- 1Department of Psychology, Memorial University of Newfoundland, St. John's, NL, Canada
- 2Laboratory for Neurobiology of Synapse, RIKEN Brain Science Institute, 2-1 Hirosawa, Wako-shi, Saitama, Japan
We generated transgenic mice in which a trans-synaptic tracer, wheat germ agglutinin (WGA), was specifically expressed in the locus coeruleus (LC) neurons under the control of the dopamine-β-hydroxylase (DBH) gene promoter. WGA protein was produced in more than 95% of the tyrosine hydroxylase (TH)-positive LC neurons sampled. Transynaptic transfer of WGA was most evident in CA3 neurons of the hippocampus, but appeared absent in CA1 neurons. Faint but significant WGA immunoreactivity was observed surrounding the nuclei of dentate granule cells. Putative hilar mossy cells, identified by the presence of calretinin in the ventral hippocampus, appeared uniformly positive for transynaptically transferred WGA protein. GAD67-positive interneurons in the hilar and CA3 regions tended to be WGA-positive, although a subset of them did not show WGA co-localization. The same mixed WGA uptake profile was apparent when examining co-localization with parvalbumin. The selective uptake of WGA by dentate granule cells, mossy cells, and CA3 pyramidal neurons is consistent with evidence for a large proportion of conventional synapses adjacent to LC axonal varicosities in these regions. The lack of WGA uptake in the CA1 region and its relatively sparse innervation by DBH-positive fibers suggest that a majority of the TH-positive classical synapses revealed by electron microscopy in that region may be producing dopamine. The overall pattern of WGA uptake in these transgenic mice implies a selective role for the granule cell-mossy cell-CA3 network in processing novelty or the salient environmental contingency changes signaled by LC activity.
Introduction
Functionally both the locus coeruleus (LC) (e.g., Aston-Jones and Bloom, 1981) and the hippocampus (e.g., Wickelgren and Isaacson, 1963; O'Keefe, 1976) have been implicated in adapting to changed environmental contingencies. LC tonic activity is associated with level of arousal, while LC phasic activity reflects input novelty and salience (Aston-Jones and Bloom, 1981). LC-activation-induced plasticity has been extensively characterized in dentate gyrus (DG) (e.g., Kitchigina et al., 1997; Straube et al., 2003; and reviewed by Sara, 2009; and Harley, 2007) and is also described for hippocampal pyramidal cell fields (Lemon et al., 2009). We have hypothesized that a major modification of hippocampal encoding can be initiated and triggered by LC activation (Brown et al., 2005).
The present study uses a novel molecular technology developed by Yoshihara to ask whether this functional association between LC and hippocampus is reflected in transynaptic communication between the two structures. Wheat germ agglutin (WGA) transgene is a genetic tool for the visualization of “anatomically connected and functionally related neural structures” (Yoshihara et al., 1999). By expressing WGA transgene under the control of neuron type-specific promoters in transgenic mice, neural connectivity patterns were successfully visualized in point-to-point sensory pathways such as those of taste (Damak et al., 2008; Matsumoto et al., 2009; Ohmoto et al., 2008, 2010), olfaction (Horowitz et al., 1999), and vision (Hanno et al., 2003), and motor circuits such as those of the cerebellum (Yoshihara et al., 1999). In all cases, this methodology has confirmed results from classical tract tracing studies and permitted insights not previously attained with classical methods.
The LC was first revealed to be the major source of noradrenergic modulation in the rodent forebrain when fluorescent aldehyde visualization methods permitted detailed examination of its axonal projections. LC axons project to neocortex, hippocampus, amygdala, septum, thalamus, hypothalamus, cerebellum, and to spinal cord (Foote et al., 1983). The LC projection patterns in mouse are described as almost identical to those in rat (Levitt and Noebels, 1981).
Radiographic methodology in rat has provided a quantitative estimate of 300,000 neocortical axonal varicosities (sites of noradrenaline release) per LC neuron (Audet et al., 1988). Given the collateralization of LC neurons (Foote et al., 1983), the number of release sites supported by a given LC neuron is likely considerably higher. Electron microscopic examination suggests the majority of LC neocortical varicosities do not make conventional postsynaptic contacts, instead influencing noradrenergic receptors through what is termed “volume conduction” (Seguela et al., 1990; Latsari et al., 2002). This pattern of termination appears characteristic of all arousal-associated neuromodulator systems (Vizi, 2000).
Electron microscopic examination of tyrosine hydroxylase (TH)-reactive varicosities in hippocampus suggests a larger proportion of classical synapses for noradrenergic terminals in CA3 and DG than reported in neocortex (Milner and Bacon, 1989).
In the present study, we generated transgenic mice harboring WGA transgene conjugated to a dopamine-β-hydroxylase (DBH) gene promoter to examine neural connectivity from the LC. In the mouse line examined, WGA was faithfully and robustly produced in LC neurons. However, transynaptic transfer of WGA did not occur widely throughout the neuraxis, arguing against efficient uptake at sites of volume conduction. Robust uptake was observed in CA3 pyramidal cells of the hippocampus and in the DG and hilar regions, implying strong synaptic connections from LC to these hippocampal subregions.
Materials and Methods
DBH-WGA Transgenic Mouse Generation
Transgene construction
The C terminus-truncated WGA cDNA (Yoshihara et al., 1999) was fused with IRES-eYFPmem (internal ribosomal entry site, enhanced yellow fluorescent protein with a membrane-targeting signal, Clontech) to generate a bicistronic expression cassette, tWGA-IRES-eYFPmem. A human DBH promoter region (4 kb) was excised with XbaI from pDBH-GAL (a gift from Dr. Kobayashi, Fukushima Medical University) and inserted into the SpeI site of pBstN, which contains a human β-globin gene intron and SV40 polyadenylation signal, to generate pBstN-DBH. The tWGA-IRES-eYFPmem cassette was ligated into the EcoRI site of pBstN-DBH to generate pDBH-WGA-IRES-eYFPmem.
Generation of transgenic mice
Transgenic mouse lines were generated by microinjection of fertilized eggs as described (Nohmi et al., 1996). The purified inserts of pDBH-WGA-IRES-eYFPmem were injected into the male pronucleus of fertilized eggs from the FVB/N (Japan CLEA) strain of mice. The manipulated eggs were cultured and transferred into the oviducts of ICR (CREA Japan) pseudopregnant recipients. Integration of the transgenes was screened by PCR analysis of tail DNA. All animal experiments were approved by the Animal Care and Use Committees of RIKEN.
Preparation of Tissue
Animals were anesthetized (sodium pentobarbital; 50 mg/kg, i.p.) and perfused transcardially with saline (0.9%) followed by 4% paraformaldehyde in PBS. Brains were dissected out and immersed in the same fixative (24 h, 4°C), cryoprotected in 30% sucrose, flash frozen (−76°C), and sectioned by cryostat in the coronal plane (30 μm).
Immunohistochemical Procedures
Brightfield diaminobenzodine (DAB) processing: Sections were washed in 0.1 M trishydroxymethyl-amino methane (Tris buffer; pH 7.6, 3 × 5 min) followed by 30 min in H2O2 (1%, in Tris buffer) to block endogenous peroxidase activity. Sections were again washed in Tris buffer (5 min) followed by 10 min in both Tris buffer containing 0.1% Triton X-100 (Tris A) and Tris buffer containing 0.1% Triton X-100 and 0.005% bovine serum albumin (Tris B). Sections were then treated with 10% normal horse serum made in Tris B containing avidin (Blocking Kit; Vector Labs) followed by 10 min each in Tris A, then Tris B. Preparation of the goat antiserum to WGA (Vector) consisted of preabsorption of the antibody (1:2000 in Tris B) with 2% mouse brain acetone powder (Rockland, Gilbertsville, PA) for 12–24 h at 4°C. The antibody slurry was centrifuged for 10 min (14,000 RPM) and the supernatant filtered using a 0.22 μm filter (Millex-GS; Millipore Corp., Bedford, MA) and biotin (Blocking Kit; Vector) was added to constitute the final antibody solution. Sections were incubated with anti-WGA antibody for 24–48 h (4–8°C) with gentle agitation, then washed 10 min each in Tris A and Tris B. Sections were then treated with biotin-conjugated anti-goat secondary antibody (horse IgG, 1:400 in Tris B; Vector) for 60 min at room temperature then washed in Tris A and Tris B (10 min each) followed by goat peroxidase anti-peroxidase (Jackson Immunoresearch; 1:500 in Tris B) for 60 min at room temperature. After washes in Tris A and Tris D (0.5 M Tris containing 0.1% Triton X and 0.005% BSA), sections were incubated for 2 h in avidin-biotin-horseradish peroxidase complex (ABC Elite Kit; Vector; 1:1000) made in Tris D. Sections were briefly washed in Tris buffer (3 × 5 min) and visualized using nickel-enhanced DAB (Sigma; St. Louis). Sections were mounted on subbed slides, air dried, dehydrated, cleared, and coverslipped with Microkitt (Serum International Inc, Montreal, QC). In the case of WGA-parvalbumin double-labeling, parvalbumin antibody (mouse monoclonal; 1:100,000; Sigma) was visualized with SG Gray (Vector).
Immunofluorescence tissue preparation
Preparation of tissue for double-label confocal microscopy consisted of sequential incubation in anti-WGA solution using the above procedures (with the exclusion of H2O2) and either rabbit anti-TH (1:2500, Calbiochem), mouse anti-parvalbumin (1:100,000; Sigma), or mouse anti-calretinin (1:10,000; Chemicon), in Tris B. WGA was visualized using donkey Alexa555-conjugated anti-goat IgG (Invitrogen; 1:400 in Tris B). All other primary antibodies were visualized using Alexa 647 (Invitrogen) targeted at the host species of the primary antibody (1:400, in Tris B). To insure no contamination from the eYFPmem signal, fluorescence secondary antibodies were chosen in the far red spectrum. The eYFPmem fluorescence was not analyzed as its signal occurred at low levels in hippocampus. Sections were mounted and covered with Slow Fade Light with DAPI (Molecular Probes; Eugene, OR).
Brightfield images of WGA immunoreactivity in the hippocampus were captured on an Olympus (Richmond Hill, ON) BX51 digital imaging system. An Olympus FV300 confocal digital microimaging system was used to capture fluorescence-labeled sections. Images were compiled using Adobe Photoshop software (San Jose, CA).
Results
LC Noradrenergic Neurons Express WGA in DBH-WGA Transgenic Mice
TH-positive neurons in the LC were quantitatively examined for WGA expression in five male DBH-WGA transgenic mice using confocal microscopy. Figure 1 shows an example of LC staining for the TH antibody, the WGA antibody, and their co-localization in one representative cross-section. Comparison with a non-transgenic mouse cross-section processed under the same conditions reveals no cellular reactivity for WGA, as expected.
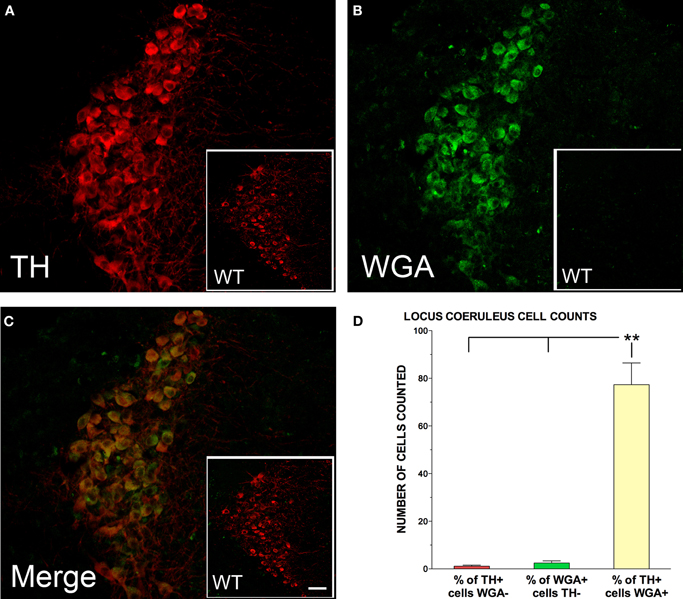
Figure 1. Tyrosine hydroxylase (TH; red) and wheat germ agglutinin (WGA; green) immunolabelling of the locus coeruleus (LC) for representative DBH-WGA (A–C) and wildtype (WT; A–C inset) mice. (C) TH-positive neurons in the LC of DBH-WGA mice were also positive for WGA. LC neurons of wildtype mice were not immunoreactive for WGA (C inset). (D) Percentage of LC cells in DBH-WGA mice immunoreactive for TH only (red), or WGA only (green) and immunoreactive for both TH and WGA (yellow). Error bars represent s.e.m. and ** indicates min p < 0.001 differences (paired t-test; n = 5). Scale represents 150 μm in inset, and 30 μm in main DBH-WGA images.
LC sections in the transgenic mice were sampled along the rostral-caudal axis with 2–4 sections assessed for each mouse. A mean of 77 cells was counted per section. The mean percentage of WGA/TH-double-positive neurons in each section was 98.7%. The remaining 1.3% of TH-expressing cells was negative for WGA. The pattern was the same in two transgenic female mice examined. The percentage of cells in each labeling category for the five male mice is shown in Figure 1D. Paired t-tests evaluated the differences.
Transynaptic Transfer of WGA from LC to Specific Subsets of Hippocampal Neurons
The hippocampus was initially examined for transynaptic transfer of WGA from the axon terminals of LC neurons using a DAB reaction for the WGA antibody. Within the hippocampus, and relative to the extra-hippocampal forebrain, the CA3 pyramidal neurons were the most strongly reactive elements for the WGA antibody in the DBH-WGA mice (Figures 2A,C). CA1 pyramidal cells did not appear reactive. Non-transgenic mice did not show WGA immunoreactivity (Figures 2B,D). This pattern was confirmed in confocal images with fluorescent antibodies in all transgenic mice examined (n = 8, see Figures 3–5).
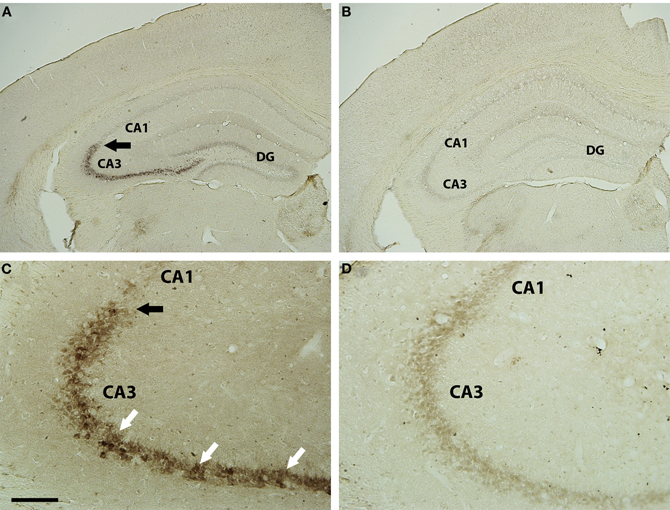
Figure 2. Brightfield WGA immunostaining in hippocampus of DBH-WGA (A,C) and wild type (B,D) mice. CA3 pyramidal cell bodies (A) were heavily filled with reaction product for WGA (white arrows in C). There was a clear demarcation at the CA3 border, with adjacent pyramidal neurons lacking reaction product (black arrows). Pyramidal neurons throughout the hippocampus of wild type mice lacked WGA reaction product. Scale bar is 500 μm in A,B and 100 μm in C,D.
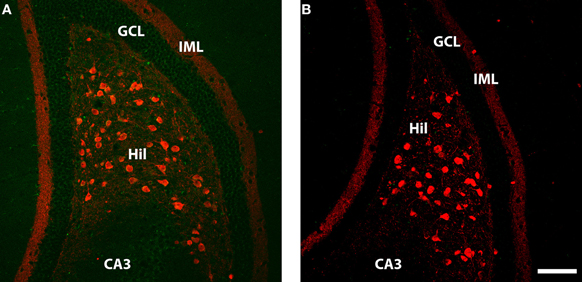
Figure 3. Calretenin (red) and WGA (green) immunolabelling in ventral dentate gyrus of DBH-WGA (A) and wildtype (B) mice. (A) Calretenin+/WGA+ double immunolabeling is evident in the majority of large hilar neurons, putative mossy cells known to contain calretenin in ventral mouse hippocampus. WGA staining in CA3 and the granule cell layer (GCL) in also evident. (B) No double-labeled cells or WGA-positive cells occur in the wild type mouse. Scale is 100 μm.
Double immunofluoescence labeling revealed that WGA protein was present in all calretinin-positive hilar cells (n = 7 transgenic mice examined; see Figure 3A). The calretinin-expressing large hilar cells of the ventral hippocampus have been identified as glutamatergic mossy cells in the mouse (Liu et al., 1996; Blasco-Ibanez and Freund, 1997; Fujise et al., 1998). WGA protein was also detected in the DG granule cells (Figure 4A). WGA-reactive CA3 pyramidal cells were again readily observed. Sections from a non-transgenic mouse did not show evidence of WGA in any cells (Figure 3B).
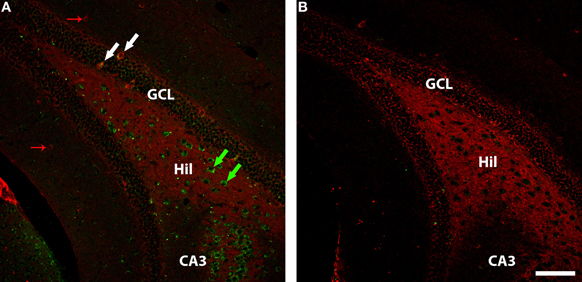
Figure 4. GAD-67 (red) and WGA (green) immunolabelling in ventral dentate gyrus of DBH-WGA (A) and wildtype (B) mice. (A) Co-labelling of GAD67 and WGA is seen among granule cell interneurons (e.g., white arrows). GAD-67+ only interneurons are seen in the molecular layer (small red arrows). WGA+ hilar interneurons void of GAD67 staining are putative glutamatergic mossy cells. Scale is 100 μm.
Subsets of Gabaergic Interneurons in DG and CA3 are Positive for WGA
In the DG, GAD67-expressing GABAergic interneurons in or near the cell body layer were generally positive for WGA in sections from the five transgenic mice (see Figure 4 granule cell layer). However, GAD67-positive interneurons in the molecular layer of DG were negative for WGA (Figure 4 molecular layer). The large number of WGA-positive/GAD67-negative hilar interneurons seen in ventral hippocampus in Figure 4 is consistent with the observation of calretinin/WGA-double-positive putative glutamatergic mossy cells (Figure 3). The paucity of GAD67-positive interneurons seen in CA3 precludes generalizations, but both positive and negative co-localization was observed.
In other sections from transgenic mice (n = 3), a parvalbumin antibody was used to highlight a subset of GABAergic interneurons. As with the overall set of GAD67-positive interneurons, there were both WGA-positive and WGA-negative parvalbumin-expressing cells. In Figure 6A, parvalbumin-expressing interneurons in CA3 that are WGA-positive are indicated with white arrows, whereas other parvalbumin-expressing interneurons were observed to be WGA-negative (indicated with a red arrow). A similar pattern was seen in the DG, as shown in Figure 6B. No WGA-positive cells were seen in the parvalbumin-expressing population in layer CA1 (Figure 5C) consistent with the general failure to see WGA reactivity in the CA1 layer.
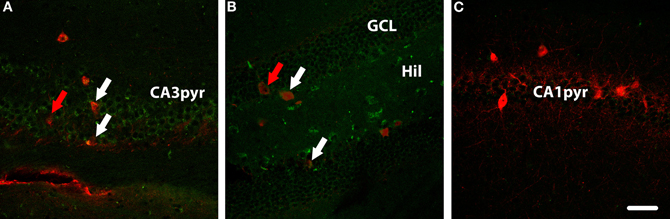
Figure 5. Parvalbumin (red) and WGA (green) immunolabelling in hippocampus of a DBH-WGA mouse. (A) In CA3, both parvalbumin+/WGA+ interneurons (white arrows) and parvalbumin+/WGA− interneurons (red arrow) were found. In dentate gyrus (B) the same patterns were seen. (C) In CA1, only parvalbumin+/WGA− interneruons were observed. Scale is 50 μm.
Using brightfield images double-labeled for parvalbumin and WGA, the same mixed pattern of WGA-positive and negative interneurons was seen in DG and CA3. In Figure 6A a low power micrograph shows parvalbumin-expressing cells in all areas of the hippocampus, again the strongest WGA immunoreactivity was seen in the CA3 pyramidal layer. Co-localization of parvalbumin and WGA was not seen at higher power in CA1 (Figure 6B), but occurred in some parvalbumin-expressing interneurons in CA3 (Figure 6C) and DG (Figure 6D).
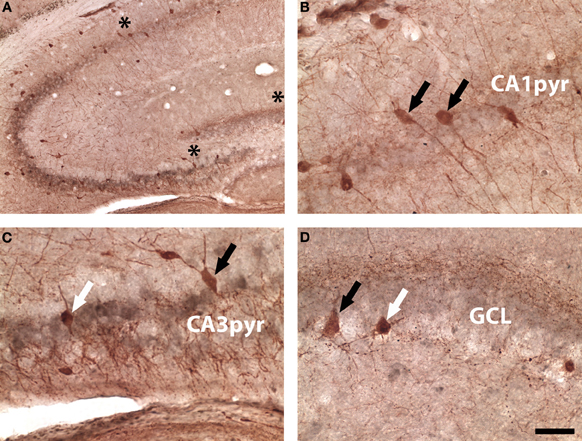
Figure 6. Brightfield parvalbumin (red) and WGA (gray) labeling in a DBH-WGA mouse. (A) Low magnification image showing CA3-WGA pattern. (B) CA1 parvalbumin-only reactivity (black arrows). (C) Interneurons in CA3 were both parvalbumin+/WGA+ (white arrow) and parvalbumin+/WGA− (black arrow). (D) A similar mixed pattern occurred in DG. Asterisks in A denote regions sampled in B–D Scale is 50 μm except A = 100 μm.
Discussion
The nearly complete filling of TH-positive cells in LC with WGA confirmed the success of the transgenic line examined. The large number of LC varicosities that do not make classical synaptic contacts might have either precluded significant amounts of WGA uptake or rendered WGA uptake widespread. Instead we found a highly selective hippocampal WGA uptake pattern. Although not systematically examined, there was relatively little evidence, at low magnification, of forebrain uptake in extra-hippocampal structures. It seems unlikely that WGA would not have been detected with our confocal methodology, however, we cannot rule out the possibility that higher copy numbers of the transgene in another mouse line or more sensitive methods than those used would reveal more extensive WGA uptake from LC innervations than seen here. Widespread uptake of 3,3′,5-triiodothyronine in forebrain neurons, for example, has been reported as occurring via anterograde synaptic transport from LC (Gordon et al., 1999) suggesting it acted as a marker of volume conduction. Multi-synaptic transport of WGA has also been observed in other systems (Yoshihara, 2002), but was not apparent in the CA3–CA1 connection, for example, in the present experiments. This suggests transport here was dependent on only initial synaptic contact.
WGA Uptake in the Hippocampal Trisynaptic Pathway
WGA protein was most abundant in the cytoplasm of CA3 pyramidal neurons. This was evident in both light and confocal microscope images. Despite the strong Schaffer collateral connections of CA3 neurons with CA1 pyramidal cells, there was no evidence of secondary transynaptic transport of WGA into CA1 pyramidal neurons.
In fluorescent images, rings of cytoplasmic WGA were seen around granule cell nuclei. The narrow cytoplasmic shell likely contributed to less evident filling of granule cells with WGA in light microscope images, although rings of WGA could be seen at higher power in numerous sections.
The apparent absence of WGA in CA1 pyramidal cells suggests the nature of LC varicosity contacts differs between CA3, DG, and CA1. While the proportion of classical TH terminals in CA1 has been reported as similar to that of CA3 and DG (Milner and Bacon, 1989), CA1, unlike those areas, receives a minor DBH-reactive input consistent with the observed absence of WGA uptake (Swanson and Hartman, 1975; Moudy et al., 1993), but has a significant dopamine input (Gasbarri et al., 1997), which would entail TH-positive fibers.
WGA Uptake in Hilar Interneurons
GABAergic interneurons in the DG and hilar region showed selective uptake of WGA. While numerous GAD67-positive interneurons were positive for WGA, there were also negative subpopulations. GABAergic interneurons located near or in the granule cell layer often showed WGA uptake, while those in the molecular layer were typically unreactive for WGA. LC activation when recording from GABAergic interneurons near granule cells reveals a rapid onset, short-term inhibitory effect on feedforward interneurons (Brown et al., 2005) Feedback interneurons show both excitation and inhibition to LC activation. Molecular layer interneurons have not been recorded with this approach.
The parvalbumin subset of GABAergic interneurons was examined in DG and CA3. In both areas, parvalbumin cells positive for WGA and negative for WGA were observed. These variable effects occurred in parvalbumin cells near and among the cell layers, suggesting WGA uptake by cell layer GABAergic interneurons is not uniform. A more comprehensive survey of inhibitory interneurons identified with peptidergic markers would be valuable. While not examined systematically in confocal images, no double-labeled parvalbumin interneurons were found in the adjacent CA1 region consistent again with a general lack of WGA uptake in CA1.
Parvalbumin cells (Wulff et al., 2009) and feedback interneurons at the cell layers have been associated with theta oscillation generation. LC activation recruits a brief and selective activation of ~7.7 Hz theta oscillation in awake rats (Walling et al., 2011). A similar frequency has been reported in the hippocampus with exposure to novel environments and may depend on the LC (Jeewajee et al., 2008). The rapid onset and transient electrophysiological effects of LC activation in hippocampus on feedforward and feedback networks may be associated with classical synaptic contacts. However, volume conducting systems have also been reported to elicit similarly transient patterns of electrophysiology (Sarter et al., 2009).
The mossy cell glutamatergic interneurons of the ventral hilus were strongly labeled for WGA. Calretinin is localized in the mossy cells of ventral DG in the mouse, providing an identifying marker for mossy cells (Liu et al., 1996; Blasco-Ibanez and Freund, 1997; Fujise et al., 1998). Co-localization of WGA with calretinin was evident in all slides reacted for calretinin. WGA-only hilar cells were also widespread in sections reacted for GABAergic markers, which is easily understood if WGA was taken up by glutamatergic hilar interneurons.
Classical Synaptic LC Terminals in Hippocampus
The proportion of classical synapses on neuronal cell dendrites and cell bodies for TH-positive fibers in DG and CA3 is more than 50% of the terminals examined using electron microscopy (Milner and Bacon, 1989). This proportion of classical contacts is considerably higher than reported for LC varicosities in neocortex (Vizi, 2000) and may account for the selective pattern of WGA uptake observed here, particularly considering evidence for strong WGA binding to synaptic glycoproteins (Wood et al., 1981). The receptor subtype opposite classical synapses in the DG and CA3 areas is likely to be the α1 receptor, as neither hippocampal α2 (Milner et al., 1998) nor β-adrenergic receptor sites (Milner et al., 2000) are characterized by classical synaptic connectivity. Relatively little is known about functional effects of hippocampal α1 activation in behaving rodents.
A Novelty-Encoding Subnetwork
Based on the extensive co-localization of LC-WGA in the glutamatergic cells of DG, hilus, and CA3, including both principle cells and interneurons, we suggest these components of the hippocampal network make a unique contribution to the detection and encoding of novel events. This pattern supports other observations revealing a selective role for CA3 in rapid one trial contextual learning (McHugh et al., 2007). The data suggest that transynaptic modulation acts in parallel with the contributions of the volume-conducted β-adrenergic system to promote adaptation to novel events. We (Brown et al., 2005), and others (Bouret and Sara, 2005), hypothesize that a central role of the LC signal in hippocampus is a resetting of cognitive maps when novel or unexpected events occur. The present connectivity patterns are consistent with such a hypothesis.
Conflict of Interest Statement
The authors declare that the research was conducted in the absence of any commercial or financial relationships that could be construed as a potential conflict of interest.
Acknowledgments
The authors would like to acknowledge Dr. Kazuto Kobayashi (Fukushima Medical University) for the DBH gene promoter plasmid and Michiko Saito and Sachiko Mitsui (RIKEN BSI) for technical assistance in generation of the DBH-WGA transgenic mice. This work was supported in part by a Grant-in-Aid for Scientific Research from the Ministry of Education, Culture, Sports, Science and Technology of Japan to Yoshihiro Yoshihara. Support from the Natural Sciences and Engineering Research Council to Carolyn W. Harley (Grant A9794) is also gratefully acknowledged.
References
Aston-Jones, G., and Bloom, F. E. (1981). Norepinephrine-containing locus coeruleus neurons in behaving rats exhibit pronounced responses to non-noxious environmental stimuli. J. Neurosci. 1, 887–900.
Audet, M. A., Doucet, G., Oleskevich, S., and Descarries, L. (1988). Quantified regional and laminar distribution of the noradrenaline innervation in the anterior half of the adult rat cerebral cortex. J. Comp. Neurol. 274, 307–318.
Blasco-Ibanez, J. M., and Freund, T. F. (1997). Distribution, ultrastructure, and connectivity of calretinin-immunoreactive mossy cells of the mouse dentate gyrus. Hippocampus 7, 307–320.
Bouret, S., and Sara, S. J. (2005). Network reset: a simplified overarching theory of locus coeruleus noradrenaline function. Trends Neurosci. 28, 574–582.
Brown, R. A., Walling, S. G., Milway, J. S., and Harley, C. W. (2005). Locus ceruleus activation suppresses feedforward interneurons and reduces beta-gamma electroencephalogram frequencies while it enhances theta frequencies in rat dentate gyrus. J. Neurosci. 25, 1985–1991.
Damak, S., Mosinger, B., and Margolskee, R. F. (2008). Transsynaptic transport of wheat germ agglutinin expressed in a subset of type II taste cells of transgenic mice. BMC Neurosci. 9, 96.
Foote, S. L., Bloom, F. E., and Aston-Jones, G. (1983). Nucleus locus ceruleus: new evidence of anatomical and physiological specificity. Physiol. Rev. 63, 844–914.
Fujise, N., Liu, Y., Hori, N., and Kosaka, T. (1998). Distribution of calretinin immunoreactivity in the mouse dentate gyrus: II. Mossy cells, with special reference to their dorsoventral difference in calretinin immunoreactivity. Neuroscience 82, 181–200.
Gasbarri, A., Sulli, A., and Packard, M. G. (1997). The dopaminergic mesencephalic projections to the hippocampal formation in the rat. Prog. Neuropsychopharmacol. Biol. Psychiatry 21, 1–22.
Gordon, J. T., Kaminski, D. M., Rozanov, C. B., and Dratman, M. B. (1999). Evidence that 3,3′,5-triiodothyronine is concentrated in and delivered from the locus coeruleus to its noradrenergic targets via anterograde axonal transport. Neuroscience 93, 943–954.
Hanno, Y., Nakahira, M., Jishage, K., Noda, T., and Yoshihara, Y. (2003). Tracking mouse visual pathways with WGA transgene. Eur. J. Neurosci. 18, 2910–2914.
Horowitz, L. F., Montmayeur, J. P., Echelard, Y., and Buck, L. B. (1999). A genetic approach to trace neural circuits. Proc. Natl. Acad. Sci. U.S.A. 96, 3194–3199.
Jeewajee, A., Lever, C., Burton, S., O'Keefe, J., and Burgess, N. (2008). Environmental novelty is signaled by reduction of the hippocampal theta frequency. Hippocampus 18, 340–348.
Kitchigina, V., Vankov, A., Harley, C., and Sara, S. J. (1997). Novelty-elicited, noradrenaline-dependent enhancement of excitability in the dentate gyrus. Eur. J. Neurosci. 9, 41–47.
Latsari, M., Dori, I., Antonopoulos, J., Chiotelli, M., and Dinopoulos, A. (2002). Noradrenergic innervation of the developing and mature visual and motor cortex of the rat brain: a light and electron microscopic immunocytochemical analysis. J. Comp. Neurol. 445, 145–158.
Lemon, N., Aydin-Abidin, S., Funke, K., and Manahan-Vaughan, D. (2009). Locus coeruleus activation facilitates memory encoding and induces hippocampal LTD that depends on beta-adrenergic receptor activation. Cereb. Cortex 19, 2827–2837.
Levitt, P., and Noebels, J. L. (1981). Mutant mouse tottering: selective increase of locus ceruleus axons in a defined single-locus mutation. Proc. Natl. Acad. Sci. U.S.A. 78, 4630–4634.
Liu, Y., Fujise, N., and Kosaka, T. (1996). Distribution of calretinin immunoreactivity in the mouse dentate gyrus. I. General description. Exp. Brain Res. 108, 389–403.
Matsumoto, I., Ohmoto, M., Yasuoka, A., Yoshihara, Y., and Abe, K. (2009). Genetic tracing of the gustatory neural pathway originating from T1R3-expressing sweet/umami taste receptor cells. Ann. N.Y. Acad. Sci. 1170, 46–50.
McHugh, T. J., Jones, M. W., Quinn, J. J., Balthasar, N., Coppari, R., Elmquist, J. K., Lowell, B. B., Fanselow, M. S., Wilson, M. A., and Tonegawa, S. (2007). Dentate gyrus NMDA receptors mediate rapid pattern separation in the hippocampal network. Science 317, 94–99.
Milner, T. A., and Bacon, C. E. (1989). Ultrastructural localization of tyrosine hydroxylase-like immunoreactivity in the rat hippocampal formation. J. Comp. Neurol. 281, 479–495.
Milner, T. A., Lee, A., Aicher, S. A., and Rosin, D. L. (1998). Hippocampal alpha2a-adrenergic receptors are located predominantly presynaptically but are also found postsynaptically and in selective astrocytes. J. Comp. Neurol. 395, 310–327.
Milner, T. A., Shah, P., and Pierce, J. P. (2000). Beta-adrenergic receptors primarily are located on the dendrites of granule cells and interneurons but also are found on astrocytes and a few presynaptic profiles in the rat dentate gyrus. Synapse 36, 178–193.
Moudy, A. M., Kunkel, D. D., and Schwartzkroin, P. A. (1993). Development of dopamine-beta-hydroxylase-positive fiber in-nervation of the rat hippocampus. Synapse 15, 307–318.
Nohmi, T., Kato, M., Suzuki, H., Matsui, M., Yamada, M., Watanabe, M., Suzuki, M., Horiya, N., Ueda, O., Shibuya, T., Ikeda, H., and Sofuni, T. (1996). A new transgenic mouse mutagenesis test system using Spi- and 6-thioguanine selections. Environ. Mol. Mutagen. 28, 465–470.
Ohmoto, M., Maeda, N., Abe, K., Yoshihara, Y., and Matsumoto, I. (2010). Genetic tracing of the neural pathway for bitter taste in t2r5-WGA transgenic mice. Biochem. Biophys. Res. Commun. 400, 734–738.
Ohmoto, M., Matsumoto, I., Yasuoka, A., Yoshihara, Y., and Abe, K. (2008). Genetic tracing of the gustatory and trigeminal neural pathways originating from T1R3-expressing taste receptor cells and solitary chemoreceptor cells. Mol. Cell. Neurosci. 38, 505–517.
O'Keefe, J. (1976). Place units in the hippocampus of the freely moving rat. Exp. Neurol. 51, 78–109.
Sara, S. J. (2009). The locus coeruleus and noradrenergic modulation of cognition. Nat. Rev. Neurosci. 10, 211–223.
Sarter, M., Parikh, V., and Howe, W. M. (2009). Phasic acetylcholine release and the volume transmission hypothesis: time to move on. Nat. Rev. Neurosci. 10, 383–390.
Seguela, P., Watkins, K. C., Geffard, M., and Descarries, L. (1990). Noradrenaline axon terminals in adult rat neocortex: an immunocytochemical analysis in serial thin sections. Neuroscience 35, 249–264.
Straube, T., Korz, V., Balschun, D., and Frey, J. U. (2003). Requirement of beta-adrenergic receptor activation and protein synthesis for LTP-reinforcement by novelty in rat dentate gyrus. J. Physiol. 552, 953–960.
Swanson, L. W., and Hartman, B. K. (1975). The central adrenergic system. An immunofluorescence study of the location of cell bodies and their efferent connections in the rat utilizing dopamine-beta-hydroxylase as a marker. J. Comp. Neurol. 163, 467–505.
Vizi, E. S. (2000). Role of high-affinity receptors and membrane transporters in nonsynaptic communication and drug action in the central nervous system. Pharmacol. Rev. 52, 63–89.
Walling, S. G., Brown, R. A., Milway, J. S., Earle, A. G., and Harley, C. W. (2011). Selective tuning of hippocampal oscillations by phasic locus coeruleus activation in awake male rats. Hippocampus 21, 1250–1262.
Wood, J. G., Byrd, F. I., and Gurd, J. W. (1981). Lectin cytochemistry of carbohydrates on cell membranes of rat cerebellum. J. Neurocytol. 10, 149–159.
Wickelgren, W. O., and Isaacson, R. L. (1963). Effect of the introduction of an irrelevant stimulus on runway performance of the hippocampectomized rat. Nature 200, 48–50.
Wulff, P., Ponomarenko, A. A., Bartos, M., Korotkova, T. M., Fuchs, E. C., Bahner, F., Both, M., Tort, A. B., Kopell, N. J., Wisden, W., and Monyer, H. (2009). Hippocampal theta rhythm and its coupling with gamma oscillations require fast inhibition onto parvalbumin-positive interneurons. Proc. Natl. Acad. Sci. U.S.A. 106, 3561–3566.
Yoshihara, Y. (2002). Visualizing selective neural pathways with WGA transgene: combination of neuroanatomy with gene technology. Neurosci. Res. 44, 133–140.
Yoshihara, Y., Mizuno, T., Nakahira, M., Kawasaki, M., Watanabe, Y., Kagamiyama, H., Jishage, K., Ueda, O., Suzuki, H., Tabuchi, K., Sawamoto, K., Okano, H., Noda, T., and Mori, K. (1999). A genetic approach to visualization of multisynaptic neural pathways using plant lectin transgene. Neuron 22, 33–41.
Keywords: noradrenaline, volume transmission, CA3, CA1, dentate gyrus, GABAergic interneurons, mossy cells, tracing
Citation: Walling SG, Brown RA, Miyasaka N, Yoshihara Y and Harley CW (2012) Selective wheat germ agglutinin (WGA) uptake in the hippocampus from the locus coeruleus of dopamine-β-hydroxylase-WGA transgenic mice. Front. Behav. Neurosci. 6:23. doi: 10.3389/fnbeh.2012.00023
Received: 14 February 2012; Paper pending published: 13 March 2012;
Accepted: 10 May 2012; Published online: 28 May 2012.
Edited by:
Sebastien Bouret, Institut du Cerveau et de la Moelle épinière, FranceCopyright: © 2012 Walling, Brown, Miyasaka, Yoshihara and Harley. This is an open-access article distributed under the terms of the Creative Commons Attribution Non Commercial License, which permits non-commercial use, distribution, and reproduction in other forums, provided the original authors and source are credited.
*Correspondence: Carolyn W. Harley, Department of Psychology, Memorial University of Newfoundland, St. John's, NL, A1B 3X9, Canada. e-mail:Y2hhcmxleUBwbGF5LnBzeWNoLm11bi5jYQ==