- 1Department of Neurobiology and Anatomy, Wake Forest School of Medicine, Winston-Salem, NC, United States
- 2Department of Biotechnical and Clinical Laboratory Sciences, University at Buffalo, State University of New York, Buffalo, NY, United States
- 3Department of Pharmacology and Toxicology, University at Buffalo, State University of New York, Buffalo, NY, United States
- 4Department of Physiology and Pharmacology, Wake Forest School of Medicine, Winston-Salem, NC, United States
The current rodent study applied in vivo fast-scan cyclic voltammetry (FSCV), paired with a pharmacological approach, to measure the release of the catecholamines (CA) dopamine (DA) and norepinephrine (NE) in the basolateral amygdala (BLA) following locus coeruleus (LC) stimulation. The primary goal was to determine if exposure to either social (social defeat) or non-social (forced swim) stress altered LC-evoked catecholamine release dynamics in the BLA. We used idazoxan (α2 adrenergic receptor antagonist) and raclopride (D2 dopamine receptor antagonist) to confirm the presence of NE and DA, respectively, in the measured CA signal. In non-stressed rats, injection of idazoxan, but not raclopride, resulted in a significant increase in the detected CA signal, indicating the presence of NE but not DA. Following exposure to either stress paradigm, the measured CA release was significantly greater after injection of either drug, suggesting the presence of both NE and DA in the LC-induced CA signal after social or non-social stress. Furthermore, acute administration of alcohol significantly decreased the CA signal in stressed rats, while it did not have an effect in naïve animals. Together, these data reveal that, while LC stimulation primarily elicits NE release in the BLA of control animals, both social and non-social stress unmask a novel dopaminergic component of LC catecholamine signaling. Future studies will be needed to identify the specific neural mechanism(s) responsible for these plastic changes in LC-BLA catecholamine signaling and to assess the possible contribution of these changes to the maladaptive behavioral phenotypes that develop following exposure to these stressors.
Introduction
Mood and anxiety disorders are highly comorbid with alcohol use disorder (AUD; Grant et al., 2004). For example, those diagnosed with post-traumatic stress disorder are three times more likely to develop an AUD (Kessler et al., 1997). Even in individuals without a formal diagnosis, alcohol is a common tool for those with self-reported social anxiety to cope with stressful situations. These people are more likely to consume alcohol to feel more comfortable socially (Thomas et al., 2003). Despite the strong relationship between stress and alcohol, much remains unknown about how one can alter the neurobiological consequences of the other.
The locus coeruleus (LC) is a key brainstem nucleus involved in stress and arousal (Szabadi, 2013; Naegeli et al., 2018; Daviu et al., 2019). This pontine structure is the primary source of noradrenergic projections in the central nervous system which target many regions throughout the brain, such as the amygdala, thalamus, hypothalamus, and prefrontal cortex, associating the LC with myriad behavioral, motor, and sensory processes (Samuels and Szabadi, 2008; Deal et al., 2019). In particular, the projection from the LC to the basolateral amygdala (BLA) has been proposed as an important pathway in anxiety-related processes and behaviors. Recent work has shown that optogenetic activation of this pathway produced anxiety-like behavior in rats as demonstrated by a reduction in time spent in the center of an open field test and a reduction in time spent in the open portions of an elevated zero maze (McCall et al., 2017). Moreover, it was recently revealed that the LC-norepinephrine (NE) circuitry can bidirectionally control alcohol-drinking behaviors through different patterns of optoactivation (Deal A. L. et al., 2020).
On other hand, stress has been shown to be a key influencer of catecholamine (CA) activity and dynamics throughout the brain (Tanaka et al., 1991; Ferry et al., 1999; Inglis and Moghaddam, 1999; Anstrom et al., 2009; Karkhanis et al., 2015; Rajbhandari et al., 2015; Holly and Miczek, 2016; Deal et al., 2018; Giustino et al., 2020). For example, studies using ex vivo fast-scan cyclic voltammetry (FSCV) have reported that adolescent social isolation stress (Yorgason et al., 2013, 2016) as well as social defeat stress (Deal et al., 2018) resulted in increased dopamine (DA) release and its consequent reuptake in striatal subregions. Furthermore, social isolation stress during adolescence was found to elicit lasting changes to DA and DA transporters in the BLA, specifically a decrease in DA levels as measured by microdialysis and an increase in DA transporters in adulthood (Karkhanis et al., 2015). These findings are further reinforced by in vivo voltammetric experiments demonstrating an increased uptake rate and decreased release of NE in the bed nucleus of the stria terminals in rats who experienced social isolation (Fox et al., 2015). Notably, no studies have examined post-stress catecholamine dynamics within the LC-BLA circuitry or whether stress-related alterations in this pathway alter the effects of alcohol on DA and NE transmission.
To explore these questions, we used in vivo FSCV, a methodology that permits the real-time detection of extracellular DA and NE concentrations at terminals following electrical stimulation of cell body regions (Budygin et al., 2000, 2007, 2017; Mateo et al., 2004; Oleson et al., 2009; Park et al., 2011, 2012; Fox et al., 2015, 2016). This approach allowed us to examine the effects of two different stressors, repeated social defeat stress (RSDS) and forced swim test (FST), on LC-evoked CA release in the BLA and the sensitivity of the CA signal to acute alcohol. These stress paradigms have been shown to significantly elevate serum corticosterone levels in rats, a key marker of stress circuitry activity (Báez and Volosin, 1994; Miczek et al., 1999; Covington and Miczek, 2005; Barnum et al., 2007; Hueston et al., 2011; Moravcova et al., 2021). After first confirming the CA signal, we pharmacologically identified the components of the CA signal in stressed and non-stressed subjects as NE or DA by injecting either idazoxan (α2 adrenergic receptor antagonist) or raclopride [dopamine (DA) D2 receptor antagonist], respectively, and determined whether the evoked CA signal was altered by acute alcohol.
Materials and Methods
Animals
Adult male Sprague–Dawley rats (300–350 g) were used as intruder (stressed) subjects and retired breeder male Long Evans rats (>450 g) served as resident (aggressor) rats (Wood et al., 2010, 2013; Arora et al., 2019). The utilization of different strains resulted in a more robust stress environment due to the aggressive nature of the Long Evans rat (Henry et al., 1993; Snyder et al., 2018). A total of 28 Sprague–Dawley and 6 Long Evans rats were used during this study. Animals were kept on a 12-h light/dark cycle with food and water available ad libitum. Animal handling and all procedures were conducted in accordance with the National Institutes of Health Guide for the Care and Use of Laboratory Animals. All procedures were approved by the Wake Forest University School of Medicine Institutional Animal Care and Use Committee (protocol A19-190).
Repeated Social Defeat Stress
Intruder rats were exposed to five 45-min social defeat sessions prior to use for voltammetric recordings (or remained in their home cage to serve as a control). For five consecutive days, intruders were acclimated for 1 h to the testing room and then placed in the cage of a larger, aggressive resident male (see Figure 1 for experimental design). The rats were allowed to physically interact for 15 min. If blood was observed following a physical bout, the injury was assessed by an observing experimenter for severity. Minor injury resulted in immediate transferal to a protective mesh cage within the resident cage for the remainder of the session. Major injuries, those requiring suturing or other invasive interventions, would have resulted in removal of the intruder rat from the study (no major injuries occurred and thus no rats were excluded from the experiment). Typical sessions included several aggressive physical interactions that resulted in the intruder rat exhibiting subordinate behavior through assuming a supine position. At the conclusion of the physical interaction time, the remainder of the session (30 min) took place while the intruder was protected within a wire mesh cage inside of the resident cage. Visual, auditory, and olfactory signal exposure was still possible during this time.
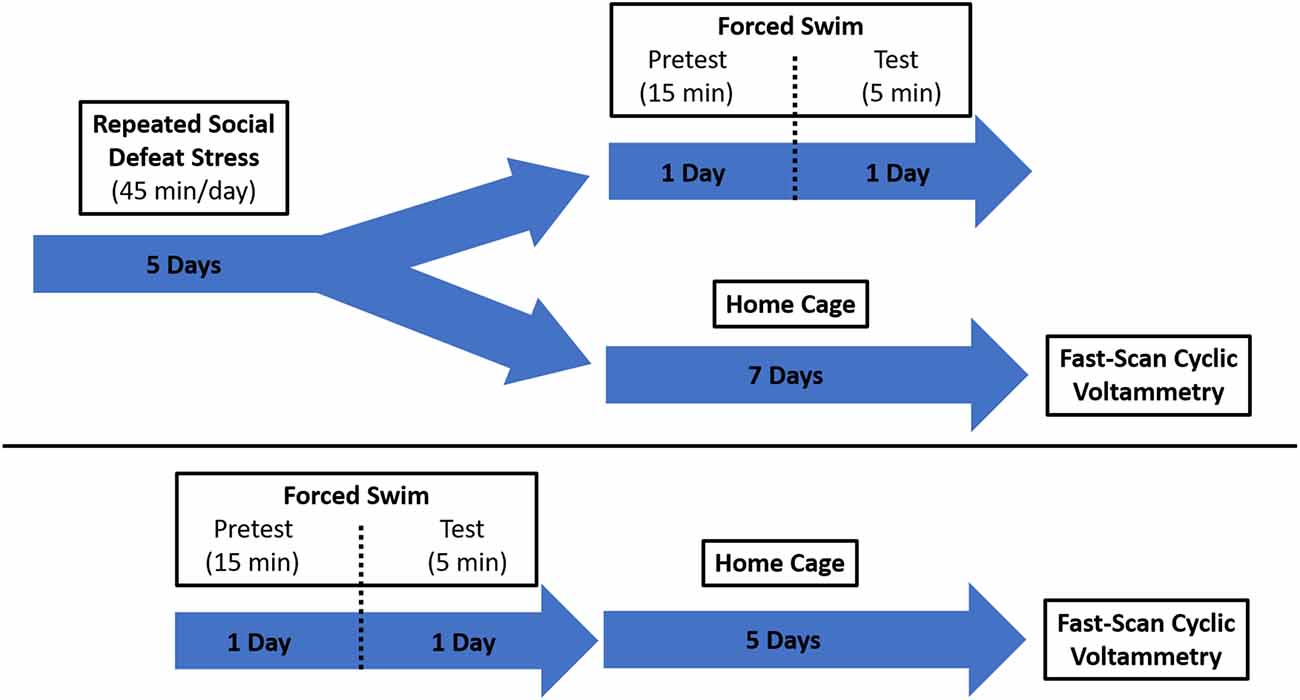
Figure 1. Schematic showing the experimental design. Two groups of rats were exposed to different stress paradigms, social defeat and forced swim. They were then evaluated for protracted effects of stress on catecholamine dynamics using fast-scan cyclic voltammetry (FSCV).
Forced Swim Test
Rats were forced to swim as described previously (Porsolt et al., 1978). Briefly, animals were allowed to acclimate to the testing room for 1 h and then placed in a transparent acrylic cylinder (30 × 50 cm) filled with 40 cm of water (25 ± 1°C) for 15 min (Linthorst et al., 2008; Bogdanova et al., 2013). One day later, the rats were placed in the water-filled cylinder again for 5 min. The rats were allowed to dry off in their home cage, which was placed on a heating pad, for at least 1 h before the cage was returned to the colony room. The cylinder was cleaned with soap and water after each rat.
A separate group of RSDS rats underwent the FST procedure immediately following the last day of social defeat exposure to behaviorally verify a stress-like phenotype. These rats were not included in subsequent voltammetry experiments. Video recordings were made for each rat on both days of FST exposure. Day 2 (test day) recordings were analyzed by an experimenter blinded to the condition and scored for bouts of immobility. The experimenter evaluated behavior every 5 s for the duration of the test (5 min) as either swimming or immobile (Deal A. W. et al., 2020).
Fast-Scan Cyclic Voltammetry
Rats were taken 1 week after the final defeat session or 5 days after the second forced swim session, anesthetized via urethane injection (1.35 g/kg, i.p.), and secured in a stereotaxic frame. Holes were drilled to allow for the placement of a carbon fiber recording electrode in the basolateral amygdala (from bregma: AP −2.9; ML + 4.6; DV −8.0), a bipolar stimulating electrode in the ipsilateral locus coeruleus (AP −9.8; ML + 1.3; DV −7.2), and a Ag/AgCl reference electrode in the contralateral hemisphere. The reference electrode was connected to a voltammetric amplifier (UNC Electronics Design Facility, Chapel Hill, NC, USA). The carbon fiber microelectrode (exposed fiber length: 75–100 μm; diameter: 6 μm) was connected to the voltammetric amplifier and secured to the stereotaxic frame arm. Stimulations were made every 10 min by an electrical pulse. Extracellular CA was recorded at the carbon fiber electrode every 100 ms for 15 s by applying a triangular waveform (−0.4 V to +1.3 V and back to −0.4 V vs Ag/AgCl, 400 V/s). The catecholamine signal was identified by observing background-subtracted cyclic voltammograms characterized by oxidation and reduction peaks occurring at +0.6 and −0.2 V, respectively. A stable baseline signal was established (three consecutive recordings within 10% variability) and an average baseline value was calculated. Immediately after establishing a baseline, an injection (i.p.) of saline, raclopride (dopamine D2 receptor antagonist; 2 mg/kg), idazoxan (α2 adrenergic receptor antagonist; 5 mg/kg), or alcohol (2 g/kg ethanol) was administered. These idazoxan and raclopride doses effectively increase an electrically-evoked catecholamine efflux clarifying whether DA (raclopride) or NE (idazoxan) is detected in the rat brain with FSCV (Park et al., 2015; Fox et al., 2016; Mikhailova et al., 2019; Deal et al., 2018; Deal A. L. et al., 2020). Some rats received more than one drug during the FSCV experiment. In such cases, the CA signal was allowed to completely return to pre-injection levels before a new baseline was established. Additionally, the order of injections was balanced to prevent any confounding effects of drug interactions. Voltammetric recordings were taken for 60 min after injection. Data were digitized (National Instruments, Austin, TX, USA) and stored on a computer. Each carbon fiber electrode was calibrated after use in an experiment in a flow injection analysis system. Calibrations were performed in triplicate using a known concentration (10 μM) of DA (Sigma–Aldrich, St. Louis, MO, USA) and calibrated again using a known concentration (10 μM) of NE (Sigma–Aldrich, St. Louis, MO, USA). The voltammetric current was measured at the peak potential.
Histology
Verification of electrode placements was performed as previously described (Park et al., 2010; Budygin et al., 2012). Briefly, a constant current (20 μA, 10 s) was applied to the carbon fiber electrode to create a lesion at the site of recording. Brains were removed and submerged in 10% formaldehyde for a minimum of 3 days. After adequate fixation, the brains were coronally sliced at a thickness of 40–50 μm using a cryostat. Once the slices were mounted on slides, they were stained with 0.2% thionine and cover slipped before imaging was performed using a light microscope. Representative slices from two different rats demonstrating lesions at the site of the recording electrode are shown with accompanying atlas diagrams (Figure 2).
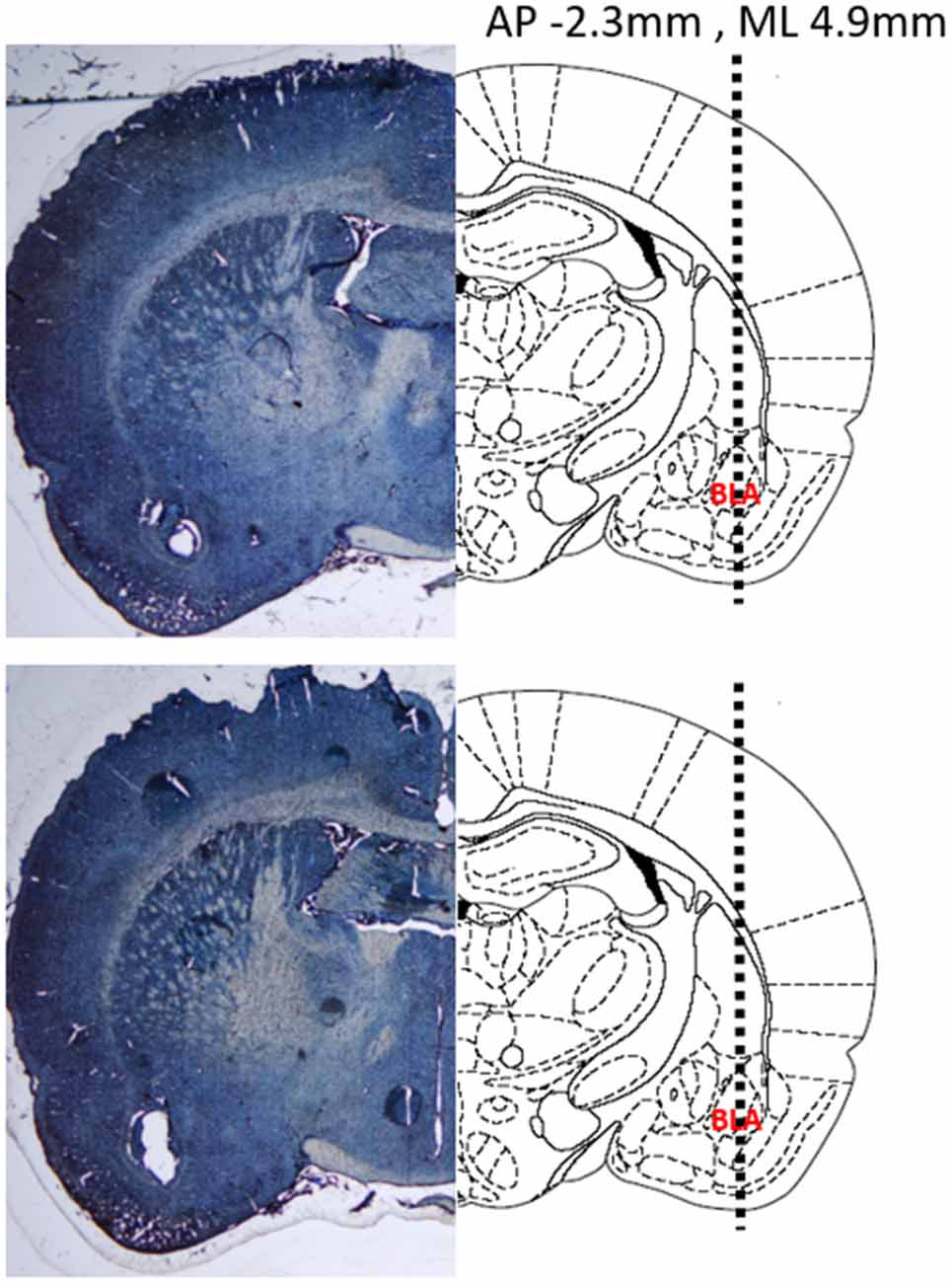
Figure 2. Representative coronal sections demonstrating the location of the recording electrode in two different rat brains. (Left) Thionine stained brain slices showing a lesion at the recording site in the basolateral amygdala (BLA). (Right) Atlas diagrams with the BLA labeled and a dashed line showing the insertion trajectory of the recording electrode. Coordinates provided are relative to Bregma. Diagram adapted from Paxinos and Watson (2004).
Data Analysis
Data were analyzed using GraphPad Prism (GraphPad Software version 7.04, San Diego, CA, USA). Unpaired t-test, repeated measures two-way ANOVAs, and Tukey’s multiple comparisons tests were used to analyze data where appropriate. Data are presented as mean ± SEM and the criterion for significance was set at p < 0.05.
Results
Electrical Stimulation of LC Elicits NE Release in the BLA
We have previously shown that optogenetic activation of the LC results in a robust noradrenergic signal in the BLA, as detected by FSCV (Deal A. L. et al., 2020). Here, we evoked release by electrical LC stimulation that resulted in a consistent, robust, frequency-dependent signal in the BLA which matched the characteristics of CA release (Figure 3). Next, in order to identify the constitutive components of this signal, a pharmacological approach was used. More specifically, doses of idazoxan (n = 5) or raclopride (n = 5) were administered to determine the presence of norepinephrine or dopamine, respectively, compared to a saline control (n = 7; Figure 4). A repeated measure two-way ANOVA found the main effect of drug (F(2, 14) = 20.28; p < 0.0001), time (F(8, 112) = 9.354; p < 0.0001), and a significant interaction (F(16, 112) = 9.101; p < 0.0001). A follow-up post hoc Tukey’s multiple comparison test found that idazoxan significantly increased the measured CA signal compared to saline (p < 0.0001) and raclopride (p < 0.001) and there was no significant difference after raclopride injection (p = 0.718). These results demonstrate that NE is the predominant CA evoked in the BLA following LC electrical stimulation.
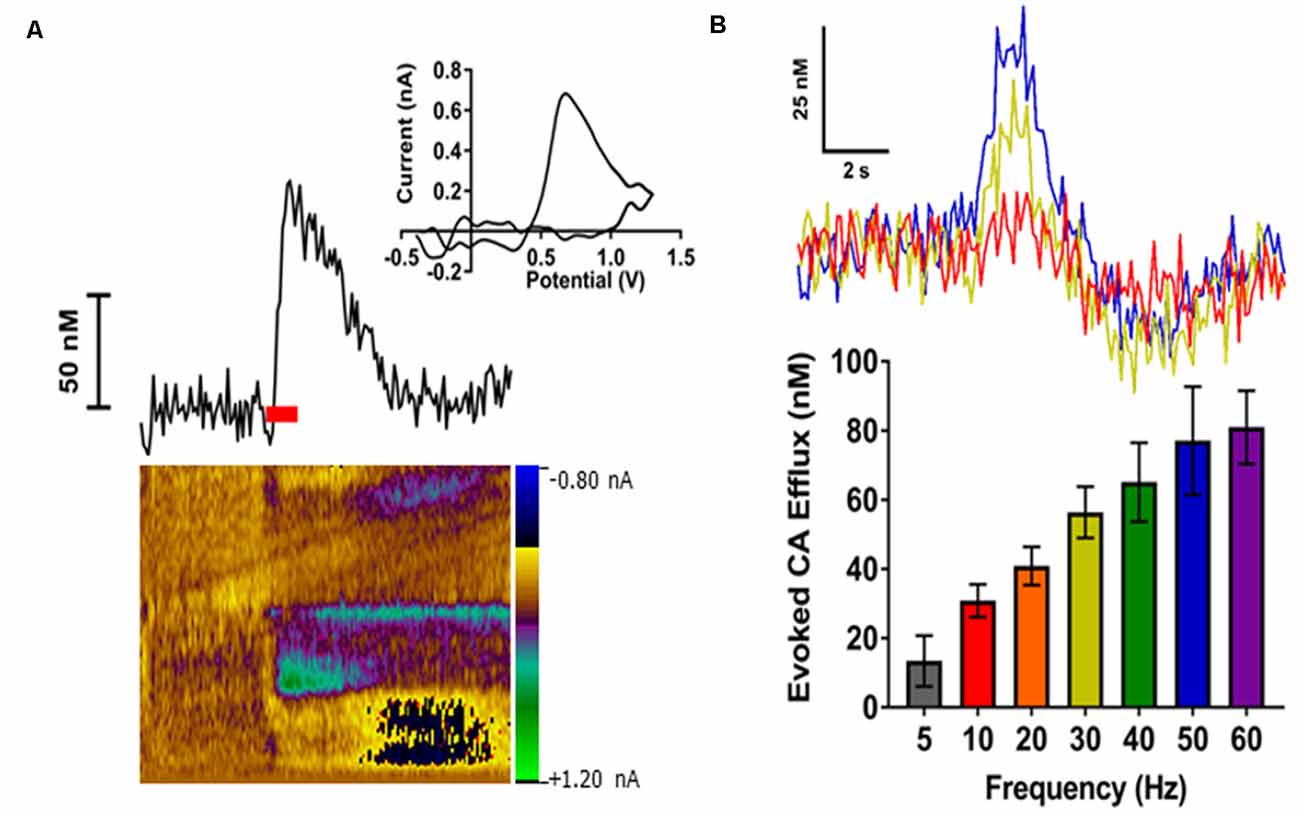
Figure 3. Electrical stimulation of the locus coeruleus (LC) reliably evoked catecholamines (CA) release in the BLA in a frequency-dependent manner. (A) A representative trace showing changes in CA concentration over time (top left). The respective cyclic voltammogram (top right) and color plot (bottom) are also shown. The red bar indicates duration of electrical stimulus. (B) Representative concentration-time traces of CA release (top) in the BLA of an individual rat demonstrate an increased response as stimulation frequency increases (Red = 10 Hz; Yellow = 30 Hz; Blue = 50 Hz). Averaged CA responses (bottom) show higher frequency stimulations resulted in increased efflux concentrations compared to lower frequency stimulations. Data are presented as mean ± SEM.
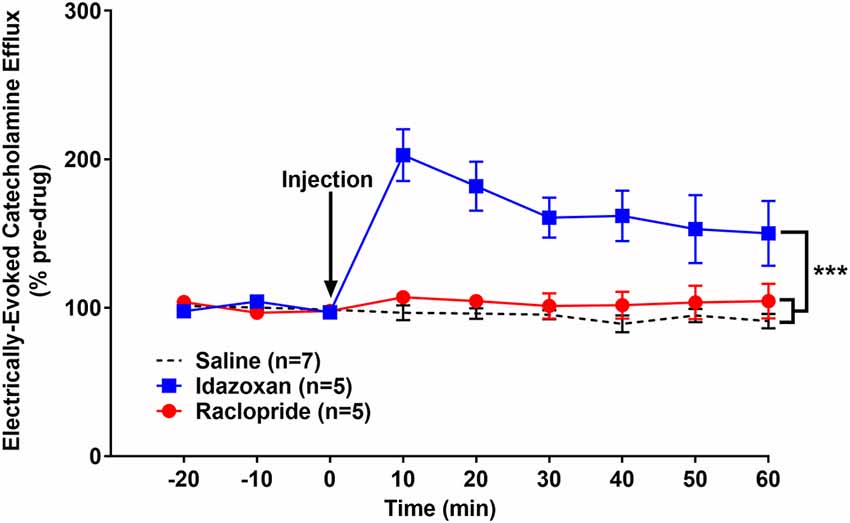
Figure 4. Idazoxan significantly increased norepinephrine (NE) in the basolateral amygdala (BLA) following locus coeruleus (LC) electrical stimulation in non-stressed rats. Anesthetized rats were used to quantify LC-evoked CA release in the BLA, as measured by fast-scan cyclic voltammetry. After a stable baseline was established, saline, idazoxan, or raclopride was administered (black arrow). Idazoxan resulted in a significant increase in the measured signal compared to the saline control. There was no change in the CA signal following injection of raclopride compared to the saline control. Efflux values are presented as mean (± SEM) percent of a pre-injection baseline average. Saline: n = 7; Idazoxan: n = 5; Raclopride: n = 5. ***p < 0.001.
Electrical LC Stimulation in Socially Stressed Subjects Elicits NE and DA Release in BLA
To determine the effect of stress on evoked CA transients in the BLA, intruder rats were used for in vivo FSCV for 1 week following the last 5 days of repeated social defeat stress. In a separate group of rats, we verified that the social defeat procedure resulted in stress by exposing RSDS rats to a forced swim test. A one-tailed unpaired t-test found a significantly higher average number of bouts of immobility exhibited by rats exposed to RSDS (11.1 ± 1.65; n = 5) compared to non-stressed controls (7.21 ± 1.32; n = 7; t(10) = 1.854; p < 0.05) suggesting a stress-like phenotype.
Similar to the naïve group, idazoxan (n = 5) and raclopride (n = 5) were administered to determine the catecholamines (CA) present in the detected signal in rats exposed to RSDS and compared to a saline control (n = 7; Figure 5). A repeated measure two-way ANOVA found the main effect of drug (F(2, 14) = 22.77; p < 0.0001), time (F(8, 112) = 20.02; p < 0.0001), and a significant interaction (F(16, 112) = 9.855; p < 0.0001). A post hoc Tukey’s multiple comparisons test showed that injection of either idazoxan or raclopride significantly increased the evoked CA signal compared to saline (p < 0.0001 and p = 0.0164, respectively) and idazoxan increased the signal significantly more than raclopride (p = 0.0145). These data show that electrical stimulation of the LC evokes both NE and DA release in the BLA of subjects exposed to repeated social defeat stress.
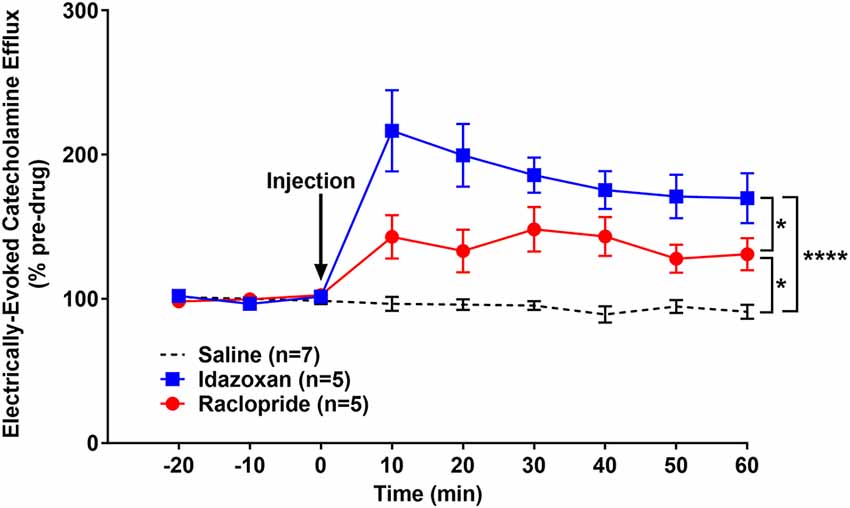
Figure 5. Idazoxan and raclopride significantly increased norepinephrine and dopamine (DA), respectively, in the basolateral amygdala (BLA) following locus coeruleus (LC) electrical stimulation in rats exposed to repeated social defeat stress (RSDS). Rats exposed to 5 days of social defeat stress were anesthetized and used to quantify LC-evoked CA release in the BLA, as measured by fast-scan cyclic voltammetry. After a stable baseline was established, saline, idazoxan, or raclopride was administered (black arrow). Idazoxan and raclopride resulted in a significant increase in the measured signal compared to the saline control. Efflux values are presented as mean (± SEM) percent of a pre-injection baseline average. Saline: n = 7; Idazoxan: n = 5; Raclopride: n = 5. *p < 0.05; ****p < 0.0001.
Exposure to Forced Swim Stress Results in NE and DA Release in the BLA Following LC Stimulation
In order to determine if this effect of social stress on CA release was unique or generalizable to other stressors, a separate cohort of naïve rats (not exposed to RSDS) was exposed to FST and then used for in vivo FSCV (Figure 6). A repeated measures two-way ANOVA calculated the main effect of drug (F(2, 13) = 10.3; p < 0.01), time (F(8, 104) = 21.26; p < 0.0001), and a significant interaction (F(16, 104) = 7.489; p < 0.0001). A post hoc Tukey’s multiple comparisons test found that both idazoxan (n = 5) and raclopride (n = 5) increased the measured CA release compared to saline controls (n = 6; p = 0.0458 and p < 0.01, respectively). Taken together these experiments suggest that stress, either social and non-social, results in the release of DA, in addition to NE, in the BLA following LC electrical stimulation.
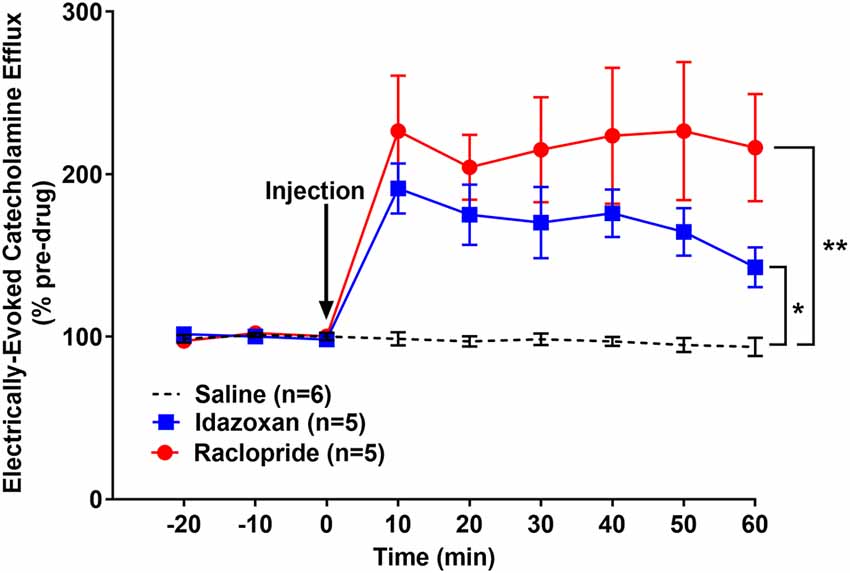
Figure 6. Idazoxan and raclopride significantly increased norepinephrine and dopamine, respectively, in the basolateral amygdala (BLA) following locus coeruleus (LC) electrical stimulation in rats exposed to a forced swim test (FST). Rats exposed to a forced swim test were anesthetized and used to quantify LC-evoked CA release in the BLA, as measured by fast-scan cyclic voltammetry. After a stable baseline was established, saline, idazoxan, or raclopride was administered (black arrow). Idazoxan and raclopride resulted in a significant increase in the measured signal compared to the saline control. Efflux values are presented as mean (± SEM) percent of a pre-injection baseline average. Saline: n = 6; Idazoxan: n = 5; Raclopride: n = 5. *p < 0.05; **p < 0.01.
Interestingly, when compared across stress paradigms, raclopride administration resulted in a significantly higher efflux of dopamine following FST compared to either RSDS or non-stressed, naïve controls (n = 5 for all groups; two-way RM ANOVA: drug, F(3, 18) = 12.26, p < 0.0001; time, F(8, 144) = 14.36, p < 0.0001; interaction, F(24, 144) = 7.814, p < 0.0001; Tukey’s multiple comparisons test: p < 0.05). In a similar comparison, injection of idazoxan did not result in a significant difference between the stress and control groups (n = 5 for all groups; two-way RM ANOVA: drug, F(3, 18) = 13.32, p < 0.0001; time, F(8, 144) = 41.71, p < 0.0001; interaction, F(24, 144) = 6.848, p < 0.0001; Tukey’s multiple comparisons test: p > 0.05) This suggests that there are possible stressor-specific effects on dopamine release which should be considered when using these different paradigms.
Stress Exposure Does Not Alter LC-Evoked NE in the BLA
Additional analysis of the signal prior to injection of saline or drug was conducted to determine if stress exposure altered NE release. A one-way ANOVA found that there was no difference in NE release between the non-stressed (n = 5; 251.2 ± 62.6), RSDS (n = 8; 214.7 ± 30.2), and FST subjects (n = 6; 184.9 ± 29.6; F(2, 16) = 0.617; p = 0.552). However, though not significant, some tendency towards reduced electrically-evoked NE release following forced swim test exposure was evident. A comparison of DA release was not possible because raclopride did not significantly alter the CA signal in non-stressed subjects thus suggesting DA was not present (Figure 4).
Alcohol Decreases the Evoked CA Signal in the BLA by LC Stimulation in Stressed Subjects
In order to explore whether stress is capable of altering the effect of alcohol on CA dynamics, ethanol (2 g/kg, i.p.) was acutely administered to anesthetized subjects previously exposed to RSDS (n = 5) or FST (n = 4; Figure 7). A repeated measures, two-way ANOVA was conducted and found a main effect of condition (F(3, 16) = 8.408; p < 0.001), time (F(8, 128) = 11.79; p < 0.0001), and a significant interaction (F(24, 128) = 4.026; p < 0.0001). A post hoc Tukey’s multiple comparisons test found that alcohol attenuated CA release in both RSDS and FST groups compared to saline (p < 0.01 and p = 0.0309, respectively) and non-stressed controls (p < 0.01 and p = 0.0262, respectively). In addition, there was no significant change following alcohol injection in control animals compared to saline (p > 0.05). These data show that alcohol decreases LC-evoked BLA CA release in subjects exposed to stress but does not have an effect in non-stressed controls.
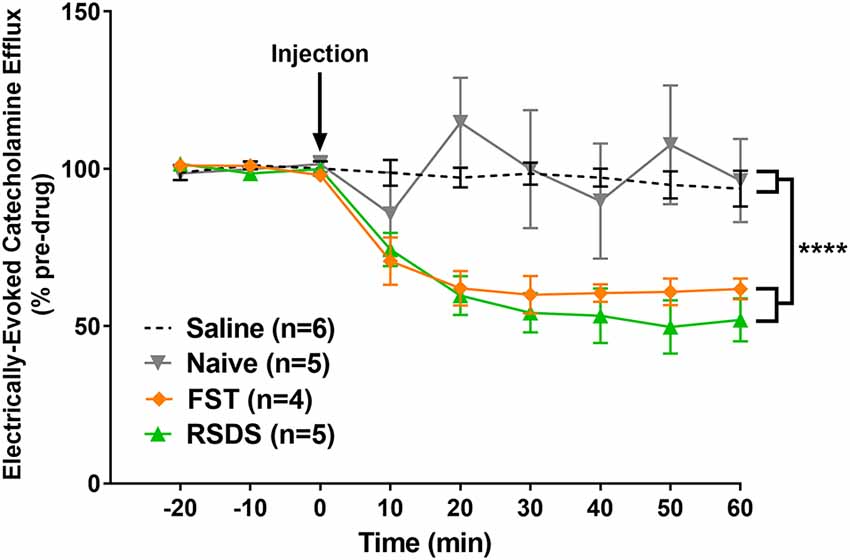
Figure 7. Alcohol significantly reduced the measured catecholamine (CA) efflux in the basolateral amygdala (BLA) following locus coeruleus (LC) electrical stimulation of stressed subjects. Rats were exposed to either repeated social defeat stress or the forced swim test prior to being used to quantify LC-evoked CA release in the BLA as measured by fast-scan cyclic voltammetry. After a stable baseline was established, alcohol was injected (black arrow). In stressed subjects, those exposed to either repeated social defeat stress (RSDS) or forced swim test (FST), alcohol resulted in a significant decrease in the measured CA signal compared to non-stressed, naïve controls. Efflux values are presented as mean (± SEM) percent of a pre-injection baseline average. Saline: n = 6; Naïve: n = 5; FST: n = 4; RSDS: n = 5. ****p < 0.0001.
Discussion
These experiments demonstrate robust stress-dependent alterations in LC-evoked CA release in the BLA following LC stimulation. In control subjects, LC electrical stimulation elicits a strong, predominantly noradrenergic signal in the BLA whose efflux is not altered by acute alcohol. However, in animals that have been exposed to stress, through either social defeat or forced swim, electrical stimulation of the LC results in a CA efflux that is mediated by both noradrenergic as well as dopaminergic components and this response is attenuated following administration of alcohol. Taken together, these findings suggest a role for stress in altering catecholamine dynamics in the BLA and a change in the sensitivity of evoked CA to alcohol.
Although NE release evoked by LC stimulation was previously detected with in vivo FSCV in the prefrontal cortex and bed nucleus of the stria terminals (Park et al., 2011, 2012, 2013; Fox et al., 2016; Deal et al., 2019), our results for the first time demonstrate the possibility to measure real-time NE efflux in the BLA. Based on pharmacological characterizations, we ruled out the involvement of DA efflux in the signal measured in naïve rats as previously reported for the prefrontal cortex and bed nucleus of the stria terminals (Park et al., 2011; Fox et al., 2016; Deal et al., 2019). As expected, the electrically-evoked NE release was frequency-dependent, while the average maximum concentrations were ~2–3 fold lower than concentrations in the prefrontal cortex (Deal et al., 2019). An intriguing finding was the raclopride-induced increase in the detected CA signal in subjects following exposure to both stress paradigms. These data clearly indicate the appearance of a DA component that was not detected in control animals. The origins of the evoked DA signal are unclear and therefore should be further explored. However, some speculations can be offered.
A major dopaminergic source in the brain is the ventral tegmental area (VTA), which has connections with the LC and BLA (Shelkar et al., 2017; Breton et al., 2019). Indeed, social defeat stress has been shown to increase phasic DA release in the nucleus accumbens (NA), a region with strong dopaminergic input from the VTA (Anstrom et al., 2009). Further, chronic stress exposure can lead to morphological changes in VTA DA neurons and enhanced excitability of this neuronal population (Douma and de Kloet, 2020). Perhaps a stress-promoted increase in the excitability of VTA dopaminergic neurons could result in the triggering of DA release in the BLA in response to LC stimulation. Therefore, one hypothesis to address the current findings is that the stress-triggered DA portion of the evoked CA signal reflects a persistent increase in the excitability of VTA projections.
Stress has also been shown to alter the electrophysiological characteristics of LC neurons (Jedema and Grace, 2003; Borodovitsyna et al., 2018). Previous studies have reported that exposure of adolescent rats to restraint and predator odor stressors resulted in increased excitability and spontaneous discharge of LC neurons, which were evident 1 week after stress exposure (Borodovitsyna et al., 2018). Thus, it is possible that in the present study, forced swim or repeated social defeat stress induced a similar increase in LC neuron excitability, altering the activation dynamics of LC inputs to VTA DA neurons, resulting in amygdalar DA release. Therefore, another possible origin for the DA component in the CA signal of stressed subjects is from changes to the NE neurons of the LC that innervate the VTA. In fact, a third possibility can combine both mentioned above scenarios.
Finally, multiple studies have found that various stressors result in an increased expression of tyrosine hydroxylase (TH) mRNA in LC neurons (Mamalaki et al., 1992; Rusnák et al., 2001). While these studies did not confirm the lasting effects of stress on TH mRNA, such an increase in this rate-limiting enzyme of DA synthesis could result in elevated levels of DA in LC NE neurons. Supporting this possibility, another study found that chronic social defeat stress resulted in an upregulation of dopamine β-hydroxylase (DBH) mRNA and protein in the LC as well as increased DBH protein levels in terminal regions of the LC, such as the amygdala, hippocampus, and frontal cortex (Fan et al., 2013). The increase in the production of this ezyme that converts DA to NE can be induced by accumulation of DA inside of NE neurons. Consequently, LC stimulation of stressed rats could result in the efflux of both DA and NE from noradrenergic projections to the BLA due to elevated levels of DA production within the LC NE neurons. Though not induced by stress, there is evidence to support the release of DA from noradrenaline neurons in some brain areas, including prefrontal cortex and hippocampus (Devoto et al., 2003, 2019; Kempadoo et al., 2016).
The finding that consequences of social and non-social stress on CA dynamics are not different is somewhat surprising. However, it is important to highlight that our experiments focused on evoked NE and DA releases which are not necessarily correlated with basal activity of neurotransmitters. Therefore, this question requires further exploration with complimentary techniques which can evalutate changes in other modes of CA neurotransmission.
Another stress-induced change in the CA signal was observed following the administration of alcohol. In non-stressed animals, alcohol did not significantly alter the measured CA efflux. However, following stress exposure, alcohol attenuated the evoked CA signal in the BLA. Unfortunately, due to the presence of both NE and DA in the CA signal, as evidenced by idazoxan and raclopride increasing the measured efflux, it is not currently possible to disentangle the effects of alcohol on NE or DA in the stressed subjects. However, it is notable that when only NE was evoked in the BLA following LC stimulation, alcohol did not affect the measured signal. These findings may suggest that CA efflux decreased after stress because alcohol only influenced the DA portion. In fact, divergent effects of alcohol on NE and DA transmission under normal conditions have been observed in some brain areas. For example, it was found using FSCV that LC-induced NE in the mPFC is not affected by acute alcohol, while VTA-induced DA in the same brain region is decreased by alcohol (Shnitko et al., 2014; Deal et al., 2019). Moreover, a microdialysis study revealed that alcohol (2 g/kg) increased extracellular DA but not NE in the BLA of group housed rats (Karkhanis et al., 2015). As discussed previously (Jones et al., 2006), an increase in DA levels, as measured by microdialysis, would be consistent with a decrease in the amount of electrically-evoked DA detected with FSCV in vivo. This may be simply due to autoreceptor feedback induced by increased cell firing rates and accumulating DA concentrations in terminals. Further, the effect of acute alcohol on extracellular DA concentrations can be sensitized in the BLA following stress through adolescent social isolation (Karkhanis et al., 2015). Therefore, there are multiple studies which provide indirect evidence to support the involvment of DA in the effect of alcohol on evoked CA release in the BLA.
However, we cannot exclude the role of NE in this effect. Indeed, while extracellular NE concentrations were unchanged by alcohol under normal conditions, social isolation stress resulted in a significant increase in this measure (Karkhanis et al., 2015). Strikingly, alcohol-induced increases in both NE and DA levels measured by microdialysis exhibited a similar time course to the decrease in electrically-evoked CA release mesured by voltammetry. Taken together, the current and previous findings support the hypothesis that alcohol may be differently targeting DA and NE release in the BLA following stress exposure.
In conclusion, we have shown that stress, whether social or non-social, can lead to lasting alterations in CA activity that, in turn, affect how alcohol influences CA release in the BLA. Stress changes the LC-induced CA landscape of the BLA, introducing a locus of effect for alcohol that is not present in non-stressed subjects.
Data Availability Statement
The raw data supporting the conclusions of this article will be made available by the authors, without undue reservation.
Ethics Statement
The animal study was reviewed and approved by Wake Forest University School of Medicine Institutional Animal Care and Use Committee.
Author Contributions
EB and JW designed the electrochemical and behavioral experiments. AD performed the electrochemical and behavioral tests and analyzed the data. JP conducted the histology. All authors contributed to the article and approved the submitted version.
Funding
The work presented in this manuscript was supported by the National Institutes of Health (National Institute on Alcohol Abuse and Alcoholism, NIH) grants T32AA007565 (AD), DA045284 (JP), AA17531 (JW), AA26551 (JW), P50 AA026117 (EB and JW), and AA022449 (EB), as well as the Tab Williams Family Endowment Fund (EB).
Conflict of Interest
The authors declare that the research was conducted in the absence of any commercial or financial relationships that could be construed as a potential conflict of interest.
Acknowledgments
We would like to thank Collin J. McKinney, Director of the Electronics Design Facility at University of North Carolina at Chapel Hill, for providing excellent technical support with voltammetry equipment. We would also like to thank Valentina Grinevich for her help with FSCV data collection.
References
Anstrom, K. K., Miczek, K. A., and Budygin, E. A. (2009). Increased phasic dopamine signaling in the mesolimbic pathway during social defeat in rats. Neuroscience 161, 3–12. doi: 10.1016/j.neuroscience.2009.03.023
Arora, V., Martin, T. J., Aschenbrenner, C. A., Hayashida, K., Kim, S. A., Parker, R. A., et al. (2019). Psychosocial stress delays recovery of postoperative pain following incisional surgery in the rat. Neuroscience 382, 35–47. doi: 10.1016/j.neuroscience.2018.04.014
Báez, M., and Volosin, M. (1994). Corticosterone influences forced swim-induced immobility. Pharmacol. Biochem. Be. 49, 729–736. doi: 10.1016/0091-3057(94)90093-0
Barnum, C. J., Blandino, P. Jr., and Deak, T. (2007). Adaptation in the corticosterone and hyperthermic responses to stress following repeated stressor exposure. J. Neuroendocrinol. 19, 632–642. doi: 10.1111/j.1365-2826.2007.01571.x
Bogdanova, O. V., Kanekar, S., D’Anci, K. E., and Renshaw, P. F. (2013). Factors influencing behavior in the forced swim test. Physiol. Behav. 118, 227–239. doi: 10.1016/j.physbeh.2013.05.012
Borodovitsyna, O., Flamini, M. D., and Chandler, D. J. (2018). Acute stress persistently alters locus coeruleus function and anxiety-like behavior in adolescent rats. Neuroscience 373, 7–19. doi: 10.1016/j.neuroscience.2018.01.020
Breton, J. M., Charbit, A. R., Snyder, B. J., Fong, P. T. K., Dias, E. V., Himmels, P., et al. (2019). Relative contributions and mapping of ventral tegmental area dopamine and GABA neurons by projection target in the rat. J. Comp. Neurol. 527, 916–941. doi: 10.1002/cne.24572
Budygin, E. A., Kilpatrick, M. R., Gainetdinov, R. R., and Wightman, R. M. (2000). Correlation between behavior and extracellular dopamine levels in rat striatum: comparison of microdialysis and fast-scan cyclic voltammetry. Neurosci. Lett. 281, 9–12. doi: 10.1016/s0304-3940(00)00813-2
Budygin, E. A., Oleson, E. B., Lee, Y. B., Blume, L. C., Bruno, M. J., Howlett, A. C., et al. (2017). Acute depletion of D2 receptors from the rat substantia nigra alters dopamine kinetics in the dorsal striatum and drug responsivity. Front. Behav. Neurosci. 10:248. doi: 10.3389/fnbeh.2016.00248
Budygin, E. A., Oleson, E. B., Mathews, T. A., Läck, A. K., Diaz, M. R., McCool, B. A., et al. (2007). Effects of chronic alcohol exposure on dopamine uptake in rat nucleus accumbens and caudate putamen. Psychopharmacology 193, 495–501. doi: 10.1007/s00213-007-0812-1
Budygin, E. A., Park, J., Bass, C. E., Grinevich, V. P., Bonin, K. D., and Wightman, R. M. (2012). Aversive stimulus differentially triggers subsecond dopamine release in reward regions. Neuroscience 201, 331–337. doi: 10.1016/j.neuroscience.2011.10.056
Covington, H. E. III., and Miczek, K. A. (2005). Intense cocaine self-administration after episodic social defeat stress, but not after aggressive behavior: dissociation from corticosterone activation. Psychopharmacology 183, 331–340. doi: 10.1007/s00213-005-0190-5
Daviu, N., Bruchas, M. R., Moghaddam, B., Sandi, C., and Beyeler, A. (2019). Neurobiological links between stress and anxiety. Neurobiol. Stress 11:100191. doi: 10.1016/j.ynstr.2019.100191
Deal, A. L., Bass, C. E., Grinevich, V. P., Delbono, O., Bonin, K. D., Weiner, J. L., et al. (2020). Bidirectional control of alcohol-drinking behaviors through locus coeruleus optoactivation. Neuroscience 443, 84–92. doi: 10.1016/j.neuroscience.2020.07.024
Deal, A. L., Konstantopoulos, J. K., Weiner, J. L., and Budygin, E. A. (2018). Exploring the consequences of social defeat stress and intermittent ethanol drinking on dopamine dynamics in the rat nucleus accumbens. Sci. Rep. 8:332. doi: 10.1038/s41598-017-18706-y
Deal, A. L., Mikhailova, M. A., Grinevich, V. P., Weiner, J. L., Gainetdinov, R. R., and Budygin, E. A. (2019). In vivo voltammetric evidence that locus coeruleus activation predominantly releases norepinephrine in the infralimbic cortex: effect of acute ethanol. Synapse 73:e22080. doi: 10.1002/syn.22080
Deal, A. W., Seshie, O., Lenzo, A., Cooper, N., Ozimek, N., and Solberg Woods, L. C. (2020). High-fat diet negatively impacts both metabolic and behavioral health in outbred heterogeneous stock rats. Physiol. Genomics 52, 379–390. doi: 10.1152/physiolgenomics.00018.2020
Devoto, P., Flore, G., Longu, G., Pira, L., and Gessa, G. L. (2003). Origin of extracellular dopamine from dopamine and noradrenaline neurons in the medial prefrontal and occipital cortex. Synapse 50, 200–205. doi: 10.1002/syn.10264
Devoto, P., Flore, G., Saba, P., Scheggi, S., Mulas, G., Gambarana, C., et al. (2019). Noradrenergic terminals are the primary source of α2-adrenoceptor mediated dopamine release in the medial prefrontal cortex. Prog. Neuropsychopharmacol. Biol. Psychiatry 90, 97–103. doi: 10.1016/j.pnpbp.2018.11.015
Douma, E. H., and de Kloet, E. R. (2020). Stress-induced plasticity and functioning of ventral tegmental dopamine neurons. Neurosci. Biobehav. Rev. 108, 48–77. doi: 10.1016/j.neubiorev.2019.10.015
Fan, Y., Chen, P., Li, Y., and Zhu, M.-Y. (2013). Effects of chronic social defeat on expression of dopamine β-hydroxylase in rat brains. Synapse 67, 300–312. doi: 10.1002/syn.21641
Ferry, B., Roozendaal, B., and McGaugh, J. L. (1999). Role of norepinephrine in mediating stress hormone regulation of long-term memory storage: a critical involvement of the amygdala. Biol. Psychiatry 46, 1140–1152. doi: 10.1016/s0006-3223(99)00157-2
Fox, M. E., Bucher, E. S., Johnson, J. A., and Wightman, R. M. (2016). Medullary norepinephrine projections release norepinephrine into the contralateral bed nucleus of the stria terminalis. ACS Chem. Neurosci. 7, 1681–1689. doi: 10.1021/acschemneuro.6b00210
Fox, M. E., Studebaker, R. I., Swofford, N. J., and Wightman, R. M. (2015). Stress and drug dependence differentially modulate norepinephrine signaling in animals with varied HPA axis function. Neuropsychopharmacology 40, 1752–1761. doi: 10.1038/npp.2015.23
Giustino, T. F., Ramanathan, K. R., Totty, M. S., Miles, O. W., and Maren, S. (2020). Locus coeruleus norepinephrine drives stress-induced increases in basolateral amygdala firing and impairs extinction learning. J. Neurosci. 40, 907–916. doi: 10.1523/JNEUROSCI.1092-19.2019
Grant, B. F., Stinson, F. S., Dawson, D. A., Chou, S. P., Dufour, M. C., Compton, W., et al. (2004). Prevalence and co-occurrence of substance use disorders and independentmood and anxiety disorders: results from the national epidemiologic survey on alcohol and related conditions. Arch. Gen. Psychiatry 61, 807–816. doi: 10.1001/archpsyc.61.8.807
Henry, J. P., Liu, Y.-Y., Nadra, W. E., Qian, C.-G., Mormede, P., Lemaire, V., et al. (1993). Psychosocial stress can induce chronic hypertension in normotensive strains of rats. Hypertension 21, 714–723. doi: 10.1161/01.hyp.21.5.714
Holly, E. N., and Miczek, K. A. (2016). Ventral tegmental area dopamine revisited: effects of acute and repeated stress. Psychopharmacology 233, 163–186. doi: 10.1007/s00213-015-4151-3
Hueston, C. M., Barnum, C. J., Eberle, J. A., Ferraioli, F. J., Buck, H. M., and Deak, T. (2011). Stress-dependent changes in neuroinflammatory markers observed after common laboratory stressors are not seen following acute social defeat of the Sprague–Dawley rat. Physiol. Behav. 104, 187–198. doi: 10.1016/j.physbeh.2011.03.013
Inglis, F. M., and Moghaddam, B. (1999). Dopaminergic innervation of the amygdala is highly responsive to stress. J. Neurochem. 72, 1088–1094. doi: 10.1046/j.1471-4159.1999.0721088.x
Jedema, H. P., and Grace, A. A. (2003). Chronic exposure to cold stress alters electrophysiological properties of locus coeruleus neurons recorded in vitro. Neuropsychopharmacology 28, 63–72. doi: 10.1038/sj.npp.1300020
Jones, S. R., Mathews, T. A., and Budygin, E. A. (2006). Effect of moderate ethanol dose on dopamine uptake in rat nucleus accumbens in vivo. Synapse 60, 251–255. doi: 10.1002/syn.20294
Karkhanis, A. N., Alexander, N. J., McCool, B. A., Weiner, J. L., and Jones, S. R. (2015). Chronic social isolation during adolescence augments catecholamine response to acute ethanol in the basolateral amygdala. Synapse 69, 385–395. doi: 10.1002/syn.21826
Kempadoo, K. A., Mosharov, E. V., Choi, S. J., Sulzer, D., and Kandel, E. R. (2016). Dopamine release from the locus coeruleus to the dorsal hippocampus promotes spatial learning and memory. Proc. Natl. Acad. Sci. U S A 113, 14835–14840. doi: 10.1073/pnas.1616515114
Kessler, R. C., Crum, R. M., Warner, L. A., Nelson, C. B., Schulenberg, J., and Anthony, J. C. (1997). Lifetime co-occurrence of DSM-III-R alcohol abuse and dependence with other psychiatric disorders in the National Comorbidity Survey. Arch. Gen. Psychiatry 54, 313–321. doi: 10.1001/archpsyc.1997.01830160031005
Linthorst, A. C. E., Flachskamm, C., and Reul, J. M. H. M. (2008). Water temperature determines neurochemical and behavioral responses to forced swim stress: an in vivo microdialysis and biotelemetry study in rats. Stress 11, 88–100. doi: 10.1080/10253890701533231
Mamalaki, E., Kvetnansky, R., Brady, L. S., Gold, P. W., and Herkenham, M. (1992). Repeated immobilization stress alters tyrosine hydroxylase, corticotropin-releasing hormone and corticosteroid receptor messenger ribonucleic acid levels in rat brain. J. Neuroendocrinol. 4, 689–699. doi: 10.1111/j.1365-2826.1992.tb00220.x
Mateo, Y., Budygin, E. A., Morgan, D., Roberts, D. C., and Jones, S. R. (2004). Fast onset of dopamine uptake inhibition by intravenous cocaine. Eur. J. Neurosci. 20, 2838–2842. doi: 10.1111/j.1460-9568.2004.03736.x
McCall, J. G., Siuda, E. R., Bhatti, D. L., Lawson, L. A., McElligott, Z. A., Stuber, G. D., et al. (2017). Locus coeruleus to basolateral amygdala noradrenergic projections promote anxiety-like behavior. eLife 6:e18247. doi: 10.7554/eLife.18247
Miczek, K. A., Nikulina, E., Kream, R. M., Carter, G., and Espejo, E. F. (1999). Behavioral sensitization to cocaine after a brief social defeat stress: c-fos expression in the PAG. Psychopharmacology 141, 225–234. doi: 10.1007/s002130050829
Mikhailova, M. A., Deal, A. L., Grinevich, V. P., Bonin, K. D., Gainetdinov, R. R., and Budygin, E. A. (2019). Real-time accumbal dopamine response to negative stimuli: effects of ethanol. ACS Chem. Neurosci. 10, 1986–1991. doi: 10.1021/acschemneuro.8b00272
Moravcova, S., Cervena, K., Mikova, H., Pacesova, D., Pallag, G., Novotny, J., et al. (2021). Social defeat stress affects resident’s clock gene and BDNF expression in the brain. Stress 24, 206–212. doi: 10.1080/10253890.2020.1759548
Naegeli, C., Zeffiro, T., Piccirelli, M., Jaillard, A., Weilenmann, A., Hassanpour, K., et al. (2018). Locus coeruleus activity mediates hyperresponsiveness in posttraumatic stress disorder. Biol. Psychiatry 83, 254–262. doi: 10.1016/j.biopsych.2017.08.021
Oleson, E. B., Talluri, S., Childers, S. R., Smith, J. E., Roberts, D. C., Bonin, K. D., et al. (2009). Dopamine uptake changes associated with cocaine self-administration. Neuropsychopharmacol. 34, 1174–1184. doi: 10.1038/npp.2008.186
Park, J., Aragona, B. J., Kile, B. M., Carelli, R. M., and Wightman, R. M. (2010). In vivo voltammetric monitoring of catecholamine release in subterritories of the nucleus accumbens shell. Neuroscience 169, 132–142. doi: 10.1016/j.neuroscience.2010.04.076
Park, J., Bucher, E. S., Budygin, E. A., and Wightman, R. M. (2015). Norepinephrine and dopamine transmission in 2 limbic regions differentially respond to acute noxious stimulation. Pain 156, 318–327. doi: 10.1097/01.j.pain.0000460312.79195.ed
Park, J., Bucher, E. S., Fontillas, K., Owesson-White, C., Ariansen, J. L., Carelli, R. M., et al. (2013). Opposing catecholamine changes in the bed nucleus of the stria terminalis during intracranial self-stimulation and its extinction. Biol. Psychiatry 74, 69–76. doi: 10.1016/j.biopsych.2012.11.008
Park, J., Takmakov, P., and Wightman, R. M. (2011). In vivo comparison of norepinephrine and dopamine release in rat brain by simultaneous measurements with fast-scan cyclic voltammetry. J. Neurochem. 119, 932–944. doi: 10.1111/j.1471-4159.2011.07494.x
Park, J., Wheeler, R. A., Fontillas, K., Keithley, R. B., Carelli, R. M., and Wightman, R. M. (2012). Catecholamines in the bed nucleus of the stria terminalis reciprocally respond to reward and aversion. Biol. Psychiatry 71, 327–334. doi: 10.1016/j.biopsych.2011.10.017
Paxinos, G., and Watson, C. (2004). The Rat Brain in Stereotaxic Coordinates, 5th edition. New York, NY: Academic Press.
Porsolt, R. D., Anton, G., Blavet, N., and Jalfre, M. (1978). Behavioral despair in rats: a new model sensitive to antidepressant treatments. Eur. J. Pharmacol. 47, 379–391. doi: 10.1016/0014-2999(78)90118-8
Rajbhandari, A. K., Baldo, B. A., and Bakshi, V. P. (2015). Predator stress-induced CRF release causes enduring sensitization of basolateral amygdala norepinephrine systems that promote PTSD-like startle abnormalities. J. Neurosci. 35, 14270–14285. doi: 10.1523/JNEUROSCI.5080-14.2015
Rusnák, M., Kvetňanský, R., Jeloková, J., and Palkovits, M. (2001). Effect of novel stressors on gene expression of tyrosine hydroxylase and monoamine transporters in brainstem noradrenergic neurons of long-term repeatedly immobilized rats. Brain Res. 899, 20–35. doi: 10.1136/ijgc-2020-001698
Samuels, E. R., and Szabadi, E. (2008). Functional neuroanatomy of the noradrenergic locus coeruleus: its roles in the regulation of arousal and autonomic function part I: principles of functional organisation. Curr. Neuropharmacol. 6, 235–253. doi: 10.2174/157015908785777229
Shelkar, G. P., Kumar, S., Singru, P. S., Subhedar, N. K., and Kokare, D. M. (2017). Noradrenergic inputs from locus coeruleus to posterior ventral tegmental area are essential to support ethanol reinforcement. Addict. Biol. 22, 291–302. doi: 10.1111/adb.12321
Shnitko, T. A., Kennerly, L. C., Spear, L. P., and Robinson, D. L. (2014). Ethanol reduces evoked dopamine release and slows clearance in the rat medial prefrontal cortex. Alcohol. Clin. Exp. Res. 38, 2969–2977. doi: 10.1111/acer.12587
Snyder, B., Duong, P., Tenkorang, M., Wilson, E. N., and Cunningham, R. L. (2018). Rat strain and housing conditions alter oxidative stress and hormone responses to chronic intermittent hypoxia. Front. Physiol. 9:1554. doi: 10.3389/fphys.2018.01554
Szabadi, E. (2013). Functional neuroanatomy of the central noradrenergic system. J. Psychopharmacol. 27, 659–693. doi: 10.1177/0269881113490326
Tanaka, T., Yokoo, H., Mizoguchi, K., Yoshida, M., Tsuda, A., and Tanaka, M. (1991). Noradrenaline release in the rat amygdala is increased by stress: studies with intracerebral microdialysis. Brain Res. 544, 174–176. doi: 10.1016/0006-8993(91)90902-8
Thomas, S. E., Randall, C. L., and Carrigan, M. H. (2003). Drinking to cope in socially anxious individuals: a controlled study. Alcohol. Clin. Exp. Res. 27, 1937–1943. doi: 10.1097/01.ALC.0000100942.30743.8C
Wood, S. K., Walker, H. E., Valentino, R. J., and Bhatnagar, S. (2010). Individual differences in reactivity to social stress predict susceptibility and resilience to a depressive phenotype: role of corticotropin-releasing factor. Endocrinology 151, 1795–1805. doi: 10.1210/en.2009-1026
Wood, S. K., Zhang, X.-Y., Reyes, B. A. S., Lee, C. S., Van Bockstaele, E. J., and Valentino, R. J. (2013). Cellular adaptations of dorsal raphe serotonin neurons associated with the development of active coping in response to social stress. Biol. Psychiatry 73, 1087–1094. doi: 10.1016/j.biopsych.2013.01.026
Yorgason, J. T., Calipari, E. S., Ferris, M. J., Karkhanis, A. N., Fordahl, S. C., Weiner, J. L., et al. (2016). Social isolation rearing increases dopamine uptake and psychostimulant potency in the striatum. Neuropharmacology 101, 471–479. doi: 10.1016/j.neuropharm.2015.10.025
Keywords: norepinephrine, dopamine, FSCV, fast-scan cyclic voltammetry, locus coeruleus (LC), social defeat stress, forced swim test (FST)
Citation: Deal AL, Park J, Weiner JL and Budygin EA (2021) Stress Alters the Effect of Alcohol on Catecholamine Dynamics in the Basolateral Amygdala. Front. Behav. Neurosci. 15:640651. doi: 10.3389/fnbeh.2021.640651
Received: 11 December 2020; Accepted: 23 March 2021;
Published: 15 April 2021.
Edited by:
Allan V. Kalueff, Saint Petersburg State University, RussiaCopyright © 2021 Deal, Park, Weiner and Budygin. This is an open-access article distributed under the terms of the Creative Commons Attribution License (CC BY). The use, distribution or reproduction in other forums is permitted, provided the original author(s) and the copyright owner(s) are credited and that the original publication in this journal is cited, in accordance with accepted academic practice. No use, distribution or reproduction is permitted which does not comply with these terms.
*Correspondence: Evgeny A. Budygin, ebudygin@wakehealth.edu