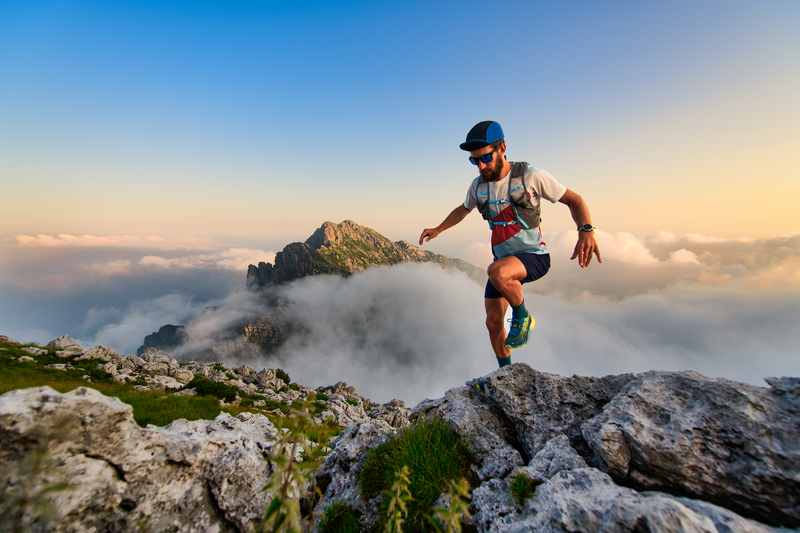
94% of researchers rate our articles as excellent or good
Learn more about the work of our research integrity team to safeguard the quality of each article we publish.
Find out more
ORIGINAL RESEARCH article
Front. Behav. Neurosci. , 19 September 2019
Sec. Pathological Conditions
Volume 13 - 2019 | https://doi.org/10.3389/fnbeh.2019.00214
This article is part of the Research Topic Pathological Conditions - Editor’s Pick 2021 View all 10 articles
Reelin (Reln) is an extracellular glycoprotein that is important for brain patterning. During development Reln coordinates the radial migration of postmitotic cortical neurons, cerebellar and hippocampal neurons, whereas it promotes dendrite maturation, synaptogenesis, synaptic transmission, plasticity and neurotransmitter release in the postnatal and adult brain. Genetic studies of human patients have demonstrated association between the RELN locus and autism spectrum disorder, schizophrenia, bipolar disorder, and Alzheimer’s disease. In this study we have characterized the behavioral phenotype of reelin (reln) mutant zebrafish, as well as two canonical signaling pathway targets DAB adaptor protein 1a (dab1a) and the very low density lipoprotein receptor (vldlr). Zebrafish reln–/– mutants display a selective reduction in preference for social novelty that is not observed in dab1a–/– or vldlr–/– mutant lines. They also exhibit an increase in 5-HT signaling in the hindbrain that parallels but does not underpin the alteration in social preference. These results suggest that zebrafish reln–/– mutants can be used to model some aspects of human diseases in which changes to Reln signaling alter social behavior.
Reelin (Reln) is an extracellular glycoprotein that is important for brain patterning and synaptogenesis. During mammalian development Reln is secreted by Cajal-Retzius (C-R) neurons in cerebral cortex and granule cells in the external granule cell layer of the cerebellum (Pesold et al., 1998; Lee and D’Arcangelo, 2016). In the adult brain Reln is expressed by γ-aminobutyric acid (GABA)-positive interneurons in the cortex and hippocampus, and glutamatergic granule cells in the cerebellum (Pesold et al., 1998). The mammalian neocortex develops in an inside-out manner in which late-born neurons migrate past existing neurons to form a laminar structure (Rakic, 2009). Reln coordinates the radial migration of postmitotic cortical neurons, cerebellar and hippocampal neurons (D’Arcangelo, 2014). Reeler mice that lack Reln function display a disorganization of cortical lamination and cerebellar hypoplasia caused by the failure of Purkinje neurons to migrate (Goffinet, 1983; Miyata et al., 1997). Similar abnormalities have been identified in human patients with homozygous null mutations in RELN, who display lissencephaly and cerebellar hypoplasia (Hong et al., 2000). In the mature brain, RELN promotes dendrite maturation, synaptogenesis, synaptic transmission, plasticity and the release of neurotransmitters (D’Arcangelo, 2005; Herz and Chen, 2006; Levenson et al., 2008; Förster et al., 2010; Levy et al., 2014).
Reln signaling is transduced by several downstream pathways including two low-density lipoprotein receptor family members: apolipoprotein E receptor 2 (ApoER2, also called low-density lipoprotein receptor-related protein 8, LRP8) and the very low density lipoprotein receptor (Vldlr) (Trommsdorff et al., 1999). ApoER2 and Vldlr are expressed on the membrane of target neurons. Their activation leads to internalization of Reln in endocytic vesicles, phosphorylation of the DAB adapter protein 1 (Dab1; Howell et al., 1997; Sheldon et al., 1997) and activation of Src/Fyn kinases as part of canonical Reln signaling (D’Arcangelo, 2006; Bock and May, 2016). ApoER2 and VLDLR have partially redundant functions and double Apoer2/Vldlr knock-out mice exhibit a reeler-like neuroanatomical phenotype (Trommsdorff et al., 1999). However, they also play specific roles in neuronal migration. ApoER2 is mostly expressed in hippocampal and cortical neurons and single Apoer2 knock-out mice show defects in forebrain structures. Vldlr is mostly expressed in the Purkinje cells of the cerebellum, and these neurons fail to assemble in a tight layer in Vldlr knock-out mice (Trommsdorff et al., 1999). Other signaling pathways regulated by Reln include CrK/Rap1 (neuronal migration and lamination) and the P13K/Akt/mTOR pathway (dendrite and spine development). Activity at NMDA receptors and the MEK/Erk1/2 pathway enhances the expression of synaptic plasticity and learning genes (Chen et al., 2010).
Animal studies further support a link between decreased Reln signaling and behavioral abnormalities. Both homozygous and heterozygous reeler mice exhibit behavioral and cognitive defects (Tueting et al., 1999; Costa, 2002; Qiu et al., 2006). Reln+/– mice show impaired prepulse inhibition, a sensory motor gaiting phenotype associated with schizophrenia (Tueting et al., 1999; Barr et al., 2008; Kutiyanawalla et al., 2011; Teixeira et al., 2011) although not all studies agree (Qiu et al., 2006; Teixeira et al., 2011). Reln+/– mice also exhibit neophobia in the elevated plus maze, a decrease in cortical dendritic spine density and defects in hippocampus-based learning (Qiu et al., 2006), long-term potentiation and long-term depression (Iafrati et al., 2013). Cross-modal prepulse inhibition is increased in Apoer2–/– knock-out mice and decreased in Vldlr–/– (Weeber et al., 2002); and Dab1-conditional knockout mice exhibit hyperactivity and deficits in anxiety and working memory (Imai et al., 2016).
Abnormal Reln signaling has also been linked to several human psychiatric disorders. Genetic studies have demonstrated an association between the RELN locus and autism spectrum disorder (ASD; Wang et al., 2014), schizophrenia (Ovadia and Shifman, 2011; Li et al., 2015), bipolar disorder (Ovadia and Shifman, 2011), and Alzheimer’s disease (Bufill et al., 2013). In humans, RELN is located at chromosome 7q22, the peak region of linkage and first autism susceptibility locus (AUTS1). Linkage and epidemiologic studies have yielded both positive and negative findings as to whether RELN plays a major role in ASD susceptibility (Persico et al., 2001; Krebs et al., 2002; Zhang et al., 2002; Bonora et al., 2003; Li et al., 2004; Dutta et al., 2006, 2008; Serajee et al., 2006; Li et al., 2008; He et al., 2011). Meta-analysis of several studies has identified a single nucleotide polymorphism that segregates with ASD (Wang et al., 2014) and unique inherited and de novo Reelin variants have been uncovered by exome sequencing of ASD patients (Bonora et al., 2003; Neale et al., 2012; Koshimizu et al., 2013; De Rubeis et al., 2014; Yuen et al., 2015; Zhang et al., 2015). Further evidence for a role of RELN in the etiology of ASD are the reduction of Reelin levels in the cerebellum, frontal cortices and blood of ASD patients (Fatemi, 2001; Fatemi et al., 2005); a reduction of RELN and DAB1 mRNA levels; and an increase in VLDLR mRNA levels (Fatemi et al., 2005).
In this study we have characterized the behavior of three zebrafish lines with mutations in Reln signaling pathway components: reelin, dab1a and vldlr (Di Donato et al., 2018). We hypothesized that reduced Reln signaling would decrease social interaction in zebrafish (including shoaling, social preference and aggression) with parallel alterations to monoamine neurotransmitter signaling. Our results demonstrate that reln–/– mutants display a selective decrease in preference for social novelty that is not observed in dab1a–/– or vldlr–/– mutant lines.
Zebrafish (Danio rerio) were kept at the University of Leicester in accordance with institute animal welfare guidelines. The lighting conditions were 14:10 h (light:dark). Fish were fed twice per day with ZEBRAFEED 400–600 dry food (Sparos). Groups of 15 fish were kept in 3.5 l tanks (Tecniplast). All experiments were approved by a local Animal Welfare and Ethical Review board and were covered by a UK Home Office license to Will Norton. The following strains were used: heterozygous and homozygous reelinΔ28–/– mutants; DAB adaptor protein 1aΔ22–/– mutants; very low density lipoprotein receptor+13–/– mutants (Di Donato et al., 2018); and AB wild-type (WT) zebrafish.
Behavior was recorded using FlyCapture2 2.5.2.3 software and a digital camera (Point Grey Research). All behavioral experiments were carried out between 11:00 and 17:00. Experiments were performed in a dedicated room with light and temperature kept constant. Zebrafish were moved to the behavior room in holding tanks on the same day as the analysis. Fish were allowed to habituate to the testing room for 1 h. Previous research in our laboratory has demonstrated no sex differences in behavior in our recording setups (e.g., Norton et al., 2011). We therefore used mixed groups of male and female adult zebrafish (3–6 months old) in our analyses. The age and size of fish were carefully matched between genotypes. The sample size (n) for each animal group was calculated based upon power analysis of previous behavioral experiments carried out in our laboratory (e.g., Norton et al., 2011, 2019; Carreno Gutierrez et al., 2017). We only used one experimental setup for each behavioral test, but we cleaned the setup between recordings and changed the water in the tank for each fish tested. Ethovision XT (Noldus) software was used for video tracking. Each film was analyzed by two researchers blind to the genotype or treatment. This removes observer bias in manual quantification.
Anxiety-like behavior and exploratory activity was recorded in the novel tank test (NTT) which was performed in a 1.5 L trapezoid tank (Egan et al., 2009). Fish were recorded in this setup for 5 min. We analyzed the time spent in the bottom (geotaxis) and the total distance swum. For reln: n = 13 WT, n = 13 reln+/–, n = 12 reln–/–. For dab1a: n = 10 WT, n = 7 dab1a–/–. For vldlr: n = 7 WT, n = 7 vldlr–/–.
The open field test was carried out in a open tank (40 × 25 cm) filled with water to a depth of 8 cm. Single adult zebrafish were filmed from above for 5 min. The total distance swum, duration of thigmotaxis (time spent at a distance of 2 cm or less from the walls) and time in the center of the tank (representing half of the total tank area) were quantified. For reln: n = 12 WT, n = 12 reln+/–, n = 12 reln–/–. For dab1a: n = 10 WT, n = 7 dab1a–/–. For vldlr: n = 8 WT, n = 7 vldlr–/–.
Shoaling was recorded in tanks measuring 43 × 22 cm filled with water to a depth of 8 cm. Groups of five familiar adult fish were introduced to the tank, allowed to acclimatize and recorded from above for 10 min (Parker et al., 2013). A mix of males and females were examined. We tracked the fish and measured the average inter-individual distance, polarization and speed of locomotion using VpCore2 software (ViewPoint Life Sciences). n = 2 groups of 5 WT, n = 2 groups of 5 reln+/– and n = 2 groups of 5 reln–/–.
The social preference test was carried out as described in Carreno Gutierrez et al. (2019). We used a transparent plastic tank containing five compartments: a central area (13 × 19 cm) with two small 6.5 × 9 cm areas either side. The walls separating the central and the side compartments contained holes (1 mm) to allow movement of water and odorants. A single fish was introduced into the central area and permitted to interact with a group of three fish placed in one of the side compartments. To analyze the interactions the central arena was divided in four equal sections and the time spent by the focal fish in each area was recorded. Social preference. In the first session (interaction 1) a group of three unfamiliar WT fish (1st strangers) were placed into one of the side compartments. The behavior of the focal fish was recorded for 5 min, and the time spent closest to the 1st group of strangers was compared to the time spent near the empty area diagonally opposite. Preference for social novelty. A second group of three unfamiliar WT fish (2nd strangers) were placed in the compartment diagonally opposite the first group. The focal fish was recorded for a further 5 min. The time in the area nearest the 1st strangers and the time spent in the quadrant nearest the 2nd strangers was compared. We used a mixture of size-matched males and females as stimuli since can attract both male and female zebrafish (Ruhl et al., 2009). The stimulus fish were changed after recording three focal fish, to standardize the stimulus used and reduce the amount of stress that each animal was exposed to. For reln: n = 12 WT, n = 12 reln+/–, n = 10 reln–/–. For dab1a: n = 10 WT, n = 7 dab1a–/–. For vldlr: n = 8 WT, n = 7 vldlr–/–.
Aggression was measured using a mirror as a stimulus (Norton et al., 2011). Fish were recorded for 5 min from above. The time spent being aggressive (biting the mirror image and thrashing the tail fin) was quantified manually using LabWatcher (ViewPoint Life Sciences). Films were renamed so that the observer was blind to the genotype being analyzed. For reln: n = 12 WT, n = 12 reln+/–, n = 8 reln–/–. For dab1a: n = 10 WT, n = 7 dab1a–/–. For vldlr: n = 8 WT, n = 7 vldlr–/–.
Buspirone hydrochloride was bought from Tocris (Cat. no. 0962), oxytocin was purchased from Sigma Aldrich (Cat. no. O3251) and risperidone was purchased from Tocris (Cat. no. 2865). Administration and concentration of the treatments were chosen according to previously published studies. Buspirone was applied by immersion in water containing 10 mg/L drug for 1 h (Bencan et al., 2009). Oxytocin was applied at a concentration of 10 ng/kg by intraperitoneal injection 30 min before recording (Zimmermann et al., 2016). Risperidone was applied by immersion in water containing 170 μg/L drug for 15 min (Idalencio et al., 2015). For buspirone: n = 8 WT, n = 8 reln–/–. For oxytocin: n = 9 WT, n = 9 reln–/–. For risperidone: n = 9 WT, n = 9 reln–/–.
The anti-Reelin antibody was purchased from Millipore (Cat. no. MAB5366). Immunohistochemistry labeling was carried out using the following protocol. Dissected brains were fixed in 4% PFA for 24 h at 4°C. Brains were washed in phosphate buffered saline (PBS) and stored in methanol at −20°C until processing. Coronal and sagittal sections (100 μM) were cut using a Leica VT1000 S vibratome (Leica Biosystems). After blocking in PBS with 5% normal goat serum (Sigma Cat. no. G9023), 1% dimethyl sulphoxide (Sigma, Cat. no. 276855) and 0.2% Triton X-100 (Fisher, Cat. no. 10254640), sections were incubated in primary antibody for 24 h at 4°C. The secondary antibody (Biotinylated Universal Antibody anti-mouse and rabbit IgG (H + L); Vector Laboratories Cat. no. BA-1400) was incubated for 2 h at room temperature and staining was developed using diaminobenzidine (DAB). Stained sections were mounted and then photographed using an optical microscope (GXM L3200B, GT Vision) and images were assembled in Adobe Photoshop version CS2 (Adobe systems).
HPLC was performed as described in Carreno Gutierrez et al. (2017). Fish were sacrificed using a schedule 1 procedure. Dissected brains were divided into telencephalon, diencephalon, optic tectum and hindbrain. Samples were prepared in 100 μl ice-cold 0.1 N perchloric acid and centrifuged. HPLC with electrochemical detection was used to measure dopamine (DA), serotonin (5-HT), 3,4-dihydroxyphenylacetic acid (DOPAC), homovanillic acid (HVA) and 5-hydroxyindoleacetic acid (5-HIAA). Samples were compared to standard neurotransmitter solutions and the results were expressed as pmol/mg of brain. 8 reln–/– and 8 AB brains were processed for HPLC.
All data were stored in Excel (Microsoft). Statistical analysis was performed in GraphPad Prism7. Bars represent average values and error bars denote standard error of the mean (SEM). The distribution of the data was verified before choosing an appropriate statistical test, using either the D’Agostino-Pearson or Shapiro–Wilk normality test. A Student’s t-test (with Holm–Sidak correction) or Mann–Whitney U-test (with Welch correction if appropriate) was used to compare two data sets. When comparing three groups, a one-way ANOVA followed by a Dunnett’s post hoc test was used. For non-parametric data a Kruskal–Wallis test followed by Dunn post hoc was used. Three-way ANOVA followed by Sidak’s post hoc test for multiple comparisons was used to analyze the drug treatment experiments, and two-way ANOVA for the social interaction tests as described in the figure legends. The Statistical significance was shown as follows: ∗p < 0.05, ∗∗p < 0.01, ∗∗∗p < 0.001, ****p < 0.0001. The number of animals used is denoted by n in the figure legends.
We first examined the location of Reelin (Reln) protein in the adult zebrafish brain. In agreement with other published studies, Reln was expressed in a restricted pattern in the adult zebrafish brain, including areas that are important for synaptic organization and plasticity (Costagli et al., 2002). In WT, Reln protein is detected in the medial part of the dorsal telencephalon (Dm) and the ventral nucleus of the ventral telencephalon (Vv, Figure 1a). In the diencephalon, Reelin is found in the ventral thalamic nuclei (Th, Figure 1b) and in the ventral hypothalamic nuclei (Hy, Figure 1c). In the midbrain Reelin is detected in the torus longitudinalis (TL, Figure 1d) as well as the stratum fibrosum marginale (sfm) and stratum opticum (so, Figure 1e) layers of the tectal neuropil. A few labeled cells were observed in the interpeduncular nucleus (nin, Figure 1f). In the hindbrain Reelin is localized to three areas of the cerebellum: the granular cell layer of the corpus cerebelli (CCe), the caudal lobe (LCa) and the crista cerebellaris (CC, Figure 1g). No Reelin expression is observed in the Purkinje and molecular cell layer. Scattered nuclei expressing Reelin are also seen in the intermediate and inferior part of the reticula formation (RF) of the medulla oblongata (Figure 1h). As expected, there is a strong reduction in the level of Reln protein detected in reln–/– mutant zebrafish (Figures 1i,j), confirming the specificity of our immunohistochemical analysis.
Figure 1. Reelin immunohistochemistry. Anti-Reelin antibody labeling of coronal and sagittal sections of the adult zebrafish brain. In wild-type anti-Reelin antibody labeling is seen in the (a) medial part of the dorsal telencephalon (Dm) and in the ventral nucleus of the ventral telencephalon (Vm), in the (b) ventral thalamic nuclei (Th) and (c) the ventral hypothalamic nuclei (Hy). Labeling is also detected in the (d) torus longitudinalis (TL), (e) stratum fibrosum marginale (sfm) and the stratum opticum (so) of the optic tectum. (f) A few labeled cells are observed in the interpeduncular nucleus (nin). In the hindbrain, Reelin protein localizes to the (g) corpus cerebelli (CCe), the lobus caudalis cerebelli (LCa), crista cerebellaris (CC) and (h) the intermediate and inferior part of the reticular formation (RF). (i,j) Anti-Reelin antibody labeling is not detected in the brain of reln–/– mutants. Black arrowheads show position of Reelin-positive areas in (b,c,f).
The well-established links between Reelin and social behavior prompted us to measure shoaling and social preference in reln mutants. We examined social interaction and discrimination in the social preference. We introduced a focal WT or mutant fish in the central compartment of the social preference tank and measured its interaction with a group of unfamiliar WT stimulus fish (1st strangers) (Figure 2C). WT, reln+/– and reln–/– spent more time next to the strangers than in the empty control area [Figure 2A; p < 0.0001 for all genotypes; two-way ANOVA followed by Sidak’s post hoc, genotype factor: F(2,62) = 1.818, p = 0.17, stranger factor: F(1,62) = 2392, p < 0.0001, interaction genotype × stranger: F(2,62) = 4.418, p = 0.02]. We assessed social novelty preference by placing a group of unfamiliar fish (2nd strangers) into the setup (Figure 2D). Although both WT and reln+/– changed their preference and spent an equal amount of time interacting with both groups of fish (Figure 2B; p = 0.99 for WT and p = 0.38 for reln+/–), reln–/– mutants failed to switch preference and remained with the first group of strangers [Figure 2B; p < 0.0001; two-way ANOVA followed by Sidak’s post hoc, genotype factor: F(2,62) = 0.5184, p = 0.60, stranger factor: F(1,62) = 21.49, p < 0.0001, interaction genotype × stranger: F(2,62) = 6.886, p = 0.002]. We next recorded shoaling in groups of WT or mutants. We placed 5 WT or reln mutants into a large tank and measured the inter-individual distance. In contrast to the social preference test, reln–/– displayed a similar inter-individual distance (Kruskal–Wallis test with Dunn’s multiple comparisons test: chi-square = 3.71, p = 0.36), polarization (Kruskal–Wallis test with Dunn’s multiple comparisons test: chi-square = 0, p > 0.99) and velocity [one-way ANOVA Dunnett’s multiple comparisons test: F(2,3) = 5.498, p = 0.07) as WT when interacting with a group of conspecifics (Figures 3A–C)]. Taken together, these results suggest that reln–/– mutants display a selective reduction in their preference for social novelty in the absence of global changes to social interactions.
Figure 2. Social preference test. (A) Social preference. WT, reln+/– and reln–/– show a significant preference to spend time near a group of unfamiliar fish [1st strangers; p < 0.0001 for all genotypes; two-way ANOVA followed by Sidak’s post hoc, genotype factor: F(2,62) = 1.818, p = 0.17, stranger factor: F(1,62) = 2392, p < 0.0001, interaction genotype × stranger: F(2,62) = 4.418, p = 0.02]. (B) Preference for social novelty. Both WT and reln+/– spend an equal amount of time near both groups of unfamiliar fish (1st and 2nd strangers; p = 0.99 for WT and p = 0.38 for reln+/–). reln–/– do not switch preference to the second group of unfamiliar fish [2nd strangers; p < 0.0001; two-way ANOVA followed by Sidak’s post hoc, genotype factor: F(2,62) = 0.5184, p = 0.60, stranger factor: F(1,62) = 21.49, p < 0.0001, interaction genotype × stranger: F(2,62) = 6.886, p = 0.002]. n = 12 wild-type, n = 12 reln+/– and n = 10 reln–/–. ****p < 0.0001. Mean ± SEM. (C,D) Photographs showing the experimental setup for social preference (C) and preference for social novelty (D).
Figure 3. Behavior of reln+/– and reln–/– zebrafish. (A–C) Both genotypes shoal normally. (A) Inter-individual distance (Kruskal–Wallis test with Dunn’s multiple comparisons test: chi-square = 3.71, p > 0.99 for reln+/– and p = 0.36 for reln–/–); (B) Polarization (Kruskal–Wallis test with Dunn’s multiple comparisons (test: chi-square = 0, p > 0.99 for both genotypes) and (C) velocity [one-way ANOVA Dunnett’s multiple comparisons test: F(2,3) = 5.498, p = 0.09 for WT, p = 0.31 for reln+/– and p = 0.07 for reln–/–]. n = 2 groups of 5 WT, n = 2 groups of 5 reln+/– and n = 2 groups of 5 reln–/–. (D–F) Both reln+/– and reln–/– behave similarly to WT in the open field test. (D) Time at the side of the tank [thigmotaxis, one-way ANOVA Dunnett’s multiple comparisons test: F(2,33) = 1,687, p = 0.20, p = 0.13 for WT vs. reln+/– and p = 0.50 for WT vs. reln–/–]; (E) time spent in the center of the tank [one-way ANOVA Dunnett’s multiple comparisons test: F(2,33) = 1,431, p = 0.25 for WT, p = 0.25 for reln+/– and p = 0.99 for reln–/–]. (F) Locomotion is not affected in the open field test [one-way ANOVA Dunnett’s multiple comparisons test: F(2,33) = 1,625, p = 0.21, p = 0.14 for reln+/– and p = 0.61 for reln–/–]. n = 12 WT, n = 12 reln+/–, n = 12 reln–/–. (G,H) Both genotypes exhibit normal anxiety-like behavior. (G) Time at the bottom of a novel tank (Kruskal–Wallis test with Dunn’s multiple comparisons test: chi-square = 0,5705, p = 0.93 for reln+/– and p > 0.99 for reln–/–); (H) locomotion in a novel tank [one-way ANOVA Dunnett’s multiple comparisons test: F(2,35) = 1,54, p = 0.23 for WT, p = 0.75 for reln+/– and p = 0.43 for reln–/–) n = 13 wild-type, n = 13 reln+/–, n = 12 reln–/–. (I) No difference in aggression levels between WT, reln+/– and reln–/– (Kruskal–Wallis test with Dunn’s multiple comparisons test: chi-square = 3,372, p = 0.97 for reln+/– and p = 0.13 for reln–/–) n = 12 wild-type, n = 12 reln+/–, n = 8 reln–/–. Mean ± SEM.)
We examined the selectivity of this behavioral phenotype by measuring changes to exploration, anxiety-like behavior and aggression in reln–/– mutant zebrafish. In the open field test, both WT and reln–/– spent a similar amount of time at the side [Figure 3D; thigmotaxis, p = 0.13 for WT vs. reln+/– and p = 0.50 for WT vs. reln–/–; one-way ANOVA with Dunnett’s multiple comparisons test: F(2,33) = 1.687, p = 0.20] and in the center of the tank [Figure 3E; p = 0.25 for reln+/– and p = 0.99 for reln–/–; one-way ANOVA with Dunnett’s multiple comparisons test: F(2,33) = 1.431, p = 0.25]. They also swam a similar distance suggesting that exploration is not altered in mutant fish [Figure 3F; p = 0.14 for reln+/– and p = 0.61 for reln–/–; one-way ANOVA with Dunnett’s multiple comparisons test: F(2,33) = 1.625, p = 0.21]. reln–/– also exhibited normal anxiety-like behavior in the NTT. They spent a similar amount of time at the bottom of the tank (Figure 3G; Kruskal–Wallis test with Dunn’s multiple comparisons test: chi-square = 0.5705, p = 0.93 for reln+/– and p > 0.99 for reln–/–) and swam a similar distance [Figure 3H; p = 0.75 for reln+/– and p = 0.43 for reln–/–; one-way ANOVA with Dunnett’s multiple comparisons test: F(2,35) = 1.54, p = 0.23 as WT]. Both genotypes also spent a similar amount of time being aggressive in a mirror test, quantified as the amount of time spent biting the mirror image and thrashing the caudal fin (Figure 3I; Kruskal–Wallis test with Dunn’s multiple comparisons test: chi-square = 3,372, p = 0.97 for reln+/– and p = 0.13 for reln–/–). Taken together, these results suggest that reln–/– mutants exhibit a selective reduction in the reaction to social novelty.
Reelin activity is transduced by several downstream pathways including canonical signaling via DAB adaptor protein 1 and the Very low density lipoprotein receptor (Vldlr) (Trommsdorff et al., 1999; Bock and May, 2016). We characterized dab1a–/– (one of the zebrafish homologs of mammalian Dab1) and vldlr–/– mutant lines to examine the contribution of canonical signaling to the phenotype of reln–/– zebrafish. Interestingly, both dab1a–/– and vldlr–/– displayed different behavioral profiles. In the social preference test, dab1a–/– showed a similar social preference [Figure 4A; WT, p = 0.0005 and dab1a–/–, p < 0.0001; two-way ANOVA followed by Sidak’s post hoc, genotype factor: F(1,30) = 0.9841, p = 0.3291, stranger factor: F(1,30) = 72.81 p < 0.0001, interaction genotype × stranger: F(1,30) = 7.716 p = 0.0093] and reaction to social novelty as WT [Figure 4B; p = 0.61 for WT and p = 0.44 for dab1a–/–; two-way ANOVA followed by Sidak’s post hoc, genotype factor: F(1,30) = 0.004, p = 0.95, stranger factor: F(1,30) = 0.1016, p = 0.75, interaction genotype × stranger: F(1,30) = 2.175, p = 0.15]. Exploration in the open field test was similar in dab1a–/– and WT [Figures 4C–E; thigmotaxis, t-test (Welch): t(0.3262) = 9.971, p = 0.75; time in center of the tank, t-test (Welch): t(0.1684) = 14.64, p = 0.87 and locomotion, t-test (Welch): t(0.5277) = 13.05, p = 0.61]. Both genotypes also spent a similar amount of time at the bottom of a novel tank (Figure 4F; Mann–Whitney test: U = 17, p = 0.06), a readout of anxiety-like behavior, whereas mutants were hyperactive compared to WT [Figure 4G; t-test (Welch): t(3.234) = 7.409, p = 0.01]. Finally, dab1a–/– mutants were more aggressive than WT in the mirror aggression test (Figure 4H; Mann–Whitney test: U = 11.5, p = 0.0445).
Figure 4. Behavior of dab1a–/– zebrafish. (A,B) Social preference test. (A) Both genotypes prefer to spend time near a group of unfamiliar fish [1st strangers; p = 0.0005 and p < 0.0001 respectively; two-way ANOVA followed by Sidak’s post hoc, genotype factor: F(1,30) = 0.9841, p = 0.3291, stranger factor: F(1,30) = 72.81, p < 0.0001, interaction genotype × stranger: F(1,30) = 7.716, p = 0.0093]. (B) WT and dab1a–/– spend equal time near both groups when a second unfamiliar group is added [p = 0.61 for WT and p = 0.44 for dab1a–/–; two-way ANOVA followed by Sidak’s post hoc, genotype factor: F(1,30) = 0.004, p = 0.95, stranger factor: F(1,30) = 0.1016, p = 0.75, interaction genotype × stranger: F(1,30) = 2.175, p = 0.15. n = 10 wild-type and n = 7 dab1a–/–]. (C–E) dab1a–/– exhibit normal behavior in the open field test. (C) Time at side of the tank [thigmotaxis, t-test (Welch): t(0.3262) = 9.971, p = 0.75] and (D) time in center of the tank [t-test (Welch): t(0.1684) = 14.64, p = 0.87] (E) locomotion [t-test (Welch): t(0.5277) = 13.05, p = 0.61. n = 10 wild-type and n = 7 dab1a–/–]. (F) dab1a–/– exhibit normal anxiety-like behavior. Time at the bottom of novel tank (Mann–Whitney test: U = 17, p = 0.06). (G) dab1a–/– fish are more active than WT in the NTT [t-test (Welch): t(3.234) = 7.409, p = 0.0133. n = 10 wild-type and n = 7 dab1a–/–]. (H) dab1a–/– display heightened aggression levels compared to WT (Mann–Whitney test: U = 11.5, p = 0.0445. n = 10 wild-type and n = 7 dab1a–/–). ∗p < 0.05, ∗∗∗p < 0.001, ****p < 0.0001. Mean ± SEM.
vldlr–/– mutants also showed both a similar interaction with the first group of strangers [Figure 5A; p < 0.0001 for both genotypes; two-way ANOVA followed by Sidak’s post hoc, genotype factor: F(1,24) = 0.0039, p = 0.95, stranger factor: F(1,24) = 120.1, p < 0.0001, interaction genotype × stranger: F(1,24) = 0.4912, p = 0.49] and a normal reaction to social novelty [Figure 5B; p = 0.58 for WT and p = 0.73 for vldlr–/–; two-way ANOVA followed by Sidak’s post hoc, genotype factor: F(1,24) = 1.353, p = 0.26, stranger factor: F(1,24) = 0.005, p = 0.94, interaction genotype × stranger: F(1,24) = 0.02779, p = 0.87] as WT in the social preference test. In the open field test, vldlr–/– spent the same time at side of the tank [Figure 5C; t-test (Welch): t(1.581) = 9.068, p = 0.15] compared to WT but vldlr–/– mutants spent more significantly more time in the center of an open field tank than WT [Figure 5D; t-test (Welch): t(2.595) = 9.47, p = 0.03]. However, the distance swum in the open field was the same as WT [Figure 5E; t-test (Welch): t(0.3779) = 12.84, p = 0.71] suggesting that vldlr–/– are more explorative or less anxious than WT without being hyperactive. Anxiety-like behavior in the NTT was not altered in mutants [Figures 5F,G; time at bottom of tank, Mann–Whitney test: U = 16, p = 0.32 and locomotion, t-test (Welch): t(0.1803) = 10.92, p = 0.86]. Finally, mutants also exhibited a trend decrease in aggression levels that did not reach significance [Figure 5H; t-test (Welch): t(1.87) = 11.41, p = 0.09]. In summary, although mutation of reln leads to a specific alteration in social interactions, this phenotype does not appear to be mediated by the canonical Reln signaling components dab1a and vldlr, which display different behavioral alterations.
Figure 5. Behavior of vldlr–/– zebrafish. (A,B) Social preference test. (A) Both genotypes prefer to spend time near a group of unfamiliar fish [1st strangers; p < 0.0001 for both genotypes; two-way ANOVA followed by Sidak’s post hoc, genotype factor: F(1,24) = 0.0039, p = 0.95, stranger factor: F(1,24) = 120.1, p < 0.0001, interaction genotype × stranger: F(1,24) = 0.4912, p = 0.49]. (B) WT and vldlr–/– spend equal time near both groups when a second unfamiliar group is added [p = 0.58 for WT and p = 0.73 for vldlr–/–; two-way ANOVA followed by Sidak’s post hoc, genotype factor: F(1,24) = 1.353, p = 0.26, stranger factor: F(1,24) = 0.005, p = 0.94, interaction genotype × stranger: F(1,24) = 0.02779, p = 0.87. n = 8 wild-type and n = 7 vldlr–/–]. (C–E) vldlr–/– behavior in the open field test. (C) vldlr–/– spent the same time at side of the tank [t-test (Welch): t(1.581) = 9.068, p = 0.15] compared to WT but more (D) time in center of the tank [t-test (Welch): t(2.595) = 9.47, p = 0.03]. (E) Locomotion [t-test (Welch): t(0.3779) = 12.84, p = 0.71. n = 8 wild-type and n = 7 vldlr–/–]. (F) vldlr–/– exhibit normal anxiety-like behavior. Time at the bottom of novel tank (Mann Whitney test: U = 16, p = 0.32), (G) locomotion in a novel tank [t-test (Welch): t(0.1803) = 10.92, p = 0.86. n = 7 wild-type and n = 7 vldlr–/–]. (H) No difference in aggression levels between WT and vldlr–/– [t-test (Welch): t(1.87) = 11.41, p = 0.09. n = 8 WT and n = 7 vldlr–/–]. ∗p < 0.05, ****p < 0.0001.
The control of social behavior, aggression and anxiety has been linked to monoamine signaling in the brain (Popova, 2008; Herculano and Maximino, 2014; Carreno Gutierrez et al., 2019). We used high pressure liquid chromatography (HPLC) to quantify the levels of 5-HT, DA and metabolites in WT and reln mutant zebrafish. Since heterozygous reln+/– mutants did not show behavioral alterations compared to WT we focused on reln–/– animals in these experiments. Using HPLC we uncovered a selective increase of 5-HT in the hindbrain of reln–/– compared to WT [Figure 6D; t-test: t(3.694) = 70, p = 0.0021, multiple t-tests with Holm–Sidak multiple comparisons correction]. In contrast, we detected similar levels of dopamine (DA), homovanillic acid (HVA), dihydroxyphenylacetic acid (DOPAC) and 5-hydroxyindoleacetic acid (5HIAA) in the brain of both genotypes (Figures 6A–D). We also calculated the utilization ratio of each neurotransmitter. HPLC measures the sum basal level of analytes in the brain regardless of whether or not have been released at the synapse. Since neurotransmitters are broken down to their metabolites upon release the utilization ratio gives an approximation of neurotransmitter activity (Kilpatrick et al., 1986). The utilization ratio of 5-HT and DA was unaffected in reln–/– suggesting that release and reuptake of neurotransmitters is unaffected in these mutants (Figures 6E–G).
Figure 6. High pressure liquid chromatography. 5-HT levels are increased in the hindbrain of reln–/– compared to WT [t-test: t(3.694) = 70, p = 0.0021, multiple t-tests with Holm–Sidak multiple comparisons correction. n = 8 WT, n = 8 reln–/–]. (A) Telencephalon, (B) diencephalon, (C) optic tectum and (D) hindbrain. No differences in the breakdown of (E) DOPAC/DA, (F) HVA/DA, (G) 5-HIAA/-5HT. DA, dopamine; Di, diencephalon; DOPAC, 3,4-Dihydroxyphenylacetic acid; Hb, Hindbrain; HVA, homovanillic acid; Tel, telencephalon; TeO, optic tectum; 5-HIAA, 5-hydroxyindoleacetic acid; 5-HT, 5-hydroxytryptamine. ∗∗p < 0.01. Mean ± SEM.
HPLC analysis identified a significant increase of 5-HT in the hindbrain of reln–/–. We applied the 5HT-1A receptor agonist buspirone that decreases the concentration of 5-HT at the synapse (Loane and Politis, 2012) and measured behavior. Immersion in buspirone increased the interaction of WT with the second group of unfamiliar fish in the visually mediated social preference test (Figure 7A; first strangers p = 0.51 and second strangers p = 0.004). However, buspirone did not rescue the mutant phenotype, and reln–/– still failed to switch social preference following drug application [Figure 7B; second strangers p = 0.01; three-way ANOVA followed by Sidak’s post hoc, genotype factor: F(1,56) = 0.2488, p = 0.62, stranger factor: F(1,56) = 2.11, p = 0.16, treatment factor: F(1,56) = 0.0124, p = 0.91, interaction genotype × stranger: F(1,56) = 40.28, p < 0.0001, interaction genotype × treatment: F(1,56) = 0.01879, p = 0.89, interaction stranger × treatment: F(1,56) = 3.362, p = 0.07, interaction stranger × genotype × treatment: F(1,56) = 0.0566, p = 0.81]. In humans, mutations in RELN are linked to autism spectrum disorder. We applied the autism treatment drugs oxytocin (Hollander et al., 2003; Andari et al., 2010; Guastella et al., 2010) and risperidone (Canitano and Scandurra, 2008) to reln–/– mutants and quantified individual social interactions in the social preference test. However, neither oxytocin nor risperidone altered the preference for social novelty in zebrafish of either genotype [Figure 8A; WT saline p = 0.57, WT oxytocin p = 0.53 and WT risperidone p = 0.14, Figure 8B; reln–/– saline p = 0.014, reln–/– oxytocin p = 0.048, reln–/– risperidone p = 0.014; Three-way ANOVA followed by Sidak’s post hoc for oxytocin, genotype factor: F(1,64) = 0.3928, p = 0.53, stranger factor: F(1,64) = 2.133, p = 0.15, treatment factor: F(1,64) = 0.0143, p = 0.90, interaction genotype × stranger: F(1,64) = 17.44, p < 0.0001, interaction genotype × treatment: F(1,64) = 0.0282, p = 0.87, interaction stranger × treatment: F(1,64) = 0.0707, p = 0.79, interaction stranger × genotype × treatment: F(1,64) = 0.04759, p = 0.83. Three-way ANOVA followed by Sidak’s post hoc for risperidone, genotype factor: F(1,64) = 1.073, p = 0.30, stranger factor: F(1,64) = 2.573, p = 0.11, treatment factor: F(1,64) = 0.8058, p = 0.37, interaction genotype × stranger: F(1,64) = 25.65, p < 0.0001, interaction genotype × treatment: F(1,64) = 0.3363, p = 0.56, interaction stranger × treatment: F(1,64) = 0.01244, p = 0.91, interaction stranger × genotype × treatment: F(1,64) = 0.4869, p = 0.49].
Figure 7. Buspirone increase the social preference for novelty in WT but not reln–/–. (A) WT treated with saline spend equal time near the two groups of unfamiliar fish, whereas WT treated with buspirone switch preference and spend more time near the new group of unfamiliar fish. (B) Buspirone treatment does not completely rescue the impaired preference for social novelty of reln–/– zebrafish (WT with saline p = 0.51; WT with buspirone p = 0.0039; reln–/– with saline p < 0.0001; reln–/– with buspirone p = 0.012). Three-way ANOVA followed by Sidak’s post hoc, genotype factor: F(1,56) = 0.2488, p = 0.62, stranger factor: F(1,56) = 2.11, p = 0.16, treatment factor: F(1,56) = 0.0124, p = 0.91, interaction genotype × stranger: F(1,56) = 40.28, p < 0.0001, interaction genotype × treatment: F(1,56) = 0.01879, p = 0.89, interaction stranger × treatment: F(1,56) = 3.362, p = 0.07, interaction stranger × genotype × treatment: F(1,56) = 0.0566, p = 0.81). n = 8 WT and n = 8 reln–/–. ∗p < 0.05, ∗∗p < 0.01, ****p < 0.0001. Mean ± SEM.
Figure 8. Oxytocin and risperidone do not rescue preference for social novelty. (A) WT treated with saline (p = 0.57), oxytocin (p = 0.53), and risperidone (p = 0.14) spend equal time near the two groups of unfamiliar fish. (B) Neither oxytocin (p = 0.048) nor risperidone (p = 0.0023) treatment rescues the impaired preference for social novelty of reln–/– zebrafish (saline control, p = 0.014). Three-way ANOVA followed by Sidak’s post hoc for oxytocin, genotype factor: F(1,64) = 0.3928, p = 0.53, stranger factor: F(1,64) = 2.133, p = 0.15, treatment factor: F(1,64) = 0.0143, p = 0.90, interaction genotype × stranger: F(1,64) = 17.44, p < 0.0001, interaction genotype × treatment: F(1,64) = 0.0282, p = 0.87, interaction stranger × treatment: F(1,64) = 0.0707, p = 0.79, interaction stranger × genotype × treatment: F(1,64) = 0.04759, p = 0.83. Three-way ANOVA followed by Sidak’s post hoc for risperidone, genotype factor: F(1,64) = 1.073, p = 0.30, stranger factor: F(1,64) = 2.573, p = 0.11, treatment factor: F(1,64) = 0.8058, p = 0.37, interaction genotype × stranger: F(1,64) = 25.65, p < 0.0001, interaction genotype × treatment: F(1,64) = 0.3363, p = 0.56, interaction stranger × treatment: F(1,64) = 0.01244, p = 0.91, interaction stranger × genotype × treatment: F(1,64) = 0.4869, p = 0.49. n = 9 WT and n = 9 reln–/–. ∗p < 0.05, ∗∗p < 0.01. Mean ± SEM.
In this study we have quantified the contribution of Reelin pathway signaling to adult zebrafish behavior. Zebrafish reln–/– mutants display a decrease in preference for social novelty, without altering shoaling, anxiety-like behavior, aggression or exploration. Conversely mutants for the canonical Reln targets dab1a and vldlr display normal social preference, but heightened aggression (dab1a–/–) and exploration (vldlr–/–). This suggests that Reln signaling has a pleiotropic role in controlling zebrafish behavior.
The zebrafish telencephalon has a non-laminar structure that develops through eversion, a process whereby the dorsal pallial region of the forebrain folds over the more ventral subpallium (Folgueira et al., 2012). Conversely, the mammalian cortex has a six-layered laminar structure that develops through evagination (Nieuwenhuys, 1994; Mueller, 2011). In zebrafish, Reln protein is secreted from superficial inhibitory interneurons (SINs) in a similar manner to the localization of Reln in interneurons in other adult vertebrates (Di Donato et al., 2018). However, Reln could have a different function in the non-laminar zebrafish brain compared to other vertebrates. Antibody labeling of the adult zebrafish brain agrees with the distribution of reln mRNA described by Costagli et al. (2002). The optic tectum is the principal retinorecipient brain region in zebrafish and is homologous to the superior colliculus in mammals (Gebhardt et al., 2013). The stratum fibrosum marginale (sfm) is located underneath the pia mater and glia limitans and the stratum opticum (so) is directly below it. The sfm is a plexiform-type layer containing a dense assortment of axons, axon terminals and dendrites, whereas the so is formed of optic nerve terminal bundles (Corbo et al., 2012). Reln is also found in the torus longitudinalis (TL), a ray-finned fish-specific paired longitudinal eminence of granule cells located at the medial margins of the optic tectum. The granule cells of the torus longitudinalis project unmyelinated axons through the contralateral sfm of the optic tectum to synapse upon pyramidal cells. The torus longitudinalis is thought to be involved in learning complex visual scenes (Northmore, 2017). In the isthmic tegmentum, Reln is found in a few cells in the interpeduncular nucleus (nin), a structure that receives projections from the dorsal habenula and may be involved in fear conditioning (Amo et al., 2010). In the hindbrain, granule cells of the corpus cerebelli (CCe) send their axons to Purkinje cells in the molecular layer of the cerebellum, whereas the granule cells of the caudal lobe of the cerebellum (LCa) project to crest cells in the crista cerebellaris (CC; Takeuchi et al., 2015). Therefore, the hindbrain areas that contain Reln are all linked to each other functionally. Scattered Reln-positive cells are also present in the intermediate and inferior part of the reticular formation, an intricate network of excitatory and inhibitory neurons and diffuse nuclei extending from the spinal cord through the medulla oblongata to the mesencephalon.
The presence of Reln in the laminated structures of the optic tectum and cerebellum suggest that this protein may have a conserved role in neuronal migration in some areas of the zebrafish brain. This hypothesis has been confirmed in a study showing synaptic lamination defects in the optic tectum of reln–/– larvae (Di Donato et al., 2018). Although the optic tectum of adult zebrafish reln–/– mutants does not show patterning defects, in the cerebellum Purkinje cells, eurydendroid cells and Bergmann glia are found in ectopic positions in the absence of hypoplasia (Nimura et al., 2019). The localization of Reln in the zebrafish brain fits with the idea that this protein could play a role in synaptic organization or the integration of sensory afferent information from tectal or diencephalic brain areas to the dorsal telencephalon (Costagli et al., 2002).
The presence of RELN at the peak region of linkage and first autism susceptibility locus (chromosome 7q22) suggested that loss of reln function might alter zebrafish social behavior. In a shoaling test, groups of mutants displayed similar social interactions as groups of WT zebrafish including inter-individual distance (Figure 3A), polarization (Figure 3B) and velocity (Figure 3C). Furthermore, in a social preference test, reln–/– mutants interacted normally with a first group of conspecifics (Figure 2A) suggesting that social interactions occur normally in this mutant line. However, reln–/– fail to interact with a second group of animals placed into the same tank demonstrating a reduction in preference for social novelty (Figure 2B). Other behavioral measures, including exploration of a large tank (Figures 3D–F), anxiety-like behavior (Figures 3G,H), and aggression (Figure 3I) were not altered.
Homozygous Reln–/– mutant mice are not suitable for behavioral studies since they display severe ataxia, likely due to their severe neuronal migration defects. However, heterozygous reeler mice (Reln+/–) display behavioral, cognitive and neuroanatomical defects that are milder than those seen in Reln–/–. RELN haploinsufficiency causes loss of Purkinje neurons in the cerebellum of Reln+/– (Biamonte et al., 2009; Maloku et al., 2010), a phenotype that is similar to the loss of Purkinje cells seen in the cerebellum of some ASD patients (Ritvo et al., 1986). Behavioral characterization of Reln+/– has provided ambiguous results. Some studies have reported increased anxiety, impaired executive function, motor impulsivity, abnormal prepulse inhibition, decreased contextual fear conditioning and associative learning impairments in Reln+/– mice (Tueting et al., 1999; Tremolizzo et al., 2002; Krueger et al., 2006; Qiu et al., 2006) whereas other groups report that Reln+/– display normal locomotor activity, prepulse inhibition, anxiety, coordination, social behavior, spatial learning and transitions in the light–dark test, a measure of anxiety (Podhorna and Didriksen, 2004; Qiu et al., 2006). Regarding social interactions and communication, hallmarks of ASD, Reln+/– spend more time engaging in social investigation (Podhorna and Didriksen, 2004) but do not display alterations in social and vocal repertoires during courtship (Michetti et al., 2014). However, deletion of the positively charged carboxy-terminal region of RELN attenuates canonical RELN signaling and leads to decreased social interaction, impaired working memory, hyperactivity and decreased anxiety compared to WT (Kohno et al., 2015; Sakai et al., 2016). The surprising range of different behavioral phenotypes that has been reported could be due to the genetic background of the mutant animals, the age of testing or the behavioral protocol used.
Reln signals via several downstream pathways including the canonical receptors apolipoprotein E receptor 2 and the very low density lipoprotein receptor (Trommsdorff et al., 1999) both of which lead to Disabled-1 activation (Howell et al., 1997; Sheldon et al., 1997). We investigated the possible contribution of canonical signaling to the phenotype of reln–/– zebrafish by characterizing the behavior of dab1a–/– and vldr–/– mutant lines. Both dab1a–/– and vldlr–/– displayed different behavioral profiles. dab1a–/– fish were hyperactive (Figure 4F) and more aggressive (Figure 4D) compared to WT without showing alterations to social behavior (Figure 4). Conversely, vldlr–/– exhibited a selective increase in exploration of an open field tank (Figure 5D). Although we did not have access to an apoer2–/– mutant line, the essential adaptor protein Dab1a represents a common target for both receptors. Therefore, the lack of phenotypic overlap with reln–/– suggests that we can rule out a contribution of canonical signaling to the decreased preference for social novelty. Studies to address this issue could include examining other Reln signaling targets such as the CrK/Rap1, P13K/Akt/mTOR, and MEK/Erk1/2 pathways (Chen et al., 2010). For example, this could include rescuing the social deficit of reln–/– mutants by using drugs that stimulate these signaling pathways.
Irregularities in several neurotransmitter pathways, including the monoamine 5-HT, have been implicated in the pathology of ASD. We used high pressure liquid chromatography to measure the monoamines dopamine, 5-HT and their metabolites (Figure 6). We observed a significant increase of 5-HT that was restricted to the hindbrain of reln–/– (Figure 6D), the brain region that contains the raphe nucleus [the largest group of 5-HT producing neurons in zebrafish (Lillesaar et al., 2009) and that expresses the signaling pathways components tryptophan hydroxylase 2, vesicular monoamine transporter and the 5-HT transporter-encoding gene solute carrier family 6 member 4a (Teraoka et al., 2004; Wang et al., 2006; Norton et al., 2008; Wen et al., 2008)]. As well as regulating cell division and differentiation, neurite growth, and synaptogenesis, 5-HT also modulates human behaviors associated with psychiatric disorders (Chugani, 2002). Both hypo- and hyper-serotonemia can occur in ASD patients (Schain and Freedman, 1961; Connors et al., 2006; Yang et al., 2014). We investigated the connection between aberrant 5-HT signaling and social preference by applying the 5-HT1A receptor partial agonist buspirone (Loane and Politis, 2012). Buspirone acts presynaptically to reduce 5-HT levels (Gebauer et al., 2011) and it can have both anxiolytic and pro-social effects in zebrafish (File and Seth, 2003; Bencan et al., 2009; Gould, 2011; Barba-Escobedo and Gould, 2012; Gould et al., 2012; Maaswinkel et al., 2012; Maaswinkel et al., 2013). Although immersion in buspirone increased the interaction of WT with the second group of unfamiliar fish (Figure 7A) it did not rescue the mutant phenotype, with reln–/– failing to switch social preference following drug treatment (Figure 7B). This suggests that the 5-HT signaling does not underpin the lack of preference for social novelty seen in reln–/– zebrafish, and further research is required to understand the neurotransmitter basis of this behavioral phenotype.
Multiple lines of evidence suggest that RELN is a vulnerability factor for ASD. Characterization of the behavioral phenotype of reln–/– mutant zebrafish provided an opportunity to investigate which adult zebrafish behaviors can be linked to ASD-candidate genes. Using a combination of behavioral tests we were able to identify a specific alteration in preference for social novelty without a general change to social behavior. It is difficult to compare this finding to other model species such as mouse because manipulation of Reln signaling leads to such a wide range of different phenotypes (Podhorna and Didriksen, 2004; Qiu et al., 2006). The reduction in preference for social novelty that we observed is surprisingly specific and would fit with the definition of ASD that includes restricted interests and repetitive patterns of behavior that are abnormal in intensity or focus. Arguing against this idea, however, we were unable to rescue this phenotype by applying two drugs that are used to treat ASD: oxytocin and risperidone. One explanation for this result could be that we need to apply these drugs for a longer time period or at higher concentration. For example, oxytocin administration to mouse models of ASD symptoms only rescued social deficits behavior 1–2 weeks following sub-chronic application (Teng et al., 2013). Furthermore, since there are extensive connections between oxytocin and 5-HT neurotransmitter signaling co-application of drugs targeting both these pathways may be required to rescue the behavioral phenotype of reln–/–.
In summary, the abnormal preference for social novelty displayed by reln–/– zebrafish mutants suggest that they may represent a good model to analyze some aspects of ASD, including restricted or repetitive behavior. Further research comparing the simultaneous presentation of different types of stimuli in the visually mediated preference test could be used to investigate this idea in more detail. Creation of novel zebrafish reln mutant lines that either harbor mutations associated with ASD in human patients or in different genetic backgrounds might provide further insights into the pleiotropic behavioral phenotypes seen in animals lacking Reln signaling.
The datasets generated for this study are available on request to the corresponding author.
This study was carried out in accordance with the recommendations of the local Animal Welfare and Ethical Review board at the University of Leicester. The protocol was approved by the local Animal Welfare and Ethical Review board at the University of Leicester.
ED designed the experiments, collected and analyzed the data, and wrote a first version of the manuscript. VD created the reln–/–, dab1a–/– and vldlr–/– mutant lines. AY helped collect and analyze the HPLC data. FD provided the novel mutant lines. WN analyzed the data and wrote the manuscript. All authors approved the final manuscript before submission.
The research leading to this publication received funding from the European Union’s Horizon2020 Research and Innovation Program under grant agreement no 643051.
The authors declare that the research was conducted in the absence of any commercial or financial relationships that could be construed as a potential conflict of interest.
We are grateful to Carl Breaker and members of the preclinical research facility for zebrafish care, Ceinwen Tilley for technical support and all members of the Norton lab for discussions about this data.
Amo, R., Aizawa, H., Takahoko, M., Kobayashi, M., Takahashi, R., Aoki, T., et al. (2010). Identification of the zebrafish ventral habenula as a homolog of the mammalian lateral habenula. J. Neurosci. 30, 1566–1574. doi: 10.1523/JNEUROSCI.3690-09.2010
Andari, E., Duhamel, J. R., Zalla, T., Herbrecht, E., Leboyer, M., and Sirigu, A. (2010). Promoting social behavior with oxytocin in high-functioning autism spectrum disorders. Proc. Natl. Acad. Sci. U.S.A. 107, 4389–4394. doi: 10.1073/pnas.0910249107
Barba-Escobedo, P. A., and Gould, G. G. (2012). Visual social preferences of lone zebrafish in a novel environment: strain and anxiolytic effects. Genes Brain Behav. 11, 366–373. doi: 10.1111/j.1601-183X.2012.00770.x
Barr, A. M., Fish, K. N., Markou, A., and Honer, W. G. (2008). Heterozygous reeler mice exhibit alterations in sensorimotor gating but not presynaptic proteins. Eur. J. Neurosci. 27, 2568–2574. doi: 10.1111/j.1460-9568.2008.06233.x
Bencan, Z., Sledge, D., and Levin, E. D. (2009). Buspirone, chlordiazepoxide and diazepam effects in a zebrafish model of anxiety. Pharmacol. Biochem. Behav. 94, 75–80. doi: 10.1016/j.pbb.2009.07.009
Biamonte, F., Assenza, G., Marino, R., D’amelio, M., Panteri, R., Caruso, D., et al. (2009). Interactions between neuroactive steroids and reelin haploinsufficiency in Purkinje cell survival. Neurobiol. Dis. 36, 103–115. doi: 10.1016/j.nbd.2009.07.001
Bock, H. H., and May, P. (2016). Canonical and non-canonical reelin signaling. Front. Cell. Neurosci. 10:166. doi: 10.3389/fncel.2016.00166
Bonora, E., Beyer, K. S., Lamb, J. A., Parr, J. R., Klauck, S. M., Benner, A., et al. (2003). Analysis of reelin as a candidate gene for autism. Mol. Psychiatry 8, 885–892. doi: 10.1038/sj.mp.4001310
Bufill, E., Roura-Poch, P., Sala-Matavera, I., Antón, S., Lleó, A., Sánchez-Saudinós, B., et al. (2013). Reelin signaling pathway genotypes and alzheimer disease in a spanish population. Alzheimer Dis. Assoc. Disord. 29, 169–172. doi: 10.1097/WAD.0000000000000002
Canitano, R., and Scandurra, V. (2008). Risperidone in the treatment of behavioral disorders associated with autism in children and adolescents. Neuropsychiatr. Dis. Treat. 4, 723–730.
Carreno Gutierrez, H., Colanesi, S., Cooper, B., Reichmann, F., Young, A. M. J., Kelsh, R. N., et al. (2019). Endothelin neurotransmitter signalling controls zebrafish social behaviour. Sci. Rep. 9:3040. doi: 10.1038/s41598-019-39907-7
Carreno Gutierrez, H., O’leary, A., Freudenberg, F., Fedele, G., Wilkinson, R., Markham, E., et al. (2017). Nitric oxide interacts with monoamine oxidase to modulate aggression and anxiety-like behaviour. Eur. Neuropsychopharmacol. doi: 10.1016/j.euroneuro.2017.09.004 [Epub ahead of print].
Chen, Y., Durakoglugil, M. S., Xian, X., and Herz, J. (2010). ApoE4 reduces glutamate receptor function and synaptic plasticity by selectively impairing ApoE receptor recycling. Proc. Natl. Acad. Sci. U.S.A. 107, 12011–12016. doi: 10.1073/pnas.0914984107
Chugani, D. (2002). Role of altered brain serotonin mechanisms in autism. Mol. Psychiatry 7(Suppl. 2), S16–S17.
Connors, S. L., Matteson, K. J., Sega, G. A., Lozzio, C. B., Carroll, R. C., and Zimmerman, A. W. (2006). Plasma serotonin in autism. Pediatr. Neurol. 35, 182–186.
Corbo, C. P., Othman, N. A., Gutkin, M. C., Alonso Adel, C., and Fulop, Z. L. (2012). Use of different morphological techniques to analyze the cellular composition of the adult zebrafish optic tectum. Microsc. Res. Tech. 75, 325–333. doi: 10.1002/jemt.21061
Costa, E. (2002). The heterozygote reeler mouse as a model for the development of a new generation of antipsychotics. Curr. Opin. Pharmacol. 2, 56–62. doi: 10.1016/s1471-4892(01)00121-7
Costagli, A., Kapsimali, M., Wilson, S. W., and Mione, M. (2002). Conserved and divergent patterns of Reelin expression in the zebrafish central nervous system. J. Comp. Neurol. 450, 73–93. doi: 10.1002/cne.10292
D’Arcangelo, G. (2005). The reeler mouse: anatomy of a mutant. Int. Rev. Neurobiol. 71, 383–417. doi: 10.1016/s0074-7742(05)71016-3
D’Arcangelo, G. (2006). Reelin mouse mutants as models of cortical development disorders. Epilepsy Behav. 8, 81–90. doi: 10.1016/j.yebeh.2005.09.005
D’Arcangelo, G. (2014). Reelin in the years: controlling neuronal migration and maturation in the mammalian brain. Adv. Neurosci. 2014, 1–19. doi: 10.1155/2014/597395
De Rubeis, S., He, X., Goldberg, A. P., Poultney, C. S., Samocha, K., Cicek, A. E., et al. (2014). Synaptic, transcriptional and chromatin genes disrupted in autism. Nature 515, 209–215. doi: 10.1038/nature13772
Di Donato, V., De Santis, F., Albadri, S., Auer, T. O., Duroure, K., Charpentier, M., et al. (2018). An attractive reelin gradient establishes synaptic lamination in the vertebrate visual system. Neuron 97, 1049.e6–1062.e6. doi: 10.1016/j.neuron.2018.01.030
Dutta, S., Guhathakurta, S., Sinha, S., Chatterjee, A., Ahmed, S., Ghosh, S., et al. (2006). Reelin gene polymorphisms in the Indian population: a possible paternal 5’UTR-CGG-repeat-allele effect on autism. Am. J. Med. Genet. Part B Neuropsychiatr. Genet. 144B, 106–112. doi: 10.1002/ajmg.b.30419
Dutta, S., Sinha, S., Ghosh, S., Chatterjee, A., Ahmed, S., and Usha, R. (2008). Genetic analysis of reelin gene (RELN) SNPs: no association with autism spectrum disorder in the Indian population. Neurosci. Lett. 441, 56–60. doi: 10.1016/j.neulet.2008.06.022
Egan, R. J., Bergner, C. L., Hart, P. C., Cachat, J. M., Canavello, P. R., Elegante, M. F., et al. (2009). Understanding behavioral and physiological phenotypes of stress and anxiety in zebrafish. Behav. Brain Res. 205, 38–44. doi: 10.1016/j.bbr.2009.06.022
Fatemi, S. H. (2001). Reelin mutations in mouse and man: from reeler mouse to schizophrenia, mood disorders, autism and lissencephaly. Mol. Psychiatry 6, 129–133. doi: 10.1038/sj.mp.4000129
Fatemi, S. H., Snow, A. V., Stary, J. M., Araghi-Niknam, M., Reutiman, T. J., Lee, S., et al. (2005). Reelin signaling is impaired inFsample autism. Biol. Psychiatry 57, 777–787. doi: 10.1016/j.biopsych.2004.12.018
File, S. E., and Seth, P. (2003). A review of 25 years of the social interaction test. Eur. J. Pharmacol. 463, 35–53. doi: 10.1016/s0014-2999(03)01273-1
Folgueira, M., Bayley, P., Navratilova, P., Becker, T. S., Wilson, S. W., and Clarke, J. D. (2012). Morphogenesis underlying the development of the everted teleost telencephalon. Neural Dev. 7:32. doi: 10.1186/1749-8104-7-32
Förster, E., Bock, H. H., Herz, J., Chai, X., Frotscher, M., and Zhao, S. (2010). Emerging topics in Reelin function. Eur. J. Neurosci. 31, 1511–1518. doi: 10.1111/j.1460-9568.2010.07222.x
Gebauer, D. L., Pagnussat, N., Piato, A. L., Schaefer, I. C., Bonan, C. D., and Lara, D. R. (2011). Effects of anxiolytics in zebrafish: similarities and differences between benzodiazepines, buspirone and ethanol. Pharmacol. Biochem. Behav. 99, 480–486. doi: 10.1016/j.pbb.2011.04.021
Gebhardt, C., Baier, H., and Del Bene, F. (2013). Direction selectivity in the visual system of the zebrafish larva. Front. Neural Circuits 7:111. doi: 10.3389/fncir.2013.00111
Goffinet, A. M. (1983). The embryonic development of the cerebellum in normal and reeler mutant mice. Anat. Embryol. 168, 73–86. doi: 10.1007/bf00305400
Gould, G. G. (2011). “Aquatic light/dark plus maze novel environment for assessing anxious versus exploratory behavior in zebrafish (Danio rerio) and other small teleost fish,” in Zebrafish Neurobehavioral Protocols, eds A. Kalueff, and J. Cachat, (Totowa, NJ: Humana Press), 99–108. doi: 10.1007/978-1-60761-953-6_8
Gould, G. G., Seillier, A., Weiss, G., Giuffrida, A., Burke, T. F., Hensler, J. G., et al. (2012). Acetaminophen differentially enhances social behavior and cortical cannabinoid levels in inbred mice. Prog. Neuro Psychopharmacol. Biol. Psychiatry 38, 260–269. doi: 10.1016/j.pnpbp.2012.04.011
Guastella, A. J., Einfeld, S. L., Gray, K. M., Rinehart, N. J., Tonge, B. J., Lambert, T. J., et al. (2010). Intranasal oxytocin improves emotion recognition for youth with autism spectrum disorders. Biol. Psychiatry 67, 692–694. doi: 10.1016/j.biopsych.2009.09.020
He, Y., Xun, G., Xia, K., Hu, Z., Lv, L., Deng, Z., et al. (2011). No significant association between RELN polymorphism and autism in case-control and family-based association study in Chinese Han population. Psychiatry Res. 187, 462–464. doi: 10.1016/j.psychres.2010.04.051
Herculano, A. M., and Maximino, C. (2014). Serotonergic modulation of zebrafish behavior: towards a paradox. Prog. Neuropsychopharmacol. Biol. Psychiatry 55, 50–66. doi: 10.1016/j.pnpbp.2014.03.008
Herz, J., and Chen, Y. (2006). Reelin, lipoprotein receptors and synaptic plasticity. Nat. Rev. Neurosci. 7, 850–859. doi: 10.1038/nrn2009
Hollander, E., Novotny, S., Hanratty, M., Yaffe, R., Decaria, C. M., Aronowitz, B. R., et al. (2003). Oxytocin infusion reduces repetitive behaviors in adults with autistic and Asperger’s disorders. Neuropsychopharmacology 28, 193–198. doi: 10.1038/sj.npp.1300021
Hong, S. E., Shugart, Y. Y., Huang, D. T., Shahwan, S. A., Grant, P. E., Hourihane, J. O. B., et al. (2000). Autosomal recessive lissencephaly with cerebellar hypoplasia is associated with human RELN mutations. Nat. Genet. 26, 93–96. doi: 10.1038/79246
Howell, B. W., Hawkes, R., Soriano, P., and Cooper, J. A. (1997). Neuronal position in the developing brain is regulated by mouse disabled-1. Nature 389, 733–737. doi: 10.1038/39607
Iafrati, J., Orejarena, M. J., Lassalle, O., Bouamrane, L., and Chavis, P. (2013). Reelin, an extracellular matrix protein linked to early onset psychiatric diseases, drives postnatal development of the prefrontal cortex via GluN2B-NMDARs and the mTOR pathway. Mol. Psychiatry 19, 417–426. doi: 10.1038/mp.2013.66
Idalencio, R., Kalichak, F., Rosa, J. G., De Oliveira, T. A., Koakoski, G., Gusso, D., et al. (2015). Waterborne risperidone decreases stress response in Zebrafish. PLoS One 10:e0140800. doi: 10.1371/journal.pone.0140800
Imai, H., Shoji, H., Ogata, M., Kagawa, Y., Owada, Y., Miyakawa, T., et al. (2016). Dorsal forebrain-specific deficiency of Reelin-Dab1 signal causes behavioral abnormalities related to psychiatric disorders. Cereb. Cortex 27, 3485–3501. doi: 10.1093/cercor/bhv334
Kilpatrick, I. C., Jones, M. W., and Phillipson, O. T. (1986). A semiautomated analysis method for catecholamines, indoleamines, and some prominent metabolites in microdissected regions of the nervous system: an isocratic HPLC technique employing coulometric detection and minimal sample preparation. J. Neurochem. 46, 1865–1876. doi: 10.1111/j.1471-4159.1986.tb08506.x
Kohno, T., Honda, T., Kubo, K.-I., Nakano, Y., Tsuchiya, A., Murakami, T., et al. (2015). Importance of Reelin C-terminal region in the development and maintenance of the postnatal cerebral cortex and its regulation by specific proteolysis. J. Neurosci. 35, 4776–4787. doi: 10.1523/JNEUROSCI.4119-14.2015
Koshimizu, E., Miyatake, S., Okamoto, N., Nakashima, M., Tsurusaki, Y., Miyake, N., et al. (2013). Performance comparison of bench-top next generation sequencers using microdroplet PCR-based enrichment for targeted sequencing in patients with autism spectrum disorder. PLoS One 8:e74167. doi: 10.1371/journal.pone.0074167
Krebs, M. O., Betancur, C., Leroy, S., Bourdel, M. C., Gillberg, C., and Leboyer, M. (2002). Absence of association between a polymorphic GGC repeat in the 5’ untranslated region of the reelin gene and autism. Mol. Psychiatry 7, 801–804. doi: 10.1038/sj.mp.4001071
Krueger, D. D., Howell, J. L., Hebert, B. F., Olausson, P., Taylor, J. R., and Nairn, A. C. (2006). Assessment of cognitive function in the heterozygous reeler mouse. Psychopharmacology 189, 95–104. doi: 10.1007/s00213-006-0530-0
Kutiyanawalla, A., Promsote, W., Terry, A., and Pillai, A. (2011). Cysteamine treatment ameliorates alterations in GAD67 expression and spatial memory in heterozygous reeler mice. Int. J. Neuropsychopharmacol. 15, 1073–1086. doi: 10.1017/S1461145711001180
Lee, G. H., and D’Arcangelo, G. (2016). New insights into Reelin-mediated signaling pathways. Front. Cell. Neurosci. 10:122. doi: 10.3389/fncel.2016.00122
Levenson, J. M., Qiu, S., and Weeber, E. J. (2008). The role of reelin in adult synaptic function and the genetic and epigenetic regulation of the reelin gene. Biochim. Biophys. Acta (BBA) Gene Regul. Mech. 1779, 422–431. doi: 10.1016/j.bbagrm.2008.01.001
Levy, A. D., Omar, M. H., and Koleske, A. J. (2014). Extracellular matrix control of dendritic spine and synapse structure and plasticity in adulthood. Front. Neuroanat. 8:116. doi: 10.3389/fnana.2014.00116
Li, H., Li, Y., Shao, J., Li, R., Qin, Y., Xie, C., et al. (2008). The association analysis of RELN and GRM8 genes with autistic spectrum disorder in chinese han population. Am. J. Med. Genet. Part B Neuropsychiatr. Genet. 147B, 194–200. doi: 10.1002/ajmg.b.30584
Li, J., Nguyen, L., Gleason, C., Lotspeich, L., Spiker, D., Risch, N., et al. (2004). Lack of evidence for an association between WNT2 and RELN polymorphisms and autism. Am. J. Med. Genet. 126B, 51–57. doi: 10.1002/ajmg.b.20122
Li, W., Guo, X., and Xiao, S. (2015). Evaluating the relationship between reelin gene variants (rs7341475 and rs262355) and schizophrenia: a meta-analysis. Neurosci. Lett. 609, 42–47. doi: 10.1016/j.neulet.2015.10.014
Lillesaar, C., Stigloher, C., Tannhauser, B., Wullimann, M. F., and Bally-Cuif, L. (2009). Axonal projections originating from raphe serotonergic neurons in the developing and adult zebrafish, Danio rerio, using transgenics to visualize raphe-specific pet1 expression. J. Comp. Neurol. 512, 158–182. doi: 10.1002/cne.21887
Loane, C., and Politis, M. (2012). Buspirone: what is it all about? Brain Res. 1461, 111–118. doi: 10.1016/j.brainres.2012.04.032
Maaswinkel, H., Le, X., He, L., Zhu, L., and Weng, W. (2013). Dissociating the effects of habituation, black walls, buspirone and ethanol on anxiety-like behavioral responses in shoaling zebrafish. A 3D approach to social behavior. Pharmacol. Biochem. Behav. 108, 16–27. doi: 10.1016/j.pbb.2013.04.009
Maaswinkel, H., Zhu, L., and Weng, W. (2012). The immediate and the delayed effects of buspirone on zebrafish (Danio rerio) in an open field test: a 3-D approach. Behav. Brain Res. 234, 365–374. doi: 10.1016/j.bbr.2012.07.014
Maloku, E., Covelo, I. R., Hanbauer, I., Guidotti, A., Kadriu, B., Hu, Q., et al. (2010). Lower number of cerebellar Purkinje neurons in psychosis is associated with reduced reelin expression. Proc. Natl. Acad. Sci. U.S.A. 107, 4407–4411. doi: 10.1073/pnas.0914483107
Michetti, C., Romano, E., Altabella, L., Caruso, A., Castelluccio, P., Bedse, G., et al. (2014). Mapping pathological phenotypes in reelin mutant mice. Front. Pediatr. 2:95. doi: 10.3389/fped.2014.00095
Miyata, T., Nakajima, K., Mikoshiba, K., and Ogawa, M. (1997). Regulation of Purkinje cell alignment by reelin as revealed with CR-50 antibody. J. Neurosci. 17, 3599–3609. doi: 10.1523/jneurosci.17-10-03599.1997
Mueller, T. (2011). The conserved bauplan of the teleostean telencephalon. Brain Behav. Evol. 78, 259–260. doi: 10.1159/000331869
Neale, B. M., Kou, Y., Liu, L., Ma’ayan, A., Samocha, K. E., Sabo, A., et al. (2012). Patterns and rates of exonic de novo mutations in autism spectrum disorders. Nature 485, 242–245. doi: 10.1038/nature11011
Nieuwenhuys, R. (1994). The neocortex. An overview of its evolutionary development, structural organization and synaptology. Anat. Embryol. 190, 307–337.
Nimura, T., Itoh, T., Hagio, H., Hayashi, T., Di Donato, V., Takeuchi, M., et al. (2019). Role of reelin in cell positioning in the cerebellum and the cerebellum-like structure in zebrafish. Dev. Biol. doi: 10.1016/j.ydbio.2019.07.010 [Epub ahead of print].
Northmore, D. P. (2017). Holding visual attention for 400millionyears: a model of tectum and torus longitudinalis in teleost fishes. Vision Res. 131, 44–56. doi: 10.1016/j.visres.2016.12.001
Norton, W. H., Folchert, A., and Bally-Cuif, L. (2008). Comparative analysis of serotonin receptor (HTR1A/HTR1B families) and transporter (slc6a4a/b) gene expression in the zebrafish brain. J. Comp. Neurol. 511, 521–542. doi: 10.1002/cne.21831
Norton, W. H., Stumpenhorst, K., Faus-Kessler, T., Folchert, A., Rohner, N., Harris, M. P., et al. (2011). Modulation of Fgfr1a signaling in zebrafish reveals a genetic basis for the aggression-boldness syndrome. J. Neurosci. 31, 13796–13807. doi: 10.1523/JNEUROSCI.2892-11.2011
Norton, W. H. J., Manceau, L., and Reichmann, F. (2019). The visually mediated social preference test: a novel technique to measure social behavior and behavioral disturbances in Zebrafish. Methods Mol. Biol. 2011, 121–132. doi: 10.1007/978-1-4939-9554-7_8
Ovadia, G., and Shifman, S. (2011). The genetic variation of RELN expression in schizophrenia and bipolar disorder. PLoS One 6:e19955. doi: 10.1371/journal.pone.0019955
Parker, M. O., Brock, A. J., Millington, M. E., and Brennan, C. H. (2013). Behavioral phenotyping of casper mutant and 1-pheny-2-thiourea treated adult zebrafish. Zebrafish 10, 466–471. doi: 10.1089/zeb.2013.0878
Persico, A. M., D’agruma, L., Maiorano, N., Totaro, A., Militerni, R., Bravaccio, C., et al. (2001). Reelin gene alleles and haplotypes as a factor predisposing to autistic disorder. Mol. Psychiatry 6, 150–159. doi: 10.1038/sj.mp.4000850
Pesold, C., Impagnatiello, F., Pisu, M., Uzunov, D., Costa, E., Guidotti, A., et al. (1998). Reelin is preferentially expressed in neurons synthesizing γ-aminobutyric acid in cortex and hippocampus of adult rats. Proc. Natl. Acad. Sci. U.S.A. 95, 3221–3226. doi: 10.1073/pnas.95.6.3221
Podhorna, J., and Didriksen, M. (2004). The heterozygous reeler mouse: behavioural phenotype. Behav. Brain Res. 153, 43–54. doi: 10.1016/j.bbr.2003.10.033
Popova, N. K. (2008). From gene to aggressive behavior: the role of brain serotonin. Neurosci. Behav. Physiol. 38, 471–475. doi: 10.1007/s11055-008-9004-7
Qiu, S., Korwek, K. M., Pratt-Davis, A. R., Peters, M., Bergman, M. Y., and Weeber, E. J. (2006). Cognitive disruption and altered hippocampus synaptic function in Reelin haploinsufficient mice. Neurobiol. Learn. Mem. 85, 228–242. doi: 10.1016/j.nlm.2005.11.001
Rakic, P. (2009). Evolution of the neocortex: a perspective from developmental biology. Nat. Rev. Neurosci. 10, 724–735. doi: 10.1038/nrn2719
Ritvo, E. R., Freeman, B., Scheibel, A. B., Duong, T., Robinson, H., Guthrie, D., et al. (1986). Lower Purkinje cell counts in the cerebella of four autistic subjects: initial findings of the UCLA-NSAC research report. Am. J. Psychiatry 143, 862–866. doi: 10.1176/ajp.143.7.862
Ruhl, N., Mcrobert, S. P., and Currie, W. J. (2009). Shoaling preferences and the effects of sex ratio on spawning and aggression in small laboratory populations of zebrafish (Danio rerio). Lab. Anim. 38, 264–269. doi: 10.1038/laban0809-264
Sakai, K., Shoji, H., Kohno, T., Miyakawa, T., and Hattori, M. (2016). Mice that lack the C-terminal region of Reelin exhibit behavioral abnormalities related to neuropsychiatric disorders. Sci. Rep. 6:28636. doi: 10.1038/srep28636
Schain, R. J., and Freedman, D. X. (1961). Studies on 5-hydroxyindole metabolism in autistic and other mentally retarded children. J. Pediatr. 58, 315–320. doi: 10.1016/s0022-3476(61)80261-8
Serajee, F. J., Zhong, H., and Mahbubul Huq, A. H. M. (2006). Association of Reelin gene polymorphisms with autism. Genomics 87, 75–83. doi: 10.1016/j.ygeno.2005.09.008
Sheldon, M., Rice, D. S., D’arcangelo, G., Yoneshima, H., Nakajima, K., Mikoshiba, K., et al. (1997). Scrambler and yotari disrupt the disabled gene and produce a reeler-like phenotype in mice. Nature 389, 730–733. doi: 10.1038/39601
Takeuchi, M., Matsuda, K., Yamaguchi, S., Asakawa, K., Miyasaka, N., Lal, P., et al. (2015). Establishment of Gal4 transgenic zebrafish lines for analysis of development of cerebellar neural circuitry. Dev. Biol. 397, 1–17. doi: 10.1016/j.ydbio.2014.09.030
Teixeira, C. M., Martín, E. D., Sahún, I., Masachs, N., Pujadas, L., Corvelo, A., et al. (2011). Overexpression of Reelin prevents the manifestation of behavioral phenotypes related to schizophrenia and bipolar disorder. Neuropsychopharmacology 36, 2395–2405. doi: 10.1038/npp.2011.153
Teng, B. L., Nonneman, R. J., Agster, K. L., Nikolova, V. D., Davis, T. T., Riddick, N. V., et al. (2013). Prosocial effects of oxytocin in two mouse models of autism spectrum disorders. Neuropharmacology 72, 187–196. doi: 10.1016/j.neuropharm.2013.04.038
Teraoka, H., Russell, C., Regan, J., Chandrasekhar, A., Concha, M. L., Yokoyama, R., et al. (2004). Hedgehog and Fgf signaling pathways regulate the development of tphR-expressing serotonergic raphe neurons in zebrafish embryos. J. Neurobiol. 60, 275–288. doi: 10.1002/neu.20023
Tremolizzo, L., Carboni, G., Ruzicka, W., Mitchell, C., Sugaya, I., Tueting, P., et al. (2002). An epigenetic mouse model for molecular and behavioral neuropathologies related to schizophrenia vulnerability. Proc. Natl. Acad. Sci. U.S.A. 99, 17095–17100. doi: 10.1073/pnas.262658999
Trommsdorff, M., Gotthardt, M., Hiesberger, T., Shelton, J., Stockinger, W., and Nimpf, J. (1999). Reeler/Disabled-like disruption of neuronal migration in knockout mice lacking the VLDL receptor and ApoE receptor 2. Cell 97, 689–701. doi: 10.1016/S0092-8674(00)80782-5
Tueting, P., Costa, E., Dwivedi, Y., Guidotti, A., Impagnatiello, F., Manev, R., et al. (1999). The phenotypic characteristics of heterozygous reeler mouse. Neuro Rep. 10, 1329–1334. doi: 10.1097/00001756-199904260-00032
Wang, Y., Takai, R., Yoshioka, H., and Shirabe, K. (2006). Characterization and expression of serotonin transporter genes in zebrafish. Tohoku J. Exp. Med. 208, 267–274. doi: 10.1620/tjem.208.267
Wang, Z., Hong, Y., Zou, L., Zhong, R., Zhu, B., Shen, N., et al. (2014). Reelin gene variants and risk of autism spectrum disorders: an integrated meta-analysis. Am. J. Med. Genet. B Neuropsychiatr. Genet. 165B, 192–200. doi: 10.1002/ajmg.b.32222
Weeber, E. J., Beffert, U., Jones, C., Christian, J. M., Förster, E., Sweatt, J. D., et al. (2002). Reelin and ApoE receptors cooperate to enhance hippocampal synaptic plasticity and learning. J. Biol. Chem. 277, 39944–39952. doi: 10.1074/jbc.m205147200
Wen, L., Wei, W., Gu, W., Huang, P., Ren, X., Zhang, Z., et al. (2008). Visualization of monoaminergic neurons and neurotoxicity of MPTP in live transgenic zebrafish. Dev. Biol. 314, 84–92. doi: 10.1016/j.ydbio.2007.11.012
Yang, C.-J., Tan, H.-P., and Du, Y.-J. (2014). The developmental disruptions of serotonin signaling may involved in autism during early brain development. Neuroscience 267, 1–10. doi: 10.1016/j.neuroscience.2014.02.021
Yuen, R. K. C., Thiruvahindrapuram, B., Merico, D., Walker, S., Tammimies, K., Hoang, N., et al. (2015). Whole-genome sequencing of quartet families with autism spectrum disorder. Nat. Med. 21, 185–191. doi: 10.1038/nm.3792
Zhang, H., Liu, X., Zhang, C., Mundo, E., Macciardi, F., Grayson, D. R., et al. (2002). Reelin gene alleles and susceptibility to autism spectrum disorders. Mol. Psychiatry 7, 1012–1017. doi: 10.1038/sj.mp.4001124
Zhang, Y., Kong, W., Gao, Y., Liu, X., Gao, K., Xie, H., et al. (2015). Gene mutation analysis in 253 Chinese children with unexplained epilepsy and intellectual/developmental disabilities. PLoS One 10:e0141782. doi: 10.1371/journal.pone.0141782
Keywords: zebrafish, Reelin, behavior, autism, neurochemistry, social behavior, neuroscience, 5-HT
Citation: Dalla Vecchia E, Di Donato V, Young AMJ, Del Bene F and Norton WHJ (2019) Reelin Signaling Controls the Preference for Social Novelty in Zebrafish. Front. Behav. Neurosci. 13:214. doi: 10.3389/fnbeh.2019.00214
Received: 04 April 2019; Accepted: 30 August 2019;
Published: 19 September 2019.
Edited by:
Allan V. Kalueff, Saint Petersburg State University, RussiaReviewed by:
Eckart Förster, Ruhr University Bochum, GermanyCopyright © 2019 Dalla Vecchia, Di Donato, Young, Del Bene and Norton. This is an open-access article distributed under the terms of the Creative Commons Attribution License (CC BY). The use, distribution or reproduction in other forums is permitted, provided the original author(s) and the copyright owner(s) are credited and that the original publication in this journal is cited, in accordance with accepted academic practice. No use, distribution or reproduction is permitted which does not comply with these terms.
*Correspondence: William H. J. Norton, d2hqbjFAbGUuYWMudWs=
Disclaimer: All claims expressed in this article are solely those of the authors and do not necessarily represent those of their affiliated organizations, or those of the publisher, the editors and the reviewers. Any product that may be evaluated in this article or claim that may be made by its manufacturer is not guaranteed or endorsed by the publisher.
Research integrity at Frontiers
Learn more about the work of our research integrity team to safeguard the quality of each article we publish.