- 1Neuroscience Curriculum, Pennsylvania State University, University Park, PA, United States
- 2The Department of Biology, Pennsylvania State University, University Park, PA, United States
Recent advancements in technology have enabled researchers to probe the brain with the greater region, cell, and receptor specificity. These developments have allowed for a more thorough understanding of how regulation of the neurophysiology within a region is essential for maintaining healthy brain function. Stress has been shown to alter the prefrontal cortex (PFC) functioning, and evidence links functional impairments in PFC brain activity with neuropsychiatric disorders. Moreover, a growing body of literature highlights the importance of neuropeptides in the PFC to modulate neural signaling and to influence behavior. The converging evidence outlined in this review indicates that neuropeptides in the PFC are specifically impacted by stress, and are found to be dysregulated in numerous stress-related neuropsychiatric disorders including substance use disorder, major depressive disorder (MDD), posttraumatic stress disorder, and schizophrenia. This review explores how neuropeptides in the PFC function to regulate the neural activity, and how genetic and environmental factors, such as stress, lead to dysregulation in neuropeptide systems, which may ultimately contribute to the pathology of neuropsychiatric diseases.
Introduction
The Prefrontal Cortex in Humans and Rodents: Executive Control Over Neuropsychiatric Disorders
The prefrontal cortex (PFC), located in the anterior portion of the frontal lobe, is responsible for several higher-order behaviors including executive function and response to emotional stimuli (Salzman and Fusi, 2010; Grossmann, 2013). The PFC of humans has been implicated in many stress-related neuropsychiatric disorders, including anxiety (Park and Moghaddam, 2017), major depressive disorder (MDD; Murray et al., 2011), post-traumatic stress disorder (PTSD; Koenigs and Grafman, 2009), and substance use disorders (Goldstein and Volkow, 2011). Importantly, the PFC is one of the brain regions most sensitive to the detrimental effects of stress (Arnsten, 2009; Kolb et al., 2012). Stress has been shown to lead to PFC dysfunction observed in various neuropsychiatric disorders. Moreover, the PFC is known to undergo profound alterations throughout development (Teffer and Semendeferi, 2012), and is one of the last areas of the cortex to develop (Fuster, 2001).
In addition to the extensive human literature, the role of the PFC in behaviors associated with stress and neuropsychiatric disease has been heavily studied using rodent and non-human primate models. Though the role of the PFC in animal models has been heavily debated, recent attempts to standardize the definition and anatomical framework of the PFC have led to increased consistency of research (for review and synthesis see Carlén, 2017; Laubach et al., 2018). The PFC is divided dorsoventrally into various subregions; the human literature often divides the PFC into the lateral PFC (Broadmann areas 9–12 and 25) and the medial PFC (Broadmann Areas 9–12 and 44–46; Grossmann, 2013), whereas the rodent literature often sub-divides the PFC into infralimbic, prelimbic, and anterior cingulate cortex (Laubach et al., 2018). Animal model-based investigations of the PFC are allowing for a greater understanding of prefrontal cortical networks.
The PFC has both complex local circuitry and connections with other brain regions (Kolb et al., 2012). The PFC is heavily connected with other regions such as the brainstem, the thalamus, the basal ganglia, and limbic system (for review and synthesis see Van Eden and Buijs, 2000; Fuster, 2001). Its well-organized reciprocal connections with the mediodorsal nucleus of the thalamus (MD) is used as a criterion for identifying the PFC in a variety of species (Ferguson and Gao, 2015). Connections between the MD and PFC have been linked with cognitive impairment observed in many different neuropsychiatric disorders (for review and synthesis see Ouhaz et al., 2018). Moreover, excitatory afferents to the PFC arise from several other brain regions including limbic areas related to emotion such as the amygdala (Porrino et al., 1981; Lowery-Gionta et al., 2018), hippocampus (Thierry et al., 2000; Dégenètais et al., 2003; Bogart and O’Donnell, 2018), and hypothalamus (Kievit and Kuypers, 1975; Jacobson et al., 1978). Afferent projections from limbic regions carry to the PFC information about internal states and motivational significance and likely play a major role in executive control over emotional behavior (LeDoux, 1993; Fuster, 2001). The PFC also sends glutamatergic projections to multiple brain regions responsible for regulating emotional behaviors (some of them reciprocal) including the amygdala (McGarry and Carter, 2017; Bloodgood et al., 2018), the bed nucleus of the stria terminalis (BNST; Crowley et al., 2016), the striatum (Stuber et al., 2011; Britt et al., 2012; Bloodgood et al., 2018), and the periaqueductal gray (Siciliano et al., 2019).
Peptide Populations Within the Prefrontal Cortex
Neurons within the PFC express a variety of markers and neurotransmitters (Van De Werd et al., 2010). Neuropeptides are strings of amino acids connected by peptide bonds found in the central nervous system (CNS) which play a key role in modulating neural activity (see van den Pol, 2012 for review and synthesis). Unlike classical neurotransmitters (e.g., amino acid neurotransmitters such as GABA and glutamate), neuropeptides are large molecules that are stored in large dense-core vesicles. They are often co-released along with other amino acid neurotransmitters and neuropeptide release is not restricted to the synapse. Neuropeptides diffuse long distances to act on G-protein coupled receptors. Compared to fast-acting amino acid neurotransmitters the response of receptive cells to neuropeptides is slow (often several seconds to minutes), which makes the investigation of neuropeptides complex.
Peptide-expressing gamma-aminobutyric acid (GABA)-ergic neurons (which often co-release neuropeptides) in the PFC have received dense focus throughout the decades due to their strong regulation of both glutamatergic inputs to and outputs from the PFC, as well as their ability to modulate each other. This, combined with the extensive role of the PFC in stress and neuropsychiatric disorders, has led to a keen interest in their function (Northoff and Sibille, 2014; Fogaça and Duman, 2019; McKlveen et al., 2019; Ghosal et al., 2020). These neuronal populations release both GABA and their respective neuropeptides, allowing for complex regulatory control over PFC circuits though the precise dynamics of peptide transmission vs. GABA transmission are still being elucidated throughout the brain. Also, some GABA/peptidergic neurons are thought to express multiple peptides, and it is unclear under what conditions these individual (and sometimes functionally opposing) peptides are released. Despite these challenges in studying neuropeptides, recent technological advances have made it easier to investigate peptidergic transmission in various brain regions (Al-Hasani et al., 2015, 2018; Crowley et al., 2016), including detecting neuropeptide release within the PFC (Dao et al., 2019).
Recent research outlined in this review sheds light on the role of diverse neuropeptides in the PFC in regulating cortical networks and controlling emotional behaviors. The current review focuses on some of the major neuropeptide populations within the PFC—notably neuropeptide Y (NPY), corticotrophin-releasing factor (CRF), somatostatin (SST), dynorphin opioids (DYN), and the endorphin/enkephalin opioid systems. Where possible, each section will explore the peptide expression and known effects, the effects of known receptors, and the role the peptide and receptors play in a variety of neuropsychiatric diseases. Importantly, this review attempts to bridge clinical studies of psychiatric populations with preclinical research investigating the neural circuit actions of PFC neuropeptides and how dysregulation of these systems contributes to specific behaviors associated with diseased states (Figure 1).
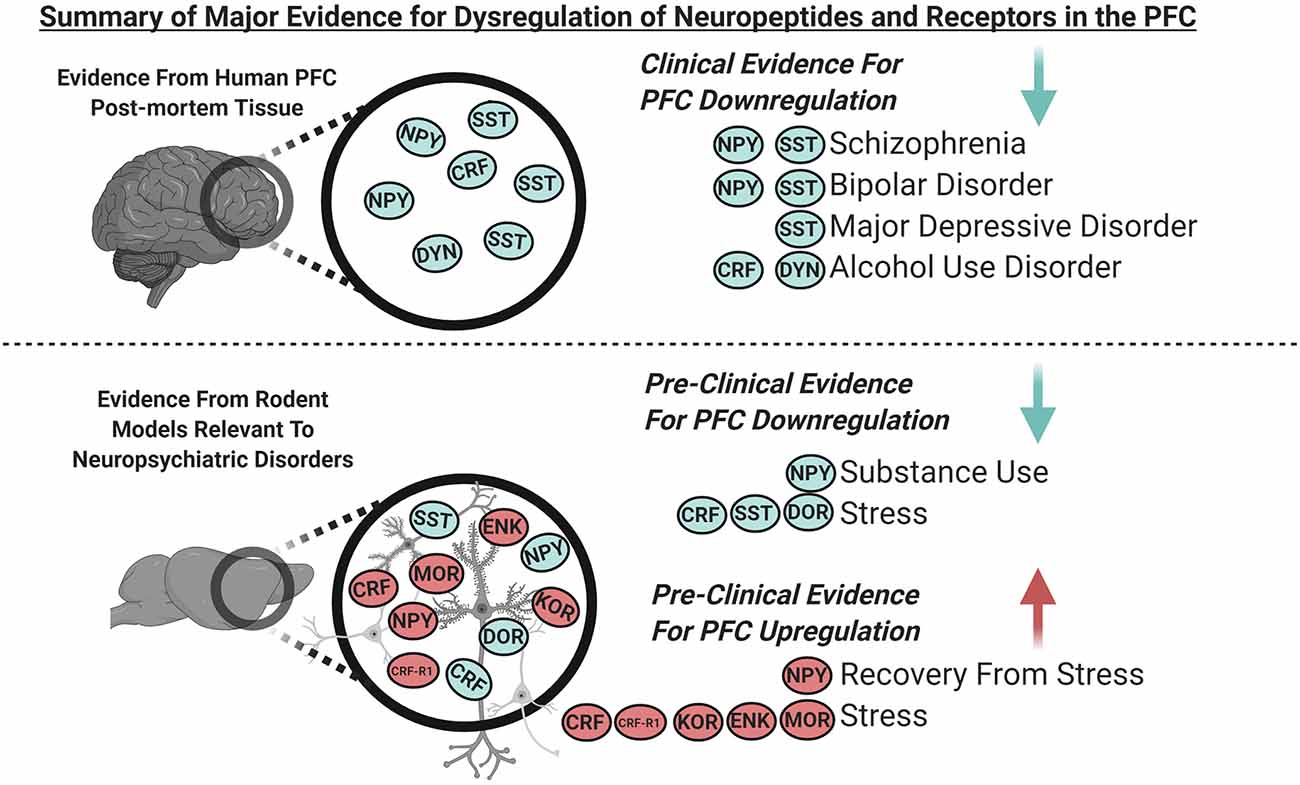
Figure 1. Graphical summary of the major clinical and pre-clinical findings covered in this review demonstrating dysregulated expression of neuropeptides and their receptors in the prefrontal cortex (PFC; blue indicates downregulation and red indicates upregulation). Clinical evidence from PFC of human post-mortem tissue indicates downregulation of somatostatin (SST) and neuropeptide Y (NPY) in schizophrenia and bipolar disorder, SST in major depressive disorder (MDD), and corticotropin-releasing factor (CRF) and dynorphin (DYN) in alcohol use disorder. Pre-clinical evidence from the PFC of rodent models relevant to neuropsychiatric disorders indicates that; NPY is downregulated with substance use, SST, and delta-opioid receptors (DOR) are downregulated following stress. NPY is upregulated during recovery from stress, and CRF receptor 1 (CRF-R1), kappa opioid receptors (KOR), enkephalin (ENK) and, mu-opioid receptors (MOR) are upregulated following stress. CRF is downregulated following acute stress and is increased following chronic stress.
It is important to note that the profound overlap and complexity in human neuropsychiatric diseases is not well recapitulated in animal models; many animal models represent a single representation of behavior involved in disease but do not fully encompass the actual human manifestation of the disease. Disorders like MDD and substance use disorder each have many criteria for diagnosis, of which an individual only needs to meet a few characteristics. This results in great heterogeneity in the expression of neuropsychiatric disorders, and likely, great heterogeneity in underlying causes. Also, the interpretation of some animal models has evolved (for example Commons et al., 2017), further muddling the literature. While these stipulations for the interpretation of the animal literature exist, preclinical behavioral models allow for greater investigation of the peptides in context: animal models allow for a greater teasing apart of the underlying neurocircuitry, synaptic dynamics, and relationship to other peptides and neurotransmitters. These positive and negative aspects of human and animal work may be in part responsible for the lack of cohesion between the preclinical and clinical literature, discussed in greater detail throughout the review.
Neuropeptide Y (NPY)
Neuropeptide Y Signaling and Overall Peptide Actions
Neuropeptide Y (NPY) is a 36-amino-acid neuropeptide with potent physiological effects and dense expression in the central and peripheral nervous systems (PNS; Tatemoto et al., 1982; Adrian et al., 1983; Allen et al., 1983). It is structurally similar to peptide YY and pancreatic polypeptide and is named for its abundance of tyrosine (Y) residues (Tatemoto et al., 1982). NPY in the PNS is co-localized with noradrenaline in sympathetic nerves (Lundberg et al., 1982), and has been shown to regulate sympathetic nervous system functions such as increasing blood pressure and causing vasoconstriction (Lundberg et al., 1982; Fuxe et al., 1983).
NPY in the CNS has been shown to modulate neural activity and regulates a variety of physiological functions including feeding, mood, and memory (Fuxe et al., 1983; Wahlestedt et al., 1989; Berglund et al., 2003; Sabban et al., 2016). A growing body of evidence indicates that NPY in the CNS plays a role in the stress response (Wahlestedt et al., 1989; Heilig, 2004; Thorsell, 2008), and stress-related neuropsychiatric disorders such as anxiety, depression (Wahlestedt et al., 1989; Zukowska-Grojec, 1995; Heilig, 2004; Hou et al., 2006), and substance use disorder (Ehlers et al., 1998; Pleil et al., 2015; Robinson and Thiele, 2017). NPY has gained attention as an anti-stress peptide, it promotes resilience to stress and reduces behaviors relevant to anxiety in vivo (Eaton et al., 2007; Cohen et al., 2012).
NPY is considered one of the most abundant neuropeptides in the CNS (Adrian et al., 1983; Allen et al., 1983). NPY containing neuronal cell bodies and axons are found in the PFC of several different species including humans (Chan-Palay et al., 1985; Eaton et al., 2007; Robinson et al., 2019a). NPY in the cortex is known to be expressed in non-pyramidal neurons, and like other neuropeptides, is co-localized with the inhibitory neurotransmitter GABA (Hendry et al., 1984). It is often co-expressed with other peptides, namely somatostatin. Furthermore, NPY is released following physiological stimulation of NPY expressing (NPY+) GABAergic neurons in other brain regions (Li et al., 2017).
NPY+ neurons in the PFC are thought to synapse locally as well as to potentially project to other regions (Chan-Palay et al., 1985). Interestingly NPY+ neurons form connections between subregions of the PFC (Saffari et al., 2016). NPY+ neurons in the infralimbic cortex have been found to synapse onto pyramidal cells in the prelimbic cortex. NPY+ neurons in the PFC are activated by the claustrum and mediate local inhibition over pyramidal cells (Jackson et al., 2018). Due to the nature of NPY signaling both locally within the PFC and connections with other regions, NPY in the PFC is positioned as a powerful regulator over cortical networks.
The effects of the NPY peptide in the CNS are mediated primarily by five different G-protein coupled receptors, Y1, Y2, Y4, Y5, and Y6 (Pedragosa-Badia et al., 2013), though like for many peptides, off-target effects have not been completely ruled out. The Y6 receptor is non-functional in humans and rats but is functional in mice (Starbäck et al., 2000). The function of NPY in the CNS has been most well-characterized on Gi/o-coupled Y1 and Y2 receptors (Kopp et al., 2002; Kash and Winder, 2006; Gilpin et al., 2011; Robinson and Thiele, 2017). The action of NPY is both cell and receptor-specific, for example, activation of Y1 but not Y5 receptors results in a rise in intracellular calcium in smooth muscle cells (Pons et al., 2008). In other regions, Y1 receptors act predominately postsynaptically, and Y2 receptors act predominately presynaptically.
NPY receptors in the PFC are found on both pyramidal neurons and GABAergic neurons and correspondingly, NPY has been shown to alter both inhibitory and excitatory signaling onto pyramidal neurons within the PFC (Vollmer et al., 2016), indicating circuit mediated effects. Vollmer et al. (2016) found that bath application of 1 μM NPY increases GABAA receptor-mediated inhibitory postsynaptic currents (IPSCs) and a decrease in evoked α-amino-3-hydroxy-5-methyl-4-isoxazolepropionic acid receptor (AMPAR)-mediated excitatory postsynaptic currents (EPSCs) onto layer 5 pyramidal cells in the infralimbic cortex. Collectively, this suggests that NPY may function to reduce action potential discharge of pyramidal neurons of the PFC. Moreover, NPY has been shown to increase the release of other neurotransmitters in the PFC such as dopamine (Ault and Werling, 1998). The physiological effect of NPY in the PFC likely depends on several factors, such as the postsynaptic cell, receptor subtype, and locus of action. More work is needed to provide a cohesive understanding of NPY’s neuromodulatory role in the PFC.
Stress and Post Traumatic Stress Disorder (Pre-clinical Evidence)
Pre-clinical rodent studies indicate that the effect of stress on NPY expression in the PFC is dependent on multiple factors, including the duration and type of stressor, the length of recovery, sex, and genotype. One study in Male Long Evans rats exposed to an acutely stressful event consisting of electric foot shocks found no significant change in NPY peptide after 7 days of recovery (Schmeltzer et al., 2015). Further, Schmeltzer et al. (2015) found that rats subjected to 7 days of chronic variable stress procedures, which also included foot shocks, showed no significant differences in NPY concentration in the PFC after 7 days of recovery. A separate study, also using male Long Evans rats subjected to 7 days of chronic variable stress, demonstrated no change in PFC NPY peptide 16 h following chronic variable stress; however, unlike the previous study, they found significantly increased NPY peptide in the PFC after 7 days of recovery (McGuire et al., 2011), suggesting NPY may be involved in adaptive responses to stress. However, a study using a longer duration of stress found that male Sprague–Dawley rats exposed to 36 days of a chronic unpredictable stress paradigm and 1 day of recovery demonstrated significantly reduced NPY mRNA in the PFC (Banasr et al., 2017). Together these studies suggest that the effect of stress on NPY may be dependent on the duration of the stressor, and specific dynamics of the stress procedure, including post-stress recovery time before data collection. It is possible that NPY is initially downregulated following long durations of stress but becomes upregulated during recovery as an adaptation, and more comprehensive studies are needed to confirm this hypothesis. Importantly, it has yet to be fully explored how dysregulation in NPY expression following stress exposure may be a positive or negative adaptation to further stress.
Sex hormones may also play a role in how stress affects NPY expression. A recent study explored NPY expression in the PFC in male, female, and ovariectomized female C57BL/6 mice exposed to 21 days of a chronic variable stress paradigm and 3 days of recovery (Karisetty et al., 2017). Karisetty et al. (2017) found increased NPY mRNA in the PFC in females but not in males or ovariectomized females—highlighting the increasing need for investigations into the role of sex differences. Taken together, these studies indicate NPY in the PFC is largely reduced during stress, whereas it becomes upregulated during recovery. However, the effect of stress on NPY in the PFC seems to be sex-dependent. Stress may be a contributing factor to the NPY pathology observed in neuropsychiatric disorders, and more studies are needed to understand how stress and recovery interact to regulate NPY expression in both males and females.
PTSD is a neuropsychiatric disorder that often occurs in individuals who have witnessed or experienced a traumatic or stressful event [American Psychological Association (APA) (2013)]. Symptoms of PTSD include intrusive thoughts, avoidance of reminders of a traumatic event, alterations in cognition and mood, alterations in arousal, and reactivity. Given the interaction between stress and NPY, NPY may play a role in behaviors associated with PTSD. McGuire et al. (2011) found that the increase in NPY after 7 days of chronic variable stress and 7 days of recovery in rats was associated with exaggerated fear response and recall. Also, NPY infused into the infralimbic region of the PFC in male Sprague–Dawley rats inhibits the consolidation of extinction, resulting in impaired retrieval of extinction memory via the Y1 receptor (Vollmer et al., 2016). In short, these preclinical findings indicate that increased NPY may contribute to fear-related behaviors associated with PTSD.
Human subjects with PTSD demonstrate decreased NPY in the cerebrospinal fluid (CSF; Sah et al., 2009, 2014). It is unknown how NPY regulation is changed in the PFC of PTSD subjects. Pre-clinical findings from Vollmer et al. (2016) would suggest increased NPY expression in the PFC of PTSD patients however clinical studies are needed to confirm this hypothesis. Importantly, the causes of PTSD in humans is incredibly varied—ranging from life events (Simon et al., 2020), natural disasters (Cénat et al., 2020), domestic violence (Kofman and Garfin, 2020), pandemics (Kaseda and Levine, 2020) to other causes, and it is unlikely that the animal literature models the breadth of these events.
Substance Use Disorder (Pre-clinical Evidence)
To meet the criteria for substance use disorder (including alcohol use disorder) individuals must display at least 2 of 11 symptoms ranging from impaired control over substance use, social impairment, risky behavior, and the development of tolerance and withdrawal [American Psychological Association (APA) (2013)]. Several animal studies that investigated the role of NPY in the CNS on alcohol consumption indicate that lack of NPY can promote alcohol consumption. For instance, NPY knockout mice exhibit increased alcohol consumption (Thiele et al., 1998, 2000; Robinson and Thiele, 2017). Recently, the relationship between NPY in the medial PFC (mPFC) and binge alcohol consumption was explored in male and female C57BL/6J mice using the drinking in the dark paradigm (Robinson et al., 2019a). Robinson et al. (2019a) found that binge drinking reduced NPY immunoreactivity in the mPFC. Also, Robinson et al. (2019a) discovered opposing effects of Y1 and Y2 receptors in the mPFC, consistent with the literature on NPY and drinking elsewhere in the brain, such as the BNST (Kash et al., 2015). Robinson et al. (2019a) found that separate activation of Y1 receptors and inhibition of Y2 receptors both resulted in decreased binge ethanol intake in the mPFC, suggesting that NPY may reduce alcohol consumption through activation of Y1 receptors. Because Y2 receptors are predominantly auto-receptors on NPY neurons, antagonism of the Y2 receptor may promote activation of NPY neurons and subsequent NPY Y1 receptor activation, thus the synaptic location may account for this differential effect. NPY Y1 and Y2 receptors both signal through Gi/o signaling cascades, little evidence thus far suggests differences in NPY affinity for these receptors, or in the intracellular signaling cascades at either receptor (Kash et al., 2015), thereby supporting Robinson et al.’s (2019a) conclusion that synaptic location and local circuitry are at play. Moreover, other drugs of abuse such as cocaine lead to reductions in NPY in the PFC (Wahlestedt et al., 1991). These findings indicate that NPY and its receptors play an important role in alcohol consumption, and the effect of NPY likely depends largely on the specific NPY receptor which is activated. NPY and its corresponding receptors in the PFC are hypothesized to regulate behaviors associated with substance use disorder in humans, but clinical investigations are needed to confirm this hypothesis. Importantly, NPY is downregulated by multiple forms of substance use (both binge alcohol and cocaine) in animal models.
Major Depressive Disorder (Pre-clinical and Clinical Evidence)
MDD is characterized by a combination of symptoms including, depressed mood and loss of interest or pleasure, present during the same 2-week period [American Psychological Association (APA) (2013)]. Clinical studies indicate that NPY concentration in the frontal cortex is largely unchanged in patients with a clinical diagnosis of MDD, however, it may be involved with emotional regulation. One study found NPY was significantly lower in victims of suicide when compared to accidental-death control subjects (Widdowson et al., 1992) suggesting that NPY deficits in this region may be linked with emotional regulation and depression. However, a subsequent study did not support a role for NPY in MDD (Ordway et al., 1995). When comparing victims of suicide with a co-occurring diagnosis of MDD to accidental-death controls with no diagnosed psychiatric disorders, there was no significant difference in NPY concentration (Ordway et al., 1995). Consistent with this result, another study in humans found no change in the levels of PFC NPY in subjects diagnosed with MDD (Kuromitsu et al., 2001). The relationship between MDD and suicide is complex and remains to be fully elucidated, particularly in terms of causality, therefore while NPY may play a role in emotional regulation, it appears largely unaffected in the clinical population diagnosed with MDD. Further, there were no significant differences in prefrontal Y1 or Y2 receptor mRNA between control and MDD subjects (Caberlotto and Hurd, 2001). Together, clinical studies indicate that in humans diagnosed with MDD, expression NPY and NPY Y1 and Y2 receptors in the PFC are largely unchanged.
Genes regulating NPY have been shown to interact with environmental factors such as stress to increase susceptibility to negative emotional symptoms associated with anxiety and depression (Sommer et al., 2010). Sommer et al. (2010) identified a variant allele in the NPY promoter which results in increased NPY mRNA in the anterior cingulate cortex subregion of the PFC. This variation in the NPY gene increases susceptibility to stress and may contribute to the symptoms of depression. This finding in humans links genetic regulation of NPY with emotional symptoms following stress, however, it does not provide direct evidence for the role of NPY in stress or MDD.
Despite these clinical findings, pre-clinical rodent models exhibiting behaviors associated with depression point towards decreased NPY in the PFC. Both types of NPY mRNA were found to be downregulated in the PFC of a rat model of depression (Flinders Sensitive Line, FSL) when compared to controls (Flinders Resistant Line, FRL; Melas et al., 2012). The FSL is a selectively bred rat line that partially resembles the behavior of depressed individuals and exhibits other neurochemical changes associated with depression (Overstreet et al., 2005). Another model using an intraperitoneal injection of lipopolysaccharide (LPS) to induce depressive-like behavior in Sprague–Dawley rats found decreased NPY and Y2 receptor expression in the mPFC (Wang et al., 2019). It is important to note that these models do not fully capitulate the disease pathology observed in humans with MDD, and their construct, face, and predictive validity must be assessed when comparing these models with clinical populations.
Preclinical studies using rodent models are helping to investigate the potential of NPY to modulate behaviors associated with depression. Wang et al. (2019) also found that NPY itself can reduce depressive-like behavior (as measured by open field test and sucrose preference test) in LPS treated rats when administered in the PFC. Local infusion of NPY into the mPFC reduced LPS-induced depressive-like behaviors in both the open field test and sucrose preference test in Sprague–Dawley rats. This effect was determined to be mediated by the Y2 receptor, as PFC administration of the Y2 receptor antagonist abolished, and administration of Y2 receptor agonist mimicked, antidepressant-like behavioral effects of NPY. However, a different study in male Sprague–Dawley rats found that NPY infusion into the infralimbic cortex subregion of the mPFC did not affect depression-like behavior in the forced swim test (Vollmer et al., 2016). As interpretations of behavior in the forced swim test continue to evolve (Commons et al., 2017), NPY may affect a specific subset of behaviors associated with depression such as the behaviors measured by the open field test and sucrose preference test and not affect other types of behaviors such as those measured by forced swim test, and more studies are needed to determine the specific behavioral effect of NPY. Another possibility is that the effect of NPY in the PFC is region-specific even within the PFC, and its effect may depend largely on the site of injection. The pre-clinical work demonstrates support for the hypothesis that NPY plays a role in regulating a specific subset of behaviors associated with depression, and further pre-clinical and clinical studies are needed to fully examine this hypothesis.
Schizophrenia and Bipolar Disorder (Pre-clinical and Clinical Evidence)
Many neuropsychiatric disorders have been linked with reductions in PFC NPY, suggesting that it plays an essential role in regulating emotional behaviors. Notably, NPY in humans is found to be altered in schizophrenia, and bipolar disorder (Wu et al., 2011). Schizophrenia is characterized by symptoms such as delusions, hallucinations, social and occupational dysfunction [American Psychological Association (APA) (2013)]. Bipolar disorder is characterized by extreme emotional states that occur at distinct times called mood episodes. These mood episodes are often characterized as manic, or depressive. Multiple studies have observed expression deficits in NPY and NPY mRNA in the PFC from post-mortem tissue of subjects with schizophrenia and subjects with bipolar disorder (Gabriel et al., 1996; Kuromitsu et al., 2001; Hashimoto et al., 2008a), although it is important to note that Caberlotto and Hurd (1999) found decreased NPY mRNA only in post mortem tissue of subjects with a clinical diagnosis of bipolar disorder, and not those with schizophrenia or other disorders. Moreover, NPY Y1 and Y2 receptor mRNA in the PFC was unaltered in post mortem tissue of subjects with bipolar disorder, and schizophrenia when compared to healthy controls (Caberlotto and Hurd, 2001). Male rats treated with Lithium exhibited increased NPY-like immunoreactivity in the frontal cortex, suggesting that NPY may be involved in the response to treatments to MDD and bipolar disorder. Together these findings indicate that deficits in NPY expression in the PFC are observed in both schizophrenia and bipolar disorder.
Corticotropin-Releasing Factor (CRF)
CRF Neuropeptide Signaling and Overall Actions
Corticotropin-releasing factor (CRF) also known as Corticotropin-releasing hormone (CRH; referred to here as CRF) is a 41-amino acid neuropeptide which belongs to a family of neuropeptides including Urocortin 1 (Vaughan et al., 1995), Urocortin 2 (Reyes et al., 2001), and Urocortin 3 (Lewis et al., 2001). CRF was first characterized by hypothalamic extracts for its ability to stimulate the release of corticotropin and beta-endorphin from rat anterior pituitary cells in vitro (Vale et al., 1981). CRF is highly expressed in the paraventricular nucleus of the hypothalamus (Swanson et al., 1983) where it acts to activate the primary stress response pathway or hypothalamic pituitary adrenal (HPA) axis by promoting the release of stress hormones such as glucocorticoids and cortisol from the adrenal gland (Turnbull and Rivier, 1997; Bale and Vale, 2004; Dedic et al., 2017).
In addition to its role in the hypothalamus, CRF acts in other regions of the CNS where it functions to robustly modulate circuit function and to regulate behaviors associated with stress and addiction (Koob and Heinrichs, 1999; Kash and Winder, 2006; Orozco-Cabal et al., 2006; Silberman et al., 2013). CRF is widely distributed throughout the mammalian brain and is highly expressed in the PFC of mammals including humans (Pandey et al., 2019), rats (Swanson et al., 1983), and mice (Chen et al., 2020). CRF expressing neurons (CRF+) are found in the PFC and are predominately in layers II and III (Swanson et al., 1983). CRF+ neurons in the PFC are a subclass of inhibitory neurons, a large portion of which also express vasoactive intestinal polypeptide (VIP) or calretinin (Chen et al., 2020). Importantly, CRF+ neurons in the PFC become active during stress (Chen et al., 2020) and withdrawal (George et al., 2012). Activation of PFC CRF+ neurons results in local CRF release to modulate cognition and behavior (Hupalo et al., 2019b). Direct administration of CRF into the PFC results in impaired working memory, and CRF antagonism improves working memory, indicating that CRF acts in the PFC to regulate cognitive behaviors (Hupalo and Berridge, 2016). The dysfunction of CRF in the CNS occurs in stress-related disorders including PTSD, MDD, and anxiety (Hupalo et al., 2019a). Taken together, these findings suggest an important interaction between stress and CRF release which may contribute to neuropsychiatric disease.
The action of CRF is exerted through two major G protein-coupled receptors subtypes: CRF-R1 and CRF-R2 (Lovenberg et al., 1995; Perrin et al., 1995; Grammatopoulos et al., 2001; Dautzenberg and Hauger, 2002; Hauger et al., 2003). CRF-R1 and CRF-R2 are present in the PFC, however, CRF-R2 is expressed at low levels in the PFC of rodents (de Souza et al., 1985; Millan et al., 1986; Sánchez et al., 1999; Van Pett et al., 2000). CRF receptor expression in the cortex shows a high correlation with the distribution of CRF (de Souza et al., 1985) and CRF depresses excitatory synaptic transmission in PFC slices (Zieba et al., 2008). This supports the importance of CRF as a neuromodulator in the PFC.
In other regions such as the BNST and CEA, CRF-R1 and CRF-R2 have been found to exert opposing roles on physiology and stress-induced behaviors (Liu et al., 2004; Funk and Koob, 2007; Lowery-Gionta et al., 2012; Tran et al., 2014). Given the differences between CRF-R1 and CRF-R2 in the PFC, this effect is likely consistent. For instance, CRF-R1 acts postsynaptically, while CRF-R2 acts presynaptically in the PFC (Orozco-Cabal et al., 2008). CRF-R2 is present on presynaptic terminals in the PFC which originate from CRF+ neurons in the basal lateral amygdala (BLA; Yarur et al., 2020). Activation of presynaptic CRF-R2 limits the excitatory transmission to the PFC from the basolateral amygdala. CRF has a higher affinity for CRF-R1 (Lovenberg et al., 1995) and CRF-R2 is expressed at lower levels as compared to CRF-R1 in the PFC of rodents (Van Pett et al., 2000). The opposing function of CRF receptors is similar to the opposing function of NPY-Y1 and Y2 receptors, and the ratio of these two CRF receptors may be responsible for the net effect of the peptide in that region. Therefore, dysregulation in the expression of one or both of these receptors may contribute to the dysfunction of the CRF system.
This review focuses specifically on CRF and its action in the PFC although CRF+ neurons whose cell bodies are located in the PFC also project to other regions where they release CRF. For instance, CRF+ neurons in the PFC project to the nucleus accumbens (NAc; Itoga et al., 2019) where they act to modulate behavior through activation of CRF receptors in those regions (Kai et al., 2018). It is important to note that males and females have been shown to exhibit different behavioral responses to CRF (Wiersielis et al., 2016). Despite this finding, much of the work on the role of PFC CRF has focused exclusively on male rodents, and sex differences have been understudied. Future work is warranted to examine how dysfunction in the CRF system may contribute to behaviors related to anxiety and neuropsychiatric disorders separately in males and females.
Stress and Post Traumatic Stress Disorder (Pre-clinical Evidence)
Multiple pre-clinical studies indicate that stress impacts the expression of CRF and CRF receptors and CRF may contribute to behaviors such as cognitive deficits associated with neuropsychiatric disease. Few preclinical studies have examined the relationship between stress and CRF-R2, and most work has focused largely on CRF-R1.
The relationship between stress, CRF, and CRF receptors in the PFC depends on the condition and duration of the stressor. Rodent models demonstrate that acute stress increases CRF and CRF-R1 mRNA in the PFC. Acute restraint stress increases both CRF mRNA and CRF-R1 mRNA in the PFC of Sprague–Dawley male rats (Meng et al., 2011). Moreover, mice (C57Bl/6N and CD1) exposed to acute social defeat stress exhibited increased CRF-R1 mRNA in the cingulate, prelimbic, and infralimbic regions of the PFC (Uribe-Mariño et al., 2016). Moreover, two stress-based rodent models of PTSD-like behaviors (though rodent models of PTSD are still evolving and emerging), one which subjected male rats to a single prolonged stressor consisting of 2-h restraint and 20 min forced swim (Wang et al., 2019), and another which subjected adolescent rats to inescapable electric foot shocks (Li et al., 2015) both resulted in increased CRF-R1 in the PFC. These experiments provide pre-clinical evidence that acute stress leads to increased CRF and CRF-R1 expression within the PFC.
Animal models of chronic stress, on the other hand, demonstrate that chronic stress leads to unchanged or decreased CRF mRNA, representing a possible adaptation to repeated stress. One study found Male Sprague–Dawley rats subjected to chronic immobilization stress (3 h a day for 21 days) showed no change in PFC CRF mRNA (Chen et al., 2008). A separate study found chronic social defeat stress (10 days) in Wistar male rats resulted in decreased CRF mRNA and increased CRF-R1 mRNA in the PFC (Boutros et al., 2016). Importantly, both acute and chronic stress lead to increased expression of CRF-R1.
CRF in the PFC has been shown to regulate behaviors associated with PTSD. Infusion of CRF into the vmPFC produces avoidance of stimuli paired with a traumatic stressor (Schreiber et al., 2017). Conversely, blockade of CRF signaling via CRF-R1 antagonism in the vmPFC reverses avoidance of stimuli paired with traumatic stress. Furthermore, CRF-R1 activation in the PFC following acute social defeat stress results in cognitive dysfunction (Uribe-Mariño et al., 2016). This suggests that increased CRF and CRF-R1 may contribute to behaviors associated with PTSD, and future clinical studies are needed to test this hypothesis.
Taken together these pre-clinical findings suggest that acute stress results in increased CRF whereas chronic stress results in decreased CRF. Both acute and chronic stress result in increased CRF-R1. Interestingly, CRF itself positively regulates the expression of CRF-R1 in cultured neurons (Meng et al., 2011). This suggests that the release of CRF in the PFC in response stressors may correspondingly regulate expression of CRF-R1. Increased CRF-R1 following acute stress may represent a neuroadaptation to increased CRF expression in response to stress, and this may upregulation may persist despite the downregulation of CRF with chronic stress. Deviation from homeostatic levels of CRF and CRF-R1 may contribute to PFC neurological dysfunction observed in neuropsychiatric disorders. Further pre-clinical studies are needed to compare the effects of acute and chronic stress on the expression of CRF, CRF-R1, and CRF-R2. Moreover, investigations into the expression of CRF and its receptors at multiple time points during a prolonged stressor may provide insight into the role of the CRF system in adaptation to stress.
Anxiety and Depression (Pre-clinical and Clinical Evidence)
Clinical and pre-clinical studies both point towards a role for CRF and its receptors in anxiety and depression (for review and synthesis see Owens and Nemeroff, 1993). It has been well validated that the concentration of CRF is increased in the CSF of depressed patients and suicide victims. In the PFC, pre-clinical evidence indicates increased CRF-R1 receptor expression in response to both acute and chronic stress. Given the connection between stress and depression this effect might suggest that the clinical literature would show an increase in CRF receptor expression in humans with symptoms of depression, however, this is paradoxically not the case. Clinical evidence indicates that suicide victims exhibit a reduced density of CRF receptors in the frontal cortex as evidenced by a 23% reduction in CRF binding sites in brain tissue compared to healthy controls (Owens and Nemeroff, 1993). There are various possible reasons for these discrepancies. Importantly, the relationship between stress and suicide is not well established (i.e., not all suicide is precipitated by clear stressors, and not all stress leads to suicide). Also, Owens and Nemeroff (1993) did not distinguish between CRF-R1 and CRF-R2, and CRF-R2 is known to be less prominent in rodents than in primates. Further clinical studies are needed to determine the expression of CRF, CRF-R1, and CRF-R2 in the PFC of patients with depression. Most pressing, is that post-mortem evidence from victims of suicide does not encompass the large range of symptomology of depression, and investigation of other categories, such as MDD, is important for comparison to the animal literature.
Pre-clinical animal models also provide support for the role of PFC CRF in behaviors associated with anxiety and depression. Microinjection of CRF (0.02 μg) into the mPFC increased anxiety-like behavior in the elevated plus-maze (EPM) in both acute and chronically stressed rats (Jaferi and Bhatnagar, 2007). Interestingly, in unstressed rats, microinjection of a larger amount of CRF (0.2 μg) into the frontal cortex reduced anxiety-like behavior in the EPM. One study using various dosages of CRF in unstressed male Wistar rats found that CRF exerts opposing effects on anxiety-related behavior in the EPM depending on dose (Ohata and Shibasaki, 2011). CRF microinjected into the mPFC increases anxiety-like behavior in the EPM at lower doses (0.05 μg) and reduces anxiety-like behavior at higher doses (1.0 μg). Together, these studies reveal that CRF acts to modulate behavior associated with anxiety and depression as measured by the EPM in rodents, and the directionality of this effect may be in part, state-dependent and in part, dose-dependent.
The effect of CRF on anxiety-like behavior depends on the activation of CRF-R1. CRF microinjected into the mPFC of male Swiss mice increased anxiety-like behavior in the EPM and importantly, when a CRF-R1 antagonist was microinfused before CRF, the effect of CRF on anxiety-like behavior was blocked (Miguel et al., 2014). This study indicates that the effect of CRF on anxiety-like behavior is dependent on the CRF-R1. A separate study in male CD1 mice exposed to a live predator demonstrated that infusion of a CRF-R1 specific agonist into the mPFC reduced anxiety-like defensive behaviors including avoidance and freezing (Pentkowski et al., 2013). This supports the hypothesis that activation of CRF-R1 within the PFC regulates various behaviors associated with anxiety. CRF via activation of CRF-R1 in the PFC has also been shown to regulate anxiety-related behaviors through the sensitization of serotonin 5-hydroxytryptamine receptor subtype 2 (5-HT2R) signaling (Magalhaes et al., 2010), indicating a possible interaction between CRF and neurotransmitters such as serotonin. Collectively, these studies indicate that CRF plays a dual role in modulating anxiety-like behaviors through the activation of CRF-R1. Deviation from homeostatic levels of CRF may contribute to the pathology of anxiety and depression, and future clinical studies are needed to confirm this hypothesis.
Substance Use Disorders (Pre-clinical and Clinical Evidence)
CRF systems in the brain become activated by stressors including excessive drug use, and dysfunction of CRF contributes to negative emotional states associated with withdrawal and addiction (Koob, 2013; McReynolds et al., 2014; Zorrilla et al., 2014). The human literature points towards altered CRF and its receptors in the PFC as playing a role in various substance use disorders. Post-mortem tissue from individuals with alcohol use disorder exhibit significantly decreased CRF, CRF-R1, and increased CRF-R2 mRNA in the PFC (Gatta et al., 2019). Genetic variation in CRF receptors also contributes to increased maladaptive substance use. Human genetic variation in CRF-R1 (rs110402) has been shown to interact with stress to modulate alcohol consumption and PFC activity (Glaser et al., 2014). Overall, the clinical data support a role for CRF, CRFR-1, and CRFR-2 in the PFC in substance use disorders specifically alcohol use disorder, and genetic variation in this system may contribute to the pathology.
Pre-clinical animal models also demonstrate that drug use can alter CRF expression through various mechanisms. Chronic nicotine use decreases CRF mRNA in the PFC of rodents (Carboni et al., 2018). Male Sprague–Dawley rats exhibited decreased CRF mRNA following chronic nicotine exposure (0.4 mg/kg intraperitoneal once daily for 5 days) while CRF was unchanged following acute administration of nicotine (0.4 mg/kg intraperitoneal once daily for 1 day; Carboni et al., 2018). No change in CRF mRNA was observed following 3 cycles of binge drinking in male C57BL/6J mice, however, these mice exhibited decreased CRF binding protein in the PFC (Ketchesin et al., 2016). CRF binding protein (CRF-BP) is expressed in the PFC and binds CRF with a high affinity to regulate the activity of CRF receptors (Ketchesin et al., 2017). On the other hand, heroin self-administration was not associated with alterations in CRF mRNA or CRF-BP mRNA in male Sprague–Dawley rats (McFalls et al., 2016). The variability in these results indicates that the relationship between drug use and altered CRF depends on several factors including the pharmacology of the drug in question, and the duration of drug use or abuse. Also, the difference may emerge based on whether the drug was experimenter-administered (i.e., intraperitoneal) or consumed by choice. Moreover, CRF activity can be regulated independently by other factors such as CRF-BP.
Alterations to CRF receptors in the PFC observed in humans with alcohol use disorder (Gatta et al., 2019) are also associated with various forms of substance use in rodents. Deficits in CRF are characteristic of high drinking alcohol-preferring male rats—for example, Ehlers et al. (1992) note decreased CRF concentration in the PFC of these rats. Besides these rats have been demonstrated to have increased cortical activity in the frontal cortex following CRF administration, suggesting that CRF receptors in this region may also be dysregulated (Ehlers et al., 1992). Increased heroin self-administration is associated with increased CRF-R1 in the PFC in male Sprague–Dawley rats (McFalls et al., 2016). CRF-R1 antagonism in the PFC reduced impulsivity and resulted in profound reductions in binge motivated alcohol drinking in male and female rats who had undergone early life maternal separation (Gondré-Lewis et al., 2016). Both chronic nicotine (Carboni et al., 2018) and repeated cocaine exposure (Orozco-Cabal et al., 2008) resulted in increased CRF-R2 expression in the PFC in male Sprague–Dawley rats.
CRF receptors have been shown to differentially regulate ethanol use behavior. In other brain regions such as the central extended amygdala (CEA) CRF-R1 (Lowery-Gionta et al., 2012) and CRF-R2 (Funk and Koob, 2007) play opposing roles in ethanol consumption. Substance use can modulate excitatory BLA inputs to mPFC through activation of presynaptic CRF-R2 (Orozco-Cabal et al., 2008). Orozco-Cabal and colleagues demonstrated that chronic cocaine results in increased functionality of presynaptic CRF-R2 and loss of postsynaptic function of CRF-R1 in the PFC of male rats. Moreover, an interesting, recent study found that inhibition of CRF-R2 and separate activation of CRF-R1 in the PFC both resulted in decreased binge-like ethanol consumption in male and female C57BL/6J mice, confirming that much like in the CEA, these two receptors may play opposite roles in substance use (Robinson et al., 2019b). In this work, Robinson et al. (2019b) demonstrated that co-administration of CRF-R1 and CRF-R2 antagonists attenuated the behavioral effect of CRF-R1 antagonist. This suggests that decreased binge-like ethanol drinking resulting from inhibition of CRF-R1 may result from increased activation of the CRF-R2, providing strong evidence in support of an important role of both CRF-R1 and CRF-R2 in the PFC in regulating substance abuse. However, in separate work, blocking CRF-R2 in the PFC partially inhibited cocaine-primed reinstatement of cocaine conditioned place preference (Guan et al., 2014). Overall, these findings highlight how different substances may differentially affect CRF and its receptors. These pre-clinical finding along with the clinical finding from Gatta et al. (2019) suggest that CRF-R1 and CRF-R2 may play opposing roles in substance use, and more studies are needed to confirm this hypothesis.
Somatostatin (SST)
SST Neuropeptide Signaling and Overall Actions
Somatostatin (SOM or SST), also known as somatotropin releasing inhibitory factor (SRIF; referred to here as SST), was characterized over 50 years ago as a hypothalamic extract capable of inhibiting the release of growth hormone from the rat anterior pituitary in vitro (Krulich et al., 1968). Somatostatin was originally described as a 14 (SST-14) amino acid peptide (Brazeau et al., 1973). Later, a second N-terminally extended bioactive form consisting of 28 amino acids (SST-28) was isolated and characterized (Pradayrol et al., 1980). Both isoforms are generated from the same precursor, prosomatostatin (Benoit et al., 1990). SST exhibits diverse physiological effects such as regulation of visceral functions, and inhibition of a variety of biological processes including anterior pituitary hormone secretion, insulin secretion, glucagon secretion, immune responses, DNA synthesis, and cell division (Brown and Taché, 1981; Kumar and Grant, 2010; Eigler and Ben-Shlomo, 2014; Morisset, 2017). In short, somatostatin is known to inhibit various cellular processes such as the secretion of hormones and other secretory proteins (Benali et al., 2000; Morisset, 2017). Somatostatin has been gaining attention for its role in the CNS as a neuromodulator, and in regulating behaviors linked to stress including substance abuse and affective disorders (Liguz-Lecznar et al., 2016; Robinson and Thiele, 2020).
SST in the CNS is highly evolutionarily conserved, and expression has been observed in several different species including humans, non-human primates, and rodents (Iritani and Satoh, 1991). There is a large amount of SST expression (both SST-14 and SST-28) in the PFC (Hayashi and Oshima, 1986; Lewis et al., 1986). Also, SST+ immunoreactive neurons are present at high densities in the PFC in several non-human species including macaque monkeys (Yamashita et al., 1989). SST expression is often used to classify inhibitory GABAergic neurons which mainly synapse on the dendrites of pyramidal cells within the cortex (Melchitzky and Lewis, 2008), though they have also been shown to project onto other populations including inhibitory neurons in the PFC (Cummings and Clem, 2020). SST+ neurons in the PFC have been shown to release SST under basal or tonic conditions as well as following activation (Dao et al., 2019); therefore, changes in the number or activity of SST cells in the PFC may not only result in altered GABAergic signaling but also altered SST tone. The GABAergic properties of SST cells in the cortex are known to control network activity, and the implications of SST-specific GABAergic dysfunction on neuropsychiatric disorders have been previously reviewed (Liguz-Lecznar et al., 2016; Urban-Ciecko and Barth, 2016; Robinson and Thiele, 2020).
SST exerts its biological function by activating any of five g protein-coupled SST receptors (SST-R1 to SST-R5) which are predominately Gi/Go coupled and result in inhibition of adenylyl cyclase (Patel et al., 1994; Liguz-Lecznar et al., 2016). SST-14 and SST-28 both bind and activate SST receptors with differing affinities (for example, SST-28 exhibits a greater affinity for SST-R5 than SST-14 (Liguz-Lecznar et al., 2016). SST-14 and SST-28 have been shown to exhibit differing biological effects (Hadjidakis et al., 1986). Several SST agonists and antagonists are used clinically for the treatment of diseases such as acromegaly and neuroendocrine tumors (Rai et al., 2015).
All five somatostatin receptors have been observed by immunohistochemistry in the frontal cortex of the human brain (Kumar, 2005). SST-R1 immunoreactivity is observed in the dendrites and soma of both pyramidal and non-pyramidal cells in the frontal cortex (Kumar, 2005) and is mainly presynaptic in other regions (Liguz-Lecznar et al., 2016). SST-R2 immunoreactivity was found to be confined mainly to pyramidal cells and was abundantly expressed in dendrites and processes (Kumar, 2005). SST-R3 immunoreactivity was less predominant and was observed in pyramidal cells as well as other cells such as immune cells in the frontal cortex (Kumar, 2005). SST-R3 has been shown to exist on neuronal cilia in other regions (Liguz-Lecznar et al., 2016). SST-R4 and SST-R5 expression was observed in the dendrites (Kumar, 2005).
In vitro studies have demonstrated that the response of cortical neurons to SST is dependent upon the concentration and corresponding receptor activation (Delfs and Dichter, 1983). Delfs and Dichter (1983) found that in cultured rat cortical neurons low concentrations of SST-14 (100 pM–1 μM) caused an excitatory response and depolarization in neurons while at higher concentrations (10 μM–1 mM) SST-14 was more likely to have no effect or to produce an inhibitory response. SST-14 and SST-28 have also been demonstrated to exhibit opposing effects on rat cortical neurons in culture (Wang et al., 1989). Wang et al. (1989) found SST-14 increased a delayed rectifier potassium current in cortical neurons, while SST-28 reduced the current. A separate study found somatostatin applied microiontophoretically to neurons in the frontal cortex elicited a dose-dependent increase in activity and caused excitation in pyramidal cells (Olpe et al., 1980). This excitatory response was likely not a result of decreased GABAergic inhibition supporting a role for somatostatin in increasing frontal cortical activity. With the recent development of receptor-specific agonists and antagonists, there is a pressing need for rigorous region and receptor-specific investigations into the neurophysiological effects of SST. Moreover, few studies have investigated both SST-14 and SST-28, and some fail to differentiate between the two. Therefore, future studies investigating the effect of both SST-14 and SST-28 are warranted.
Results concerning the action of SST on neuronal activity are sparse, and very few experiments have been conducted in the PFC. SST+ neurons in the PFC have been shown to release somatostatin in an activity dependent manner (Dao et al., 2019), and dopamine can stimulate cortical SST release (Thal et al., 1986). The release of SST in the PFC indicates that it likely functions as an important neuromodulator in this region. A thorough investigation of SST’s pharmacological action on neurotransmission in the PFC would provide the field with a framework for understanding how SST release impacts neurotransmission and behavior, and how deficits in SST observed in neuropsychiatric disorders contributes to PFC dysregulation. Importantly, studies of this nature may bridge the gap between the literature concerning the activity of SST neurons and corresponding neuropsychiatric diseases.
Stress (Pre-clinical Evidence)
Somatostatin mRNA and peptide in the PFC are reduced following stress in rats (Banasr et al., 2017; Li et al., 2018). Male Sprague–Dawley rats exposed to 36 days of chronic unpredictable stress and 1 day of recovery exhibited significantly decreased SST mRNA measured by quantitative polymerase chain reaction (qPCR) in the PFC when compared to home cage control (Banasr et al., 2017). A study using liquid chromatography-mass spectrometry to probe the effect of multiple stressful experiences during adolescence on a broad range of neuropeptides in the PFC and hippocampus in adulthood uncovered deficits in SST-28 following adolescent stress (Li et al., 2018). Li et al. (2018) found male Wistar Han rats that underwent the peripubertal stress protocol (including exposure to fox odor and elevated platform) from postnatal day 28–42 as well as 1 h of restraint stress before sacrifice demonstrated decreased SST-28 in the PFC. In both studies the decrease was not significant in the hippocampus, suggesting that changes in SST following stress are region specific (Banasr et al., 2017; Li et al., 2018).
Stress has also been shown to affect the levels of SST receptors in the PFC (Faron-Górecka et al., 2018). Male Wistar Han rats exposed to 7 weeks of chronic mild stress exhibited increased SST-R2 binding in the PFC following stress (Faron-Górecka et al., 2018). The literature shows conflicting results concerning the effect of stress on SST+ cell number and may depend on the sex and duration of stress. Male and female SST-tdT reporter mice exposed to 14 days of chronic unpredictable stress displayed significantly decreased SST-tdT+ neurons compared to control (Girgenti et al., 2019), while in a similar study, no significant decrease in SST cell number in male Wistar rats subjected to 9 weeks of chronic mild stress was observed (Czéh et al., 2018). The longer duration of stress and the use of only males in Czéh et al. (2018) may partially account for the different results. Studies investigating the effects of both acute and chronic stress of different durations may help to uncover how the concentration of SST changes throughout a stressor.
Major Depressive Disorder and Bipolar Disorder (Pre-clinical and Clinical Evidence)
Ample clinical evidence points towards decreased SST in human subjects with MDD and bipolar disorder. Subjects with bipolar disorder exhibit decreased SST mRNA in the PFC (Fung et al., 2014). Post mortem tissue from subjects with MDD exhibit a significant reduction in the expression of SST mRNA (measured by qPCR) and SST precursor protein (measured by western blot for prepro-SST) in the dorsolateral PFC (Sibille et al., 2011) and subgenual anterior cingulate cortex (Tripp et al., 2011). Recent studies have linked brain-derived neurotrophic factor (BDNF) expression with altered SST. Deficits in BDNF lead to decreased SST (Du et al., 2018), and BDNF itself may be required to maintain SST gene expression (Glorioso et al., 2006). This is consistent with the similar developmental expression profile of BDNF and SST mRNA which both increases during early adolescence (Du et al., 2018) and subsequently decrease with aging (Hayashi et al., 1997; McKinney et al., 2015). Decreased SST mRNA in the PFC in human subjects with MDD is correlated with reduced BDNF mRNA (Oh et al., 2019). Oh et al. (2019) found that C57BL/6J mice exposed to 7 weeks of chronic stress (an unpredictable chronic mild stress protocol) exhibited deficits in dendritic BDNF in the PFC and this decrease in dendritic BDNF may lead to a low neurotropic supply to SST neurons. Therefore, decreased BDNF may contribute to reduced SST expression and behavioral symptoms of depression (Oh et al., 2019).
Antidepressants have also been shown to modulate SST and SST receptors in the PFC in rodents. Male Sprague–Dawley rats chronically administered the antidepressant citalopram exhibited increased SST and SST-R2 density (measured by autoradiography) in the PFC and frontal cortex respectively (Pallis et al., 2009). No change in SST in the PFC was observed after treatment with the antidepressant desmethylimipramine indicating that the effect may be dependent on the pharmacology of the antidepressant. Male Sprague–Dawley rats also showed no differences in somatostatin receptors [measured by (125i)Tyrl 1-somatostatin binding] in the PFC following acute and chronic desipramine treatment (Gheorvassaki et al., 1992). These results indicate that the pharmacology of the antidepressant may determine the effect on SST and SST receptors. Understanding the effects of antidepressants on SST may help to uncover whether changes in SST contribute to the pharmaceutical efficacy of antidepressants.
Schizophrenia (Pre-clinical and Clinical Evidence)
SST has been well studied for its role in schizophrenia and behavior related to schizophrenia in clinical and pre-clinical studies. Multiple studies have investigated the expression of SST in schizophrenic subjects (post-mortem tissue). DNA microarray for expression of GABA-related transcripts in the dorsolateral PFC of schizophrenic subjects (post mortem tissue) and matched controls revealed a robust decrease in SST mRNA in subjects with schizophrenia (Hashimoto et al., 2008a). The difference in expression of SST from healthy controls was greater than all other transcripts analyzed including NPY, GAD67, and GABA receptor subunits. This reduction in SST was further validated using Real-time qPCR and in situ hybridization and was replicated in multiple different studies in human subjects using qPCR (Hashimoto et al., 2008b; Fung et al., 2014; Tsubomoto et al., 2019). SST mRNA was found to be reduced (through in situ hybridization histochemistry and qPCR) in the orbitofrontal cortex in subjects with schizophrenia (also post mortem tissue; Joshi et al., 2015). A subsequent study in schizophrenic subjects (post mortem tissue) found that decreased SST expression in the dorsolateral PFC in schizophrenia is confined to layers 2 through 6, and both the density of SST+ neurons and the expression of SST mRNA per neuron were reduced (Morris et al., 2008). Collectively, these studies demonstrate substantial evidence in support of decreased SST in the PFC in patients with diagnosed schizophrenia.
The levels of SST mRNA were not altered in the dorsolateral PFC of monkeys chronically exposed to antipsychotic medications (Hashimoto et al., 2008b), suggesting that administration of antipsychotics as a treatment itself is not the cause of the reduced SST mRNA in schizophrenia. Moreover Rats given a single administration of haloperidol resulted in either unchanged or increased SST mRNA in the PFC further supporting the hypothesis that the decrease in SST mRNA observed in the PFC of human schizophrenic subjects reflects the disease process and is not a byproduct of antipsychotic treatment (Sakai et al., 1995).
Differential expression of SST receptors is also seen in individuals with schizophrenia. Subjects (post mortem tissue) with schizophrenia exhibited unchanged SST-R1 but significantly decreased SST-R2 mRNA in the dorsolateral PFC, and this reduction was localized to pyramidal cells in layers 5–6 (Beneyto et al., 2012). SST-R2 expression was not affected in macaque monkeys exposed to chronically high doses of antipsychotics, or in patients on or off antipsychotics at the time of death; however, macaque monkeys exposed to low doses of the antipsychotic haloperidol (a common antipsychotic for the treatment of schizophrenia) demonstrated reduced SST-R2, suggesting the results should be interpreted cautiously (Beneyto et al., 2012).
Animal studies are providing insight into the relationship between decreased PFC SST transmission and behavioral processes disrupted in schizophrenia. A recent study used viral gene knockdown to determine the behavioral effects of SST in the PFC (Perez et al., 2019). Perez et al. (2019) found that male and female Sprague–Dawley rats which underwent viral-mediated gene knockdown of SST in the PFC exhibit behavioral deficits in the negative (social interaction test) and cognitive (reversal learning test) domains consistent with those observed in schizophrenia. In two different rodent models of schizophrenia, the MK-801 model in Long Evans rats (Murueta-Goyena et al., 2020) and the BRINP1-KO model in mice (Kobayashi et al., 2018), the number of SST+ immunoreactive neurons is decreased, although this does not necessarily represent a change in the SST peptide.
The deficits in SST and SST+ neurons observed in subjects with schizophrenia and animal models of schizophrenia may be a downstream consequence of impaired BDNF signaling. Consistent with this hypothesis, strong positive correlations between BDNF protein levels and SST mRNA levels were observed in the PFC of human subjects (post mortem tissue) with schizophrenia, suggesting that BDNF may function to regulate SST expression in the PFC (Mellios et al., 2009). This parallels the findings suggesting an interaction between BDNF and SST in MDD. SST has also been shown to be regulated by BDNF through the tyrosine receptor kinase B (trkB) receptor as evidenced by reduced expression of SST in the PFC of trkB hypomorphic mice, which express significantly lower levels of trkB (Morris et al., 2008). These studies support the hypothesis that BDNF underlies changes in SST in the PFC, and may precede changes in SST, though more work is needed to understand this relationship.
Dynorphin
Dynorphin Signaling and Overall Peptide Actions
Dynorphin, an endogenous member of the opioid neuropeptide family (Goldstein et al., 1979), is thought to mediate negative emotional states associated with stress, depression, and drug use withdrawal (Koob and Le Moal, 2008; Bruchas et al., 2010; Knoll and Carlezon, 2010; Hang et al., 2015). Dynorphin refers to a group of neuropeptides derived from the preprodynorphin gene including Dynorphin-A 32 amino acids (Fischli et al., 1982), which binds with high affinity to kappa opioid receptors (KORs; Chavkin et al., 1982; James et al., 1982; Kakidani et al., 1982; Hauser et al., 2005). Dynorphin and KORs are present throughout the brain and activation of this system generally promotes dysphoria, anxiety-like behavior, and behaviors associated with substance use disorders (Wee and Koob, 2010; Crowley and Kash, 2015).
KORs are G protein-coupled receptors, encoded by the oprk1 gene, which are selectively activated by dynorphin (Karkhanis et al., 2017). KORs can signal via multiple signaling pathways including G-i/o protein-coupled inhibition of adenylyl cyclase (Konkoy and Childers, 1993; Lawrence et al., 1995; Dhawan et al., 1996; Karkhanis et al., 2017), stimulation of inwardly rectifying potassium channels (Henry et al., 1995), activation of p38 MAPK (Bruchas et al., 2006), and activation of ERK 1/2 (McLennan et al., 2008). The effects of dynorphin/KORs on neurotransmission are variable and depend on the brain region, neuron the receptor is on, and whether the receptor is expressed pre- or post- synaptically (Karkhanis et al., 2017). In other regions of the cortex, dynorphin has been shown to act presynaptically to inhibit the release of both GABA and glutamate in the same brain region (Li et al., 2012; Crowley et al., 2016). The dynorphin/KOR system is a critical mediator of both stress response and stress-induced relapse and has been linked with the CRF system (Bruchas et al., 2010). Stress-induced CRF activation leads to dynorphin release and subsequent modulation of mood by KOR activation.
Both Dynorphin and KORs are highly evolutionarily conserved and are present in the PFC in both humans and rodents (Zamir et al., 1984a,b; Dawbarn et al., 1986; McIntosh et al., 1987; Wevers et al., 1995; Hurd, 1996; Mansour et al., 1996; Svingos and Colago, 2002). KORs predominate over other types of opioid receptors such as mu-opioid receptors (MORs) in the PFC (Lahti et al., 1989). Dynorphin neurons comprise a subset of neurons in the PFC which express pre-prodynorphin and are GABAergic (Sohn et al., 2014). Approximately one-quarter of dynorphin neurons also express the neuropeptide somatostatin (Sohn et al., 2014).
Recent studies have revealed an important neuromodulatory role for the dynorphin/KOR system in the PFC. KORs in the mPFC is thought to be largely presynaptic and are localized on axons and axon terminals (Svingos and Colago, 2002). Presynaptic KORs can regulate synaptic input from other regions onto the PFC, and activation of KORs in the PFC has been shown to negatively regulate glutamatergic synaptic transmission from the BLA (Tejeda et al., 2015). Also, Tejeda et al. (2015) demonstrated that activation of KORs in the PFC decreases the frequency of miniature EPSCs onto layer 5 pyramidal cells. This work also demonstrated that activation of KORs in the PFC also directly inhibits dopamine terminals to reduce dopamine release in the PFC. Currently, Dynorphin/KOR activation is known to reduce dopamine release and dampen glutamatergic input onto pyramidal cells in the PFC, though other effects in the PFC (both on other cell populations and other neurotransmitters and peptides) have yet to be fully elucidated.
Stress and Anxiety (Pre-clinical Evidence)
KOR mRNA and protein are affected by stress. Repeated forced swim stress in male C57BL/6 resulted in increased expression of KOR mRNA (Flaisher-Grinberg et al., 2012) and a separate study found an increase in KOR protein in male Swiss mice exposed to repeated forced swim stress (Rosa et al., 2018a). Together these results suggest that stress, specifically forced swim stress, leads to increased KOR expression. It is unknown how stress affects dynorphin expression in the PFC and future studies are needed to test this.
Dynorphin is thought to mediate dysphoria, and broadly, promote behaviors associated with anxiety. Pre-clinical rodent models indicate that the behavioral effect of dynorphin in the PFC may depend on the region of activation. Mice subjected to chronic constriction injury of the right sciatic nerve exhibit significantly increased pro-dynorphin and KOR mRNA expression in the PFC (Palmisano et al., 2019). Studies investigating the effects of dynorphin agonists and antagonists on behaviors associated with anxiety reveal a subregion specific effect of KOR activation. Microinjections of a KOR agonist (U50, 488H) or a dynorphin derivative (E-2078) into the mPFC in rats led to place aversion in the conditioned place preference paradigm suggesting that activation of KOR is associated with aversive effects in the mPFC (Bals-Kubik et al., 1993). Consistent with this, another study using the selective KOR antagonist nor-binaltorphamine (norBNI) in male Long-Evans rats found intra-mPFC injection increased center time in the open field test, suggesting decreased defense/withdraw anxiety (Tejeda et al., 2015). Together, these findings point towards a role for dynorphin and KOR activation in the mPFC in mediating aversive states and behaviors associated with anxiety.
However, these studies did not investigate the effect in specific subregions of the mPFC. Male CD-1 mice injected with the selective KOR agonist U-69, 593 in the infralimbic cortex exhibited dose-dependent decreases in avoidance behaviors in the EPM, and defensive/withdrawal anxiety in the open field (Wall and Messier, 2000). They also exhibited evidence for enhanced memory in two separate memory tests: the EPM transfer-latency test and the Y-maze test. A subsequent study by Wall and Messier (2000) investigated the effect of blocking KOR activation selectively in the infralimbic cortex using the KOR antagonist norBNI. They found that pretreatment with one injection of norBNI in the infralimbic cortex dose-dependently increased anxiety-like behavior as well as disrupted working memory in the same behavioral tasks. Importantly, the effect on anxiety-like behavior after infusion of either a KOR agonist or antagonist in the infralimbic cortex was long-lasting, and differences in EPM behavior were observed in both studies after a 24-h delay (Wall and Messier, 2000). This finding is inconsistent with the effect of KOR observed by other researchers in the mPFC, but this discrepancy may be due to the region of injection, and the effect observed by Wall and Messier may be specific to the infralimbic subregion of the PFC, which has been shown to play opposing roles to more dorsal regions of the PFC such as the prelimbic cortex in other neuropeptides. More work is needed to understand the region-specific behavioral effect of KOR activation/inhibition in different subregions of the PFC.
Substance Use Disorder (Pre-clinical and Clinical Evidence)
Human studies point toward a dysregulation of dynorphin/KOR systems in subjects with alcohol or substance use disorders. Prodynorphin CpG dinucleotides that overlap with SNPs were differentially methylated in the dlPFC of postmortem brains from alcohol-dependent individuals (Taqi et al., 2011) suggesting a possible role for dynorphin in behaviors associated with substance dependency. One study found prodynorphin and dynorphin (both A and B) mRNA were upregulated in the dlPFC of alcoholics (Bazov et al., 2013). A second study by Bazov et al. (2018) found prodynorphin is downregulated in the PFC of alcoholics while KOR expression itself was unchanged. The authors of both studies hypothesize that the different findings are likely because the first study was underpowered, while the second study from 55 control and 53 alcoholic subjects provided a more sufficient dataset (Bazov et al., 2018). Post mortem brains from individuals with a history of marijuana use or stimulant use had increased prodynorphin mRNA in the anterior cingulate and dorsolateral prefrontal cortices respectively, but no change was found in the brains of individuals with a history of alcohol use (Peckys and Hurd, 2001). The human findings point towards dysregulation of prodynorphin with substance use, but the directionality of this effect may depend on the specific pharmacology and consumption pattern of the drug of abuse, as well as severity of abuse.
Alcohol has been shown to regulate dynorphin in rodents. Alcohol increases the density of dynorphin expressing-cells in the mPFC in rats consuming ethanol chronically compared to water controls, as assessed by digoxigenin-labeled in situ hybridization histochemistry (Chang et al., 2010). Male Sprague–Dawley rats treated with alcohol at a dose of 1.5 g/kg three times for 1 day exhibited increased prodynorphin in the PFC, however, no change was detected after 5 days (D’Addario et al., 2013). Prenatal alcohol exposure can lead to increased prodynorphin mRNA in the PFC in infant rats (age not further specified; Wille-Bille et al., 2018). Like other drug use, alcohol use also causes increased expression of prodynorphin. Also, directly modulating KORs has been shown to regulate drug reward. In Male Sprague–Dawley rats which received once-daily injections of cocaine (20.0 mg/kg, intraperitoneal) for 5 days, site-specific activation of mPFC KORs exacerbated the development of behavioral sensitization and increased cocaine-evoked dopamine levels (Chefer et al., 1999). More work is needed to determine how KOR activation in the PFC influences drug reward and behaviors associated with substance use disorders.
Consistent with the human literature, pre-clinical animal studies also indicate altered dynorphin/KOR following the administration of substances of abuse other than alcohol. Acute (8 mg/kg, intraperitoneal) administration of 3,4-methylenedioxy-N-methylamphetamine (ecstasy) in male Sprague–Dawley rats raised levels of prodynorphin mRNA in the PFC and decreased levels of Dynorphin-A (Di Benedetto et al., 2006). Interestingly, Di Benedetto et al. (2006) observed no change after chronic treatment. Male Wistar rats treated with both acute and chronic morphine (8.0 mg/kg intraperitoneal, once daily for one or five consecutive days) exhibited increased KOR mRNA in the PFC (Yu et al., 2012). However, at the protein level, acute morphine treatment did not affect KORs in the PFC, while chronic morphine caused downregulated KOR protein (Yu et al., 2014). Nicotine has also been shown to lead to changes in prodynorphin expression (Carboni et al., 2016). Chronic and sub-chronic administration of nicotine led to increased expression of prodynorphin mRNA in the PFC of Sprague–Dawley rats. However, this was not observed after acute administration, and no change was found in KOR mRNA with either administration paradigm. In summary, acute ecstasy, acute and chronic morphine, and sub-chronic and chronic administration of nicotine all led to increased expression of prodynorphin. More research is needed to uncover how KOR’s and dynorphin itself is altered with drug use.
Importantly, prodynorphin and KOR mRNA were found to be unchanged in the anterior cingulate and dorsolateral prefrontal cortices of post mortem brains of subjects diagnosed with schizophrenia, bipolar disorder, or major depression, and was not associated with antipsychotic treatment or suicide (Peckys and Hurd, 2001). This points towards dynorphin having a particular sensitivity to stress and/or drugs of abuse and maybe a central part of the addiction process. Based on the postulated role of the dynorphin/KOR system in mediating negative affect (such as withdrawal from drugs of abuse), more work is needed to further characterize the role of this system in the PFC concerning substance use disorders.
Endorphin and Enkephalin
Endorphin and Enkephalin Signaling and Overall Peptide Actions
Endorphin and enkephalin, like dynorphin, are endogenous opioid neuropeptides that are present in the CNS (Rossier, 1988). Despite their similar behavioral effects, and pharmacological action, they are derived from distinct precursors. Endorphins (canonically β-endorphins; Bruijnzeel, 2009) are derived from pro-opiomelanocortin, and enkephalins (met-enkephalin and leu-enkephalin) are derived from proenkephalin (Rossier, 1988). Endorphins and enkephalins play a role in motivational and stress circuits and have been implicated in neuroadaptations to drug abuse (Koob and Volkow, 2016). Both endorphin and enkephalin have profound pain-relieving effects and promote euphoria (Shenoy and Lui, 2018; Hicks et al., 2019).
Endorphins, enkephalins, and their precursors are highly evolutionarily conserved and are expressed in the PFC in several species including humans and rodents (Matthews et al., 1992; Hurd, 1996; Leriche et al., 2007). To date, few studies have directly examined the behavioral or physiological function of these peptides in the PFC and instead have focused on their corresponding receptors. Endorphins and enkephalins modulate neural activity through activation of G protein-coupled (GPCR) opioid receptors (Corder et al., 2018) which are also present in the PFC (Lahti et al., 1989). Endorphins preferentially bind and activate mu–opioid GPCRs (MORs), while enkephalins are non-selective agonists with an affinity for both MORs and delta-opioid GPCRs (DORs; Corder et al., 2018). DORs have been shown to regulate anxiety-like behavior, and site-specific activation of DORs in the PFC in mice reduced anxiety-like behavior (Lahti et al., 1989). Despite this early finding, few studies have investigated DORs in the PFC, and instead, most studies have focused on the MORs, due to their more well-known neuromodulatory and behavioral effects. Future studies into the role of DORs in the PFC as well as studies examining the pharmacological effects of endorphins and enkephalins in the PFC are warranted.
MORs are expressed predominately on non-pyramidal GABAergic neurons in the frontal cortex, overlapping with enkephalin expression (Taki et al., 2000; Férézou et al., 2007). This suggests that MORs function as auto-receptors on enkephalin-expressing non-pyramidal neurons. In the PFC, activation of MORs has been shown to inhibit voltage-dependent sodium currents on non-pyramidal neurons through a PKA and PKC dependent mechanism (Witkowski and Szulczyk, 2006), an effect likely mediated by auto-receptors on non-pyramidal GABAergic enkephalin neurons. Reduced voltage-dependent sodium currents would result in decreased action potentials in GABAergic cells in the PFC, and then downstream would cause decreased inhibitory currents onto pyramidal cells. Consistent with this hypothesis, MOR agonists in other regions of the cortex have been shown to decrease GABAergic transmission and decrease inhibitory currents onto pyramidal cells (Férézou et al., 2007) and hippocampal cells (Capogna et al., 1993). These findings indicate that if this effect is consistent in the PFC, MORs may cause disinhibition of pyramidal cells whereby activation of MORs on GABAergic neurons increases pyramidal cell activity by decreasing GABAergic input onto these cells.
In addition to local disinhibition of pyramidal cells, MORs in the PFC regulates excitatory input from other regions projecting to the PFC, such as presynaptic suppression of glutamate release from the thalamus (Marek and Aghajanian, 1998; Marek et al., 2001). Importantly, MORs and DORs have been shown to synergistically interact to enhance dopamine D1 receptor-induced stimulation of adenylyl cyclase activity (Olianas et al., 2012). MORs and DORs in conjunction with their endogenous ligands are positioned to precisely coordinate neural activity both within the PFC and from other brain regions. As a result, dysregulation of this system can contribute to loss of inhibitory control over executive function and behavior as is observed in multiple psychiatric disorders (Baldo, 2016).
Stress and Anxiety (Pre-clinical Evidence)
Preclinical animal models suggest that stress exposure is linked to reduced expression of enkephalin in the PFC. Male Wistar Han rats that underwent peripubertal stress conditions (exposure to fox odor and elevated platform across post-natal days 28–42) exhibited downregulated enkephalin mRNA in the PFC (Li et al., 2018). Ninety minutes of cold and immobilizing stress in male Wistar rats resulted in significantly decreased enkephalin immunoreactivity, however, no change was observed after 30 or 180 min (Kurumaji et al., 1987). The decreased enkephalin observed only at the 90-min time point may indicate that enkephalin plays a role in adaptation to stress.
Stress has also been shown to increase MORs and decrease DORs in the PFC. Adult Swiss mice subjected to social defeat stress exhibited increased MOR protein and decreased DOR protein in the PFC in susceptible mice (Rosa et al., 2018b). Similarly, swiss mice subjected to repeated forced swim stress exhibited increased MOR expression but reduced DOR in the PFC (Rosa et al., 2018a). Neonatal handling, which is known to increase the ability to cope with stress and to decrease anxiety-like behavior is associated with increased levels of MORs in the PFC (Kiosterakis et al., 2009). This indicates that stress may lead to higher expression of MORs and decreased expression of DORs in the PFC. The increased MOR expression observed in the PFC in response to stress may be a neuroadaptation to decreased MOR ligands such as decreased enkephalin. Future work is needed to uncover the relationship between enkephalin and MORs/DORs as well as their relationship with stress.
Substance Use Disorder (Pre-clinical and Clinical Evidence)
The rewarding effects of drugs and the development of drug-seeking behavior involve changes in opioid peptides (Koob and Volkow, 2016). The current review will not cover the breadth of focus and attention on the modern opioid epidemic, covered in-depth elsewhere (Shipton et al., 2018; Skolnick, 2018; Marshall et al., 2019). Endorphin and enkephalin in the PFC contribute to inhibitory control over appetitive behaviors, and loss of control over these behaviors is known to occur in multiple psychiatric disorders such as substance use disorders (Baldo, 2016). Infusions of MOR agonists into the ventromedial PFC of male Sprague–Dawley rats resulted in increased appetitive motivation (Selleck et al., 2015, 2018). Moreover, male Wistar rats that binge ate a highly palatable diet exhibited increased preopiomelanocortin (endorphin precursor) in the PFC (Blasio et al., 2014). These findings point towards a role for endorphin and MORs in the regulation of consummatory behavior, and thus this system may contribute to excessive drug use and behaviors observed in substance use disorders.
Alcohol and nicotine have thus far not been found to affect endorphin or enkephalin in the PFC. Post mortem brains from human alcoholics show no changes in the levels of proenkephalin, MORs, or DORs in the PFC (Bazov et al., 2013). Nicotine treatment leads to changes in endorphin and its precursor (proopiomelanocortin) in limbic regions in mice; however, only moderate decreases in proopiomelanocortin have been observed in the PFC following chronic nicotine treatment (Gudehithlu et al., 2012). Other drugs, however, such as psychostimulants, have been shown to modulate enkephalin. Male Sprague–Dawley rats administered with amphetamine and then subsequently administered with the same dose 7 days later exhibited increased proenkephalin mRNA (Morales-Mulia et al., 2007). These findings indicate that endorphin and enkephalin are likely not directly regulated by alcohol or nicotine but may be regulated by other drugs such as amphetamines.
Also, substance use has been shown to regulate MOR functioning and this may contribute to behaviors involved with substance use disorders. In humans, long-term opiate and mixed opiate/cocaine abusers exhibit decreased midazoline receptor antisera-selected (IRAS)/nischarin, a putative I1-imidazoline receptor which regulates MOR trafficking (Keller et al., 2017). AA rats bred selectively for high alcohol consumption have a significantly greater proenkephalin mRNA and greater density of MORs in the PFC (Marinelli et al., 2000) which may contribute to increased ethanol consumption, although the connection is indirect. Rats self-administering a cannabinoid receptor agonist exhibited increased MOR levels in the PFC (Fattore et al., 2007). Taken together, these results suggest endorphin/enkephalin and MORs are differentially affected by drugs of abuse, and increases in this system may be involved with specific types of substance use. Pre-clinical studies using a consistent methodology to investigate the effects of multiple different drugs of abuse on the endorphin/enkephalin system would provide a foundation for understanding how the pharmacology of the substance may contribute to the pathology of substance use disorders. Further, clinical investigations into the expression of endorphin/enkephalin in patients with various forms of substance use disorders would help to determine how these systems are differentially affected by different substances.
Discussion
The PFC has been shown in both the clinical and pre-clinical literature to play a vital role in many neuropsychiatric disorders. Increasing evidence has shown that GABAergic and peptidergic neurons within the PFC, responsible for the modulation of glutamatergic inputs, local circuits, and pyramidal neuron outputs, play a key modulatory role in these disorders. More work is needed to understand the role of each of these individual peptide populations. Peptide effects vary depending on the model (e.g., acute stress vs. chronic stress models, length of manipulation, animal genetic strain, and sex differences). Also, further research should address interactions between peptide populations in the PFC—both those expressed in different and in the same, GABAerigc neurons. For instance, subpopulations of neurons within the PFC express both NPY and SST, while SST and dynorphin have also been found to co-express here. This overlap is surprising given the general opposing roles of NPY and dynorphin. Also, increasing technological advancements have allowed for the greater investigation of peptide release independent of co-expressed neurotransmitters, which will allow a greater understanding of when same-neuron peptides are released (i.e., under different neuronal firing frequencies or overall length of firing). Greater assessment of the combinatorial role of these peptides will better inform disease models and treatment. Overall, the literature suggests a strong and important role of peptides in the PFC in stress and neuropsychiatric disorders.
Future work, in addition to expanding the existing depth of literature, also needs to focus on filling the gap in the literature. Specifically, much of the pre-clinical work above has been conducted in male rodents, with very little focus on females. Others have eloquently expressed elsewhere (Shansky, 2018, 2019, 2020) the importance of: (a) investigating females as a stand-alone research question, as opposed to only in contrast to males as a baseline; and (b) the unlikeliness that female rodents have more behavioral and neurobiological variability, or that this variability is due to female-dominant sex hormones. Also, standard operating procedures may help to rectify some of the discrepancies seen. For instance, the multitude of stress models, with differing lengths of stress exposure and post-stress recovery periods, likely contributes to the variable effects seen.
Moreover, while pre-clinical evidence links neuropeptide systems to behaviors relevant to a disorder, these diseases themselves are incredibly complex. For example, the DSM-5 [American Psychological Association (APA) (2013)] includes five subtypes of depressive disorders (disruptive mood dysregulation disorder, MDD, persistent depressive disorder, premenstrual dysphoric disorder, substance/medication-induced depressive disorder, depressive disorder due to another medical condition, other specified depressive disorder, and unspecified depressive disorder). Animal models of depression understandably fail to capture this complexity, and importantly, often rely only on stress (whether social or environmental stress) and/or genetic manipulations (Krishnan and Nestler, 2011). This example holds for most neuropsychiatric disorders, in that animal models representing a single behavioral or biological manipulation are unlikely to fully recapitulate the complexity and range of the human disorder. Therefore, it is no surprise that animal models using a signal manipulation (i.e., forced swim) do not replicate the work of these nuanced psychiatric conditions in humans. Leading researchers in the preclinical field have agreed that animal models thus far are not fulling capturing human disorders but are nevertheless a crucial component of treatment identification and testing. These researchers identified key gaps in the literature needed for the synthesis of human and animal work: “in vivo experiments, precision, innovation, integration and complexity, and leadership setting the tone” (Bale et al., 2019). In this article, Bale et al. make a key and important statement supporting our overall characterization of peptides in the PFC, that this preclinical work does not directly model these disorders but, “Rather, the goal is to achieve a better understanding of an essential biological function that is key to the illness and to ensure that our level of understanding is actionable for translation.” Therefore, the discrepancies seen in the preclinical and clinical work for some neuropeptides provide a launching point for further investigation in humans.
Overall, though some discrepancies exist in the role of specific neuropeptides in specific disorders, their importance is clear and further investigation for each of them is paramount to understanding complex disorders in humans, and for targeting both preventions and interventions. By both filling in these gaps (investigating both sexes, consistency in models, further expanding research into humans), and expanding the focus into new questions and domains overall, a greater understanding of the role of peptidergic signaling in the PFC during stress and neuropsychiatric diseases may be obtained.
Author Contributions
DB and NC wrote the manuscript. All authors contributed to the article and approved the submitted version.
Funding
The experiments were funded by the Brain and Behavior Research Foundation (NARSAD Young Investigator Award; NC), The National Institute on Alcohol Abuse and Alcoholism (R21AA028008; NC), and the Penn State’s Social Science Research Institute (Level 2 Award; NC).
Conflict of Interest
The authors declare that the research was conducted in the absence of any commercial or financial relationships that could be construed as a potential conflict of interest.
Acknowledgments
We would like to thank Malini Suresh-Nair (Crowley Lab, Department of Biology) for thoughtful comments.
References
Adrian, T. E., Allen, J. M., Bloom, S. R., Ghatei, M. A., Rossor, M. N., Roberts, G. W., et al. (1983). Neuropeptide Y distribution in human brain. Nature 306, 584–586. doi: 10.1038/306584a0
Al-Hasani, R., McCall, J. G., Shin, G., Gomez, A. M., Schmitz, G. P., Bernardi, J. M., et al. (2015). Distinct subpopulations of nucleus accumbens dynorphin neurons drive aversion and reward. Neuron 87, 1063–1077. doi: 10.1016/j.neuron.2015.08.019
Al-Hasani, R., Wong, J. M. T., Mabrouk, O. S., McCall, J. G., Schmitz, G. P., Porter-Stransky, K. A., et al. (2018). in vivo detection of optically-evoked opioid peptide release. Elife 7:e36520. doi: 10.7554/elife.36520
Allen, Y. S., Adrian, T. E., Allen, J. M., Tatemoto, K., Crow, T. J., Bloom, S. R., et al. (1983). Neuropeptide Y distribution in the rat brain. Science 221, 877–879. doi: 10.1126/science.6136091
American Psychological Association (APA). (2013). Diagnostic and Statistical Manual of Mental Disorders: Depressive Disorders, Diagnostic and Statistical Manual of Mental Disorders. Washington, DC: American Psychiatric Publishing, Inc.
Arnsten, A. F. T. (2009). Stress signalling pathways that impair prefrontal cortex structure and function. Nat. Rev. Neurosci. 10, 410–422. doi: 10.1038/nrn2648
Ault, D. T., and Werling, L. L. (1998). Neuropeptide Y-mediated enhancement of NMDA-stimulated [3H]dopamine release from rat prefrontal cortex is reversed by σ1 receptor antagonists. Schizophr. Res. 31, 27–36. doi: 10.1016/s0920-9964(98)00002-4
Baldo, B. A. (2016). Prefrontal cortical opioids and dysregulated motivation: a network hypothesis. Trends Neurosci. 39, 366–377. doi: 10.1016/j.tins.2016.03.004
Bale, T. L., Abel, T., Akil, H., Carlezon, W. A., Moghaddam, B., Nestler, E. J., et al. (2019). The critical importance of basic animal research for neuropsychiatric disorders. Neuropsychopharmacology 44, 1349–1353. doi: 10.1038/s41386-019-0405-9
Bale, T. L., and Vale, W. W. (2004). CRF and CRF receptors: role in stress responsivity and other behaviors. Annu. Rev. Pharmacol. Toxicol. 44, 525–557. doi: 10.1146/annurev.pharmtox.44.101802.121410
Bals-Kubik, R., Ableitner, A., Herz, A., and Shippenberg, T. S. (1993). Neuroanatomical sites mediating the motivational effects of opioids as mapped by the conditioned place preference paradigm in rats. J. Pharmacol. Exp. Ther. 264, 489–495.
Banasr, M., Lepack, A., Fee, C., Duric, V., Maldonado-Aviles, J., DiLeone, R., et al. (2017). Characterization of GABAergic marker expression in the chronic unpredictable stress model of depression. Chronic Stress 1:247054701772045. doi: 10.1177/2470547017720459
Bazov, I., Kononenko, O., Watanabe, H., Kuntiä, V., Sarkisyan, D., Taqi, M. M., et al. (2013). The endogenous opioid system in human alcoholics: molecular adaptations in brain areas involved in cognitive control of addiction. Addict. Biol. 18, 161–169. doi: 10.1111/j.1369-1600.2011.00366.x
Bazov, I., Sarkisyan, D., Kononenko, O., Watanabe, H., Karpyak, V. M., Yakovleva, T., et al. (2018). Downregulation of the neuronal opioid gene expression concomitantly with neuronal decline in dorsolateral prefrontal cortex of human alcoholics. Transl. Psychiatry 8:122. doi: 10.1038/s41398-017-0075-5
Benali, N., Ferjoux, G., Puente, E., Buscail, L., and Susini, C. (2000). Somatostatin receptors. Digestion 62, 27–32. doi: 10.1159/000051852
Beneyto, M., Morris, H. M., Rovensky, K. C., and Lewis, D. A. (2012). Lamina- and cell-specific alterations in cortical somatostatin receptor 2 mRNA expression in schizophrenia. Neuropharmacology 62, 1598–1605. doi: 10.1016/j.neuropharm.2010.12.029
Benoit, R., Esch, F., Bennett, H. P. J., Ling, N., Ravazzola, M., Orci, L., et al. (1990). Processing of prosomatostatin. Metabolism 39, 22–25. doi: 10.1016/0026-0495(90)90202-n
Berglund, M. M., Hipskind, P. A., and Gehlert, D. R. (2003). Recent developments in our understanding of the physiological role of PP-fold peptide receptor subtypes. Exp. Biol. Med. 228, 217–244. doi: 10.1177/153537020322800301
Blasio, A., Steardo, L., Sabino, V., and Cottone, P. (2014). Opioid system in the medial prefrontal cortex mediates binge-like eating. Addict. Biol. 19, 652–662. doi: 10.1111/adb.12033
Bloodgood, D. W., Sugam, J. A., Holmes, A., and Kash, T. L. (2018). Fear extinction requires infralimbic cortex projections to the basolateral amygdala. Transl. Psychiatry 8:60. doi: 10.1038/s41398-018-0106-x
Bogart, L. J., and O’Donnell, P. (2018). Multiple long-range inputs evoke NMDA currents in prefrontal cortex fast-spiking interneurons. Neuropsychopharmacology 43, 2101–2108. doi: 10.1038/s41386-018-0029-5
Boutros, N., Der-Avakian, A., Semenova, S., Lee, S., and Markou, A. (2016). Risky choice and brain CRF after adolescent ethanol vapor exposure and social stress in adulthood. Behav. Brain Res. 311, 160–166. doi: 10.1016/j.bbr.2016.05.038
Brazeau, P., Vale, W., Burgus, R., Ling, N., Butcher, M., Rivier, J., et al. (1973). Hypothalamic polypeptide that inhibits the secretion of immunoreactive pituitary growth hormone. Science 179, 77–79. doi: 10.1126/science.179.4068.77
Britt, J. P., Benaliouad, F., McDevitt, R. A., Stuber, G. D., Wise, R. A., and Bonci, A. (2012). Synaptic and behavioral profile of multiple glutamatergic inputs to the nucleus accumbens. Neuron 76, 790–803. doi: 10.1016/j.neuron.2012.09.040
Brown, M., and Taché, Y. (1981). Hypothalamic peptides: central nervous system control of visceral functions. Fed. Proc. 40, 2565–2569.
Bruchas, M. R., Land, B. B., and Chavkin, C. (2010). The dynorphin/kappa opioid system as a modulator of stress-induced and pro-addictive behaviors. Brain Res. 1314, 44–55. doi: 10.1016/j.brainres.2009.08.062
Bruchas, M. R., Macey, T. A., Lowe, J. D., and Chavkin, C. (2006). Kappa opioid receptor activation of p38 MAPK is GRK3- and arrestin-dependent in neurons and astrocytes. J. Biol. Chem. 281, 18081–18089. doi: 10.1074/jbc.m513640200
Bruijnzeel, A. W. (2009). kappa-Opioid receptor signaling and brain reward function. Brain Res. Rev. 62, 127–146. doi: 10.1016/j.brainresrev.2009.09.008
Caberlotto, L., and Hurd, Y. L. (1999). Reduced neuropeptide Y mRNA expression in the prefrontal cortex of subjects with bipolar disorder. Neuroreport 10, 1747–1750. doi: 10.1097/00001756-199906030-00022
Caberlotto, L., and Hurd, Y. L. (2001). Neuropeptide Y Y1 and Y2 receptor mRNA expression in the prefrontal cortex of psychiatric subjects: relationship of Y2 subtype to suicidal behavior. Neuropsychopharmacology 25, 91–97. doi: 10.1016/s0893-133x(00)00231-1
Capogna, M., Gähwiler, B. H., and Thompson, S. M. (1993). Mechanism of mu-opioid receptor-mediated presynaptic inhibition in the rat hippocampus in vitro. J. Physiol. 470, 539–558. doi: 10.1113/jphysiol.1993.sp019874
Carboni, L., Romoli, B., Bate, S. T., Romualdi, P., and Zoli, M. (2018). Increased expression of CRF and CRF-receptors in dorsal striatum, hippocampus and prefrontal cortex after the development of nicotine sensitization in rats. Drug Alcohol Depend. 189, 12–20. doi: 10.1016/j.drugalcdep.2018.04.027
Carboni, L., Romoli, B., Romualdi, P., and Zoli, M. (2016). Repeated nicotine exposure modulates prodynorphin and pronociceptin levels in the reward pathway. Drug Alcohol Depend. 166, 150–158. doi: 10.1016/j.drugalcdep.2016.07.002
Carlén, M. (2017). What constitutes the prefrontal cortex? Science 358, 478–482. doi: 10.1126/science.aan8868
Cénat, J. M., McIntee, S. E., and Blais-Rochette, C. (2020). Symptoms of posttraumatic stress disorder, depression, anxiety and other mental health problems following the 2010 earthquake in Haiti: a systematic review and meta-analysis. J. Affect. Disord. 273, 55–85. doi: 10.1016/j.jad.2020.04.046
Chang, G. Q., Barson, J. R., Karatayev, O., Chang, S. Y., Chen, Y. W., and Leibowitz, S. F. (2010). Effect of chronic ethanol on enkephalin in the hypothalamus and extra-hypothalamic areas. Alcohol. Clin. Exp. Res. 34, 761–770. doi: 10.1111/j.1530-0277.2010.01148.x
Chan-Palay, V., Allen, Y. S., Lang, W., Haesler, U., and Polak, J. M. (1985). I. Cytology and distribution in normal human cerebral cortex of neurons immunoreactive with antisera against neuropeptide Y. J. Comp. Neurol. 238, 382–389. doi: 10.1002/cne.902380403
Chavkin, C., James, I. F., and Goldstein, A. (1982). Dynorphin is a specific endogenous ligand of the κ opioid receptor. Science 215, 413–415. doi: 10.1016/0304-3959(82)90120-8
Chefer, V., Thompson, A. C., and Shippenberg, T. S. (1999). Modulation of cocaine-induced sensitization by κ-opioid receptor agonists. Role of the nucleus accumbens and medial prefrontal cortex. Ann. N Y Acad. Sci. 877, 803–806. doi: 10.1111/j.1749-6632.1999.tb09327.x
Chen, P., Lou, S., Huang, Z.-H., Wang, Z., Shan, Q.-H., Wang, Y., et al. (2020). Prefrontal cortex corticotropin-releasing factor neurons control behavioral style selection under challenging situations. Neuron 106, 301.e7–315.e7. doi: 10.1016/j.neuron.2020.01.033
Chen, J. X., Tang, Y. T., and Yang, J. X. (2008). Changes of glucocorticoid receptor and levels of CRF mRNA, POMC mRNA in brain of chronic immobilization stress rats. Cell. Mol. Neurobiol. 28, 237–244. doi: 10.1007/s10571-007-9170-0
Cohen, H., Liu, T., Kozlovsky, N., Kaplan, Z., Zohar, J., and Mathé, A. A. (2012). The neuropeptide y (NPY)-ergic system is associated with behavioral resilience to stress exposure in an animal model of post-traumatic stress disorder. Neuropsychopharmacology 37, 350–363. doi: 10.1038/npp.2011.230
Commons, K. G., Cholanians, A. B., Babb, J. A., and Ehlinger, D. G. (2017). The rodent forced swim test measures stress-coping strategy, not depression-like behavior. ACS Chem. Neurosci. 8, 955–960. doi: 10.1021/acschemneuro.7b00042
Corder, G., Castro, D. C., Bruchas, M. R., and Scherrer, G. (2018). Endogenous and exogenous opioids in pain. Annu. Rev. Neurosci. 41, 453–473. doi: 10.1146/annurev-neuro-080317-061522
Crowley, N. A., Bloodgood, D. W., Hardaway, J. A., Kendra, A. M., McCall, J. G., Al-Hasani, R., et al. (2016). Dynorphin controls the gain of an amygdalar anxiety circuit. Cell Rep. 14, 2774–2783. doi: 10.1016/j.celrep.2016.02.069
Crowley, N. A., and Kash, T. L. (2015). Kappa opioid receptor signaling in the brain: circuitry and implications for treatment. Prog. Neuropsychopharmacol. Biol. Psychiatry 62, 51–60. doi: 10.1016/j.pnpbp.2015.01.001
Cummings, K. A., and Clem, R. L. (2020). Prefrontal somatostatin interneurons encode fear memory. Nat. Neurosci. 23, 61–74. doi: 10.1038/s41593-019-0552-7
Czéh, B., Vardya, I., Varga, Z., Febbraro, F., Csabai, D., Martis, L. S., et al. (2018). Long-term stress disrupts the structural and functional integrity of GABAergic neuronal networks in the medial prefrontal cortex of rats. Front. Cell. Neurosci. 12:148. doi: 10.3389/fncel.2018.00148
D’Addario, C., Caputi, F. F., Rimondini, R., Gandolfi, O., Del Borrello, E., Candeletti, S., et al. (2013). Different alcohol exposures induce selective alterations on the expression of dynorphin and nociceptin systems related genes in rat brain. Addict. Biol. 18, 425–433. doi: 10.1111/j.1369-1600.2011.00326.x
Dao, N. C., Brockway, D. F., and Crowley, N. A. (2019). in vitro optogenetic characterization of neuropeptide release from prefrontal cortical somatostatin neurons. Neuroscience 419, 1–4. doi: 10.1016/j.neuroscience.2019.08.014
Dautzenberg, F. M., and Hauger, R. L. (2002). The CRF peptide family and their receptors: yet more partners discovered. Trends Pharmacol. Sci. 23, 71–77. doi: 10.1016/s0165-6147(02)01946-6
Dawbarn, D., Zamir, N., Waters, C. M., Hunt, S. P., Emson, P. C., and Brownstein, M. J. (1986). Peptides derived from prodynorphin are decreased in basal ganglia of Huntington’s disease brains. Brain Res. 372, 155–158. doi: 10.1016/0006-8993(86)91469-1
de Souza, E. B., Insel, T. R., Perrin, M. H., Rivier, J., Vale, W. W., and Kuhar, M. J. (1985). Corticotropin-releasing factor receptors are widely distributed within the rat central nervous system: an autoradiographic study. J. Neurosci. 5, 3189–3203. doi: 10.1523/jneurosci.05-12-03189.1985
Dedic, N., Chen, A., and Deussing, J. M. (2017). The CRF family of neuropeptides and their receptors—mediators of the central stress response. Curr. Mol. Pharmacol. 11, 4–31. doi: 10.2174/1874467210666170302104053
Dégenètais, E., Thierry, A. M., Glowinski, J., and Gioanni, Y. (2003). Synaptic influence of hippocampus on pyramidal cells of the rat prefrontal cortex: an in vivo intracellular recording study. Cereb. Cortex 13, 782–792. doi: 10.1093/cercor/13.7.782
Delfs, J. R., and Dichter, M. A. (1983). Effects of somatostatin on mammalian cortical neurons in culture: physiological actions and unusual dose response characteristics. J. Neurosci. 3, 1176–1178. doi: 10.1523/jneurosci.03-06-01176.1983
Dhawan, B., Cesselin, F., Raghubir, R., Reisine, T., Bradley, P., Portoghese, P., et al. (1996). International union of pharmacology. XII. Classification of opioid receptors. Pharmacol. Rev. 48, 567–592.
Di Benedetto, M., D’Addario, C., Candeletti, S., and Romualdi, P. (2006). Chronic and acute effects of 3,4-methylenedioxy-N-methylamphetamine (‘Ecstasy’) administration on the dynorphinergic system in the rat brain. Neuroscience 137, 187–196. doi: 10.1016/j.neuroscience.2005.09.015
Du, X., Serena, K., Hwang, W., Grech, A. M., Wu, Y. W. C., Schroeder, A., et al. (2018). Prefrontal cortical parvalbumin and somatostatin expression and cell density increase during adolescence and are modified by BDNF and sex. Mol. Cell. Neurosci. 88, 177–188. doi: 10.1016/j.mcn.2018.02.001
Eaton, K., Sallee, F., and Sah, R. (2007). Relevance of neuropeptide Y (NPY) in psychiatry. Curr. Top. Med. Chem. 7, 1645–1659. doi: 10.2174/156802607782341037
Ehlers, C. L., Chaplin, R. I., Wall, T. L., Lumeng, L., Li, T. K., Owens, M. J., et al. (1992). Corticotropin releasing factor (CRF): studies in alcohol preferring and non-preferring rats. Psychopharmacology 106, 359–364. doi: 10.1007/bf02245418
Ehlers, C. L., Li, T.-K., Lurneng, L., Hwang, B. H., Somes, C., Jimenez, P., et al. (1998). Neuropeptide Y levels in ethanol-naive alcohol-preferring and nonpreferring rats and in wistar rats after ethanol exposure. Alcohol. Clin. Exp. Res. 22, 1778–1782. doi: 10.1111/j.1530-0277.1998.tb03979.x
Eigler, T., and Ben-Shlomo, A. (2014). Somatostatin system: molecular mechanisms regulating anterior pituitary hormones. J. Mol. Endocrinol. 53, R1–19. doi: 10.1530/jme-14-0034
Faron-Górecka, A., Kuśmider, M., Solich, J., Kolasa, M., Pabian, P., Gruca, P., et al. (2018). Regulation of somatostatin receptor 2 in the context of antidepressant treatment response in chronic mild stress in rat. Psychopharmacology 235, 2137–2149. doi: 10.1007/s00213-018-4912-x
Fattore, L., Viganò, D., Fadda, P., Rubino, T., Fratta, W., and Parolaro, D. (2007). Bidirectional regulation of mu-opioid and CB1-cannabinoid receptor in rats self-administering heroin or WIN 55,212–2. Eur. J. Neurosci. 25, 2191–2200. doi: 10.1111/j.1460-9568.2007.05470.x
Férézou, I., Hill, E. L., Cauli, B., Gibelin, N., Kaneko, T., Rossier, J., et al. (2007). Extensive overlap of mu-opioid and nicotinic sensitivity in cortical interneurons. Cereb. Cortex 17, 1948–1957. doi: 10.1093/cercor/bhl104
Ferguson, B. R., and Gao, W. J. (2015). Development of thalamocortical connections between the mediodorsal thalamus and the prefrontal cortex and its implication in cognition. Front. Hum. Neurosci. 8:1027. doi: 10.3389/fnhum.2014.01027
Fischli, W., Goldstein, A., Hunkapiller, M. W., and Hood, L. E. (1982). Isolation and amino acid sequence analysis of a 4,000-dalton dynorphin from porcine pituitary. Proc. Natl. Acad. Sci. U S A 79, 5435–5437. doi: 10.1073/pnas.79.17.5435
Flaisher-Grinberg, S., Persaud, S. D., Loh, H. H., and Wei, L. N. (2012). Stress-induced epigenetic regulation of κ-opioid receptor gene involves transcription factor c-Myc. Proc. Natl. Acad. Sci. U S A 109, 9167–9172. doi: 10.1073/pnas.1205565109
Fogaça, M. V., and Duman, R. S. (2019). Cortical GABAergic dysfunction in stress and depression: new insights for therapeutic interventions. Front. Cell. Neurosci. 13:87. doi: 10.3389/fncel.2019.00087
Fung, S. J., Fillman, S. G., Webster, M. J., and Shannon Weickert, C. (2014). Schizophrenia and bipolar disorder show both common and distinct changes in cortical interneuron markers. Schizophr. Res. 155, 26–30. doi: 10.1016/j.schres.2014.02.021
Funk, C. K., and Koob, G. F. (2007). A CRF2 agonist administered into the central nucleus of the amygdala decreases ethanol self-administration in ethanol-dependent rats. Brain Res. 1155, 172–178. doi: 10.1016/j.brainres.2007.04.009
Fuster, J. M. (2001). The prefrontal cortex - an update: time is of the essence. Neuron 30, 319–333. doi: 10.1016/s0896-6273(01)00285-9
Fuxe, K., Agnati, L. F., Hafstrand, A., Zini, I., Tatemoto, K., Pich, E. M., et al. (1983). Central administration of neuropeptide Y induces hypotension bradypnea and EEG synchronization in the rat. Acta Physiol. Scand. 118, 189–192. doi: 10.1111/j.1748-1716.1983.tb07261.x
Gabriel, S. M., Davidson, M., Haroutunian, V., Powchik, P., Bierer, L. M., Purohit, D. P., et al. (1996). Neuropeptide deficits in schizophrenia vs. Alzheimer’s disease cerebral cortex. Biol. Psychiatry 39, 82–91. doi: 10.1016/0006-3223(95)00066-6
Gatta, E., Grayson, D. R., Auta, J., Saudagar, V., Dong, E., Chen, Y., et al. (2019). Genome-wide methylation in alcohol use disorder subjects: implications for an epigenetic regulation of the cortico-limbic glucocorticoid receptors (NR3C1). Mol. Psychiatry doi: 10.1038/s41380-019-0449-6 [Epub ahead of print].
George, O., Sanders, C., Freiling, J., Grigoryan, E., Vu, S., Allen, C. D., et al. (2012). Recruitment of medial prefrontal cortex neurons during alcohol withdrawal predicts cognitive impairment and excessive alcohol drinking. Proc. Natl. Acad. Sci. U S A 109, 18156–18161. doi: 10.1073/pnas.1116523109
Gheorvassaki, E. G., Thermos, K., Liapakis, G., and Spyraki, C. (1992). Effects of acute and chronic desipramine treatment on somatostatin receptors in brain. Psychopharmacology 108, 363–366. doi: 10.1007/bf02245124
Ghosal, S., Duman, C. H., Liu, R. J., Wu, M., Terwilliger, R., Girgenti, M. J., et al. (2020). Ketamine rapidly reverses stress-induced impairments in GABAergic transmission in the prefrontal cortex in male rodents. Neurobiol. Dis. 134:104669. doi: 10.1016/j.nbd.2019.104669
Gilpin, N. W., Misra, K., Herman, M. A., Cruz, M. T., Koob, G. F., and Roberto, M. (2011). Neuropeptide y opposes alcohol effects on gamma-aminobutyric acid release in amygdala and blocks the transition to alcohol dependence. Biol. Psychiatry 69, 1091–1099. doi: 10.1016/j.biopsych.2011.02.004
Girgenti, M. J., Wohleb, E. S., Mehta, S., Ghosal, S., Fogaca, M. V., and Duman, R. S. (2019). Prefrontal cortex interneurons display dynamic sex-specific stress-induced transcriptomes. Transl. Psychiatry 9:292. doi: 10.1038/s41398-019-0642-z
Glaser, Y. G., Zubieta, J. K., Hsu, D. T., Villafuerte, S., Mickey, B. J., Trucco, E. M., et al. (2014). Indirect effect of corticotropin-releasing hormone receptor 1 gene variation on negative emotionality and alcohol use via right ventrolateral prefrontal cortex. J. Neurosci. 34, 4099–4107. doi: 10.1523/jneurosci.3672-13.2014
Glorioso, C., Sabatini, M., Unger, T., Hashimoto, T., Monteggia, L. M., Lewis, D. A., et al. (2006). Specificity and timing of neocortical transcriptome changes in response to BDNF gene ablation during embryogenesis or adulthood. Mol. Psychiatry 11, 633–648. doi: 10.1038/sj.mp.4001835
Goldstein, A., Tachibana, S., Lowney, L. I., Hunkapiller, M., and Hood, L. (1979). Dynorphin-(1–13), an extraordinarily potent opioid peptide. Proc. Natl. Acad. Sci. U S A 76, 6666–6670. doi: 10.1073/pnas.76.12.6666
Goldstein, R. Z., and Volkow, N. D. (2011). Dysfunction of the prefrontal cortex in addiction: neuroimaging findings and clinical implications. Nat. Rev. Neurosci. 12, 652–669. doi: 10.1038/nrn3119
Gondré-Lewis, M. C., Warnock, K. T., Wang, H., June, H. L., Bell, K. A., Rabe, H., et al. (2016). Early life stress is a risk factor for excessive alcohol drinking and impulsivity in adults and is mediated via a CRF/GABAA mechanism. Stress 19, 235–247. doi: 10.3109/10253890.2016.1160280
Grammatopoulos, D. K., Randeva, H. S., Levine, M. A., Kanellopoulou, K. A., and Hillhouse, E. W. (2001). Rat cerebral cortex corticotropin-releasing hormone receptors: evidence for receptor coupling to multiple G-proteins. J. Neurochem. 76, 509–519. doi: 10.1046/j.1471-4159.2001.00067.x
Grossmann, T. (2013). The role of medial prefrontal cortex in early social cognition. Front. Hum. Neurosci. 7:340. doi: 10.3389/fnhum.2013.00340
Guan, X., Wan, R., Zhu, C., and Li, S. (2014). Corticotropin-releasing factor receptor type-2 is involved in the cocaine-primed reinstatement of cocaine conditioned place preference in rats. Behav. Brain Res. 258, 90–96. doi: 10.1016/j.bbr.2013.10.019
Gudehithlu, K. P., Duchemin, A. M., Tejwani, G. A., Neff, N. H., and Hadjiconstantinou, M. (2012). Nicotine-induced changes of brain β-endorphin. Neuropeptides 46, 125–131. doi: 10.1016/j.npep.2012.03.001
Hadjidakis, D. J., Raptis, S. A., Souvatzoglou, A., Karaiskos, C., Diamantopoulos, E. J., and Moulopoulos, S. D. (1986). Differences between somatostatin-28 and somatostatin-14 with respect to their biological effects in healthy humans and acromegalics. Clin. Physiol. Biochem. 4, 372–383.
Hang, A., Wang, Y. J., He, L., and Liu, J. G. (2015). The role of the dynorphin/κ opioid receptor system in anxiety. Acta Pharmacol. Sin. 36, 783–790. doi: 10.1038/aps.2015.32
Hashimoto, T., Arion, D., Unger, T., Maldonado-Avilés, J. G., Morris, H. M., Volk, D. W., et al. (2008a). Alterations in GABA-related transcriptome in the dorsolateral prefrontal cortex of subjects with schizophrenia. Mol. Psychiatry 13, 147–161. doi: 10.1038/sj.mp.4002011
Hashimoto, T., Bazmi, H., Mirnics, K., Wu, Q., Sampson, A. R., and Lewis, D. A. (2008b). Conserved regional patterns of GABA-related transcript expression in the neocortex of subjects with schizophrenia. Am. J. Psychiatry 165, 479–489. doi: 10.1176/appi.ajp.2007.07081223
Hauger, R. L., Grigoriadis, D. E., Dallman, M. F., Plotsky, P. M., Vale, W. W., and Dautzenberg, F. M. (2003). International Union of Pharmacology. XXXVI. Current status of the nomenclature for receptors for corticotropin-releasing factor and their ligands. Pharmacol. Rev. 55, 21–26. doi: 10.1124/pr.55.1.3
Hauser, K. F., Aldrich, J. V., Anderson, K. J., Bakalkin, G., Christie, M. J., Hall, E. D., et al. (2005). Pathobiology of dynorphins in trauma and disease. Front. Biosci. 10, 216–235. doi: 10.2741/1522
Hayashi, M., and Oshima, K. (1986). Neuropeptides in cerebral cortex of macaque monkey (Macaca fuscata fuscata): regional distribution and ontogeny. Brain Res. 364, 360–368. doi: 10.1016/0006-8993(86)90848-6
Hayashi, M., Yamashita, A., and Shimizu, K. (1997). Somatostatin and brain-derived neurotrophic factor mRNA expression in the primate brain: decreased levels of mRNAs during aging. Brain Res. 749, 283–289. doi: 10.1016/s0006-8993(96)01317-0
Heilig, M. (2004). The NPY system in stress, anxiety and depression. Neuropeptides 38, 213–224. doi: 10.1016/j.npep.2004.05.002
Hendry, S., Jones, E., DeFelipe, J., Schmechel, D., Brandon, C., and Emson, P. (1984). Neuropeptide-containing neurons of the cerebral cortex are also GABAergic. Proc. Natl. Acad. Sci. U S A 81, 6526–6530. doi: 10.1073/pnas.81.20.6526
Henry, D. J., Grandy, D. K., Lester, H. A., Davidson, N., and Chavkin, C. (1995). Kappa-opioid receptors couple to inwardly rectifying potassium channels when coexpressed by Xenopus oocytes. Mol. Pharmacol. 47, 551’557. doi: 10.1016/0304-3940(95)11289-9
Hicks, S. D., Jacob, P., Perez, O., Baffuto, M., Gagnon, Z., and Middleton, F. A. (2019). The transcriptional signature of a runner’s high. Med. Sci. Sports Exerc. 51, 970–978. doi: 10.1249/MSS.0000000000001865
Hou, C., Jia, F., Liu, Y., and Li, L. (2006). CSF serotonin, 5-hydroxyindolacetic acid and neuropeptide Y levels in severe major depressive disorder. Brain Res. 1095, 154–158. doi: 10.1016/j.brainres.2006.04.026
Hupalo, S., and Berridge, C. W. (2016). Working memory impairing actions of Corticotropin-Releasing Factor (CRF) neurotransmission in the prefrontal cortex. Neuropsychopharmacology 41, 2733–2740. doi: 10.1038/npp.2016.85
Hupalo, S., Bryce, C. A., Bangasser, D. A., Berridge, C. W., Valentino, R. J., and Floresco, S. B. (2019a). Corticotropin-Releasing Factor (CRF) circuit modulation of cognition and motivation. Neurosci. Biobehav. Rev. 103, 50–59. doi: 10.1016/j.neubiorev.2019.06.010
Hupalo, S., Martin, A. J., Green, R. K., Devilbiss, D. M., and Berridge, C. W. (2019b). Prefrontal corticotropin-releasing factor (CRF) neurons act locally to modulate frontostriatal cognition and circuit function. J. Neurosci. 39, 2080–2090. doi: 10.1523/jneurosci.2701-18.2019
Hurd, Y. L. (1996). Differential messenger RNA expression of prodynorphin and proenkephalin in the human brain. Neuroscience 72, 767–783. doi: 10.1016/0306-4522(96)00002-4
Iritani, S., and Satoh, K. (1991). Distribution of somatostatin-immunoreactive cell bodies and fibers in the neocortex of Macaca fuscata. Synapse 9, 50–59. doi: 10.1002/syn.890090108
Itoga, C. A., Chen, Y., Fateri, C., Echeverry, P. A., Lai, J. M., Delgado, J., et al. (2019). New viral-genetic mapping uncovers an enrichment of corticotropin-releasing hormone-expressing neuronal inputs to the nucleus accumbens from stress-related brain regions. J. Comp. Neurol. 527, 2474–2487. doi: 10.1002/cne.24676
Jackson, J., Karnani, M. M., Zemelman, B. V., Burdakov, D., and Lee, A. K. (2018). Inhibitory control of prefrontal cortex by the claustrum. Neuron 99, 1029.e4–1039.e4. doi: 10.1016/j.neuron.2018.07.031
Jacobson, S., Butters, N., and Tovsky, N. J. (1978). Afferent and efferent subcortical projections of behaviorally defined sectors of prefrontal granular cortex. Brain Res. 159, 279–296. doi: 10.1016/0006-8993(78)90535-8
Jaferi, A., and Bhatnagar, S. (2007). Corticotropin-releasing hormone receptors in the medial prefrontal cortex regulate hypothalamic-pituitary-adrenal activity and anxiety-related behavior regardless of prior stress experience. Brain Res. 1186, 212–223. doi: 10.1016/j.brainres.2007.07.100
James, I. F., Chavkin, C., and Goldstein, A. (1982). Selectivity of dynorphin for κ opioid receptors. Life Sci. 31, 1331–1334. doi: 10.1016/0024-3205(82)90374-5
Joshi, D., Catts, V. S., Olaya, J. C., and Weickert, C. S. (2015). Relationship between somatostatin and death receptor expression in the orbital frontal cortex in schizophrenia: a postmortem brain mRNA study. NPJ Schizophr. 1:14004. doi: 10.1038/npjschz.2014.4
Kai, Y., Li, Y., Sun, T., Yin, W., Mao, Y., Li, J., et al. (2018). A medial prefrontal cortex-nucleus acumens corticotropin-releasing factor circuitry for neuropathic pain-increased susceptibility to opioid reward. Transl. Psychiatry 8:100. doi: 10.1038/s41398-018-0152-4
Kakidani, H., Furutani, Y., Takahashi, H., Noda, M., Morimoto, Y., Hirose, T., et al. (1982). Cloning and sequence analysis of cDNA for porcine β-neo-endorphin/dynorphin precursor. Nature 298, 245–249. doi: 10.1038/298245a0
Karisetty, B. C., Joshi, P. C., Kumar, A., and Chakravarty, S. (2017). Sex differences in the effect of chronic mild stress on mouse prefrontal cortical BDNF levels: a role of major ovarian hormones. Neuroscience 356, 89–101. doi: 10.1016/j.neuroscience.2017.05.020
Karkhanis, A., Holleran, K. M., and Jones, S. R. (2017). Dynorphin/kappa opioid receptor signaling in preclinical models of alcohol, drug and food addiction. Int. Rev. Neurobiol. 136, 53–88. doi: 10.1016/bs.irn.2017.08.001
Kaseda, E. T., and Levine, A. J. (2020). Post-traumatic stress disorder: a differential diagnostic consideration for COVID-19 survivors. Clin. Neuropsychol. doi: 10.1080/13854046.2020.1811894 [Epub ahead of print].
Kash, T. L., Pleil, K. E., Marcinkiewcz, C. A., Lowery-Gionta, E. G., Crowley, N., Mazzone, C., et al. (2015). Neuropeptide regulation of signaling and behavior in the BNST. Mol. Cells 38, 1–13. doi: 10.14348/molcells.2015.2261
Kash, T. L., and Winder, D. G. (2006). Neuropeptide Y and corticotropin-releasing factor bi-directionally modulate inhibitory synaptic transmission in the bed nucleus of the stria terminalis. Neuropharmacology 51, 1013–1022. doi: 10.1016/j.neuropharm.2006.06.011
Keller, B., La Harpe, R., and García-Sevilla, J. A. (2017). Upregulation of IRAS/nischarin (I1-imidazoline receptor), a regulatory protein of μ-opioid receptor trafficking, in postmortem prefrontal cortex of long-term opiate and mixed opiate/cocaine abusers. Neurochem. Int. 108, 282–286. doi: 10.1016/j.neuint.2017.04.017
Ketchesin, K. D., Huang, N. S., and Seasholtz, A. F. (2017). Cell type-specific expression of corticotropin-releasing hormone-binding protein in GABAergic interneurons in the prefrontal cortex. Front. Neuroanat. 11:90. doi: 10.3389/fnana.2017.00090
Ketchesin, K. D., Stinnett, G. S., and Seasholtz, A. F. (2016). Binge drinking decreases corticotropin-releasing factor-binding protein expression in the medial prefrontal cortex of mice. Alcohol. Clin. Exp. Res. 40, 1641–1650. doi: 10.1111/acer.13119
Kievit, J., and Kuypers, H. G. J. M. (1975). Basal forebrain and hypothalamic connections to frontal and parietal cortex in the rhesus monkey. Science 187, 660–662. doi: 10.1126/science.1114317
Kiosterakis, G., Stamatakis, A., Diamantopoulou, A., Fameli, M., and Stylianopoulou, F. (2009). Long-term effects of neonatal handling on Mu-opioid receptor levels in the brain of the offspring. Dev. Psychobiol. 51, 439–449. doi: 10.1002/dev.20383
Knoll, A. T., and Carlezon, W. A. (2010). Dynorphin, stress and depression. Brain Res. 1314, 56–73. doi: 10.1016/j.brainres.2009.09.074
Kobayashi, M., Hayashi, Y., Fujimoto, Y., and Matsuoka, I. (2018). Decreased parvalbumin and somatostatin neurons in medial prefrontal cortex in BRINP1-KO mice. Neurosci. Lett. 683, 82–88. doi: 10.1016/j.neulet.2018.06.050
Koenigs, M., and Grafman, J. (2009). Posttraumatic stress disorder: the role of medial prefrontal cortex and amygdala. Neuroscientist 15, 540–548. doi: 10.1177/1073858409333072
Kofman, Y. B., and Garfin, D. R. (2020). Home is not always a haven: the domestic violence crisis amid the COVID-19 pandemic. Psychol. Trauma 12, S199–S201. doi: 10.1037/tra0000866
Kolb, B., Mychasiuk, R., Muhammad, A., Li, Y., Frost, D. O., and Gibb, R. (2012). Experience and the developing prefrontal cortex. Proc. Natl. Acad. Sci. U S A 109, 17186–17196. doi: 10.1073/pnas.1121251109
Konkoy, C. S., and Childers, S. R. (1993). Relationship between kappa1 opioid receptor binding and inhibition of adenylyl cyclase in guinea pig brain membranes. Biochem. Pharmacol. 45, 207–216. doi: 10.1016/0006-2952(93)90394-c
Koob, G. F. (2013). Addiction is a reward deficit and stress surfeit disorder. Front. Psychiatry 4:72. doi: 10.3389/fpsyt.2013.00072
Koob, G. F., and Heinrichs, S. C. (1999). A role for corticotropin releasing factor and urocortin in behavioral responses to stressors. Brain Res. 848, 141–152. doi: 10.1016/s0006-8993(99)01991-5
Koob, G. F., and Le Moal, M. (2008). Neurobiological mechanisms for opponent motivational processes in addiction. Philos. Trans. R. Soc. Lond. B Biol. Sci. 363, 3113–3123. doi: 10.1098/rstb.2008.0094
Koob, G. F., and Volkow, N. D. (2016). Neurobiology of addiction: a neurocircuitry analysis. Lancet Psychiatry 3, 760–773. doi: 10.1016/s2215-0366(16)00104-8
Kopp, J., Xu, Z. Q., Zhang, X., Pedrazzini, T., Herzog, H., Kresse, A., et al. (2002). Expression of the neuropeptide Y Y1 receptor in the CNS of rat and of wild-type and Y1 receptor knock-out mice. Focus on immunohistochemical localization. Neuroscience 111, 443–532. doi: 10.1016/s0306-4522(01)00463-8
Krishnan, V., and Nestler, E. J. (2011). Animal models of depression: molecular perspectives. Curr. Top. Behav. Neurosci. 7, 121–147. doi: 10.1007/7854_2010_108
Krulich, L., Dhariwal, A. P., and McCann, S. M. (1968). Stimulatory and inhibitory effects of purified hypothalamic extracts on growth hormone release from rat pituitary in vitro. Endocrinology 83, 783–790. doi: 10.1210/endo-83-4-783
Kumar, U. (2005). Expression of somatostatin receptor subtypes (SSTR1–5) in Alzheimer’s disease brain: An immunohistochemical analysis. Neuroscience 134, 525–538. doi: 10.1016/j.neuroscience.2005.04.001
Kumar, U., and Grant, M. (2010). Somatostatin and somatostatin receptors. Results Probl. Cell Differ. 50, 137–184. doi: 10.1007/400_2009_29
Kuromitsu, J., Yokoi, A., Kawai, T., Nagasu, T., Aizawa, T., Haga, S., et al. (2001). Reduced neuropeptide Y mRNA levels in the frontal cortex of people with schizophrenia and bipolar disorder. Gene Expr. Patterns. 1, 17–21. doi: 10.1016/s1567-133x(01)00003-5
Kurumaji, A., Takashima, M., and Shibuya, H. (1987). Cold and immobilization stress-induced changes in pain responsiveness and brain met-enkephalin-like immunoreactivity in the rat. Peptides 8, 355–359. doi: 10.1016/0196-9781(87)90111-2
Lahti, R. A., Mickelson, M. M., Jodelis, K. S., and McCall, J. M. (1989). Comparative neuroanatomical distribution of the κ and μ opioid receptors in guinea pig brain sections. Eur. J. Pharmacol. 166, 563–566. doi: 10.1016/0014-2999(89)90377-4
Laubach, M., Amarante, L. M., Swanson, K., and White, S. R. (2018). What, if anything, is rodent prefrontal cortex? eNeuro 5:ENEURO.0315-18.2018. doi: 10.1523/ENEURO.0315-18.2018
Lawrence, D. M. P., Joseph, D. B., and Bidlack, J. M. (1995). Kappa opioid receptors expressed on three related thymoma cell lines. Differences in receptor-effector coupling. Biochem. Pharmacol. 49, 81–89. doi: 10.1016/0006-2952(94)00440-w
LeDoux, J. E. (1993). Emotional memory systems in the brain. Behav. Brain Res. 58, 69–79. doi: 10.1016/0166-4328(93)90091-4
Leriche, M., Cote-Vélez, A., and Méndez, M. (2007). Presence of pro-opiomelanocortin mRNA in the rat medial prefrontal cortex, nucleus accumbens and ventral tegmental area: studies by RT-PCR and in situ hybridization techniques. Neuropeptides 41, 421–431. doi: 10.1016/j.npep.2007.08.004
Lewis, D. A., Campbell, M. J., and Morrison, J. H. (1986). An immunohistochemical characterization of somatostatin-28 and somatostatin-281–12 in monkey prefrontal cortex. J. Comp. Neurol. 248, 1–18. doi: 10.1002/cne.902480102
Lewis, K., Li, C., Perrin, M. H., Blount, A., Kunitake, K., Donaldson, C., et al. (2001). Identification of urocortin III, an additional member of the corticotropin-releasing factor (CRF) family with high affinity for the CRF2 receptor. Proc. Natl. Acad. Sci. U S A 98, 7570–7575. doi: 10.1073/pnas.121165198
Li, C., Liu, Y., Yin, S., Lu, C., Liu, D., Jiang, H., et al. (2015). Long-term effects of early adolescent stress: dysregulation of hypothalamic-pituitary-adrenal axis and central corticotropin releasing factor receptor 1 expression in adult male rats. Behav. Brain Res. 288, 39–49. doi: 10.1016/j.bbr.2015.04.007
Li, C., Pleil, K. E., Stamatakis, A. M., Busan, S., Vong, L., Lowell, B. B., et al. (2012). Presynaptic inhibition of gamma-aminobutyric acid release in the bed nucleus of the stria terminalis by kappa opioid receptor signaling. Biol. Psychiatry 71, 725–732. doi: 10.1016/j.biopsych.2011.11.015
Li, Q., Bartley, A. F., Lynn, X., and Dobrunz, E. (2017). Endogenously released neuropeptide Y suppresses hippocampal short-term facilitation and is impaired by stress-induced anxiety. J. Neurosci. 37, 23–37. doi: 10.1523/JNEUROSCI.2599-16.2016
Li, W., Papilloud, A., Lozano-Montes, L., Zhao, N., Ye, X., Zhang, X., et al. (2018). Stress impacts the regulation neuropeptides in the rat hippocampus and prefrontal cortex. Proteomics 18:e1700408. doi: 10.1002/pmic.201700408
Liguz-Lecznar, M., Urban-Ciecko, J., and Kossut, M. (2016). Somatostatin and somatostatin-containing neurons in shaping neuronal activity and plasticity. Front. Neural Circuits 10:48. doi: 10.3389/fncir.2016.00048
Liu, J., Yu, B., Neugebauer, V., Grigoriadis, D. E., Rivier, J., Vale, W. W., et al. (2004). Corticotropin-releasing factor and urocortin I modulate excitatory glutamatergic synaptic transmission. J. Neurosci. 24, 4020–4029. doi: 10.1523/jneurosci.5531-03.2004
Lovenberg, T. W., Liaw, C. W., Grigoriadis, D. E., Clevenger, W., Chalmers, D. T., De Souza, E. B., et al. (1995). Cloning and characterization of a functionally distinct corticotropin-releasing factor receptor subtype from rat brain. Proc. Natl. Acad. Sci. U S A 92, 836–840. doi: 10.1073/pnas.92.3.836
Lowery-Gionta, E. G., Crowley, N. A., Bukalo, O., Silverstein, S., Holmes, A., and Kash, T. L. (2018). Chronic stress dysregulates amygdalar output to the prefrontal cortex. Neuropharmacology 139, 68–75. doi: 10.1016/j.neuropharm.2018.06.032
Lowery-Gionta, E. G., Navarro, M., Li, C., Pleil, K. E., Rinker, J. A., Cox, B. R., et al. (2012). Corticotropin releasing factor signaling in the central amygdala is recruited during binge-like ethanol consumption in C57BL/6J mice. J. Neurosci. 32, 3405–3413. doi: 10.1523/jneurosci.6256-11.2012
Lundberg, J. M., Terenius, L., Hokfelt, T., Martling, C. R., Tatemoto, K., Mutt, V., et al. (1982). Neuropeptide Y (NPY)-like immunoreactivity in peripheral noradrenergic neurons and effects of NPY on sympathetic function. Acta Physiol. Scand. 116, 477–480. doi: 10.1111/j.1748-1716.1982.tb07171.x
Magalhaes, A. C., Holmes, K. D., Dale, L. B., Comps-Agrar, L., Lee, D., Yadav, P. N., et al. (2010). CRF receptor 1 regulates anxiety behavior via sensitization of 5-HT2 receptor signaling. Nat. Neurosci. 13, 622–629. doi: 10.1038/nn.2529
Mansour, A., Burke, S., Pavlic, R. J., Akil, H., and Watson, S. J. (1996). Immunohistochemical localization of the cloned κ1 receptor in the rat CNS and pituitary. Neuroscience 71, 671–690. doi: 10.1016/0306-4522(95)00464-5
Marek, G. J., and Aghajanian, G. K. (1998). 5-Hydroxytryptamine-induced excitatory postsynaptic currents in neocortical layer V pyramidal cells: suppression by μ-opiate receptor activation. Neuroscience 86, 485–497. doi: 10.1016/s0306-4522(98)00043-8
Marek, G. J., Wright, R. A., Gewirtz, J. C., and Schoepp, D. D. (2001). A major role for thalamocortical afferents in serotonergic hallucinogen receptor function in the rat neocortex. Neuroscience 105, 379–392. doi: 10.1016/s0306-4522(01)00199-3
Marinelli, P. W., Kiianmaa, K., and Gianoulakis, C. (2000). Opioid propeptide mRNA content and receptor density in the brains of AA and ANA rats. Life Sci. 66, 1915–1927. doi: 10.1016/s0024-3205(00)00517-8
Marshall, B., Bland, M. K., Hulla, R., and Gatchel, R. J. (2019). Considerations in addressing the opioid epidemic and chronic pain within the USA. Pain Manag. 9, 131–138. doi: 10.2217/pmt-2018-0070
Matthews, S. G., Heavens, R. P., and Sirinathsinghji, D. J. S. (1992). Distribution and cellular localization of preproenkephalin mRNA in the ovine brain and pituitary. Mol. Brain Res. 12, 349–355. doi: 10.1016/0169-328x(92)90139-3
McFalls, A. J., Imperio, C. G., Bixler, G., Freeman, W. M., Grigson, P. S., and Vrana, K. E. (2016). Reward devaluation and heroin escalation is associated with differential expression of CRF signaling genes. Brain Res. Bull. 123, 81–93. doi: 10.1016/j.brainresbull.2015.11.009
McGarry, L. M., and Carter, A. G. (2017). Prefrontal cortex drives distinct projection neurons in the basolateral amygdala. Cell Rep. 21, 1426–1433. doi: 10.1016/j.celrep.2017.10.046
McGuire, J. L., Larke, L. E., Sallee, F. R., Herman, J. P., and Sah, R. (2011). Differential regulation of neuropeptide Y in the amygdala and prefrontal cortex during recovery from chronic variable stress. Front. Behav. Neurosci. 5:54. doi: 10.3389/fnbeh.2011.00054
McIntosh, T. K., Head, V. A., and Faden, A. I. (1987). Alterations in regional concentrations of endogenous opioids following traumatic brain injury in the cat. Brain Res. 425, 225–233. doi: 10.1016/0006-8993(87)90505-1
McKinney, B. C., Lin, C. W., Oh, H., Tseng, G. C., Lewis, D. A., and Sibille, E. (2015). Hypermethylation of BDNF and SST genes in the orbital frontal cortex of older individuals: a putative mechanism for declining gene expression with age. Neuropsychopharmacology 40, 2604–2613. doi: 10.1038/npp.2015.107
McKlveen, J. M., Moloney, R. D., Scheimann, J. R., Myers, B., and Herman, J. P. (2019). “Braking” the prefrontal cortex: the role of glucocorticoids and interneurons in stress adaptation and pathology. Biol. Psychiatry 86, 669–681. doi: 10.1016/j.biopsych.2019.04.032
McLennan, G. P., Kiss, A., Miyatake, M., Belcheva, M. M., Chambers, K. T., Pozek, J. J., et al. (2008). Kappa opioids promote the proliferation of astrocytes via Gβγ and β-arrestin 2-dependent MAPK-mediated pathways. J. Neurochem. 107, 1753–1765. doi: 10.1111/j.1471-4159.2008.05745.x
McReynolds, J. R., Peña, D. F., Blacktop, J. M., and Mantsch, J. R. (2014). Neurobiological mechanisms underlying relapse to cocaine use: contributions of CRF and noradrenergic systems and regulation by glucocorticoids. Stress 17, 22–38. doi: 10.3109/10253890.2013.872617
Melas, P. A., Mannervik, M., Mathé, A. A., and Lavebratt, C. (2012). Neuropeptide Y: identification of a novel rat mRNA splice-variant that is downregulated in the hippocampus and the prefrontal cortex of a depression-like model. Peptides 35, 49–55. doi: 10.1002/syn.20514
Melchitzky, D. S., and Lewis, D. A. (2008). Dendritic-targeting GABA neurons in monkey prefrontal cortex: comparison of somatostatin- and calretinin-immunoreactive axon terminals. Synapse 62, 456–465. doi: 10.1002/syn.20514
Mellios, N., Huang, H. S., Baker, S. P., Galdzicka, M., Ginns, E., and Akbarian, S. (2009). Molecular determinants of dysregulated GABAergic gene expression in the prefrontal cortex of subjects with schizophrenia. Biol. Psychiatry 65, 1006–1014. doi: 10.1016/j.biopsych.2008.11.019
Meng, Q. Y., Chen, X. N., Tong, D. L., and Zhou, J. N. (2011). Stress and glucocorticoids regulated corticotropin releasing factor in rat prefrontal cortex. Mol. Cell. Endocrinol. 342, 54–63. doi: 10.1016/j.mce.2011.05.035
Miguel, T. T., Gomes, K. S., and Nunes-de-Souza, R. L. (2014). Tonic modulation of anxiety-like behavior by corticotropin-releasing factor (CRF) type 1 receptor (CRF1) within the medial prefrontal cortex (mPFC) in male mice: role of protein kinase A (PKA). Horm. Behav. 66, 247–256. doi: 10.1016/j.yhbeh.2014.05.003
Millan, M. A., Jacobowitz, D. M., Hauger, R. L., Catt, K. J., and Aguilera, G. (1986). Distribution of corticotropin-releasing factor receptors in primate brain. Proc. Natl. Acad. Sci. U S A 83, 1921–1925. doi: 10.1073/pnas.83.6.1921
Morales-Mulia, M., Panayi, F., Lambás-Señas, L., Scarna, H., and Méndez, M. (2007). Changes in Proenkephalin mRNA expression in forebrain areas after amphetamine-induced behavioural sensitization. Pharmacol. Biochem. Behav. 87, 232–240. doi: 10.1016/j.pbb.2007.04.019
Morisset, J. (2017). Somatostatin: one of the rare multifunctional inhibitors of mammalian species. Pancreas 46, 8–18. doi: 10.1097/MPA.0000000000000716
Morris, H. M., Hashimoto, T., and Lewis, D. A. (2008). Alterations in somatostatin mRNA expression in the dorsolateral prefrontal cortex of subjects with schizophrenia or schizoaffective disorder. Cereb. Cortex 18, 1575–1587. doi: 10.1093/cercor/bhm186
Murray, E. A., Wise, S. P., and Drevets, W. C. (2011). Localization of dysfunction in major depressive disorder: prefrontal cortex and amygdala. Biol. Psychiatry 69, e43–e54. doi: 10.1016/j.biopsych.2010.09.041
Murueta-Goyena, A., Ortuzar, N., Lafuente, J. V., and Bengoetxea, H. (2020). Enriched environment reverts somatostatin interneuron loss in MK-801 model of schizophrenia. Mol. Neurobiol. 57, 125–134. doi: 10.1007/s12035-019-01762-y
Northoff, G., and Sibille, E. (2014). Why are cortical GABA neurons relevant to internal focus in depression? A cross-level model linking cellular, biochemical and neural network findings. Mol. Psychiatry 19, 966–977. doi: 10.1038/mp.2014.68
Oh, H., Piantadosi, S. C., Rocco, B. R., Lewis, D. A., Watkins, S. C., and Sibille, E. (2019). The role of dendritic brain-derived neurotrophic factor transcripts on altered inhibitory circuitry in depression. Biol. Psychiatry 85, 517–526. doi: 10.1016/j.biopsych.2018.09.026
Ohata, H., and Shibasaki, T. (2011). Microinjection of different doses of corticotropin-releasing factor into the medial prefrontal cortex produces effects opposing anxiety-related behavior in rats. J. Nippon Med. Sch. 78, 286–292. doi: 10.1272/jnms.78.286
Olianas, M. C., Dedoni, S., and Onali, P. (2012). Potentiation of dopamine D1-like receptor signaling by concomitant activation of δ- and μ-opioid receptors in mouse medial prefrontal cortex. Neurochem. Int. 61, 1404–1416. doi: 10.1016/j.neuint.2012.10.005
Olpe, H. R., Balcar, V. J., Bittiger, H., Rink, H., and Sieber, P. (1980). Central actions of somatostatin. Eur. J. Pharmacol. 63, 127–133. doi: 10.1016/0014-2999(80)90436-7
Ordway, G. A., Stockmeier, C. A., Meltzer, H. Y., Overholser, J. C., Jaconetta, S., and Widdowson, P. S. (1995). Neuropeptide Y in frontal cortex is not altered in major depression. J. Neurochem. 65, 1646–1650. doi: 10.1046/j.1471-4159.1995.65041646.x
Orozco-Cabal, L., Liu, J., Pollandt, S., Schmidt, K., Shinnick-Gallagher, P., and Gallagher, J. P. (2008). dopamine and corticotropin-releasing factor synergistically alter basolateral amygdala-to-medial prefrontal cortex synaptic transmission: functional switch after chronic cocaine administration. J. Neurosci. 28, 529–542. doi: 10.1523/JNEUROSCI.2666-07.2008
Orozco-Cabal, L., Pollandt, S., Liu, J., Shinnick-Gallagher, P., and Gallagher, J. P. (2006). Regulation of synaptic transmission by CRF receptors. Rev. Neurosci. 17, 279–307. doi: 10.1515/revneuro.2006.17.3.279
Ouhaz, Z., Fleming, H., and Mitchell, A. S. (2018). Cognitive functions and neurodevelopmental disorders involving the prefrontal cortex and mediodorsal thalamus. Front. Neurosci. 12:33. doi: 10.3389/fnins.2018.00033
Overstreet, D. H., Friedman, E., Mathé, A. A., and Yadid, G. (2005). The Flinders Sensitive Line rat: a selectively bred putative animal model of depression. Neurosci. Biobehav. Rev. 29, 739–759. doi: 10.1016/j.neubiorev.2005.03.015
Owens, M. J., and Nemeroff, C. B. (1993). The role of corticotropin-releasing factor in the pathophysiology of affective and anxiety disorders: laboratory and clinical studies. Ciba Found. Symp. 172, 296–308. doi: 10.1002/9780470514368.ch15
Pallis, E., Vasilaki, A., Fehlmann, D., Kastellakis, A., Hoyer, D., Spyraki, C., et al. (2009). Antidepressants influence somatostatin levels and receptor pharmacology in brain. Neuropsychopharmacology 34, 952–963. doi: 10.1038/npp.2008.133
Palmisano, M., Caputi, F. F., Mercatelli, D., Romualdi, P., and Candeletti, S. (2019). Dynorphinergic system alterations in the corticostriatal circuitry of neuropathic mice support its role in the negative affective component of pain. Genes Brain Behav. 18:e12467. doi: 10.1111/gbb.12467
Pandey, G. N., Rizavi, H. S., Bhaumik, R., and Ren, X. (2019). Increased protein and mRNA expression of corticotropin-releasing factor (CRF), decreased CRF receptors and CRF binding protein in specific postmortem brain areas of teenage suicide subjects. Psychoneuroendocrinology 106, 233–243. doi: 10.1016/j.psyneuen.2019.04.015
Park, J., and Moghaddam, B. (2017). Impact of anxiety on prefrontal cortex encoding of cognitive flexibility. Neuroscience 345, 193–202. doi: 10.1016/j.neuroscience.2016.06.013
Patel, Y. C., Greenwood, M. T., Warszynska, A., Panetta, R., and Srikant, C. B. (1994). All five cloned human somatostatin receptors (hSSTR1–5) are functionally coupled to adenylyl cyclase. Biochem. Biophys. Res. Commun. 198, 605–612. doi: 10.1006/bbrc.1994.1088
Peckys, D., and Hurd, Y. L. (2001). Prodynorphin and κ opioid receptor mRNA expression in the cingulate and prefrontal cortices of subjects diagnosed with schizophrenia or affective disorders. Brain Res. Bull. 55, 619–624. doi: 10.1016/s0361-9230(01)00525-1
Pedragosa-Badia, X., Stichel, J., and Beck-Sickinger, A. G. (2013). Neuropeptide y receptors: how to get subtype selectivity. Front. Endocrinol. 4:5. doi: 10.3389/fendo.2013.00005
Pentkowski, N. S., Tovote, P., Zavala, A. R., Litvin, Y., Blanchard, D. C., Spiess, J., et al. (2013). Cortagine infused into the medial prefrontal cortex attenuates predator-induced defensive behaviors and Fos protein production in selective nuclei of the amygdala in male CD1 mice. Horm. Behav. 64, 519–526. doi: 10.1016/j.yhbeh.2013.06.008
Perez, S. M., Boley, A., and Lodge, D. J. (2019). Region specific knockdown of Parvalbumin or Somatostatin produces neuronal and behavioral deficits consistent with those observed in schizophrenia. Transl. Psychiatry 9:264. doi: 10.1038/s41398-019-0603-6
Perrin, M., Donaldson, C., Chen, R., Blount, A., Berggren, T., Bilezikjian, L., et al. (1995). Identification of a second corticotropin-releasing factor receptor gene and characterization of a cDNA expressed in heart. Proc. Natl. Acad. Sci. U S A 92, 2969–2973. doi: 10.1073/pnas.92.7.2969
Pleil, K. E., Lowery-Gionta, E. G., Crowley, N. A., Li, C., Marcinkiewcz, C. A., Rose, J. H., et al. (2015). Effects of chronic ethanol exposure on neuronal function in the prefrontal cortex and extended amygdala. Neuropharmacology 99, 735–749. doi: 10.1016/j.neuropharm.2015.06.017
Pons, J., Kitlinska, J., Jacques, D., Perreault, C., Nader, M., Everhart, L., et al. (2008). Interactions of multiple signaling pathways in neuropeptide Y-mediated bimodal vascular smooth muscle cell growth. Can. J. Physiol. Pharmacol. 86, 438–448. doi: 10.1139/y08-054
Porrino, L. J., Crane, A. M., and Goldman-Rakic, P. S. (1981). Direct and indirect pathways from the amygdala to the frontal lobe in rhesus monkeys. J. Comp. Neurol. 198, 121–136. doi: 10.1002/cne.901980111
Pradayrol, L., Jörnvall, H., Mutt, V., and Ribet, A. (1980). N-terminally extended somatostatin: the primary structure of somatostatin-28. FEBS Lett. 109, 55–58. doi: 10.1016/0014-5793(80)81310-x
Rai, U., Thrimawithana, T. R., Valery, C., and Young, S. A. (2015). Therapeutic uses of somatostatin and its analogues: current view and potential applications. Pharmacol. Ther. 152, 98–110. doi: 10.1016/j.pharmthera.2015.05.007
Reyes, T. M., Lewis, K., Perrin, M. H., Kunitake, K. S., Vaughan, J., Arias, C. A., et al. (2001). Urocortin II: a member of the corticotropin-releasing factor (CRF) neuropeptide family that is selectively bound by type 2 CRF receptors. Proc. Natl. Acad. Sci. U S A 98, 2843–2848. doi: 10.1073/pnas.051626398
Robinson, S. L., Marrero, I. M., Perez-Heydrich, C. A., Sepulveda-Orengo, M. T., Reissner, K. J., and Thiele, T. E. (2019a). Medial prefrontal cortex neuropeptide Y modulates binge-like ethanol consumption in C57BL/6J mice. Neuropsychopharmacology 44, 1132–1140. doi: 10.1038/s41386-018-0310-7
Robinson, S. L., Perez-Heydrich, C. A., and Thiele, T. E. (2019b). Corticotropin releasing factor type 1 and 2 receptor signaling in the medial prefrontal cortex modulates Binge-like ethanol consumption in C57BL/6J mice. Brain Sci. 9:171. doi: 10.3390/brainsci9070171
Robinson, S. L., and Thiele, T. E. (2017). The role of neuropeptide Y (NPY) in alcohol and drug abuse disorders. Int. Rev. Neurobiol. 136, 177–197. doi: 10.1016/bs.irn.2017.06.005
Robinson, S. L., and Thiele, T. E. (2020). A role for the neuropeptide somatostatin in the neurobiology of behaviors associated with substances abuse and affective disorders. Neuropharmacology 167:107983. doi: 10.1016/j.neuropharm.2020.107983
Rosa, S. G., Pesarico, A. P., Martini, F., and Nogueira, C. W. (2018a). m-trifluoromethyl-diphenyl diselenide regulates prefrontal cortical MOR and KOR protein levels and abolishes the phenotype induced by repeated forced swim stress in mice. Mol. Neurobiol. 55, 8991–9000. doi: 10.1007/s12035-018-1024-x
Rosa, S. G., Pesarico, A. P., and Nogueira, C. W. (2018b). m-Trifluoromethyl-diphenyl diselenide promotes resilience to social avoidance induced by social defeat stress in mice: contribution of opioid receptors and MAPKs. Prog. Neuropsychopharmacol. Biol. Psychiatry 82, 123–135. doi: 10.1016/j.pnpbp.2017.11.021
Sabban, E. L., Alaluf, L. G., and Serova, L. I. (2016). Potential of neuropeptide Y for preventing or treating post-traumatic stress disorder. Neuropeptides 56, 19–24. doi: 10.1016/j.npep.2015.11.004
Saffari, R., Teng, Z., Zhang, M., Kravchenko, M., Hohoff, C., Ambrée, O., et al. (2016). NPY+-, but not PV+-GABAergic neurons mediated long-range inhibition from infra-to prelimbic cortex. Transl. Psychiatry 6:e736. doi: 10.1038/tp.2016.7
Sah, R., Ekhator, N. N., Jefferson-Wilson, L., Horn, P. S., and Geracioti, T. D. (2014). Cerebrospinal fluid neuropeptide Y in combat veterans with and without posttraumatic stress disorder. Psychoneuroendocrinology 40, 277–283. doi: 10.1016/j.psyneuen.2013.10.017
Sah, R., Ekhator, N. N., Strawn, J. R., Sallee, F. R., Baker, D. G., Horn, P. S., et al. (2009). Low cerebrospinal fluid neuropeptide Y concentrations in posttraumatic stress disorder. Biol. Psychiatry 66, 705–707. doi: 10.1016/j.biopsych.2009.04.037
Sakai, K., Maeda, K., Chihara, K., and Kaneda, H. (1995). Increases in cortical neuropeptide Y and somatostatin concentrations following haloperidol-depot treatment in rats. Neuropeptides 29, 157–161. doi: 10.1016/0143-4179(95)90018-7
Salzman, C. D., and Fusi, S. (2010). Emotion, cognition, and mental state representation in amygdala and prefrontal cortex. Annu. Rev. Neurosci. 33, 173–202. doi: 10.1146/annurev.neuro.051508.135256
Sánchez, M. M., Young, L. J., Plotsky, P. M., and Insel, T. R. (1999). Autoradiographic and in situ hybridization localization of corticotropin-releasing factor 1 and 2 receptors in nonhuman primate brain. J. Comp. Neurol. 408, 365–377. doi: 10.1002/(sici)1096-9861(19990607)408:3<365::aid-cne5>3.0.co;2-n
Schmeltzer, S. N., Vollmer, L. L., Rush, J. E., Weinert, M., Dolgas, C. M., and Sah, R. (2015). History of chronic stress modifies acute stress-evoked fear memory and acoustic startle in male rats. Stress 18, 244–253. doi: 10.3109/10253890.2015.1016495
Schreiber, A. L., Lu, Y. L., Baynes, B. B., Richardson, H. N., and Gilpin, N. W. (2017). Corticotropin-releasing factor in ventromedial prefrontal cortex mediates avoidance of a traumatic stress-paired context. Neuropharmacology 113, 323–330. doi: 10.1016/j.neuropharm.2016.05.008
Selleck, R. A., Giacomini, J., Buchholtz, B. D., Lake, C., Sadeghian, K., and Baldo, B. A. (2018). Modulation of appetitive motivation by prefrontal cortical mu-opioid receptors is dependent upon local dopamine D1 receptor signaling. Neuropharmacology 140, 302–309. doi: 10.1016/j.neuropharm.2018.07.033
Selleck, R. A., Lake, C., Estrada, V., Riederer, J., Andrzejewski, M., Sadeghian, K., et al. (2015). Endogenous opioid signaling in the medial prefrontal cortex is required for the expression of hunger-induced impulsive action. Neuropsychopharmacology 40, 2464–2474. doi: 10.1038/npp.2015.97
Shansky, R. M. (2018). Sex differences in behavioral strategies: avoiding interpretational pitfalls. Curr. Opin. Neurobiol. 49, 95–98. doi: 10.1016/j.conb.2018.01.007
Shansky, R. M. (2019). Are hormones a “female problem” for animal research? Science 364, 825–826. doi: 10.1126/science.aaw7570
Shansky, R. M. (2020). Sex differences in mechanisms of disease. Genes Brain Behav. 19:e12646. doi: 10.1111/gbb.12646
Shenoy, S. S., and Lui, F. (2018). Biochemistry, Endogenous Opioids, StatPearls. Treasure Island, FL: StatPearls Publishing.
Shipton, E. A., Shipton, E. E., and Shipton, A. J. (2018). A review of the opioid epidemic: what do we do about it? Pain Ther. 7, 23–36. doi: 10.1007/s40122-018-0096-7
Sibille, E., Morris, H. M., Kota, R. S., and Lewis, D. A. (2011). GABA-related transcripts in the dorsolateral prefrontal cortex in mood disorders. Int. J. Neuropsychopharmacol. 14, 721–734. doi: 10.1017/s1461145710001616
Siciliano, C. A., Noamany, H., Chang, C. J., Brown, A. R., Chen, X., Leible, D., et al. (2019). A cortical-brainstem circuit predicts and governs compulsive alcohol drinking. Science 366, 1008–1012. doi: 10.1126/science.aay1186
Silberman, Y., Matthews, R. T., and Winder, D. G. (2013). A corticotropin releasing factor pathway for ethanol regulation of the ventral tegmental area in the bed nucleus of the stria terminalis. J. Neurosci. 33, 950–960. doi: 10.1523/JNEUROSCI.2949-12.2013
Simon, N. M., Hoeppner, S. S., Lubin, R. E., Robinaugh, D. J., Malgaroli, M., Norman, S. B., et al. (2020). Understanding the impact of complicated grief on combat related posttraumatic stress disorder, guilt, suicide and functional impairment in a clinical trial of post-9/11 service members and veterans. Depress. Anxiety 37, 63–72. doi: 10.1002/da.22911
Skolnick, P. (2018). The opioid epidemic: crisis and solutions. Annu. Rev. Pharmacol. Toxicol. 58, 143–159. doi: 10.1146/annurev-pharmtox-010617-052534
Sohn, J., Hioki, H., Okamoto, S., and Kaneko, T. (2014). Preprodynorphin-expressing neurons constitute a large subgroup of somatostatin-expressing GABAergic interneurons in the mouse neocortex. J. Comp. Neurol. 522, 1506–1526. doi: 10.1002/cne.23477
Sommer, W. H., Lidström, J., Sun, H., Passer, D., Eskay, R., Parker, S. C. J., et al. (2010). Human NPY promoter variation rs16147:T >C as a moderator of prefrontal NPY gene expression and negative affect. Hum. Mutat. 31, E1594–E1608. doi: 10.1002/humu.21299
Starbäck, P., Wraith, A., Eriksson, H., and Larhammar, D. (2000). Neuropeptide Y receptor gene y6: multiple deaths or resurrections? Biochem. Biophys. Res. Commun. 277, 264–269. doi: 10.1006/bbrc.2000.3656
Stuber, G. D., Sparta, D. R., Stamatakis, A. M., Van Leeuwen, W. A., Hardjoprajitno, J. E., Cho, S., et al. (2011). Excitatory transmission from the amygdala to nucleus accumbens facilitates reward seeking. Nature 475, 377–382. doi: 10.1038/nature10194
Svingos, A. L., and Colago, E. E. O. (2002). κ-opioid and NMDA glutamate receptors are differentially targeted within rat medial prefrontal cortex. Brain Res. 946, 262–271. doi: 10.1016/s0006-8993(02)02894-9
Swanson, L. W., Sawchenko, P. E., Rivier, J., and Vale, W. W. (1983). Organization of ovine corticotropin-releasing factor immunoreactive cells and fibers in the rat brain: an immunohistochemical study. Neuroendocrinology 36, 156–186. doi: 10.1159/000123454
Taki, K., Kaneko, T., and Mizuno, N. (2000). A group of cortical interneurons expressing μ-opioid receptor-like immunoreactivity: a double immunofluorescence study in the rat cerebral cortex. Neuroscience 98, 221–231. doi: 10.1016/s0306-4522(00)00124-x
Taqi, M. M., Bazov, I., Watanabe, H., Sheedy, D., Harper, C., Alkass, K., et al. (2011). Prodynorphin CpG-SNPs associated with alcohol dependence: elevated methylation in the brain of human alcoholics. Addict. Biol. 16, 499–509. doi: 10.1111/j.1369-1600.2011.00323.x
Tatemoto, K., Carlquist, M., and Mutt, V. (1982). Neuropeptide Y—a novel brain peptide with structural similarities to peptide YY and pancreatic polypeptide. Nature 296, 659–660. doi: 10.1038/296659a0
Teffer, K., and Semendeferi, K. (2012). Human prefrontal cortex: evolution, development, and pathology. Prog. Brain Res. 195, 191–218. doi: 10.1016/B978-0-444-53860-4.00009-X
Tejeda, H. A., Hanks, A. N., Scott, L., Mejias-Aponte, C., Hughes, Z. A., and O’Donnell, P. (2015). Prefrontal cortical kappa opioid receptors attenuate responses to amygdala inputs. Neuropsychopharmacology 40, 2856–2864. doi: 10.1038/npp.2015.138
Thal, L. J., Laing, K., Horowitz, S. G., and Makman, M. H. (1986). Dopamine stimulates rat cortical somatostatin release. Brain Res. 372, 205–209. doi: 10.1016/0006-8993(86)91126-1
Thiele, T. E., Marsh, D. J., Marie, L. S., Bernstein, I. L., and Palmiter, R. D. (1998). Ethanol consumption and resistance are inversely related to neuropeptide Y levels. Nature 396, 366–369. doi: 10.1038/24614
Thiele, T. E., Miura, G. I., Marsh, D. J., Bernstein, I. L., and Palmiter, R. D. (2000). Neurobiological responses to ethanol in mutant mice lacking neuropeptide Y or the Y5 receptor. Pharmacol. Biochem. Behav. 67, 683–691. doi: 10.1016/s0091-3057(00)00413-5
Thierry, A. M., Gioanni, Y., Dégénétais, E., and Glowinski, J. (2000). Hippocampo-prefrontal cortex pathway: anatomical and electrophysiological characteristics. Hippocampus 10, 411–419. doi: 10.1002/1098-1063(2000)10:4<411::aid-hipo7>3.0.co;2-a
Thorsell, A. (2008). Central neuropeptide Y in anxiety- and stress-related behavior and in ethanol intake. Ann. N Y Acad. Sci. 1148, 136–140. doi: 10.1196/annals.1410.083
Tran, L., Schulkin, J., and Greenwood-Van Meerveld, B. (2014). Importance of CRF receptor-mediated mechanisms of the bed nucleus of the stria terminalis in the processing of anxiety and pain. Neuropsychopharmacology 39, 2633–2645. doi: 10.1038/npp.2014.117
Tripp, A., Kota, R. S., Lewis, D. A., and Sibille, E. (2011). Reduced somatostatin in subgenual anterior cingulate cortex in major depression. Neurobiol. Dis. 42, 116–124. doi: 10.1016/j.nbd.2011.01.014
Tsubomoto, M., Kawabata, R., Zhu, X., Minabe, Y., Chen, K., Lewis, D. A., et al. (2019). Expression of transcripts selective for gaba neuron subpopulations across the cortical visuospatial working memory network in the healthy state and schizophrenia. Cereb. Cortex 29, 3540–3550. doi: 10.1093/cercor/bhy227
Turnbull, A. V., and Rivier, C. (1997). Corticotropin-releasing factor (CRF) and endocrine responses to stress: CRF receptors, binding, protein and related peptides. Proc. Soc. Exp. Biol. Med. 215, 1–10. doi: 10.3181/00379727-215-44108
Urban-Ciecko, J., and Barth, A. L. (2016). Somatostatin-expressing neurons in cortical networks. Nat. Rev. Neurosci. 17, 401–409. doi: 10.1038/nrn.2016.53
Uribe-Mariño, A., Gassen, N. C., Wiesbeck, M. F., Balsevich, G., Santarelli, S., Solfrank, B., et al. (2016). Prefrontal cortex corticotropin-releasing factor receptor 1 conveys acute stress-induced executive dysfunction. Biol. Psychiatry 80, 743–753. doi: 10.1016/j.biopsych.2016.03.2106
Vale, W., Spiess, J., Rivier, C., and Rivier, J. (1981). Characterization of a 41-residue ovine hypothalamic peptide that stimulates secretion of corticotropin and β-endorphin. Science 213, 1394–1397. doi: 10.1126/science.6267699
Van De Werd, H. J. J. M., Rajkowska, G., Evers, P., and Uylings, H. B. M. (2010). Cytoarchitectonic and chemoarchitectonic characterization of the prefrontal cortical areas in the mouse. Brain Struct. Funct. 214, 339–353. doi: 10.1007/s00429-010-0247-z
van den Pol, A. N. (2012). Neuropeptide transmission in brain circuits. Neuron 76, 98–115. doi: 10.1016/j.neuron.2012.09.014
Van Eden, C. G., and Buijs, R. M. (2000). Functional neuroanatomy of the prefrontal cortex: autonomic interactions. Prog. Brain Res. 126, 49–62. doi: 10.1016/s0079-6123(00)26006-8
Van Pett, K., Viau, V., Bittencourt, J. C., Chan, R. K. W., Li, H. Y., Arias, C., et al. (2000). Distribution of mRNAs encoding CRF receptors in brain and pituitary of rat and mouse. J. Comp. Neurol. 428, 191–212. doi: 10.1002/1096-9861(20001211)428:2<191::aid-cne1>3.0.co;2-u
Vaughan, J., Donaldson, C., Bittencourt, J., Perrin, M. H., Lewis, K., Sutton, S., et al. (1995). Urocortin, a mammalian neuropeptide related to fish urotensin I and to corticotropin-releasing factor. Nature 378, 287–292. doi: 10.1038/378287a0
Vollmer, L. L., Schmeltzer, S., Schurdak, J., Ahlbrand, R., Rush, J., Dolgas, C. M., et al. (2016). Neuropeptide Y impairs retrieval of extinguished fear and modulates excitability of neurons in the infralimbic prefrontal cortex. J. Neurosci. 36, 1306–1315. doi: 10.1523/JNEUROSCI.4955-13.2016
Wall, P. M., and Messier, C. (2000). U-69,593 microinjection in the infralimbic cortex reduces anxiety and enhances spontaneous alternation memory in mice. Brain Res. 856, 259–280. doi: 10.1016/S0006-8993(99)01990-3
Wahlestedt, C., Ekman, R., and Widerlöv, E. (1989). Neuropeptide Y (NPY) and the central nervous system: distribution effects and possible relationship to neurological and psychiatric disorders. Prog. Neuropsychopharmacol. Biol. Psychiatry 13, 31–54. doi: 10.1016/0278-5846(89)90003-1
Wahlestedt, C., Karoum, F., Jaskiw, G., Wyatt, R. J., Larhammar, D., Ekman, R., et al. (1991). Cocaine-induced reduction of brain neuropeptide Y synthesis dependent on medial prefrontal cortex. Proc. Natl. Acad. Sci. U S A 88, 2078–2082. doi: 10.1073/pnas.88.6.2078
Wang, H. L., Bogen, C., Reisine, T., and Dichter, M. (1989). Somatostatin-14 and somatostatin-28 induce opposite effects on potassium currents in rat neocortical neurons. Proc. Natl. Acad. Sci. U S A 86, 9616–9620. doi: 10.1073/pnas.86.23.9616
Wang, S. C., Lin, C. C., Tzeng, N. S., Tung, C. S., and Liu, Y. P. (2019). Effects of oxytocin on prosocial behavior and the associated profiles of oxytocinergic and corticotropin-releasing hormone receptors in a rodent model of posttraumatic stress disorder. J. Biomed. Sci. 26:26. doi: 10.1186/s12929-019-0514-0
Wang, W., Xu, T., Chen, X., Dong, K., Du, C., Sun, J., et al. (2019). NPY receptor 2 mediates NPY antidepressant effect in the mPFC of LPS rat by suppressing NLRP3 signaling pathway. Mediators Inflamm. 2019:7898095. doi: 10.1155/2019/7898095
Wee, S., and Koob, G. F. (2010). The role of the dynorphin-κ opioid system in the reinforcing effects of drugs of abuse. Psychopharmacology 210, 121–135. doi: 10.1007/s00213-010-1825-8
Wevers, A., Schmidt, P., Cserpan, E., Cserpan, I., Maderspach, K., Staak, M., et al. (1995). Cellular distribution of the mRNA for the κ-opioid receptor in the human neocortex: a non-isotopic in situ hybridization study. Neurosci. Lett. 195, 125–128. doi: 10.1016/0304-3940(95)11797-z
Widdowson, P. S., Ordway, G. A., and Halaris, A. E. (1992). Reduced neuropeptide Y concentrations in suicide brain. J. Neurochem. 59, 73–80. doi: 10.1111/j.1471-4159.1992.tb08877.x
Wiersielis, K. R., Wicks, B., Simko, H., Cohen, S. R., Khantsis, S., Baksh, N., et al. (2016). Sex differences in corticotropin releasing factor-evoked behavior and activated networks. Psychoneuroendocrinology 73, 204–216. doi: 10.1016/j.psyneuen.2016.07.007
Wille-Bille, A., Miranda-Morales, R. S., Pucci, M., Bellia, F., D’Addario, C., and Pautassi, R. M. (2018). Prenatal ethanol induces an anxiety phenotype and alters expression of dynorphin and nociceptin/orphanin FQ genes. Prog. Neuropsychopharmacol. Biol. Psychiatry 85, 77–88. doi: 10.1016/j.pnpbp.2018.04.005
Witkowski, G., and Szulczyk, P. (2006). Opioid μ receptor activation inhibits sodium currents in prefrontal cortical neurons via a protein kinase A- and C-dependent mechanism. Brain Res. 1094, 92–106. doi: 10.1016/j.brainres.2006.03.119
Wu, G., Feder, A., Wegener, G., Bailey, C., Saxena, S., Charney, D., et al. (2011). Central functions of neuropeptide y in mood and anxiety disorders. Expert Opin. Ther. Targets 15, 1317–1331. doi: 10.1517/14728222.2011.628314
Yamashita, A., Hayashi, M., Shimizu, K., and Oshima, K. (1989). Ontogeny of somatostatin in cerebral cortex of macaque monkey: an immunohistochemical study. Dev. Brain Res. 45, 103–111. doi: 10.1016/0165-3806(89)90012-6
Yarur, H. E., Vega-Quiroga, I., González, M. P., Noches, V., Thomases, D. R., Andrés, M. E., et al. (2020). Inhibitory control of basolateral amygdalar transmission to the prefrontal cortex by local corticotrophin type 2 receptor. Int. J. Neuropsychopharmacol. 23, 108–116. doi: 10.1093/ijnp/pyz065
Yu, G., Yan, H., and Gong, Z.-H. (2012). Differential effects of acute and repeated morphine treatment on κ-opioid receptor mRNA levels in mesocorticolimbic system. Pharmacol. Rep. 64, 445–448. doi: 10.1016/s1734-1140(12)70786-7
Yu, G., Yan, H., and Gong, Z. H. (2014). Effects of acute and repeated morphine treatment on κ-opioid receptor protein levels in mesocorticolimbic system. J. Recept. Signal Transduct. Res. 34, 44–47. doi: 10.3109/10799893.2013.856919
Zamir, N., Palkovits, M., and Brownstein, M. J. (1984a). Distribution of immunoreactive dynorphin A1–8 in discrete nuclei of the rat brain: comparison with dynorphin A. Brain Res. 307, 61–68. doi: 10.1016/0006-8993(84)90460-8
Zamir, N., Palkovits, M., Weber, E., and Brownstein, M. J. (1984b). Distribution of immunoreactive dynorphin B in discrete areas of the rat brain and spinal cord. Brain Res. 300, 121–127. doi: 10.1016/0006-8993(84)91346-5
Zieba, B., Grzegorzewska, M., Brański, P., Domin, H., Wierońska, J. M., Hess, G., et al. (2008). The behavioural and electrophysiological effects of CRF in rat frontal cortex. Neuropeptides 42, 513–523. doi: 10.1016/j.npep.2008.05.004
Zorrilla, E. P., Logrip, M. L., and Koob, G. F. (2014). Corticotropin releasing factor: a key role in the neurobiology of addiction. Front. Neuroendocrinol. 35, 234–244. doi: 10.1016/j.yfrne.2014.01.001
Keywords: prefrontal cortex, peptides, animal models, circuitry, neurons, animal behavior
Citation: Brockway DF and Crowley NA (2020) Turning the ′Tides on Neuropsychiatric Diseases: The Role of Peptides in the Prefrontal Cortex. Front. Behav. Neurosci. 14:588400. doi: 10.3389/fnbeh.2020.588400
Received: 28 July 2020; Accepted: 09 September 2020;
Published: 20 October 2020.
Edited by:
Jessica R. Barson, Drexel University, United StatesReviewed by:
Sofiya Hupalo, National Institutes of Health (NIH), United StatesMax Joffe, Vanderbilt University, United States
Copyright © 2020 Brockway and Crowley. This is an open-access article distributed under the terms of the Creative Commons Attribution License (CC BY). The use, distribution or reproduction in other forums is permitted, provided the original author(s) and the copyright owner(s) are credited and that the original publication in this journal is cited, in accordance with accepted academic practice. No use, distribution or reproduction is permitted which does not comply with these terms.
*Correspondence: Nicole A. Crowley, bnpjMjdAcHN1LmVkdQ==