Other parameters have been proposed including rearing, the latency to enter the dark compartment, amount of activity in each chamber (expressed as a function of time in each chamber), number of times the animal “peeks” from the dark chamber into the light but then retreats (Hascoët and Bourin, 1998; Bourin and Hascoet, 2003). However, after a careful review of the literature, Hascoët and Bourin concluded that the most reliable measure for assessing anxiolytic-like activity was the time spent in the brighter chamber (with greater time in the dark chamber representing anxiety-like behavior) (Hascoët and Bourin, 1998); this parameter provided a stable baseline and consistent dose-response increases in response to anxiolytic agents.
Elevated Plus Maze and Elevated Zero Maze
The elevated plus maze (EPM; Figure 1C) is the most widely employed test for assessing anxiety-like behaviors and testing putative anxiolytic agents in rodents (Haller et al., 2013). The EPM was developed and pharmacologically validated in rats (Pellow et al., 1985), and quickly adapted for use in mice (Lister, 1987). The EPM is a plus-shaped apparatus, with two darkened and enclosed (but uncovered) arms and two exposed arms joined by a central square, elevated 50–100 cm above the floor. Compared to the OFT and LDB, the anxiogenic stimulus of the EPM is the absence of walls or thigmotactic cues in the open arms (rather than height) (Treit et al., 1993). Animals are individually placed in the center square, and activity is tracked for 5 min. Measures of anxiety include time spent in the open arms (expressed as a percent of total arm time) and number of entries into open arms (expressed as a percent of total arm entries) (Rodgers et al., 1997). Rodents with greater levels of anxiety will spend less time in (and make fewer entries into) the open exposed arms; these measures are sensitive to some anxiolytic agents (Pellow et al., 1985; Lister, 1987). An appropriate index of locomotor activity has been the subject of debate (Weiss et al., 1998).
The elevated zero maze (EZM; Figure 1D) is a modification of the EPM that is annular and has alternating “open” and “closed” quadrants (Shepherd et al., 1994). The EZM has the benefit of removing the center square, allowing simpler analysis of activity in open and closed regions of the apparatus. Interpretation of activity in the center square of the EPM, where the open and closed arms join, has been difficult, particularly in mice, who can spend at least 20–30% of the test session in this region (Lee and Rodgers, 1990; Rodgers et al., 1992). Also, there is more continuous exploration of the EZM, as it eliminates the “boxed ends” inherent to the closed arms of the EPM. A test session of the EZM is 5 min in length; animals are placed at a randomly chosen boundary between an open and closed quadrant, facing the inside of the closed zone. Measures of interest include the time spent in the open quadrants, latency to enter an open quadrant, and the number of entries to open quadrants; these variables are sensitive to the effects of standard anxiolytic agents including benzodiazepines, zolpidem and phenobarbitone (Kulkarni et al., 2007).
In addition to the measurement of approach/avoidance behavior by assessment of activity in open and closed zones, both the EPM and EZM are amenable to the study of ethological behaviors such as head dips (downward movements of the head toward the floor) and stretch attenuated postures (elongation of the body with the feet remaining in place) (Shepherd et al., 1994; Rodgers et al., 1997). These directed exploration and risk-assessment behaviors are suggested to be less sensitive to potential locomotor confounds [but see (Weiss et al., 1998)] and may be related to the “apprehension and excessive vigilance” observed in clinical anxiety (Cryan and Holmes, 2005). Head dipping may be considered directed exploration (Weiss et al., 1998), and an increase in this behavior reflects decreased levels of anxiety. Stretch attenuated postures reflect risk-assessment, and increases in this behavior suggest heightened levels of anxiety. For example, the anxiolytic agent chlordiazepoxide was shown to increase the amount of time rats spent in the open quadrants of the EZM and frequency of head dipping, and decrease the number of stretch-attenuated postures (Weiss et al., 1998).
Anxiety-Like Behaviors Following Experimental TBI
Animal Models of Experimental TBI
The literature on rodent models of TBI becomes more voluminous by the year, with numerous experimental models of TBI available to investigators. A full description of these models goes beyond the scope of this paper, but the reader is directed to several reviews (Xiong et al., 2013; Johnson et al., 2015; Marklund, 2016; Povlishock, 2016; Bodnar et al., 2019; Ma et al., 2019). In brief, pre-clinical TBI models include considerably invasive approaches that require a craniectomy, such as controlled cortical impact (CCI) (Osier and Dixon, 2016) and fluid percussion injury (FPI) (Lyeth, 2016), which result in focal and combined focal/diffuse injuries, respectively. These models have been established for decades and have produced a wide body of literature. Although CCI and FPI have remained popular, in recent years attention has turned to less invasive and more clinically relevant “closed-head” models that produce a concussive, more diffuse injury, with or without acceleration/deceleration and rotational components (Bodnar et al., 2019; McNamara et al., 2020). Also of growing interest are animal models of blast overpressure (Skotak et al., 2019; McCabe and Tucker, 2020), as TBI from blast has been the signature wound from military conflicts for the past 20 years.
A significant and important point of discussion has been the distinction between mild, moderate and severe experimental TBI, both within and between different models. Within a given translational study, the severity of the injury as defined by injury device parameters can have effects of both behavioral and pathological outcomes (Saatman et al., 2006; Washington et al., 2012; Namjoshi et al., 2017; Tucker et al., 2017). Clinically, there have been clearly defined criteria for classifying TBI as mild, moderate or severe, employing measures including duration of loss of consciousness and post-injury amnesia, structural imaging, and the Glasgow Coma Scale (Cassidy et al., 2004; Management of Concussion/mTBI Working Group, 2009; Centers for Disease Control and Prevention, 2015). Although guiding principles for classification of TBI severity within the CCI model have been proposed using injury parameters (e.g., depth and velocity of impact) and outcomes such as tissue loss, neurological severity score, and cognitive deficits (Siebold et al., 2018), other variables such as injury location and whether the skull cap was replaced can contribute to injury severity. Furthermore, high-speed imaging of CCI devices has shown that there is variability in the operation between different devices, albeit modest (Kim et al., 2018). Similar issues exist for FPI and closed-head models; thus, it remains difficult to label and compare injuries as mild, moderate or severe.
Another important but difficult distinction is between acute and chronic effects of TBI in animal models. Clinically, the effects of mild TBI or concussion typically resolve within 7–10 days, with only a percentage of patients (10–25%) having symptoms persisting beyond 3 months; symptoms that can include neuropsychiatric complaints like anxiety and depression (Dwyer and Katz, 2018; Polinder et al., 2018). There is no agreement regarding the definitions of “acute” and “chronic” with respect to functional deficits in rodents following TBI (Osier et al., 2015). In a review of long-term deficits following TBI, Gold and colleagues defined “long-term” as 1 month or later, as they were interested in an optimal time point that would enable the determination of safety and efficacy of stem cell therapies (Gold et al., 2013). In a later review on chronic effects of TBI in rodents, Osier and colleagues acknowledged the 1-month time point of the earlier review, but reduced the time point to 2 weeks to be more inclusive (Osier et al., 2015). In a recent paper employing multiple measures of function and neuroimaging following concussion with acceleration/deceleration and rotational components, the authors concluded that a post-injury time point of 7–14 days, when the injury was inflicted approximately at 13 weeks of age in mice, was approximately equivalent to 24 months of recovery in a young adult post-concussion (Barretto et al., 2021). Thus, a time point in the range of 2 weeks to 1 month post-injury in rodents may be appropriate for modeling chronic symptoms observed clinically.
Anxiety-Like Symptoms Post-TBI in Rodents
Despite the importance of testing for symptoms of anxiety in animal models of TBI, results have been woefully irreconcilable (Malkesman et al., 2013; Semple et al., 2019). Most often, to assay anxiety-related symptoms, a single behavioral test is performed following experimental TBI. For example, in the well-established and popular CCI model inflicted over parietal cortex, and using the well-validated EPM or EZM, conclusions have been inconsistent: many investigators have reported an increase in anxiety-like behaviors in rats and mice during these tests following CCI (e.g., Chauhan et al., 2010; Almeida-Suhett et al., 2014; Tchantchou et al., 2014), others have found a decrease in anxiety (often called increased risk-taking, impulsivity, or “behavioral disinhibition”) (e.g., Washington et al., 2012; Tucker et al., 2017), whereas sometimes no differences between injured and control groups are found at all (e.g., Watanabe et al., 2013; Sierra-Mercado et al., 2015). Reviewing the literature does not suggest that injury severity, when it can be compared, is a factor (Popovitz et al., 2019). The literature is voluminous and broad, even within a single injury model such as CCI, and there is a lack of standardization of injury methods as well as in behavioral testing paradigms.
Table 1 summarizes many recent studies employing unconditioned tests of anxiety in translational TBI studies. It is not meant to be all-encompassing, but to summarize studies cited in this paper and provide an update to previous reviews (Malkesman et al., 2013; Semple et al., 2019). A full systematic review has not been performed on pre-clinical studies of anxiety-related behaviors following TBI [but see (Semple et al., 2019) for an excellent summary]. The most consistency is with the FPI model, where increased anxiety-like behaviors are observed in most studies in both rats (Das et al., 2019; Dobrachinski et al., 2019; Fucich et al., 2019; Beitchman et al., 2020; Lapinlampi et al., 2020; Barretto et al., 2021) and mice (Tan et al., 2020; Tapp et al., 2020; Bhowmick et al., 2021), although in some studies this result is dependent on the time testing took place after injury. There is limited evidence from some CCI studies that results and conclusions from anxiety testing may depend on the time after injury at which behavioral testing takes place, or on the specific behavioral test employed (Tucker et al., 2017; Popovitz et al., 2019). Tucker and colleagues, for example, reported that following a severe CCI (but not mild CCI), mice showed increased thigmotaxis (indicative of greater anxiety) in the OFT for up to 3 weeks following injury; however, these same mice spent greater times in exposed, more brightly lit areas of the LDB and EZM at the same time points, suggesting decreased anxiety-like states (Tucker et al., 2017). In a similar repeated measures design study, Popovitz and colleagues demonstrated more time-dependent, rather than test-dependent results (Popovitz et al., 2019). Prior to 1 month following injury, mice with a moderate to severe CCI showed increased anxiety-like behaviors in the EZM and EPM, whereas after 5 weeks, decreased anxiety-like behaviors were measured in the EZM and OFT (Popovitz et al., 2019). Both of these studies illustrate the complexity of the changes in behavior and evolution over time in functional changes after TBI, and suggest that a comprehensive approach is necessary for drawing firmer conclusions regarding anxiety symptoms.
As stated prior, the CCI literature is broad. CCI is considered a focal injury, and unfortunately these inconsistencies in results extend to more diffuse and “closed-head” injury models (weight-drop, blast overpressure, and single or repeated concussive brain injury); further indicating that better standardization of TBI models and behavioral testing is needed. A newer TBI model, the Closed-Head Model of Engineered Rotational Acceleration (CHIMERA), a commercially available device that produces a contact force resulting in a rotational injury with acceleration/deceleration components, provides a more limited set of data on anxiety-like behaviors following experimental TBI (McNamara et al., 2020). To date, although more data are needed, results with the CHIMERA model have shown relative consistency: increased anxiety is measured in the OFT test up to a couple of weeks following injury, whereas decreased anxiety-like behaviors are found with the EZM or EPM at more chronic time points (e.g., Namjoshi et al., 2014; Nolan et al., 2018; McNamara et al., 2020). Future work with the clinically relevant CHIMERA model, employing multiple behavioral paradigms for anxiety and sophisticated analysis techniques will aid in the understanding of the development of symptoms of anxiety post-TBI, their biological underpinnings, and potential therapeutic targets.
Consideration of Potential Testing Confounds
For any behavioral test following an experimental manipulation, it is critical to consider the animals’ ability to perform the behavioral task; sensorimotor function is especially important during performance in unconditioned tests of anxiety (Cryan and Holmes, 2005). Differences between groups in general arousal or activity may lead to perceived differences in levels of anxiety. For example, Algamal and colleagues found that mice subjected to two sessions of 21 days of repeated uncontrolled stress showed anxiety-like behavior in the EPM, which was ameliorated when the animals were also subjected to repeated mild TBI (Algamal et al., 2019). The authors suggested that this finding, as well as others in their study, should be interpreted carefully as increased locomotion, or “behavioral disinhibition,” from the mild TBI procedure (Algamal et al., 2019). This “impulsive” behavior has been described as increased time and entries to the open arms or quadrants of the EPM or EZM in injured rodents, and this could be related to changes in overall arousal due to TBI (Mannix et al., 2014; Mouzon et al., 2014; Gold et al., 2018; Tucker et al., 2019). Hyperactivity has been reported in multiple experimental models of TBI, including CCI (Kimbler et al., 2012; Budinich et al., 2013; Hsieh et al., 2014; Bajwa et al., 2016; Tucker et al., 2017) and repetitive concussive brain injury (Kane et al., 2012; Mannix et al., 2014; Tucker et al., 2019; Vu et al., 2020). Dissociating anxiety from activity and impulsivity has long been a point of discussion in translational anxiety research (Dawson and Tricklebank, 1995; Weiss et al., 1998; Cryan and Holmes, 2005). Suggestions for overcoming this confound include the measurement of ethological “risk assessment” behaviors including head dips and stretch attenuated postures (in the EPM and EZM) which may be less affected by overall arousal (Weiss et al., 1998; Cryan and Holmes, 2005), or the use of locomotor activity as a covariate in statistical analysis (Braun et al., 2011).
As unconditioned tests of anxiety partly rely on discrimination between light and darkness, the visual system plays a role in task performance. Deficits in visual acuity have been demonstrated following concussive, weight-drop, CHIMERA- and blast-induced TBI in rodents (Xu et al., 2016; Desai et al., 2020; Evans et al., 2021; Morriss et al., 2021; Tucker et al., 2021), but a basic light/dark discrimination ability may be intact and adequate for performance. Filgueiras and colleagues demonstrated that rats no longer avoid open arms of the EPM if they are enclosed with clear walls, suggesting the absence of physical walls is the anxiogenic factor (Filgueiras et al., 2014). Thus, perhaps of more importance than the visual system are vibrissae and somatosensory cortex; as an animal assesses the environment it prefers to keep its vibrissae in contact with walls (Prut and Belzung, 2003; Crawley, 2007). In an OF environment, mice without vibrissae will no longer show thigmotaxis (Prut and Belzung, 2003), although a lack of vibrissae alters primary EPM measures little in rats, suggesting compensatory mechanisms are in place (Filgueiras et al., 2014) [but see (Belzung, 1999) for observations in mice].
Repeated Testing in Unconditioned Tests of Anxiety
Baseline measures prior to experimental manipulation, and/or testing at multiple time points during the experiment, may be desirable or necessary. However, performance during subsequent exposures to a behavioral test is affected by prior experience with the apparatus and environment, and the change may be treatment-dependent. Activity in the center has been shown to be relatively consistent across multiple trials (Bouwknecht et al., 2004), but the majority of behavioral data regarding OFT habituation relates to overall activity or locomotor activity in the apparatus. Hall initially described a decrease in the amount of locomotor activity in the OFT in rats with each subsequent exposure, reflective of a habituation process (Hall, 1934). Since that time, between-session habituation with repeated OFT testing has been confirmed by many in rats (Cerbone and Sadile, 1994; Dubovicky and Jezova, 2004; Haleem et al., 2015; Poveda et al., 2020) and mice (Crusio and Schwegler, 1987; Bolivar et al., 2000; Sturman et al., 2018; Rudeck et al., 2020), and is considered to be one of the simplest forms of hippocampal-dependent non-associative learning (Cerbone and Sadile, 1994; Leussis and Bolivar, 2006). To avoid this potential confound, some investigators choose to test in the OFT only once, comparing experimental groups in their response to a novel environment (Washington et al., 2012; Breu et al., 2016; Islam et al., 2021). Rudeck and colleagues recently applied repeatability analysis to data acquired for 5-min sessions in the OFT for seven consecutive days, in three strains of mice (C57BL/6J, BALB/cJ, and 129S1/SvlmJ) (Rudeck et al., 2020). The authors concluded that a 3 day habituation period is sufficient to establish a stable pattern of distance traveled, although activity in the center of the apparatus was not measured. Furthermore, the variance in the data could not be explained by mouse strain or individual animal in the first 3 days of testing, and was attributed by the authors to unknown factors such as stress or anxiety (Rudeck et al., 2020). In the following days, individual differences between mice, or “personality,” was found to be the primary contributor to the variance in exploratory behavior (Rudeck et al., 2020). Although a 3-day habituation with 5-min sessions was suggested, it is unclear if a single 15-min session would have the same effect.
With repeated testing, mice may also habituate to the LDB apparatus. They learn to locate the position of the opening between the light and dark chambers and the latency to transition from the light to the dark decreases with repeated exposures (Barnes et al., 1990). However, this requires multiple trials. Holmes and colleagues showed stable baseline behavior with two exposures to the LDB (Holmes et al., 2001); Blumstein and Crawley exposed mice to six trials over a 2 week period, and found that the number of transitions between the light and dark compartments remained stable for the first three trials, and this result was independent of inter-trial interval (ranging from 1 to 7 days) (Blumstein and Crawley, 1983). A similar study, measuring exploratory activity, found that repeated daily testing was possible for 4 days (Onaivi and Martin, 1989). Accordingly, Bouwknecht and colleagues performed four LDB trials at 1-week intervals in mice, and reported little change over those four trials in the number of transitions between the light and dark chambers, the time spent in the dark chamber, and the latency to enter the dark compartment (Bouwknecht et al., 2004). Thus, it appears that stable results may be obtained for up to four trials in the LDB.
There is a wide body of literature on repeated testing in the EPM. Briefly, initial EPM experiments suggested that behavior changed little with repeated exposure to the maze (Pellow et al., 1985; Lister, 1987). Since that time, however, the “one-trial tolerance” phenomenon has been described in which after a first exposure to the EPM, open arm exploration is significantly decreased in rats and mice, and anxiolytic agents are no longer effective at increasing the amount of time in the open arms (File et al., 1990; Rodgers et al., 1992; Rodgers and Shepherd, 1993; Treit et al., 1993; Holmes and Rodgers, 1998, 1999; Zhou et al., 2015; Bourin, 2019) [but see (Schrader et al., 2018)]. There are multiple proposed explanations for the one-trial tolerance phenomenon, discussion of which goes beyond the scope of this paper [but see (Bourin, 2019) for review]. However, it is widely agreed that the EPM is typically an unsuitable assay for longitudinal studies that require multiple trials.
The EZM may be a better choice for experimental designs that require testing at multiple time points. In a direct comparison of the EPM and EZM under identical laboratory conditions, Tucker and McCabe demonstrated, in mice, that while overall activity (distance traveled) and time spent in the open regions of the apparatus decreased significantly after one trial in the EPM, these measures remained stable for at least three trials in the EZM, regardless of inter-trial interval (weekly or daily) (Tucker and McCabe, 2017). Stability of EZM behavior has also been demonstrated in rats with a testing interval of 1–2 months (Ajao et al., 2012; Kamper et al., 2013), or with a daily interval for four trials (Blokland et al., 2012). Cook and colleagues, however, found increased anxiety-like behaviors in mice following a single exposure to the EZM, though their testing conditions varied from other studies (Cook et al., 2002).
Future Directions of Behavioral Testing in TBI Research
Use of Multiple Tests and Alternative Data Analysis Methods
In addition to better reporting of injury and testing parameters, broader testing and alternative data analysis methods may aid in the assessment of anxiety. As mentioned prior, the majority of translational TBI studies employ only one unconditioned test of anxiety at a single time point to assay anxiety-like behavior. Many experts in the field of rodent behavioral testing suggest a test battery (Cryan and Holmes, 2005; Ramos, 2008). However, a test battery presents with its own set of interpretation difficulties. As described by Ramos, “we need to test it in different (behavioral) models, but by doing so at different times, we would never know, for example, whether an animal seemed fearful in the EPM and brave in the OF because of its fluctuating mood or because of construct differences between tests” (Ramos, 2008). Testing order and interval between tests are critical decisions when employing multiple tests; testing order is recommended to proceed from least stressful to most stressful. The OFT has been shown to be a relatively non-stressful test (Bodden et al., 2018), and a proper testing order for three unconditioned anxiety tests has been suggested as OFT, LDB, and last, EZM or EPM, with at least a 48-h interval between tests (Tsuda et al., 2020). Employing a combination of conditioned and unconditioned tests has also been suggested (Pentkowski et al., 2021).
Recognizing that only a subgroup of TBI patients are affected by anxiety, recent studies in the TBI literature have highlighted the usefulness of expanding data analysis methods beyond group mean comparisons (Scholten et al., 2016). Whereas clinical studies have rigorous inclusion criteria, most pre-clinical studies assessing anxiety after TBI consider the injured group as a homogeneous distribution, rather than considering variability among individuals and using that individual variability following TBI to learn about underlying pathology. Popovitz et al. tested male mice on a battery of anxiety tests (EZM, OFT, EPM) at multiple time points following CCI (weeks 1, 3, 5 and 7) and developed a multi-dimensional behavioral profiling technique to identify “resilient” and “vulnerable” subgroups of mice (Popovitz et al., 2021). The authors reported that only about 13% of the injured animals were found to be “vulnerable,” showing increased exploration of anxiogenic regions during testing compared to baseline behavior and to sham-treated animals (Popovitz et al., 2021). The behavior of the vulnerable mice had neurobiological correlates in the medial prefrontal cortex, basolateral amygdala, and ventral hippocampus; all areas that are associated with stress and anxiety (Almeida-Suhett et al., 2014; Bryant and Barker, 2020; Kenwood et al., 2021; Liu et al., 2021; McCorkle et al., 2021; Pentkowski et al., 2021).
Statz et al. employed a different “affective profiling” technique to identify “affected” and “unaffected” rats 3 weeks or 6 months following exposure to repeated blast overpressure (Statz et al., 2019). Functional outcomes were assessed in the LDB, EZM, response to fear conditioning, and molecular markers included plasma corticosterone (CORT) and stathmin-1 (a protein associated with microtubule assembly that has been shown to be elevated in the amygdala following TBI, and is elevated when there are increased levels of fear in rodents). Approximately 30–40% of the injured rats were identified as “affected,” with increased anxiety-like behaviors and/or protein levels different from control animals. As evidenced by elevated plasma CORT levels in affected animals at that time point, the stress response was associated with anxiety-related behaviors at 3 weeks following injuries, but not at 6 months. Amygdalar stathmin-1 levels were elevated at 3 weeks, whereas prefrontal cortex stathmin-1 levels were decreased at 6 weeks post-injury in affected rats (Statz et al., 2019). This study provides further evidence that behavioral measures in “affected” or vulnerable animals can correlate with biological measures in the amygdala and/or measures that suggest heightened levels of stress.
Employment of Common Data Elements for Preclinical Anxiety Studies
Preclinical assessment of anxiety symptoms in the laboratory setting has considerable challenges. As previously noted, the literature on a particular behavioral test suggests inconsistent findings between laboratories are inexplicable; questioning the utility of a particular test and its validity for the study of anxiety in preclinical models of TBI. In part this derives, however, from the many factors that affect behavioral performance, including the animal’s phenotype, species, strain, sex, age, and housing conditions, the nature of the TBI model (level of severity, consequent neuropathology, additional factors such as peripheral sites of trauma), and testing conditions [time of day, room settings, dimensions of test apparatus, handling, test sequences, and the particular chosen behavioral measure(s)]. Certain variables are impossible to control due to inherent properties of the test system’s (i.e., animal’s) biology and interaction of a particular species with the physical properties of the test system. But many of the variables may be controllable—permitting improved cross-study comparability—by standardization of procedures across laboratories; much as in clinical studies where there are great efforts expended to employ shared procedures at member clinical test sites. Yet, it has been a long-time discussion regarding the feasibility, and perhaps futility, of promulgating and “enforcing” standards for testing apparatuses, test room conditions, and the precise behavioral measures (Wahlsten, 2011).
In addition to early discussions regarding preclinical reproducibility (Landis et al., 2012; Collins and Tabak, 2014) and inclusion of both sexes (Clayton and Collins, 2014), the development of computer technology, informatics, and machine learning has been an impetus that led researchers to an alternative view. Within reasonable parameters, individual investigators can utilize conditions for which they have established standard procedures locally. The key for progress is seen with reportage at a level that allows studies to have greater comparability, permitting higher analyses to take place by the accumulation of data, by comparable links, across individual studies. For evaluating the effects of TBI and treatment for anxiety, there are key elements that can be carefully documented for the animal phenotype, the TBI model, and the behavioral test(s) employed for measuring anxiety. An initiative spearheaded by the National Institute for Neurological Disorders and Stroke began over a decade ago to develop common data elements (CDEs) that could be ascribed as essential reportable information for clinical research that would permit data sharing, collaboration, and eventual and more efficacious comparisons across studies (see Thurmond et al., 2010; Hicks et al., 2013 for descriptions of the earliest developments), with general descriptions of variables that described research participant assessment outcomes across a number of functional domains (Wilde et al., 2010). This led to efforts to expand the practice to preclinical TBI research, where the development of a “common language” could facilitate combining data sets based upon improved and more detailed description of the TBI preclinical model used for the data collection by individual laboratories (Smith et al., 2015). The goal was to define and standardize, not how individual studies were executed, but to have a system that permitted comparability across studies by application of accepted data elements. To initiate the effort, the Federal Interagency Traumatic Brain Injury Research (FITBIR) Informatics System was created as an informatics system and data repository for Preclinical Traumatic Brain1 Injury (TBI) research.
Employment of the platform has as its first step the application of definitions for each pertinent independent and dependent variable relevant to a range of variables or data about the nature of the study, the characteristics of the animals employed, the injury model, and individual measures related to outcome, such as behavioral tests. For preclinical studies this would involve description of the core CDEs, i.e., the characteristics of the animals, pertinent information related to application of the injury model, characteristics of the device employed, and assessments and outcomes (c.f., Smith et al., 2015). A major advancement in application was the subsequent review of the original effort and the development of 913 CDEs by a TBI Preclinical Working Group that has formulated critical data elements (LaPlaca et al., 2021). The Working Group then organized the CDEs into logical groups and 46 Form Structures, that broadly organized the CDEs into a Main Group, Animal and Study Data groups, Injury Models, and Assessments and Outcomes that includes 1664 CDEs (cf., Tables 1, 2 in LaPlaca et al., 2021). The FITBR site has website links to formally document animal phenotype, TBI model, as well as behavioral readouts.2 Pertinent to preclinical studies for anxiety assessment, there are specific links for the OFT, the EZM, and EPM (Table 2). There are presently no postings for the LDB; Table 3 provides an outline that parallels the Form Structures formulated by the Working Group (LaPlaca et al., 2021).
As noted, a prime goal for application of preclinical CDEs in TBI research would enhance rigor and reproducibility, as well as providing the details and procedures of each published research report, with an endpoint for better relating findings with clinical TBI phenotypes. While this may not resolve all incongruities in findings across laboratories, it is seen as a way forward for standardized and universal data collection for improvement of data quality and sharing.
Summary and Conclusion
In summary, it is difficult to make broad conclusions regarding anxiety-like states in rodents following experimental TBI. The employment of unconditioned tests has led to a wide body of literature, yet disparate results for many rodent TBI models, across multiple tests. Unconditioned tests have been criticized for their inability to discriminate between locomotion, exploration and anxiety, yet their use will continue due to their ease of use and high-throughput.
Of course, the ultimate goal is to develop treatments for anxiety disorders post-TBI. Drug discovery for psychiatric disorders is complex; the use of single behavioral tests has been of limited use in developing approved treatments for anxiety and a more complete research program with multiple tests is more often required (Cryan and Sweeney, 2011). In this context, it should be noted that OFT results should be considered preliminary in the context of anxiety research (Crawley, 2007). It has been shown that some anxiolytic agents do not increase the time spent in the center of the OFT, demonstrating this measure to have low predictive validity (Heredia et al., 2014; Thompson et al., 2015), and it is suggested that OFT results should be followed up with anxiety-specific assays such as the LDB, EZM and/or EPM (Crawley, 2007). Additional tests that are less dependent on motor output, such as the Vogel conflict test (Millan and Brocco, 2003) and stress-induced hyperthermia (Olivier et al., 2003; Adriaan Bouwknecht et al., 2007), may also be useful additions to a battery of anxiety tests following experimental TBI. Standardization of injury and behavioral techniques, comprehensive testing, analyses that identify dysfunctional anxiety states in “affected” individual animals, and correlations between behavior and neurobiological markers can add to the value of rodent models of TBI.
Author Contributions
LT conducted the literature review and writing. JM contributed to discussion and final editing. All authors contributed to the article and approved the submitted version.
Funding
This work was supported by USUHS Preclinical Behavior and Models Core (Department of Defense), 311737-1.00-66311.
Author Disclaimer
The opinions, interpretations, conclusions and recommendations are those of the authors and are not necessarily endorsed by the U.S. Army, Department of Defense, the U.S. Government or the Uniformed Services University of the Health Sciences. The use of trade names does not constitute an official endorsement or approval of the use of reagents or commercial hardware or software. This document may not be cited for purposes of advertisement.
Conflict of Interest
The authors declare that the research was conducted in the absence of any commercial or financial relationships that could be construed as a potential conflict of interest.
Publisher’s Note
All claims expressed in this article are solely those of the authors and do not necessarily represent those of their affiliated organizations, or those of the publisher, the editors and the reviewers. Any product that may be evaluated in this article, or claim that may be made by its manufacturer, is not guaranteed or endorsed by the publisher.
Footnotes
- ^ https://fitbir.nih.gov/content/preclinical-common-data-elements
- ^ https://cde.nlm.nih.gov/form/search?selectedOrg=NINDS&classification=Preclinical%20TBI
References
Adriaan Bouwknecht, J., Olivier, B., and Paylor, R. E. (2007). The stress-induced hyperthermia paradigm as a physiological animal model for anxiety: a review of pharmacological and genetic studies in the mouse. Neurosci. Biobehav. Rev. 31, 41–59. doi: 10.1016/j.neubiorev.2006.02.002
Ajao, D. O., Pop, V., Kamper, J. E., Adami, A., Rudobeck, E., Huang, L., et al. (2012). Traumatic brain injury in young rats leads to progressive behavioral deficits coincident with altered tissue properties in adulthood. J. Neurotrauma 29, 2060–2074. doi: 10.1089/neu.2011.1883
Algamal, M., Saltiel, N., Pearson, A. J., Ager, B., Burca, I., Mouzon, B., et al. (2019). Impact of repetitive mild traumatic brain injury on behavioral and hippocampal deficits in a mouse model of chronic stress. J. Neurotrauma 36, 2590–2607. doi: 10.1089/neu.2018.6314
Almeida-Suhett, C. P., Prager, E. M., Pidoplichko, V., Figueiredo, T. H., Marini, A. M., Li, Z., et al. (2014). Reduced GABAergic inhibition in the basolateral amygdala and the development of anxiety-like behaviors after mild traumatic brain injury. PLoS One 9:e102627. doi: 10.1371/journal.pone.0102627
American Psychiatric Association (2013). Diagnostic and Statistical Manual of Mental Disorders. Washington, DC: American Psychiatric Association.
Bajwa, N. M., Halavi, S., Hamer, M., Semple, B. D., Noble-Haeusslein, L. J., Baghchechi, M., et al. (2016). Mild concussion, but not moderate traumatic brain injury, is associated with long-term depression-like phenotype in mice. PLoS One 11:e0146886. doi: 10.1371/journal.pone.0146886
Barnes, N. M., Costall, B., Kelly, M. E., Onaivi, E. S., and Naylor, R. J. (1990). Ketotifen and its analogues reduce aversive responding in the rodent. Pharmacol. Biochem. Behav. 37, 785–793. doi: 10.1016/0091-3057(90)90564-x
Barretto, T. A., Park, E., Telliyan, T., Liu, E., Gallagher, D., Librach, C., et al. (2021). Vascular dysfunction after modeled traumatic brain injury is preserved with administration of umbilical cord derived mesenchymal stromal cells and is associated with modulation of the angiogenic response. J. Neurotrauma doi: 10.1089/neu.2021.0158 [Epub ahead of print].
Beitchman, J. A., Griffiths, D. R., Hur, Y., Ogle, S. B., Bromberg, C. E., Morrison, H. W., et al. (2020). Experimental traumatic brain injury induces chronic glutamatergic dysfunction in amygdala circuitry known to regulate anxiety-like behavior. Front. Neurosci. 13:1434. doi: 10.3389/fnins.2019.01434
Belzung, C. (1999). “Measuring rodent exploratory behavior,” in Techniques in the Behavioral and Neural Sciences, eds W. E. Crusio and R. T. Gerlai (Amsterdam: Elsevier), 738–749.
Belzung, C., and Griebel, G. (2001). Measuring normal and pathological anxiety-like behaviour in mice: a review. Behav. Brain Res. 125, 141–149. doi: 10.1016/s0166-4328(01)00291-1
Bhowmick, S., Malat, A., Caruso, D., Ponery, N., D’Mello, V., Finn, C., et al. (2021). Intercellular adhesion molecule-1-induced post-traumatic brain injury neuropathology in the prefrontal cortex and hippocampus leads to sensorimotor function deficits and psychological stress. eNeuro [Epub ahead of print]. doi: 10.1523/eneuro.0242-21.2021
Blokland, A., Ten Oever, S., van Gorp, D., van Draanen, M., Schmidt, T., Nguyen, E., et al. (2012). The use of a test battery assessing affective behavior in rats: order effects. Behav. Brain Res. 228, 16–21. doi: 10.1016/j.bbr.2011.11.042
Blumstein, L. K., and Crawley, J. N. (1983). Further characterization of a simple, automated exploratory model for the anxiolytic effects of benzodiazepines. Pharmacol. Biochem. Behav. 18, 37–40.
Bodden, C., Siestrup, S., Palme, R., Kaiser, S., Sachser, N., and Richter, S. H. (2018). Evidence-based severity assessment: impact of repeated versus single open-field testing on welfare in C57BL/6J mice. Behav. Brain Res. 336, 261–268. doi: 10.1016/j.bbr.2017.08.029
Bodnar, C. N., Roberts, K. N., Higgins, E. K., and Bachstetter, A. D. (2019). A systematic review of closed head injury models of mild traumatic brain injury in mice and rats. J. Neurotrauma 36, 1683–1706. doi: 10.1089/neu.2018.6127
Bolivar, V. J., Caldarone, B. J., Reilly, A. A., and Flaherty, L. (2000). Habituation of activity in an open field: a survey of inbred strains and F1 hybrids. Behav. Genet. 30, 285–293. doi: 10.1023/A:1026545316455
Bourin, M. (2019). The test retest model of anxiety: an appraisal of findings to explain benzodiazepine tolerance. Pharmacol. Biochem. Behav. 178, 39–41. doi: 10.1016/j.pbb.2017.12.009
Bourin, M., Petit-Demoulière, B., Dhonnchadha, B. N., and Hascöet, M. (2007). Animal models of anxiety in mice. Fundam. Clin. Pharmacol. 21, 567–574. doi: 10.1111/j.1472-8206.2007.00526.x
Bouwknecht, J. A., van der Gugten, J., Groenink, L., Olivier, B., and Paylor, R. E. (2004). Effects of repeated testing in two inbred strains on flesinoxan dose-response curves in three mouse models for anxiety. Eur. J. Pharmacol. 494, 35–44. doi: 10.1016/j.ejphar.2004.04.036
Braun, A. A., Skelton, M. R., Vorhees, C. V., and Williams, M. T. (2011). Comparison of the elevated plus and elevated zero mazes in treated and untreated male Sprague-Dawley rats: effects of anxiolytic and anxiogenic agents. Pharmacol. Biochem. Behav. 97, 406–415. doi: 10.1016/j.pbb.2010.09.013
Breu, M., Zhang, J., Porambo, M., Pletnikov, M. V., Goeral, K., Kakara, M., et al. (2016). Diffusion tensor imaging abnormalities in the cerebral white matter correlate with sex-dependent neurobehavioral deficits in adult mice with neonatal ischemia. Dev. Neurosci. 38, 83–95. doi: 10.1159/000442943
Bryant, K. G., and Barker, J. M. (2020). Arbitration of approach-avoidance conflict by ventral hippocampus. Front. Neurosci. 14:615337. doi: 10.3389/fnins.2020.615337
Budinich, C. S., Tucker, L. B., Lowe, D., Rosenberger, J. G., and McCabe, J. T. (2013). Short and long-term motor and behavioral effects of diazoxide and dimethyl sulfoxide administration in the mouse after traumatic brain injury. Pharmacol. Biochem. Behav. 108, 66–73. doi: 10.1016/j.pbb.2013.04.001
Cassidy, J. D., Carroll, L. J., Peloso, P. M., Borg, J., von Holst, H., Holm, L., et al. (2004). Incidence, risk factors and prevention of mild traumatic brain injury: results of the WHO collaborating centre task force on mild traumatic brain injury. J. Rehabil. Med. 43(43 Suppl.), 28–60.
Castanheira, L., Ferreira, M. F., Sebastião, A. M., and Telles-Correia, D. (2018). Anxiety assessment in pre-clinical tests and in clinical trials: a critical review. Curr. Top. Med. Chem. 18, 1656–1676. doi: 10.2174/1568026618666181115102518
Centers for Disease Control and Prevention (2015). Report to Congress on Traumatic Brain Injury in the United States: Epidemiology and Rehabilitation. Atlanta, GA: National Center for Injury Prevention and Control; Division of Unintentional Injury Prevention.
Cerbone, A., and Sadile, A. G. (1994). Behavioral habituation to spatial novelty: interference and noninterference studies. Neurosci. Biobehav. Rev. 18, 497–518. doi: 10.1016/0149-7634(94)90004-3
Chauhan, N. B., Gatto, R., and Chauhan, M. B. (2010). Neuroanatomical correlation of behavioral deficits in the CCI model of TBI. J. Neurosci. Methods 190, 1–9. doi: 10.1016/j.jneumeth.2010.04.004
Clayton, J. A., and Collins, F. S. (2014). Policy: NIH to balance sex in cell and animal studies. Nature 509, 282–283.
Collins, F. S., and Tabak, L. A. (2014). Policy: NIH plans to enhance reproducibility. Nature 505, 612–613.
Cook, M. N., Crounse, M., and Flaherty, L. (2002). Anxiety in the elevated zero-maze is augmented in mice after repeated daily exposure. Behav. Genet. 32, 113–118. doi: 10.1023/A:1015249706579
Crawley, J., and Goodwin, F. K. (1980). Preliminary report of a simple animal behavior model for the anxiolytic effects of benzodiazepines. Pharmacol. Biochem. Behav. 13, 167–170.
Crusio, W. E., and Schwegler, H. (1987). Hippocampal mossy fiber distribution covaries with open-field habituation in the mouse. Behav. Brain Res. 26, 153–158. doi: 10.1016/0166-4328(87)90163-x
Cryan, J. F., and Holmes, A. (2005). The ascent of mouse: advances in modelling human depression and anxiety. Nat. Rev. Drug Discov. 4, 775–790. doi: 10.1038/nrd1825
Cryan, J. F., and Sweeney, F. F. (2011). The age of anxiety: role of animal models of anxiolytic action in drug discovery. Br. J. Pharmacol. 164, 1129–1161. doi: 10.1111/j.1476-5381.2011.01362.x
Cunha, J. M., and Masur, J. (1978). Evaluation of psychotropic drugs with a modified open field test. Pharmacology 16, 259–267.
Das, M., Mayilsamy, K., Tang, X., Han, J. Y., Foran, E., Willing, A. E., et al. (2019). Pioglitazone treatment prior to transplantation improves the efficacy of human mesenchymal stem cells after traumatic brain injury in rats. Sci. Rep. 9:13646. doi: 10.1038/s41598-019-49428-y
Dawson, G. R., and Tricklebank, M. D. (1995). Use of the elevated plus maze in the search for novel anxiolytic agents. Trends Pharmacol. Sci. 16, 33–36.
de la Tremblaye, P. B., Wellcome, J. L., Wiley, K., Lomahan, C. A., Moschonas, E. H., Cheng, J. P., et al. (2021). Chronic unpredictable stress during adolescence protects against adult traumatic brain injury-induced affective and cognitive deficits. Brain Res. 1767:147544. doi: 10.1016/j.brainres.2021.147544
Desai, A., Chen, H., and Kim, H. Y. (2020). Multiple mild traumatic brain injuries lead to visual dysfunction in a mouse model. J. Neurotrauma 37, 286–294. doi: 10.1089/neu.2019.6602
Dobrachinski, F., Gerbatin, R. R., Sartori, G., Golombieski, R. M., Antoniazzi, A., Nogueira, C. W., et al. (2019). Guanosine attenuates behavioral deficits After traumatic brain injury by modulation of adenosinergic receptors. Mol. Neurobiol. 56, 3145–3158. doi: 10.1007/s12035-018-1296-1
Draper, K., Ponsford, J., and Schönberger, M. (2007). Psychosocial and emotional outcomes 10 years following traumatic brain injury. J. Head Trauma Rehabil. 22, 278–287. doi: 10.1097/01.HTR.0000290972.63753.a7
Dubovicky, M., and Jezova, D. (2004). Effect of chronic emotional stress on habituation processes in open field in adult rats. Ann. N. Y. Acad. Sci. 1018, 199–206. doi: 10.1196/annals.1296.023
Dwyer, B., and Katz, D. I. (2018). Postconcussion syndrome. Handb. Clin. Neurol. 158, 163–178. doi: 10.1016/b978-0-444-63954-7.00017-3
Evans, L. P., Roghair, A. M., Gilkes, N. J., and Bassuk, A. G. (2021). Visual outcomes in experimental rodent models of blast-mediated traumatic brain injury. Front. Mol. Neurosci. 14:659576. doi: 10.3389/fnmol.2021.659576
File, S. E. (2001). Factors controlling measures of anxiety and responses to novelty in the mouse. Behav. Brain Res. 125, 151–157.
File, S. E., Mabbutt, P. S., and Hitchcott, P. K. (1990). Characterisation of the phenomenon of “one-trial tolerance” to the anxiolytic effect of chlordiazepoxide in the elevated plus-maze. Psychopharmacology 102, 98–101. doi: 10.1007/BF02245751
Filgueiras, G. B., Carvalho-Netto, E. F., and Estanislau, C. (2014). Aversion in the elevated plus-maze: role of visual and tactile cues. Behav. Process. 107, 106–111. doi: 10.1016/j.beproc.2014.08.005
Fucich, E. A., Mayeux, J. P., McGinn, M. A., Gilpin, N. W., Edwards, S., and Molina, P. E. (2019). A novel role for the endocannabinoid system in ameliorating motivation for alcohol drinking and negative behavioral affect after traumatic brain injury in rats. J. Neurotrauma 36, 1847–1855. doi: 10.1089/neu.2018.5854
Fujimoto, S. T., Longhi, L., Saatman, K. E., Conte, V., Stocchetti, N., and McIntosh, T. K. (2004). Motor and cognitive function evaluation following experimental traumatic brain injury. Neurosci. Biobehav. Rev. 28, 365–378. doi: 10.1016/j.neubiorev.2004.06.002
Gold, E. M., Su, D., López-Velázquez, L., Haus, D. L., Perez, H., Lacuesta, G. A., et al. (2013). Functional assessment of long-term deficits in rodent models of traumatic brain injury. Regen. Med. 8, 483–516. doi: 10.2217/rme.13.41
Gold, E. M., Vasilevko, V., Hasselmann, J., Tiefenthaler, C., Hoa, D., Ranawaka, K., et al. (2018). Repeated mild closed head injuries induce long-term white matter pathology and neuronal loss that are correlated with behavioral deficits. ASN Neuro 10:1759091418781921. doi: 10.1177/1759091418781921
Haleem, D. J., Inam, Q.-U.-A., and Haleem, M. A. (2015). Effects of clinically relevant doses of methyphenidate on spatial memory, behavioral sensitization and open field habituation: a time related study. Behav. Brain Res. 281, 208–214. doi: 10.1016/j.bbr.2014.12.031
Hall, C. S. (1934). Emotional behavior in the rat. I. Defecation and urination as measures of individual differences in emotionality. J. Compar. Psychol. 18, 385–403.
Haller, J., Aliczki, M., and Gyimesine Pelczer, K. (2013). Classical and novel approaches to the preclinical testing of anxiolytics: a critical evaluation. Neurosci. Biobehav. Rev. 37(10 Pt 1), 2318–2330. doi: 10.1016/j.neubiorev.2012.09.001
Harro, J. (2018). Animals, anxiety, and anxiety disorders: how to measure anxiety in rodents and why. Behav. Brain Res. 352, 81–93. doi: 10.1016/j.bbr.2017.10.016
Hascoët, M., and Bourin, M. (1998). A new approach to the light/dark test procedure in mice. Pharmacol. Biochem. Behav. 60, 645–653. doi: 10.1016/s0091-3057(98)00031-8
Heredia, L., Torrente, M., Colomina, M. T., and Domingo, J. L. (2014). Assessing anxiety in C57BL/6J mice: a pharmacological characterization of the open-field and light/dark tests. J. Pharmacol. Toxicol. Methods 69, 108–114. doi: 10.1016/j.vascn.2013.12.005
Hicks, R., Giacino, J., Harrison-Felix, C., Manley, G., Valadka, A., and Wilde, E. A. (2013). Progress in developing common data elements for traumatic brain injury research: version Two-the end of the beginning. J. Neurotrauma 30, 1852–1861. doi: 10.1089/neu.2013.2938
Holmes, A., Iles, J. P., Mayell, S. J., and Rodgers, R. J. (2001). Prior test experience compromises the anxiolytic efficacy of chlordiazepoxide in the mouse light/dark exploration test. Behav. Brain Res. 122, 159–167. doi: 10.1016/S0166-4328(01)00184-X
Holmes, A., and Rodgers, R. J. (1998). Responses of Swiss-Webster mice to repeated plus-maze experience: further evidence for a qualitative shift in emotional state? Pharmacol. Biochem. Behav. 60, 473–488. doi: 10.1016/S0091-3057(98)00008-2
Holmes, A., and Rodgers, R. J. (1999). Influence of spatial and temporal manipulations on the anxiolytic efficacy of chlordiazepoxide in mice previously exposed to the elevated plus-maze. Neurosci. Biobehav. Rev. 23, 971–980. doi: 10.1016/S0149-7634(99)00030-5
Hsieh, C. L., Niemi, E. C., Wang, S. H., Lee, C. C., Bingham, D., Zhang, J., et al. (2014). CCR2 deficiency impairs macrophage infiltration and improves cognitive function after traumatic brain injury. J. Neurotrauma 31, 1677–1688. doi: 10.1089/neu.2013.3252
Islam, M., Davis, B. T. T., Kando, M. J., Mao, Q., Procissi, D., Weiss, C., et al. (2021). Differential neuropathology and functional outcome after equivalent traumatic brain injury in aged versus young adult mice. Exp. Neurol. 341:113714. doi: 10.1016/j.expneurol.2021.113714
James, S. L., Theadom, A., Ellenbogen, R. G., Bannick, M. S., Montjoy-Venning, W., Lucchesi, L. R., et al. (2019). Global, regional, and national burden of traumatic brain injury and spinal cord injury, 1990–2016: a systematic analysis for the Global Burden of Disease Study 2016. Lancet Neurol. 18, 56–87. doi: 10.1016/S1474-4422(18)30415-0
Johnson, V. E., Meaney, D. F., Cullen, D. K., and Smith, D. H. (2015). Animal models of traumatic brain injury. Handb. Clin. Neurol. 127, 115–128. doi: 10.1016/b978-0-444-52892-6.00008-8
Kamper, J. E., Pop, V., Fukuda, A. M., Ajao, D. O., Hartman, R. E., and Badaut, J. (2013). Juvenile traumatic brain injury evolves into a chronic brain disorder: behavioral and histological changes over 6months. Exp. Neurol. 250, 8–19. doi: 10.1016/j.expneurol.2013.09.016
Kane, M. J., Angoa-Perez, M., Briggs, D. I., Viano, D. C., Kreipke, C. W., and Kuhn, D. M. (2012). A mouse model of human repetitive mild traumatic brain injury. J. Neurosci. Methods 203, 41–49. doi: 10.1016/j.jneumeth.2011.09.003
Karelina, K., Schneiderman, K., Shah, S., Fitzgerald, J., Cruz, R. V., Oliverio, R., et al. (2021). Moderate intensity treadmill exercise increases survival of newborn hippocampal neurons and improves neurobehavioral outcomes after traumatic brain injury. J. Neurotrauma 38, 1858–1869. doi: 10.1089/neu.2020.7389
Kenwood, M. M., Kalin, N. H., and Barbas, H. (2021). The prefrontal cortex, pathological anxiety, and anxiety disorders. Neuropsychopharmacology [Epub ahead of print]. doi: 10.1038/s41386-021-01109-z
Kim, Y., Fu, A. H., Tucker, L. B., Liu, J., and McCabe, J. T. (2018). Characterization of controlled cortical impact devices by high-speed image analysis. J. Neurosci. Res. 96, 501–511. doi: 10.1002/jnr.24099
Kimbler, D. E., Shields, J., Yanasak, N., Vender, J. R., and Dhandapani, K. M. (2012). Activation of P2X7 promotes cerebral edema and neurological injury after traumatic brain injury in mice. PLoS One 7:e41229. doi: 10.1371/journal.pone.0041229
Koponen, S., Taiminen, T., Kurki, T., Portin, R., Isoniemi, H., Himanen, L., et al. (2006). MRI findings and Axis I and II psychiatric disorders after traumatic brain injury: a 30-year retrospective follow-up study. Psychiatry Res. 146, 263–270. doi: 10.1016/j.pscychresns.2005.05.015
Koponen, S., Taiminen, T., Portin, R., Himanen, L., Isoniemi, H., Heinonen, H., et al. (2002). Axis I and II psychiatric disorders after traumatic brain injury: a 30-year follow-up study. Am. J. Psychiatry 159, 1315–1321. doi: 10.1176/appi.ajp.159.8.1315
Kulkarni, S. K., Singh, K., and Bishnoi, M. (2007). Elevated zero maze: a paradigm to evaluate antianxiety effects of drugs. Methods Find. Exp. Clin. Pharmacol. 29, 343–348. doi: 10.1358/mf.2007.29.5.1117557
Landis, S. C., Amara, S. G., Asadullah, K., Austin, C. P., Blumenstein, R., Bradley, E. W., et al. (2012). A call for transparent reporting to optimize the predictive value of preclinical research. Nature 490, 187–191. doi: 10.1038/nature11556
Lapinlampi, N., Andrade, P., Paananen, T., Hämäläinen, E., Ekolle Ndode-Ekane, X., Puhakka, N., et al. (2020). Postinjury weight rather than cognitive or behavioral impairment predicts development of posttraumatic epilepsy after lateral fluid-percussion injury in rats. Epilepsia 61, 2035–2052. doi: 10.1111/epi.16632
LaPlaca, M. C., Huie, J. R., Alam, H. B., Bachstetter, A. D., Bayir, H., Bellgowan, P. F., et al. (2021). Pre-clinical common data elements for traumatic brain injury research: progress and use cases. J. Neurotrauma 38, 1399–1410. doi: 10.1089/neu.2020.7328
Lee, C., and Rodgers, R. J. (1990). Antinociceptive effects of elevated plus-maze exposure: influence of opiate receptor manipulations. Psychopharmacology 102, 507–513. doi: 10.1007/BF02247133
Lee, S. W., Jang, M. S., Jeong, S. H., and Kim, H. (2019). Exploratory, cognitive, and depressive-like behaviors in adult and pediatric mice exposed to controlled cortical impact. Clin. Exp. Emerg. Med. 6, 125–137. doi: 10.15441/ceem.18.019
Leussis, M. P., and Bolivar, V. J. (2006). Habituation in rodents: a review of behavior, neurobiology, and genetics. Neurosci. Biobehav. Rev. 30, 1045–1064. doi: 10.1016/j.neubiorev.2006.03.006
Lister, R. G. (1987). The use of a plus-maze to measure anxiety in the mouse. Psychopharmacology 92, 180–185. doi: 10.1007/BF00177912
Lister, R. G. (1990). Ethologically-based animal models of anxiety disorders. Pharmacol. Ther. 46, 321–340.
Liu, Q., Zhang, Z., and Zhang, W. (2021). Optogenetic dissection of neural circuits underlying stress-induced mood disorders. Front. Psychol. 12:600999. doi: 10.3389/fpsyg.2021.600999
Lyeth, B. G. (2016). Historical review of the fluid-percussion TBI model. Front. Neurol. 7:217. doi: 10.3389/fneur.2016.00217
Ma, X., Aravind, A., Pfister, B. J., Chandra, N., and Haorah, J. (2019). Animal models of traumatic brain injury and assessment of injury severity. Mol. Neurobiol. 56, 5332–5345. doi: 10.1007/s12035-018-1454-5
Malkesman, O., Tucker, L. B., Ozl, J., and McCabe, J. T. (2013). Traumatic brain injury - modeling neuropsychiatric symptoms in rodents. Front. Neurol. 4:157. doi: 10.3389/fneur.2013.00157
Management of Concussion/mTBI Working Group (2009). VA/DoD clinical practice guideline for management of concussion/mild traumatic brain injury. J. Rehabil. Res. Dev. 46, 1–68.
Mannix, R., Berglass, J., Berkner, J., Moleus, P., Qiu, J., Andrews, N., et al. (2014). Chronic gliosis and behavioral deficits in mice following repetitive mild traumatic brain injury. J. Neurosurg. 121, 1342–1350. doi: 10.3171/2014.7.jns14272
Marklund, N. (2016). Rodent models of traumatic brain injury: methods and challenges. Methods Mol. Biol. 1462, 29–46. doi: 10.1007/978-1-4939-3816-2_3
McAteer, K. M., Corrigan, F., Thornton, E., Turner, R. J., and Vink, R. (2016). Short and long term behavioral and pathological changes in a novel rodent model of repetitive mild traumatic brain injury. PLoS One 11:e0160220. doi: 10.1371/journal.pone.0160220
McCabe, J. T., and Tucker, L. B. (2020). Sex as a biological variable in preclinical modeling of blast-related traumatic brain injury. Front. Neurol. 11:541050. doi: 10.3389/fneur.2020.541050
McCorkle, T. A., Barson, J. R., and Raghupathi, R. (2021). A role for the amygdala in impairments of affective behaviors following mild traumatic brain injury. Front. Behav. Neurosci. 15:601275. doi: 10.3389/fnbeh.2021.601275
McNamara, E. H., Grillakis, A. A., Tucker, L. B., and McCabe, J. T. (2020). The closed-head impact model of engineered rotational acceleration (CHIMERA) as an application for traumatic brain injury pre-clinical research: a status report. Exp. Neurol. 333:113409. doi: 10.1016/j.expneurol.2020.113409
Millan, M. J., and Brocco, M. (2003). The Vogel conflict test: procedural aspects, γ-aminobutyric acid, glutamate and monoamines. Eur. J. Pharmacol. 463, 67–96. doi: 10.1016/S0014-2999(03)01275-5
Morriss, N., Conley, G., Hodgson, N., Boucher, M., Ospina-Mora, S., Fagiolini, M., et al. (2021). Visual dysfunction after repetitive mild traumatic brain injury in a mouse model and ramifications on behavioral metrics. J. Neurotrauma [Epub ahead of print]. doi: 10.1089/neu.2021.0165
Mouzon, B. C., Bachmeier, C., Ferro, A., Ojo, J. O., Crynen, G., Acker, C. M., et al. (2014). Chronic neuropathological and neurobehavioral changes in a repetitive mild traumatic brain injury model. Ann. Neurol. 75, 241–254. doi: 10.1002/ana.24064
Namjoshi, D. R., Cheng, W. H., Bashir, A., Wilkinson, A., Stukas, S., Martens, K. M., et al. (2017). Defining the biomechanical and biological threshold of murine mild traumatic brain injury using CHIMERA (Closed head impact model of engineered rotational acceleration). Exp. Neurol. 292, 80–91. doi: 10.1016/j.expneurol.2017.03.003
Namjoshi, D. R., Cheng, W. H., McInnes, K. A., Martens, K. M., Carr, M., Wilkinson, A., et al. (2014). Merging pathology with biomechanics using CHIMERA (Closed-Head Impact Model of Engineered Rotational Acceleration): a novel, surgery-free model of traumatic brain injury. Mol. Neurodegener. 9:55. doi: 10.1186/1750-1326-9-55
Nolan, A., Hennessy, E., Krukowski, K., Guglielmetti, C., Chaumeil, M. M., Sohal, V. S., et al. (2018). Repeated mild head injury leads to wide-ranging deficits in higher-order cognitive functions associated with the prefrontal cortex. J. Neurotrauma 35, 2425–2434. doi: 10.1089/neu.2018.5731
O’Brien, L. D., Smith, T. L., Donvito, G., Cravatt, B. F., Newton, J., Spiegel, S., et al. (2021). Diacylglycerol lipase-β knockout mice display a sex-dependent attenuation of traumatic brain injury-induced mortality with no impact on memory or other functional consequences. Cannabis Cannabinoid Res. [Epub ahead of print]. doi: 10.1089/can.2020.0175
Olivier, B., Zethof, T., Pattij, T., van Boogaert, M., van Oorschot, R., Leahy, C., et al. (2003). Stress-induced hyperthermia and anxiety: pharmacological validation. Eur. J. Pharmacol. 463, 117–132. doi: 10.1016/S0014-2999(03)01326-8
Onaivi, E. S., and Martin, B. R. (1989). Neuropharmacological and physiological validation of a computer-controlled two-compartment black and white box for the assessment of anxiety. Prog. Neuropsychopharmacol. Biol. Psychiatry 13, 963–976. doi: 10.1016/0278-5846(89)90047-x
Osier, N. D., Carlson, S. W., DeSana, A., and Dixon, C. E. (2015). Chronic histopathological and behavioral outcomes of experimental traumatic brain injury in adult male animals. J. Neurotrauma 32, 1861–1882. doi: 10.1089/neu.2014.3680
Osier, N. D., and Dixon, C. E. (2016). The controlled cortical impact model: applications, considerations for researchers, and future directions. Front. Neurol. 7:134. doi: 10.3389/fneur.2016.00134
Pellow, S., Chopin, P., File, S. E., and Briley, M. (1985). Validation of open:closed arm entries in an elevated plus-maze as a measure of anxiety in the rat. J. Neurosci. Methods 14, 149–167. doi: 10.1016/0165-0270(85)90031-7
Pentkowski, N. S., Rogge-Obando, K. K., Donaldson, T. N., Bouquin, S. J., and Clark, B. J. (2021). Anxiety and Alzheimer’s disease: behavioral analysis and neural basis in rodent models of Alzheimer’s-related neuropathology. Neurosci. Biobehav. Rev. 127, 647–658. doi: 10.1016/j.neubiorev.2021.05.005
Polinder, S., Cnossen, M. C., Real, R. G. L., Covic, A., Gorbunova, A., Voormolen, D. C., et al. (2018). A multidimensional approach to post-concussion symptoms in mild traumatic brain injury. Front. Neurol. 9:1113. doi: 10.3389/fneur.2018.01113
Popovitz, J., Mysore, S. P., and Adwanikar, H. (2019). Long-term effects of traumatic brain injury on anxiety-like behaviors in mice: behavioral and neural correlates. Front. Behav. Neurosci. 13:6. doi: 10.3389/fnbeh.2019.00006
Popovitz, J., Mysore, S. P., and Adwanikar, H. (2021). Neural markers of vulnerability to anxiety outcomes after traumatic brain injury. J. Neurotrauma 38, 1006–1022. doi: 10.1089/neu.2020.7320
Poveda, C. M., Popović, N., Morales-Delgado, N., De la Cruz-Sánchez, E., Caballero Bleda, M., and Popović, M. (2020). The diurnal variation of open-field habituation in rats. Behav. Process. 178:104186. doi: 10.1016/j.beproc.2020.104186
Povlishock, J. (2016). The history and evolution of experimental traumatic brain injury models. Methods Mol. Biol. 1462, 3–7. doi: 10.1007/978-1-4939-3816-2_1
Prut, L., and Belzung, C. (2003). The open field as a paradigm to measure the effects of drugs on anxiety-like behaviors: a review. Eur. J. Pharmacol. 463, 3–33.
Ramos, A. (2008). Animal models of anxiety: do I need multiple tests? Trends Pharmacol. Sci. 29, 493–498. doi: 10.1016/j.tips.2008.07.005
Ritter, K., Jung, K., Dolderer, C., Appel, D., Oswald, C. C., Ritz, U., et al. (2021). Early reciprocal effects in a murine model of traumatic brain injury and femoral fracture. Mediators Inflamm. 2021:8835730. doi: 10.1155/2021/8835730
Rodgers, R. J., Cao, B. J., Dalvi, A., and Holmes, A. (1997). Animal models of anxiety: an ethological perspective. Braz. J. Med. Biol. Res. 30, 289–304. doi: 10.1590/S0100-879X1997000300002
Rodgers, R. J., Lee, C., and Shepherd, J. K. (1992). Effects of diazepam on behavioural and antinociceptive responses to the elevated plus-maze in male mice depend upon treatment regimen and prior maze experience. Psychopharmacology 106, 102–110. doi: 10.1007/BF02253596
Rodgers, R. J., and Shepherd, J. K. (1993). Influence of prior maze experience on behaviour and response to diazepam in the elevated plus-maze and light/dark tests of anxiety in mice. Psychopharmacology 113, 237–242. doi: 10.1007/BF02245704
Rodriguez, U. A., Zeng, Y., Deyo, D., Parsley, M. A., Hawkins, B. E., Prough, D. S., et al. (2018). Effects of mild blast traumatic brain injury on cerebral vascular, histopathological, and behavioral outcomes in rats. J. Neurotrauma 35, 375–392. doi: 10.1089/neu.2017.5256
Rudeck, J., Vogl, S., Banneke, S., Schönfelder, G., and Lewejohann, L. (2020). Repeatability analysis improves the reliability of behavioral data. PLoS One 15:e0230900. doi: 10.1371/journal.pone.0230900
Saatman, K. E., Feeko, K. J., Pape, R. L., and Raghupathi, R. (2006). Differential behavioral and histopathological responses to graded cortical impact injury in mice. J. Neurotrauma 23, 1241–1253. doi: 10.1089/neu.2006.23.1241
Scholten, A. C., Haagsma, J. A., Cnossen, M. C., Olff, M., van Beeck, E. F., and Polinder, S. (2016). Prevalence of and risk factors for anxiety and depressive disorders after traumatic brain injury: a systematic review. J. Neurotrauma 33, 1969–1994. doi: 10.1089/neu.2015.4252
Schrader, A. J., Taylor, R. M., Lowery-Gionta, E. G., and Moore, N. L. T. (2018). Repeated elevated plus maze trials as a measure for tracking within-subjects behavioral performance in rats (Rattus norvegicus). PLoS One 13:e0207804. doi: 10.1371/journal.pone.0207804
Seibenhener, M. L., and Wooten, M. C. (2015). Use of the Open Field Maze to measure locomotor and anxiety-like behavior in mice. J. Vis. Exp. 96:e52434. doi: 10.3791/52434
Semple, B. D., Zamani, A., Rayner, G., Shultz, S. R., and Jones, N. C. (2019). Affective, neurocognitive and psychosocial disorders associated with traumatic brain injury and post-traumatic epilepsy. Neurobiol. Dis. 123, 27–41. doi: 10.1016/j.nbd.2018.07.018
Shepherd, J., Grewal, S., Fletcher, A., Bill, D., and Dourish, C. (1994). Behavioural and pharmacological characterisation of the elevated “zero-maze” as an animal model of anxiety. Psychopharmacology 116, 56–64. doi: 10.1007/bf02244871
Siebold, L., Obenaus, A., and Goyal, R. (2018). Criteria to define mild, moderate, and severe traumatic brain injury in the mouse controlled cortical impact model. Exp. Neurol. 310, 48–57. doi: 10.1016/j.expneurol.2018.07.004
Sierra-Mercado, D., McAllister, L. M., Lee, C. C., Milad, M. R., Eskandar, E. N., and Whalen, M. J. (2015). Controlled cortical impact before or after fear conditioning does not affect fear extinction in mice. Brain Res. 1606, 133–141. doi: 10.1016/j.brainres.2015.02.031
Skotak, M., Townsend, M. T., Ramarao, K. V., and Chandra, N. (2019). A comprehensive review of experimental rodent models of repeated blast TBI. Front. Neurol. 10:1015. doi: 10.3389/fneur.2019.01015
Smith, D. H., Hicks, R. R., Johnson, V. E., Bergstrom, D. A., Cummings, D. M., Noble, L. J., et al. (2015). Pre-clinical traumatic brain injury common data elements: toward a common language across laboratories. J. Neurotrauma 32, 1725–1735. doi: 10.1089/neu.2014.3861
Statz, J. K., Ciarlone, S. L., Goodrich, J. A., McCarron, R. M., Walker, P. B., Norris, J. N., et al. (2019). Affective profiling for anxiety-like behavior in a rodent model of mTBI. Behav. Brain Res. 368:111895. doi: 10.1016/j.bbr.2019.04.009
Steimer, T. (2002). The biology of fear- and anxiety-related behaviors. Dialog. Clin. Neurosci. 4, 231–249. doi: 10.31887/DCNS.2002.4.3/tsteimer
Steimer, T. (2011). Animal models of anxiety disorders in rats and mice: some conceptual issues. Dialog. Clin. Neurosci. 13, 495–506.
Stielper, Z. F., Fucich, E. A., Middleton, J. W., Hillard, C. J., Edwards, S., Molina, P. E., et al. (2021). Traumatic brain injury and alcohol drinking alter basolateral amygdala endocannabinoids in female rats. J. Neurotrauma 38, 422–434. doi: 10.1089/neu.2020.7175
Sturman, O., Germain, P. L., and Bohacek, J. (2018). Exploratory rearing: a context- and stress-sensitive behavior recorded in the open-field test. Stress 21, 443–452. doi: 10.1080/10253890.2018.1438405
Tan, X. L., Zheng, P., Wright, D. K., Sun, M., Brady, R. D., Liu, S., et al. (2020). The genetic ablation of tau improves long-term, but not short-term, functional outcomes after experimental traumatic brain injury in mice. Brain Inj. 34, 131–139. doi: 10.1080/02699052.2019.1667539
Tapp, Z. M., Kumar, J. E., Witcher, K. G., Atluri, R. R., Velasquez, J. A., O’Neil, S. M., et al. (2020). Sleep disruption exacerbates and prolongs the inflammatory response to traumatic brain injury. J. Neurotrauma 37, 1829–1843. doi: 10.1089/neu.2020.7010
Tchantchou, F., Miller, C., Goodfellow, M., Puche, A., and Fiskum, G. (2021). Hypobaria-induced oxidative stress facilitates homocysteine transsulfuration and promotes glutathione oxidation in rats with mild traumatic brain injury. J. Cent. Nerv. Syst. Dis. 13:1179573520988193.
Tchantchou, F., Tucker, L. B., Fu, A. H., Bluett, R. J., McCabe, J. T., Patel, S., et al. (2014). The fatty acid amide hydrolase inhibitor PF-3845 promotes neuronal survival, attenuates inflammation and improves functional recovery in mice with traumatic brain injury. Neuropharmacology 85, 427–439. doi: 10.1016/j.neuropharm.2014.06.006
Thompson, T., Grabowski-Boase, L., and Tarantino, L. M. (2015). Prototypical anxiolytics do not reduce anxiety-like behavior in the open field in C57BL/6J mice. Pharmacol. Biochem. Behav. 133, 7–17. doi: 10.1016/j.pbb.2015.03.011
Thurmond, V. A., Hicks, R., Gleason, T., Miller, A. C., Szuflita, N., Orman, J., et al. (2010). Advancing integrated research in psychological health and traumatic brain injury: common data elements. Arch. Phys. Med. Rehabil. 91, 1633–1636. doi: 10.1016/j.apmr.2010.06.034
Treit, D., Menard, J., and Royan, C. (1993). Anxiogenic stimuli in the elevated plus-maze. Pharmacol. Biochem. Behav. 44, 463–469. doi: 10.1016/0091-3057(93)90492-C
Tsuda, M. C., Mahdi, S., Namchuk, A., Wu, T. J., and Lucki, I. (2020). Vendor differences in anxiety-like behaviors in female and male Sprague Dawley rats. Physiol. Behav. 227:113131. doi: 10.1016/j.physbeh.2020.113131
Tucker, L., and McCabe, J. (2017). Behavior of male and female C57BL/6J mice is more consistent with repeated trials in the elevated zero maze than in the elevated plus maze. Front. Behav. Neurosci. 11:13. doi: 10.3389/fnbeh.2017.00013
Tucker, L. B., Burke, J. F., Fu, A. H., and McCabe, J. T. (2017). Neuropsychiatric symptom modeling in male and female C57BL/6J mice after experimental traumatic brain injury. J. Neurotrauma 34, 890–905. doi: 10.1089/neu.2016.4508
Tucker, L. B., Fu, A. H., and McCabe, J. T. (2021). Hippocampal-dependent cognitive dysfunction following repeated diffuse rotational brain injury in male and female mice. J. Neurotrauma 38, 1585–1606. doi: 10.1089/neu.2021.0025
Tucker, L. B., Velosky, A. G., Fu, A. H., and McCabe, J. T. (2019). Chronic neurobehavioral sex differences in a murine model of repetitive concussive brain injury. Front. Neurol. 10:509. doi: 10.3389/fneur.2019.00509
Vu, P. A., McNamara, E. H., Liu, J., Tucker, L. B., Fu, A. H., and McCabe, J. T. (2020). Behavioral responses following repeated bilateral frontal region closed head impacts and fear conditioning in male and female mice. Brain Res. 1750:147147. doi: 10.1016/j.brainres.2020.147147
Wahlsten, D. (2011). Mouse Behavioral Testing: How to Use Mice in Behavioral Neuroscience. London: Academic Press.
Washington, P. M., Forcelli, P. A., Wilkins, T., Zapple, D. N., Parsadanian, M., and Burns, M. P. (2012). The effect of injury severity on behavior: a phenotypic study of cognitive and emotional deficits after mild, moderate, and severe controlled cortical impact injury in mice. J. Neurotrauma 29, 2283–2296. doi: 10.1089/neu.2012.2456
Watanabe, J., Shetty, A. K., Hattiangady, B., Kim, D.-K., Foraker, J. E., Nishida, H., et al. (2013). Administration of TSG-6 improves memory after traumatic brain injury in mice. Neurobiol. Dis. 59, 86–99. doi: 10.1016/j.nbd.2013.06.017
Weiss, S. M., Wadsworth, G., Fletcher, A., and Dourish, C. T. (1998). Utility of ethological analysis to overcome locomotor confounds in elevated maze models of anxiety. Neurosci. Biobehav. Rev. 23, 265–271.
Wilde, E. A., Whiteneck, G. G., Bogner, J., Bushnik, T., Cifu, D. X., Dikmen, S., et al. (2010). Recommendations for the use of common outcome measures in traumatic brain injury research. Arch. Phys. Med. Rehabil. 91, 1650–1660. doi: 10.1016/j.apmr.2010.06.033
Xiong, Y., Mahmood, A., and Chopp, M. (2013). Animal models of traumatic brain injury. Nat. Rev. Neurosci. 14, 128–142. doi: 10.1038/nrn3407
Xu, L., Nguyen, J. V., Lehar, M., Menon, A., Rha, E., Arena, J., et al. (2016). Repetitive mild traumatic brain injury with impact acceleration in the mouse: multifocal axonopathy, neuroinflammation, and neurodegeneration in the visual system. Exp. Neurol. 275(Pt 3), 436–449. doi: 10.1016/j.expneurol.2014.11.004
Yu, F., Wang, Z., Tchantchou, F., Chiu, C. T., Zhang, Y., and Chuang, D. M. (2012). Lithium ameliorates neurodegeneration, suppresses neuroinflammation, and improves behavioral performance in a mouse model of traumatic brain injury. J. Neurotrauma 29, 362–374. doi: 10.1089/neu.2011.1942
Keywords: anxiety, brain injury, behavior, common data elements, open field, light-dark box, elevated plus maze, elevated zero maze
Citation: Tucker LB and McCabe JT (2021) Measuring Anxiety-Like Behaviors in Rodent Models of Traumatic Brain Injury. Front. Behav. Neurosci. 15:682935. doi: 10.3389/fnbeh.2021.682935
Received: 19 March 2021; Accepted: 06 October 2021;
Published: 29 October 2021.
Edited by:
Cole Vonder Haar, The Ohio State University, United StatesReviewed by:
Aric Flint Logsdon, University of Washington, United StatesEleni Konsolaki, American College of Greece, Greece
Copyright © 2021 Tucker and McCabe. This is an open-access article distributed under the terms of the Creative Commons Attribution License (CC BY). The use, distribution or reproduction in other forums is permitted, provided the original author(s) and the copyright owner(s) are credited and that the original publication in this journal is cited, in accordance with accepted academic practice. No use, distribution or reproduction is permitted which does not comply with these terms.
*Correspondence: Joseph T. McCabe, Sm9zZXBoLk1jQ2FiZUB1c3Vocy5lZHU=