- 1 System Emotional Science, Graduate School of Medicine and Pharmaceutical Sciences, University of Toyama, Toyama, Japan
- 2 Department of Neurosurgery, Faculty of Medicine, Kagoshima University, Kagoshima, Japan
- 3 Integrative Neuroscience, Graduate School of Medicine and Pharmaceutical Sciences, University of Toyama, Toyama, Japan
- 4 Judo Neurophysiotherapy, Graduate School of Medicine and Pharmaceutical Sciences, University of Toyama, Toyama, Japan
It has been suggested that septal nuclei are important in the control of behavior during various reward and non-reward situations. In the present study, neuronal activity was recorded from rat septal nuclei during discrimination of conditioned sensory stimuli (CSs) of the medial forebrain bundle associated with or without a reward (sucrose solution or intracranial self-stimulation, ICSS). Rats were trained to lick a spout protruding close to the mouth just after a CS to obtain a reward stimulus. The CSs included both elemental and configural stimuli. In the configural condition, the reward contingency of the stimuli presented together was opposite to that of each elemental stimulus presented alone, although the same sensory stimuli were involved. Of the 72 responsive septal neurons, 18 responded selectively to the CSs predicting reward (CS+-related), four to the CSs predicting non-reward (CS0-related), nine to some CSs predicting reward or non-reward, and 15 non-differentially to all CSs. The remaining 26 neurons responded mainly during the ingestion/ICSS phase. A multivariate analysis of the septal neuronal responses to elemental and configural stimuli indicated that septal neurons encoded the CSs based on reward contingency, regardless of the stimulus physical properties and were categorized into three groups; CSs predicting the sucrose solution, CSs predicting a non-reward, and CSs predicting ICSS. The results suggest that septal nuclei are deeply involved in discriminating the reward contingency of environmental stimuli to manifest appropriate behaviors in response to changing stimuli.
Introduction
Septal nuclei receive afferents from various areas in the limbic system not only from the hippocampal formation, but also from the amygdala, entorhinal, and cingulate cortex, which are all involved in various higher brain functions such as cognition and memory. In turn, the septal nuclei send efferents to the hypothalamus and brainstem, which play an important role in emotional expression or behavioral manifestations such as emotional behavior, hormone release, and autonomic reactions (see review by Risold and Swanson, 1997b). Thus, the septal region acts as an interface between these higher cognitive and lower executive systems (Swanson et al., 1987).
Septal nuclei have a functionally strong relations to the hippocampal formation and hypothalamus. Septal or fornical lesions impair spatial learning and memory (Thomas and Gash, 1986; Numan and Quaranta Jr., 1990; Gaffan et al., 1991), which is comparable to the effects of hippocampal lesions (Gray, 1987). Furthermore, septal lesions alter food and water intake, which is also observed after hypothalamic lesions (Harvey and Hunt, 1965; Lorens and Kondo, 1969; Stoller, 1972). Electrically stimulating septal nuclei results in lowered arousal, hypoactivity, reduced rates of response, and even sleep and induces autonomic responses such as decreased blood pressure, cardiac deceleration, and inhibition of pituitary–adrenal activity as well as somatomotor effects in rats and cats (Malmo, 1961, 1965; Covian et al., 1964; Holdstock, 1967; Baldino et al., 1988). Furthermore, abnormalities in the septal region have been associated with schizophrenia and depression (Zeman and King, 1958; Averback, 1981; Brisch et al., 2011). Evidence suggests that septal nuclei control emotional behavior and autonomic and hormonal functions by modulating hypothalamic and lower brainstem activity, based on higher cognitive and mnemonic information received through reciprocally dense connections with other areas of the limbic system including the hippocampal formation.
Furthermore, the septal region was the first area where intracranial self-stimulation (ICSS) behavior was observed in rats (Olds and Milner, 1954). Septal nuclei have intimate connections to brain regions involved in goal-directed behaviors for various reward, such as the nucleus accumbens, medial prefrontal cortex, and ventral tegmental area (VTA) and are implicated in amphetamine, morphine, phencyclidine, and lysergic acid diethylamide abuse (Sheehan et al., 2004). These craving behaviors may be mediated partly through an interaction between the septal nuclei and the dopaminergic system (Merrer et al., 2007). Additionally, nearly all antipsychotic antidepressant drugs affect septal neuron activity (Sheehan et al., 2004). Taken together, these results suggest that septal neuron activity is an important determinant of behavioral manifestations during various reinforcing and non-reinforcing situations.
In the present study, neuronal activity was recorded from rat septal nuclei while rats performed elemental and configural association tasks to elucidate the information processing mechanisms in the septal nuclei that control motivated and emotional behaviors. During these tasks, conditioned sensory stimuli (CSs) included both elemental (auditory or visual) and configural (simultaneously presented auditory and visual) stimuli. In one case, each stimulus predicted reward when presented alone but predicted non-reward when presented together. In the other case, each stimulus predicted non-reward when presented alone but predicted reward when presented together. Previous anatomical and behavioral studies suggest that septal nuclei may integrate information from other brain regions to influence behavioral output, particularly during reinforcing situations. If the septal neurons are involved in behavioral output during reinforcing situations, they would respond to the CSs in terms of reward availability regardless of the physical properties of the CSs.
Materials and Methods
Subjects
Twenty-six male albino Wistar rats, weighing 270–330 g (10–16 weeks old; SLC, Hamamatsu, Japan), were used. The housing area was temperature controlled at 23°C and maintained on a 12-h light–dark cycle. Prior to surgery, rats were individually housed in clean cages with free access to water and laboratory chow. All efforts were made to minimize the number of animals used and their suffering.
Surgery
Surgery was performed under aseptic conditions in two stages. First, a cranioplastic cap was attached to the skull. After a recovery and training period, a permanent indifferent electrode was implanted. The rats were treated in strict compliance with the policies of the National Institutes of Health on the Care of Humans and Laboratory Animals and the Guidelines for the Care and Use of Laboratory Animals at the University of Toyama.
As described in our previous studies (Uwano et al., 1995; Nishijo et al., 1998), the head restraint system of Nishijo and Norgren(1990, 1991, 1997), modified from a method described by Ono et al. (1985), was used. After being anesthetized (sodium pentobarbital, 40 mg/kg, i.p.), the rats were mounted in a stereotaxic apparatus with the skull leveled between the bregma and lambda suture points. The cranium was exposed, and 2–3 mm of the temporal end of the bilateral temporal muscle was removed, and seven small, sterile, stainless screws were threaded into holes in the skull to serve as anchors for cranioplastic acrylic. Stainless-steel wires were soldered onto two screws as a ground. Two bipolar electrodes were implanted into the lateral hypothalamic area (A, −4.3 from bregma; L, ± 1.2; V, 8.4) to intracranially stimulate the medial forebrain bundle, according to the atlas of Paxinos and Watson (1986). After covering the cut end of the temporal muscle with overlying skin, the cranioplastic acrylic was built up on the skull and molded around the conical ends of two sets of stainless-steel bars that had a single steel bar on one end and two bars on the other end. Once the cement had hardened, these bars were removed, leaving a negative impression of the double end on each side of the acrylic block. During subsequent surgery or during a recording session, the double end of these artificial earbars was pressed into the indentations in the acrylic block, while the single end was inserted into the normal earbar slots in the stereotaxic instrument and rigidly attached (Figure 1A). Hence, these artificial earbars served the same function as regular earbars but could be used in unanesthetized animals because they did not involve a painful insertion into the ear canal. A short length of 27-gage stainless-steel tubing was embedded into the cranioplastic acrylic near the bregma to serve as a reference pin during chronic recording. After surgery, an antibiotic (gentamicin sulfate, Gentacin® injection, Schering-Plough, Osaka, Japan) was administered topically and systematically (2 mg, i.m.).
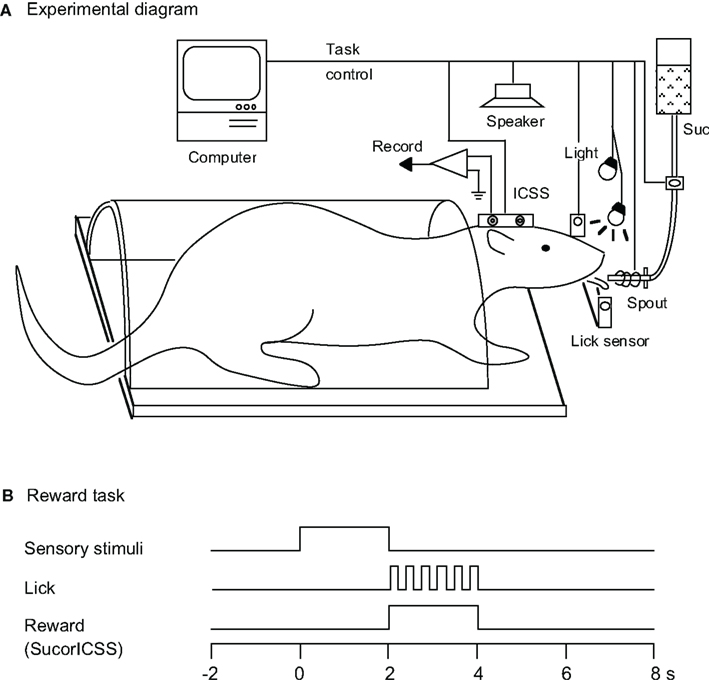
Figure 1. Experimental diagram. (A) Rats were prepared for chronic recording by forming dental cement receptacles to accept fake earbars. Electrodes were implanted in the lateral hypothalamic area for intracranial self-stimulation (ICSS) of the medial forebrain bundle. Rats were trained to lick when the spout was automatically placed close to their mouth. Licking was signaled by a photoelectric sensor triggered by the tongue. Auditory and visual CSs were presented by a speaker above the rat’s head and by a light in front of each eye, respectively. A sucrose solution was delivered from a spout, and electric current for the ICSS was applied to the stimulating electrode. Suc, 0.3 M sucrose solution. (B) Time chart for the reward task. During this task, the conditioned sensory stimulus (tone, light, or tone + light: configural) associated with or without a reward stimulus (sucrose solution or ICSS) was presented for 2 s prior to placing the spout close to the rat’s mouth.
After recovery from surgery (10–14 days) and training (2 weeks; see below), rats were anesthetized (sodium pentobarbital, 40 mg/kg, i.p.) again and mounted with the artificial earbars. A hole (diameter, 2.8–3.0 mm) was drilled through the cranioplastic and the underlying skull (A, −1.5 to 1.5 from bregma; L, 0.2 to 3.0 right) for chronic recording. The exposed dura was excised, and the hole was covered with hydrocortisone ointment (Rinderon-VG® ointment, Shionogi Co., Ltd., Tokyo, Japan), or one or two drops of chloramphenicol (Chloromycetin® succinate, Sankyo Co., Ltd., Tokyo, Japan) solution (0.1 g/ml) were dropped into the hole. The hole was covered with a sterile Teflon sheet and sealed with epoxy glue. A second small hole (diameter, 1.5 mm) was then drilled just contralateral to the recording hole. A stainless-steel wire (diameter, 130 μm), which was insulated except at the cross section of the tip, was implanted near the lateral end of the left lateral septal nuclei through the hole to serve as an indifferent electrode. This hole was then filled with cranioplastic acrylic. After the animal recovered (5–7 days), it was placed back on the water-deprivation regimen (see Training and Task paradigms).
Training and Task Paradigms
Before surgery, the rats were acclimated by handling and became accustomed to being placed into a small, plastic restraining cage for brief periods. The threshold level for ICSS was determined after recovery from the first stage of surgery (Ono et al., 1985), and three rats for which the threshold exceeded 300 μA were excluded. The remaining rats were reacclimated to the plastic enclosure and placed on a 22-h water-deprivation regimen. While in the enclosure (1–2 h daily), they had access to a spout from which they learned to take fluid within 1–2 days, which was initially 0.3 M sucrose. Subsequently, their heads were rigidly and painlessly fixed by inserting the artificial earbars into the impressions in the cranioplastic cap. While restrained, the rats were trained to lick a spout, which was automatically extended close to their mouths for 2 s to obtain the sucrose solution or ICSS. The rats were then trained to discriminate between conditioned elemental (auditory or visual) or configural stimuli to obtain a reward. Sensory stimuli included auditory (1200, 2800, or 4300 Hz), visual (white light), and configural (simultaneous presentation of tone and light) stimuli (Table 1). A mid range speaker, located 1 m above the rat, delivered the auditory stimuli, and two white lights, 3 cm in front of each eye, delivered the visual stimuli. Licking was signaled by a photoelectric sensor triggered by the tongue. The rats were trained to lick the spout to obtain a reward (sucrose solution or ICSS; reward task; Figure 1B). A 1200-Hz tone (Tone 1), a white light in front of the right eye (Light 1), or the simultaneous presentation of a 2800-Hz tone (Tone 2) and a white light in front of the left eye (Light 2; Tone 2 + Light 2: configural stimulus) signaled availability of the 0.3-M sucrose solution. A 4300-Hz tone (Tone 3) signaled the ICSS (0.5 s train of 100 Hz, 0.3 ms capacitor-coupled negative square wave pulses). Tone 2, Light 2, or simultaneous presentation of Tone 1 and Light 1 (Tone 1 + Light 1: configural stimulus) signaled that no reward was available.
Training with or without a reward was conducted in one block of 10 or 20 trials. The total number of trials per day was 400–500 in 4 h from 16:00 to 20:00. Throughout the training and recording period, a rat was permitted to ingest 20–30 ml of sucrose solution while in the restrainer. If the rat failed to consume 30 ml of the sucrose solution while restrained, it was given the remainder when it was returned to its home cage. Only sucrose solution was available during the task.
Our previous study using the same paradigm in rats reported that the latencies of licking after offset of the CS became shorter than 300 ms after learning the association between the CS and reward (Toyomitsu et al., 2002). After the rats had learned the tasks described above (i.e., when licking latencies became shorter than 300 ms), septal neurons were recorded from during performance of these elemental and configural association tasks.
Electrophysiological Recording
An individual rat was usually tested every other day. After being placed in the enclosure, the ointment was removed, and a glass-insulated tungsten microelectrode (Z = 1.0–1.5 MΩ at 1000 Hz) was stereotaxically inserted stepwise with a pulse motor-driven manipulator (SM-20, Narishige, Tokyo, Japan) into various parts of the right septal region. Extracellular neuronal and electromyographic (EMG) activity was passed through a dual channel differential amplifier with a preamplifier (DPA-220, DIA Medical System Co., Tokyo, Japan), monitored on an oscilloscope, and recorded on a data recorder (RT-145T Dat Data Recorder, TEAC, Tokyo, Japan). Neuronal activity was quantified with a two-level voltage discriminator. The analog signal, the trigger levels, and the output of the discriminator were monitored continuously on an oscilloscope during analysis. The discriminator output pulses were accumulated and displayed as peri-stimulus histograms by an on-line minicomputer (ATAC-450, Nihon Kohden, Tokyo, Japan). Another computer (PC-98 21 Bp, NEC, Tokyo, Japan) stored the events and times of the trigger signals, output pulses from the discriminator, and lick signals for display of rasters and histograms off-line.
Data Analysis
Both neuronal and behavioral data of each trial were counted from the peri-stimulus histograms in successive 100-ms bins for three phases: a pre-trial control phase (2 s), a CS phase (2 s), and a reward (or non-reward) phase (2 s). Neuronal activities were compared among firing rates during these three phases. Neuronal excitation or inhibition was determined by the post hoc test (new multiple range test, p < 0.05) between the mean firing rate during the control phases (mean spontaneous firing rate) and that during each CS or reward phase after a one-way analysis of variance (ANOVA). The new multiple range test was selected because of its protection from Type II errors (Duncan, 1955). Each neuronal response to each CS was also compared with a one-way ANOVA and the post hoc test.
The mean firing rates among various neuronal types and recording sites were also compared by one-way ANOVA and post hoc tests (p < 0.05).
Neuronal data were also treated with multidimensional scaling (MDS) to examine the relationships among seven CSs represented by the septal neurons. MDS is a method to simplify the relationships within a complex array of data; it constructs a geometric representation of the data to show the relationship between stimuli represented by the data matrix (see Young, 1987 for more details). MDS employs the metric ratio and Euclidean model (SYSTAT statistical package, Guttman scaling method). The similarities (Pearson’s correlation coefficients) between each possible pair of CSs were calculated. The MDS program computed the Pearson’s correlation coefficients between all possible pairs of two CSs to give inter-stimulus relationships by plotting the relative positions of seven CSs in two-dimensional space. The statistical criteria, categories, and numerical analyses were identical to those used in two previous studies that used correlation coefficients for the MDS (Nishijo and Norgren, 1990, 1991).
Histology
After the last recording session, rats were anesthetized again with sodium pentobarbital (50 mg/kg, i.p.) and several small electrolytic lesions (20 μA for 20 s) were made stereotaxically around the recorded sites with a glass-insulated tungsten microelectrode. Rats were then given an overdose of anesthetic and perfused transcardially with heparinized 0.9% saline followed by 10% buffered formalin. The brain was removed, and cut into 50 μm frontal sections with a freezing microtome. Sections were stained with cresyl violet. All marking and stimulation sites were then carefully verified microscopically. Positions of neurons were stereotaxically located on the actual tissue sections and plotted on the corresponding sections of the atlas of Paxinos and Watson (1986).
The rat septal region consists of medial (MS), lateral (LS), and posterior divisions (PS) based on topography, cytoarchitecture, and connections (Jakab and Leranth, 1995). The MS included the medial septal nucleus and diagonal band of Broca. The LS consisted of dorsal, intermediate, and ventral parts. The PS included the septofimbrial nucleus and triangular septal nucleus. The classification and terminology of these septal subnuclei were based on the atlas of Paxinos and Watson (1986).
Results
Classification of the Neurons in the Septal Nuclei
Recording was performed over a period of 1–2 months for each rat. The activities of 307 neurons in and around the septal nuclei were recorded during the reward task. Of these, 284 neurons were located in septal nuclei. Table 2 summarizes the response patterns of these 284 neurons resulting from statistical analyses by one-way ANOVA and post hoc comparisons. Seventy-two neurons (25.4%) responded during one or more phases of the task: 42 with excitation and 30 with inhibition. These 72 responsive neurons were classified into two types based on response magnitudes during the CS and reward phases; CS-related and ingestion/ICSS-related. When the neurons responded during both the CS and reward phases, ingestion/ICSS-related neurons were defined as neurons with responses during the reward phase that were more than twice as large as the responses during the CS phase. There were 46 CS-related and 26 ingestion/ICSS-related neurons. The 46 conditioned stimulus-related neurons were further subclassified based on their responsiveness to each CS. Eighteen CS-related neurons responded selectively to the CSs predicting reward (CS+-related), four to the CSs predicting non-reward (CS0-related), and nine differentially to some CSs predicting reward or non-reward (miscellaneous). The remaining 15 neurons responded non-differentially to all CSs (non-differential) regardless of reward contingency.
CS+-Related Neurons
Eighteen CS+-related neurons responded selectively during presentation of the CSs predicting reward (Table 2): the activity of 12 and 6 neurons increased and decreased, respectively, during the CS phase. A typical example of this type of neuron is shown in Figure 2. Raster displays and each dot below a raster line indicate neuronal activity and one lick. Upper and lower histograms show accumulated neuronal activity and licks (Figures 2A–G). This neuron displayed excitatory responses to Tone 1 (Figure 2A), Light 1 (Figure 2B), Tone 3 (Figure 2D), and Tone 2 + Light 2 (Figure 2G), predicting sucrose solution or ICSS, but not to Tone 1 + Light 1 (Figure 2C), Tone 2 (Figure 2E), or Light 2 (Figure 2F) predicting non-reward. The mean firing rates during presentation of the CSs predicting reward (Tone 1, Light 1, Tone 2 + Light 2, or Tone 3) were significantly larger than those during the control phase (p < 0.05). The mean response magnitudes of the neuron to various CSs are indicated in Figure 2H. Statistical comparison using a one-way ANOVA indicated a significant difference in the response magnitudes among the CSs [F(6, 21) = 20.534, p < 0.01]. Post hoc comparisons indicated that the mean firing rates during presentation of the CSs predicting reward (Tone 1, Light 1, Tone 2 + Light 2, or Tone 3) were significantly larger than those during presentation of the CSs predicting non-reward (Tone 1 + Light 1, Tone 2, or Light 2; p < 0.05). Thus, the neuron responded only to the CSs associated with reward, but not to the CSs associated with non-reward.
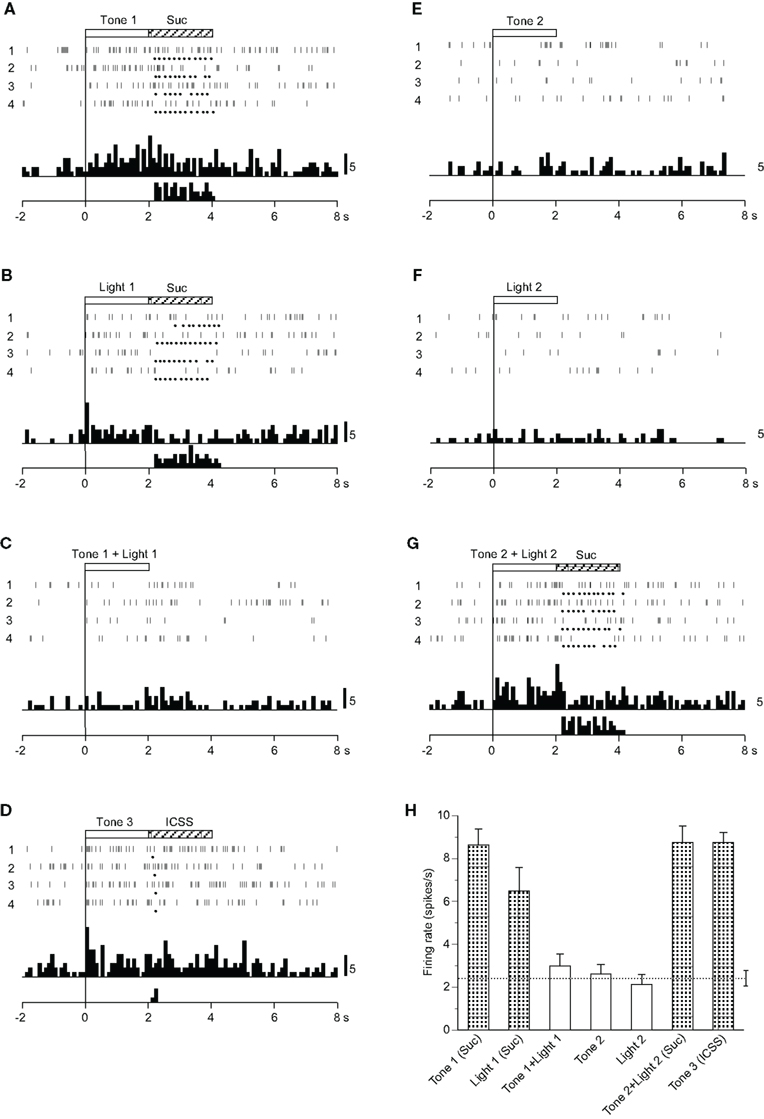
Figure 2. Activity of a conditioned stimulus predicting reward (CS)+-related neuron that responded differently to the conditioned sensory stimuli predicting reward. (A–G) Raster displays and histograms of neuronal responses to Tone 1 predicting the sucrose solution (A), Light 1 predicting the sucrose solution (B), Tone 1 + Light 1 predicting non-reward (C), Tone 3 predicting intracranial self-stimulation (ICSS) (D), Tone 2 predicting non-reward (E), Light 2 predicting non-reward (F), and Tone 2 + Light 2 predicting the sucrose solution (G). Note that neuronal activity increased during presentation of the CS+. White and hatched rectangles at the top indicate CS duration and time of reward, respectively. Each dot below the raster line indicates one lick; each upper histogram shows accumulated neuronal responses; and each lower histogram shows accumulated licks. Time scale, seconds; onset of conditioned stimulus at time 0; minus is a pre-trial control. Each histogram bin, 100 ms. Suc, 0.3 M sucrose solution. (H) Histogram of neuronal responses during presentation of each CS (mean firing rate ± SEM). A broken line with error bars indicates the mean spontaneous firing rate and the SEM during the pre-trial control phase. Shaded column, significant difference between the activity during presentation of a given CS and the spontaneous firing rate (new multiple range test after one-way ANOVA, p < 0.05).
Among the 18 CS+-related neurons, 12 showed similar responsiveness to that of the neuron shown in Figure 2. Of the remaining six neurons, one responded to the CSs including the auditory stimulus predicting reward (Tone 1, Tone 3, Tone 2 + Light 2), two to the CSs predicting the sucrose solution (Tone 1, Light 1, Tone 2 + Light 2), and three to the CS predicting ICSS (Tone 3).
CS0-Related Neurons
Four CS0-related neurons responded selectively during presentation of CSs, including the visual stimulus predicting non-reward (Table 2): the activity of three neurons increased and one decreased during the CS phase. A typical example of this type of neuron is shown in Figure 3. The activity of this neuron increased in response to Tone 1 + Light 1 (Figure 3C) and Tone 2 (Figure 3E), predicting non-reward, but not to Tone 1 (Figure 3A), Light 1 (Figure 3B), Tone 3 (Figure 3D), or Tone 2 + Light 2 (Figure 3G), predicting reward, nor to the visual stimulus (Light 2) predicting non-reward. This pattern of responsiveness indicated that the neuron responded to the CSs if the CSs predicted non-reward and included auditory stimuli. The mean firing rates during presentation of the CSs, including the auditory stimuli predicting non-reward (Tone 1 + Light 1 or Tone 2), were significantly larger than those during the control phase (p < 0.05). The mean response magnitudes of the neuron during the CS phase are presented in Figure 3H. A statistical comparison using a one-way ANOVA indicated a significant difference in the response magnitudes among the CSs [F(6, 21) = 5.770, p < 0.01]. Post hoc comparisons indicated that the mean firing rates during presentation of the CSs predicting non-reward (Tone 1 + Light 1 or Tone 2) were significantly larger than those during presentation of the CSs predicting reward (Tone 1, Light 1, or Tone 2 + Light 2, Tone 3; p < 0.05).
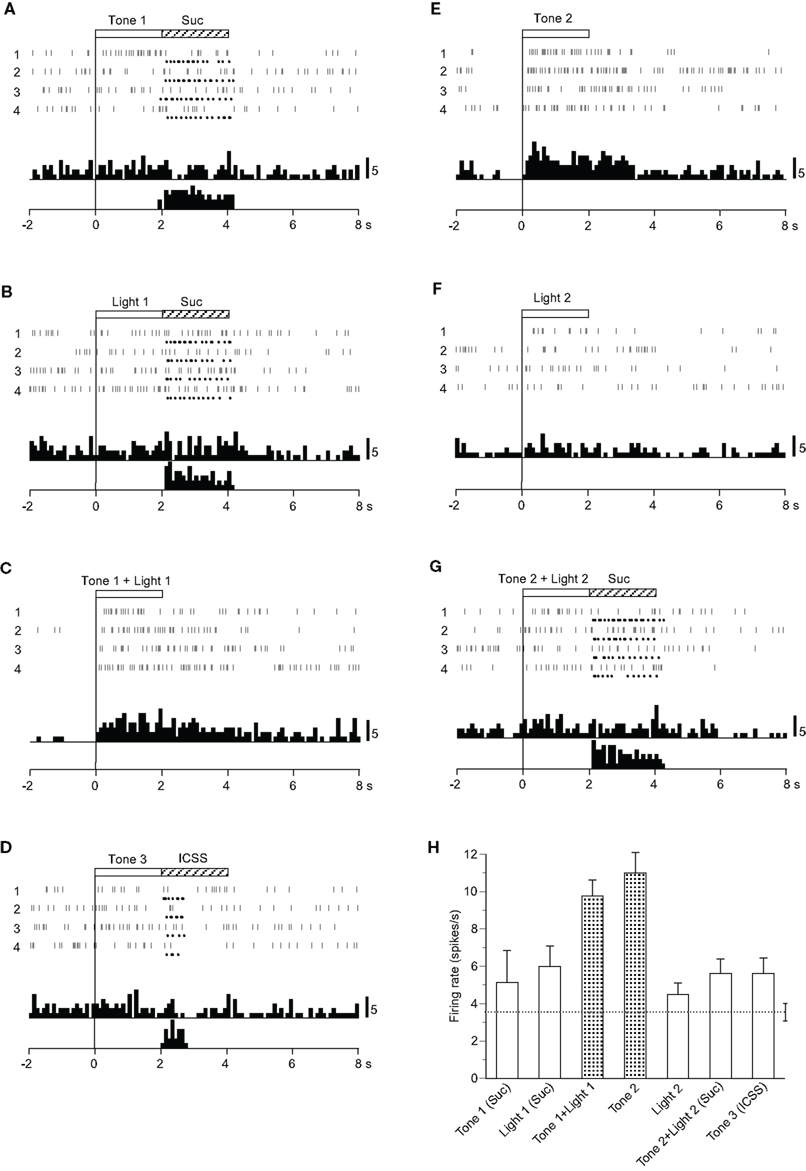
Figure 3. Activity of a conditioned stimulus predicting non-reward (CS0)-related neuron that responded differently to the conditioned sensory stimuli predicting non-reward. (A–G) Raster displays and histograms of neuronal responses to Tone 1 predicting sucrose solution (A), Light 1 predicting sucrose solution (B), Tone 1 + Light 1 predicting non-reward (C), Tone 3 predicting intracranial self-stimulation (ICSS) (D), Tone 2 predicting non-reward (E), Light 2 predicting non-reward (F), and Tone 2 + Light 2 predicting the sucrose solution (G). Note that neuronal activity increased during presentation of a CS0 that included the auditory stimulus (i.e., Tone 2 and Tone 1 + Light 1) but not during presentation of a visual CS0 (Light 2) nor the CS+ (Tone 1, Light 1, Tone 3, and Tone 2 + Light 2). (H) Histogram of neuronal responses during presentation of each CS (mean firing rate ± SEM). Other descriptions as in Figure 2.
Among four CS0-related neurons, three showed the same responsiveness as that of the neuron shown in Figure 3. The remaining neuron responded to all of the CSs predicting non-reward (Tone 2, Light 2, or Tone 1 + Light 1).
Miscellaneous Neurons
Of 31 differential CS-related neurons, nine showed different responses from those of CS+- and CS0-related neurons (Table 2). Among these nine neurons, one responded to the CSs if they included auditory stimuli regardless of reward contingency (Tone 1, Tone 2, Tone 3, Tone 1 + Light 1, or Tone 2 + Light 2), one only to the configural stimuli (Tone 1 + Light 1, Tone 2 + Light 2), and one to all CSs but it showed significantly different responses to the CSs (p < 0.05). The remaining six neurons responded to various CSs, but their responsiveness was unable to be classified.
Figure 4 shows the activity of a neuron that displayed excitatory responses to the CSs if the CS included auditory stimuli. Neuronal activity increased during presentation of Tone 1 (Figure 4A), Tone 1 + Light 1 (Figure 4C), Tone 3 (Figure 4D), Tone 2 (Figure 4E), and Tone 2 + Light 2 (Figure 4G) but not during presentation of Light 1 (Figure 4B) or Light 2 (Figure 4F). The mean firing rates during presentation of the CSs, including the auditory stimuli (Tone 1, Tone 1 + Light 1, Tone 2, Tone 2 + Light 2, or Tone 3), were significantly larger than those during the control phase (p < 0.05). The mean response magnitudes of the neuron during the CS phase are presented in Figure 4H. Statistical comparison by one-way ANOVA indicated a significant difference in the response magnitudes among the CSs [F(6, 21) = 10.007, p < 0.01].
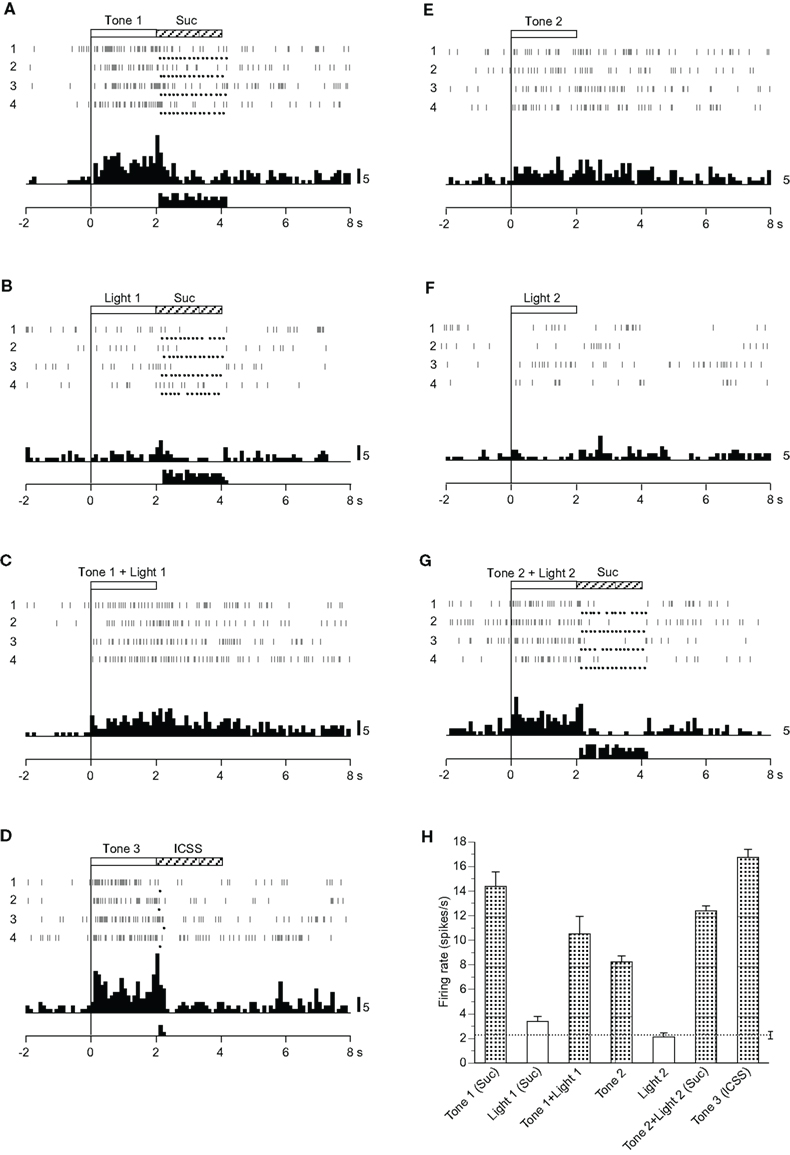
Figure 4. Activity of a miscellaneous differential neuron that responded differently to the conditioned auditory stimuli. (A–G) Raster displays and histograms of neuronal responses to Tone 1 predicting the sucrose solution (A), Light 1 predicting the sucrose solution (B), Tone 1 + Light 1 predicting non-reward (C), Tone 3 predicting intracranial self-stimulation (ICSS) (D), Tone 2 predicting non-reward (E), Light 2 predicting non-reward (F), and Tone 2 + Light 2 predicting sucrose solution (G). Note that neuronal activity increased during presentation of the CSs that included an auditory stimulus (i.e., Tone 1, Tone 1 + Light 1, Tone 3, Tone 2, and Tone 2 + Light 2) but not during presentation of a visual CS (Light 1 or Light 2). (H) Histogram of neuronal responses during presentation of each CS (mean firing rate ± SEM). Other descriptions as in Figure 2.
Non-Differential CS-Related Neurons
Fifteen neurons responded non-differentially to all CSs with or without reward (Table 2): the activity of seven and eight neurons increased and decreased, respectively. during the CS phase. A typical example of a non-differential neuron is shown in Figure 5. The activity of this neuron increased in response to all CSs regardless of reward contingency, and the responses continued after the CS phase (Figures 5A–G). The mean firing rates during the CSs were significantly larger than those during the control phase (p < 0.05). The mean response magnitudes of the neuron during the CS phase are indicated in Figure 5H. However, no significant differences among these responses during the CS phase were observed [F(6, 21) = 1.500, p > 0.05].
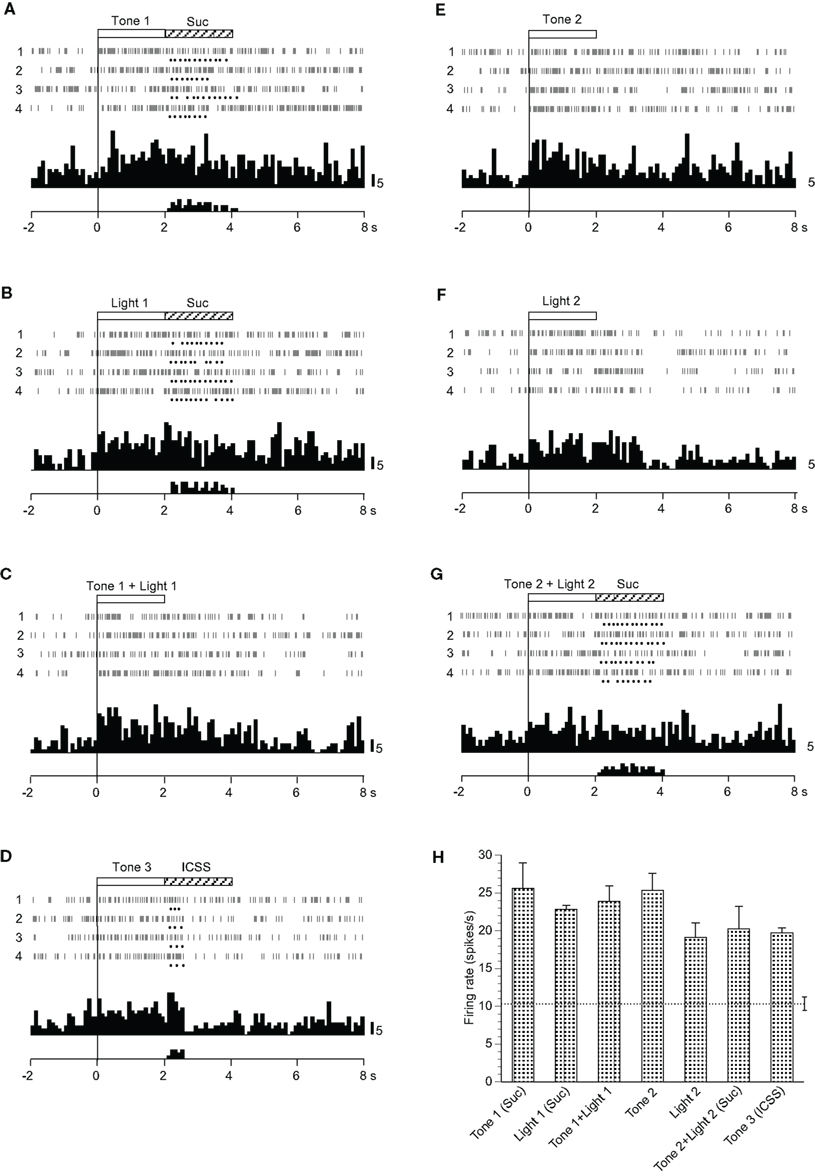
Figure 5. Activity of a non-differential neuron that responded indiscriminately to the conditioned sensory stimuli. (A–G) Raster displays and histograms of neuronal responses to Tone 1 predicting the sucrose solution (A), Light 1 predicting the sucrose solution (B), Tone 1 + Light 1 predicting non-reward (C), Tone 3 predicting intracranial self-stimulation (ICSS) (D), Tone 2 predicting non-reward (E), Light 2 predicting non-reward (F), and Tone 2 + Light 2 predicting the sucrose solution (G). Note that neuronal activity increased during presentation of all CSs. (H) Histogram of neuronal responses during presentation of each CS (mean firing rate ± SEM). Other descriptions as in Figure 2.
MDS Analysis
The responses of 31 differential CS-related neurons to each CS were analyzed by MDS using Pearson’s correlation coefficients between all possible CS pairs to elucidate the inter-stimulus relationship among the seven CSs. In the MDS analysis, if response patterns of all differential septal neurons to a given pair of CSs are similar (i.e., Pearson’s correlation coefficient between the CSs is nearly 1.0), those CSs are located close to one another in two-dimensional space. In Figure 6, the seven CSs were classified into the following three groups in a two-dimensional space based on the distance between the CSs: (i) CSs predicting the sucrose solution (Tone 1, Light 1, or Tone 2 + Light 2), (ii) CS predicting ICSS (Tone 3), and (iii) CSs predicting non-reward (Tone 2, Light 2, or Tone 1 + Light 1).
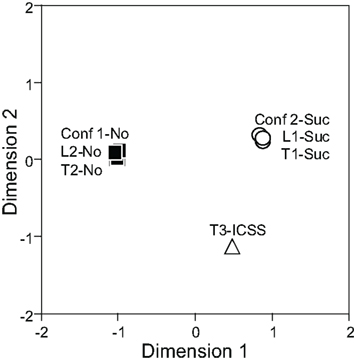
Figure 6. Two-dimensional representation of the seven conditioned stimuli (CSs) resulting from multidimensional scaling using responses of 31 differential CS-related neurons. Note that three groups of CSs are recognized; CSs predicting the sucrose solution (Tone 1, Light 1, and Tone 2 + Light 2) indicated by white circles, the CS predicting intracranial self-stimulation (ICSS; Tone 3), indicated by a white triangle, and the CSs predicting non-reward (Tone 2, Light 2, Tone 1 + Light 1) indicated by black squares. Conf 1, Tone 1 + Light 1; Conf 2, Tone 2 + Light 2; L, Light; T, Tone.
Ingestion/ICSS-Related Neurons
Twenty-six neurons responded mainly during the reward phase (Table 2). A typical example of this type of neuron is shown in Figure 7. This neuron displayed excitatory responses during ingestion of a reward (sucrose solution and ICSS; Figures 7A,B,D,G). The activity of this neuron also increased in response to all CSs, except Light 2 (Figures 7A–G). The mean firing rates during presentation of all CSs except Light 2 and those during reward phases were significantly larger than those during the control phase (p < 0.05). The mean response magnitudes of the neuron during the CS and reward phases are presented in Figure 7H. Statistical comparisons indicated that significant differences occurred in the response magnitudes among the CSs [F(6, 21) = 5.982, p < 0.01], and in the response magnitudes among the reward phases [F(3, 12) = 7.031, p < 0.01]. Furthermore, the responses to the sucrose solution were significantly larger than those to ICSS (p < 0.05).
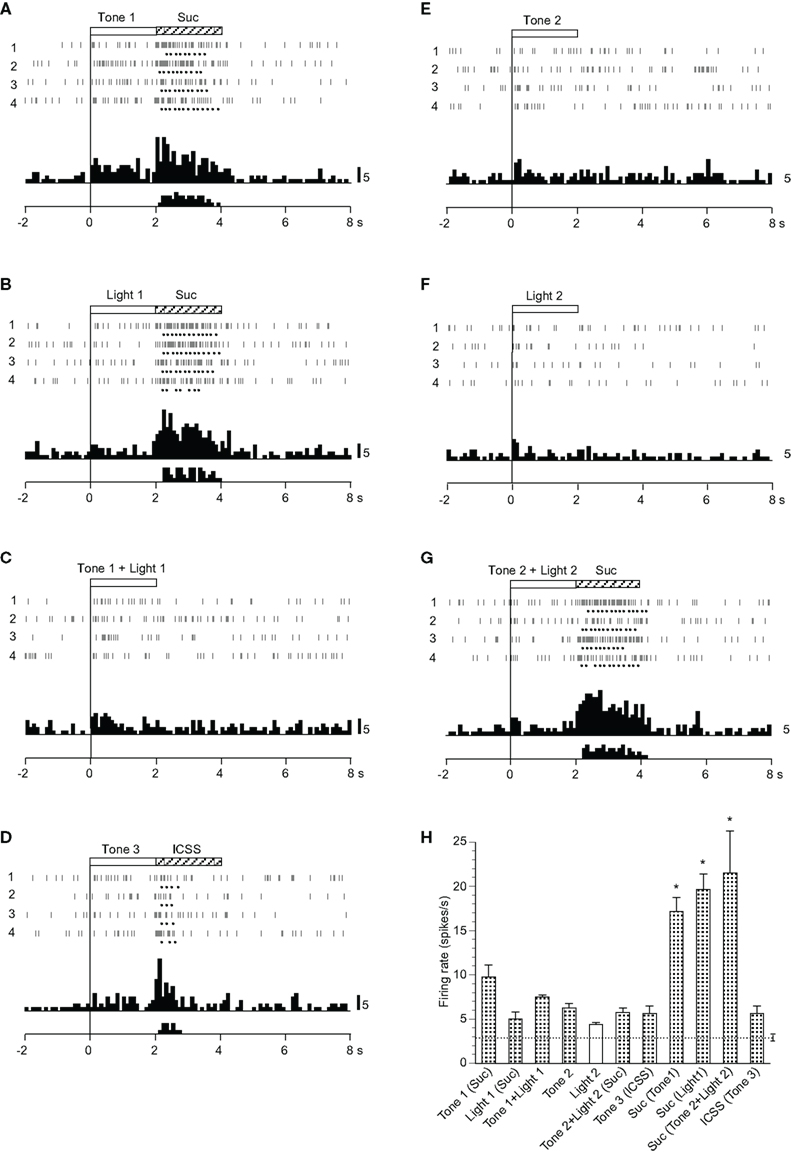
Figure 7. Activity of an ingestion/ intracranial self-stimulation (ICSS)-related neuron that responded mainly during the reward phase. (A–G) Raster displays and histograms of neuronal responses to Tone 1 predicting sucrose solution (A), Light 1 predicting sucrose solution (B), Tone 1 + Light 1 predicting non-reward (C), Tone 3 predicting ICSS (D), Tone 2 predicting non-reward (E), Light 2 predicting non-reward (F), and Tone 2 + Light 2 predicting sucrose solution (G). Note that neuronal activity increased during ingestion of a reward (i.e., sucrose solution or ICSS) and also during presentation of some CSs (Tone 1, Light 1, Tone 1 + Light 1, Tone 3, Tone 2, and Tone 2 + Light 2), but not Light 2. (H) Histogram of neuronal responses during presentation of each CS and ingestion of reward (mean firing rate ± SEM). *significant difference from other responses (new multiple range test after one-way ANOVA, p < 0.05). Other descriptions as in Figure 2.
Spontaneous Firing Rate
The spontaneous firing rates of differential CS-related neurons ranged from 1.57 to 55.94 spikes/s (8.33 ± 2.07 spikes/s, mean ± SEM, n = 31); those of non-differential CS-related neurons ranged from 1.62 to 83.61 spikes/s (15.21 ± 6.10 spikes/s, n = 15); those of ingestion/ICSS-related neurons ranged from 1.05 to 85.61 spikes/s (13.02 ± 3.43 spikes/s, n = 26); and those of non-responsive neurons ranged from 0.26 to 78.07 spikes/s (11.86 ± 0.91 spikes/s, n = 212; Figure 8A). A statistical comparison by one-way ANOVA indicated that no significant differences in mean spontaneous firing rates were observed among these four types of neurons [F(3, 280) = 0.970, p > 0.05].
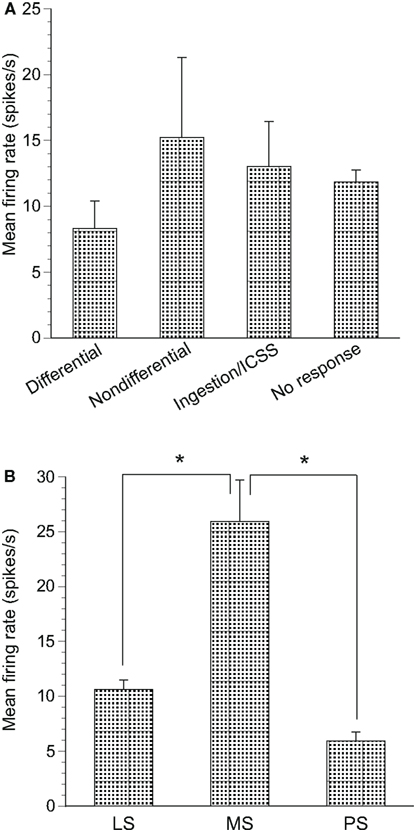
Figure 8. Mean firing rates of neurons recorded in the septal nuclei. (A) The mean spontaneous firing rates (±SEM) of differential conditioned stimulus (CS)-related, non-differential CS-related, ingestion/intracranial self-stimulation (ICSS)-related and non-responsive neurons. No significant differences in mean spontaneous firing rates were found among the three groups (one-way ANOVA, p > 0.05). (B) The mean spontaneous firing rates (±SEM) of the lateral septal nucleus (LS), medial septal nucleus (MS), and posterior septal nucleus (PS) neurons. *significant difference in mean spontaneous firing rates (new multiple range test after a one-way ANOVA, p < 0.05).
Significant differences were found for the mean spontaneous firing rates in septal neuron locations. Spontaneous firing rates of the LS, MS, and PS neurons ranged from 0.26 to 85.6 spikes/s (10.64 ± 0.84 spikes/s, n = 249), from 3.02 to 82.50 spikes/s (25.93 ± 3.75 spikes/s, n = 24), and from 2.22 to 9.49 spikes/s (5.95 ± 0.81 spikes/s, n = 11), respectively (Figure 8B). Statistical comparison by one-way ANOVA indicated a significant difference in mean spontaneous firing rates among the three regions [F(2, 281) = 14.96, p < 0.01]. The mean spontaneous firing rate of the MS neurons was significantly larger than that of the LS and PS neurons (p < 0.01).
Distributions of Septal Neuron Recording Sites
The recording sites of all septal neurons are shown in Figure 9. Of 284 septal neurons recorded, 249 were located in the LS, 24 in the MS, and 11 in the PS. The distributions of each type of responsive neurons are shown in Figure 10. Differential CS-related neurons were located mainly in the LS (n = 26; excitation/inhibition: 18/8), and a few in the MS (n = 2; 0/2) and PS (n = 3; 2/1). Non-differential CS-related neurons were located only in the LS (n = 15; 7/8). Ingestion/ICSS-related neurons were located mainly in the LS (n = 23; 14/9) and a few in the MS (n = 3; 1/2). The responsive neurons in the LS tended to be located more densely in the intermediate and dorsal part of the LS.
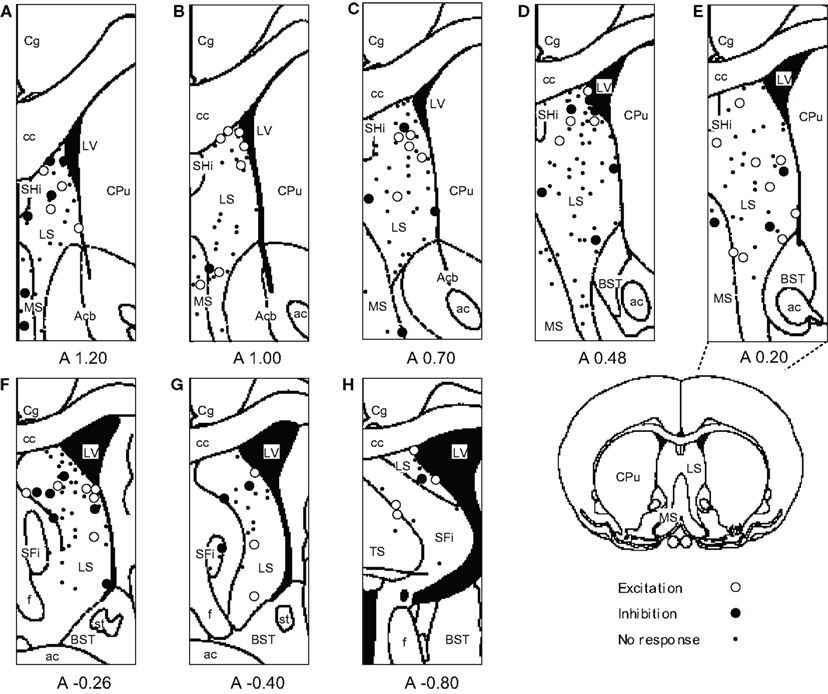
Figure 9. Distributions of all neurons recorded from septal nuclei. (A–H) Frontal sections, based on the atlas of Paxinos and Watson, are arranged rostro-caudally from left to right. Values below each section indicate distance (mm) anterior (positive value) or posterior (negative value) from the bregma. White circles, excitation; black circles, inhibition; dots, no response. ac, anterior commissure; Acb, accumbens nucleus; BST, bed nucleus of the stria terminalis; cc, corpus callosum; Cg, cingulate cortex; CPu, caudate putamen; f, fornix; LS, lateral septal nucleus; LV, lateral ventricle; MS, medial septal nucleus; SFi, septofimbrial nucleus; SHi, septohippocampal nucleus; st, stria terminalis; TS, triangular septal nucleus.
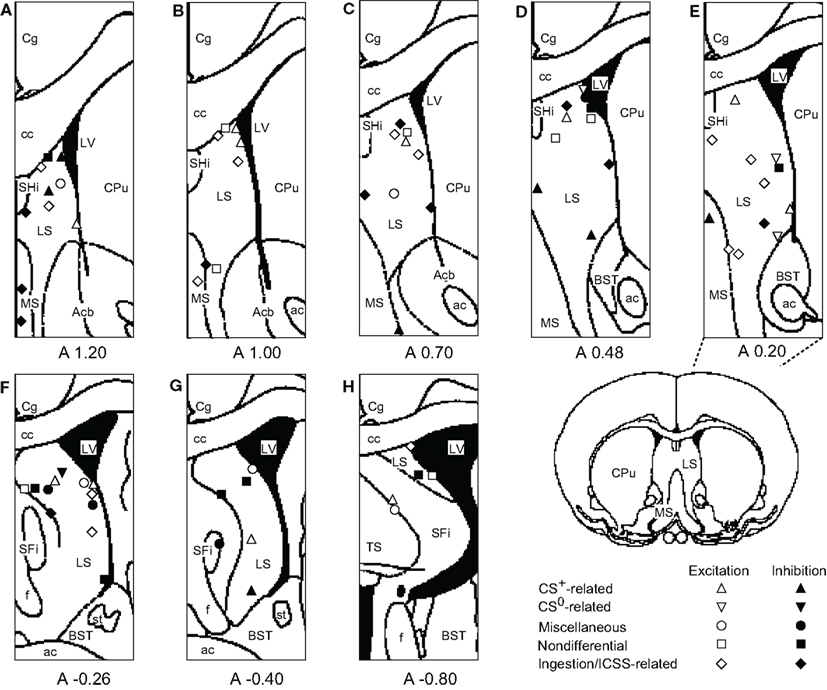
Figure 10. Distributions of responsive septal neurons. (A-H) Frontal sections, based on the atlas of Paxinos and Watson, are arranged rostro-caudally from left to right. White symbols, excitation; black symbols, inhibition; triangles, conditioned stimulus predicting reward (CS+)-related neurons; inverted triangles, conditioned stimulus predicting non-reward (CS0)-related neurons; circles, miscellaneous differential neurons; squares, non-differential neurons; diamonds, ingestion/intracranial self-stimulation (ICSS)-related neurons. Other descriptions as in Figure 9.
Discussion
Notably, most of the septal neurons were recorded from the LS, and only a few neurons were recorded from the MS and PS. Therefore, the following discussion is based largely on data from the LS.
Differential CS-Related Neurons
Previous unit recording studies in the MS and LS during classical conditioning reported that MS neurons display excitatory responses to a CS that predicts an aversive stimulus but display inhibitory responses to the CS predicting an appetitive stimulus (Yadin, 1989; Thomas et al., 1991). In contrast, LS neurons display inhibitory responses to the CS predicting an aversive stimulus and excitatory responses to the CS predicting an appetitive stimulus (Thomas et al., 1991). The present study was partially consistent with these results. Of 15 CS+-related neurons in the LS, 11 showed excitatory responses, and two CS+-related neurons in the MS showed inhibitory responses. These results are consistent with the idea that reciprocal relationships exist between LS and MS neurons. Neuroanatomical studies have reported that LS neurons receive excitatory glutamatergic afferents and send inhibitory gamma-aminobutyric acid (GABA)ergic efferents to the MS and lateral hypothalamic area (Panula et al., 1984; Risold and Swanson, 1997b), where inhibitory responses to the CS+ have been reported (Ono et al., 1986; Yadin, 1989; Thomas et al., 1991; present results). This neurophysiological evidence along with neuroanatomical results indicating direct and indirect connections between the LS and MS (Risold and Swanson, 1997b) suggests that the LS and MS work as a functional unit during conditioned associative learning. In contrast, four LS neurons showed inhibitory responses to the CS+. A neuroanatomical study reported that LS neurons send their collaterals for intrinsic connections (Staiger and Nürnberger, 1991), which could induce inhibitory responses to CS+ in the LS.
In the present study, four CS0-related neurons were recorded from the LS. Similar CS0-related neurons were previously reported only in monkey septal nuclei (Kita et al., 1995). Our previous studies indicated that no such neurons occur in the prefrontal area (Yamatani et al., 1990), cingulate cortex (Nishijo et al., 1997b; Takenouchi et al., 1999), amygdala (Nishijo et al., 1988a,b; Uwano et al., 1995), mediodorsal thalamic nucleus (Oyoshi et al., 1996), lateral hypothalamic area (Ono et al., 1986), and basal ganglia (Nishino et al., 1984, 1985a,b) in rats and monkeys. However, more recent studies in other laboratories have reported that some prefrontal cortical neurons predict the absence of reward (Kobayashi et al., 2002; Watanabe et al., 2002). Consistent with these results, the LS is positioned at an essential node point for integrating cognitive information from the various brain regions, including the prefrontal cortex, and relaying it to diencephalic and mesencephalic regions to directly control behavioral responses (Sheehan et al., 2004). Furthermore, the LS and MS have reciprocal connections with the nucleus of basalis of Meynert (substantia innominata; Lamour et al., 1984), which is involved in negative patterning similar to that (Tone1 + Light1) in the present study (Butt et al., 2002). Taken together, the existence of CS0-related neurons in the LS suggests that cognitive information from the prefrontal cortex and the nucleus of basalis of Meynert may exert inhibitory control on emotional and motivational behaviors through the LS. Previous behavioral studies have consistently reported that electrically stimulating the septal area inhibits drinking (Wishart and Mogenson, 1970; Gordon and Johnson, 1981) and bar pressing for a food reward (Altman and Wishart, 1971) under a deprived condition.
In the present study, 18 CS+-related and four CS0-related neurons responded differently to the CSs in terms of reward contingency. For example, CS+-related neurons responded to Tone 1 and Light 1 presented alone, which predicted reward, but not to these stimuli presented together, which predicted non-reward. On the contrary, CS0-related neurons responded to Tone 1 and Light 1 presented together, but not to Tone 1 and Light 1 presented alone. It should be noted that, in the configural situation, the reward contingency of the stimuli presented together was opposite to that of the elemental stimuli presented alone, although the same sensory stimuli were involved. Therefore, these patterns strongly suggest that these responses to the CSs were related to the reward contingency of the CSs rather than to the physical properties of the stimuli. The results of the MDS support this idea. In two-dimensional space, seven CSs were categorized into the following three groups: (i) CSs predicting the sucrose solution (Tone 1, Light 1, or Tone 2 + Light 2), (ii) the CS predicting ICSS (Tone 3), and (iii) CSs predicting non-reward (Tone 2, Light 2, or Tone 1 + Light 1). Furthermore, the arrangement of these three groups of conditioned stimuli suggests that dimension 1 may reflect reward availability, whereas dimension 2 may reflect reward content (i.e., difference between sucrose and ICSS). This grouping of CSs based on reward contingency regardless of physical properties of the stimuli strongly suggests that reward contingency is a main determinant of LS neuronal responsiveness. This flexible encoding of reward contingency regardless of the physical properties of the CSs in the LS is reminiscent of the functions of both orbital and medial prefrontal cortices (flexible stimulus–reward associations; Jones and Mishkin, 1972; Ferry et al., 2000; McAlonan and Brown, 2003; Baxter et al., 2007; Tait and Brown, 2007; Hsu and Packard, 2008), from which the LS receives afferent inputs (Johnson et al., 1968; Sesack et al., 1989; Buchanan et al., 1994). These finding also suggest that the LS might be a key relay station for the prefrontal cortex to exert control over behavior.
Non-Differential CS-Related Neurons
The 15 non-differential CS-related neurons in the LS responded indiscriminately to all CSs regardless of reward contingency, suggesting that activity of non-differential CS-related neurons may reflect non-specific inputs such as arousal. The LS receives strong projections from brainstem nuclei such as the laterodorsal tegmental nucleus, locus coeruleus, raphe nucleus, and VTA, which are associated with modulating arousal level (Saper, 1987; Risold and Swanson, 1997b). Furthermore, the LS shares intimate connections with the lateral hypothalamic area (Jakab and Leranth, 1995), which composes a rostral portion of the ascending reticular formation (Nieuwenhuys et al., 1982). This evidence is consistent with a previous suggestion that septal nuclei are important for modulating hippocampal θ-rhythm, which is associated with behavioral arousal (Stewart and Vanderwolf, 1987a,b; Stewart and Steven, 1990).
Ingestion/ICSS-Related Neurons
Fifteen ingestion/ICSS-related neurons responded mainly during ingestion of the reward. However, it is unclear whether the responses of these ingestion/ICSS-related neurons were related to reward ingestion or licking behavior, because EMGs were not monitored during recording of these neurons. However, activity of these neurons was not correlated to individual licks (data not shown). Furthermore, no neuronal activity changes were observed when spontaneous licking occurred during intertrial intervals. Additionally, about half of these neurons responded to both the sucrose solution and ICSS during the reward phases. These results suggest that the responses of ingestion/ICSS-related neurons are related to reward recognition. Similar ingestion-related neurons have been reported in monkey septal nuclei (Nishijo et al., 1997a). Activity of these neurons is modulated by satiation and correlated to the motivational level of the animal (Nishijo et al., 1997a) suggesting that septal nuclei play an important role in motivational aspects of ingestive behavior.
Functional Consideration of the Septal Nuclei
The LS consists of dorsal, intermediate, and ventral parts based on cytoarchitectonic parcelation (Swanson and Cowan, 1979). The LS receives major inputs from the hippocampal formation (Raisman, 1966a,b, 1969; Meibach and Siegel, 1977; Swanson and Cowan, 1977, 1979), and the intermediate part of the LS receives most of the inputs (Staiger and Nürnberger, 1989). In the present study, most of the responsive neurons were located in the dorsal and intermediate part of the LS, and fewer neurons were located in the ventral part. The LS can also be divided into three parts based on chemoarchitecture (i.e., rostral, caudal, and ventral parts; Risold and Swanson, 1996, 1997a). The areas where responsive neurons were located (i.e., the dorsal and intermediate part of the LS by Swanson and Cowan, 1979) roughly corresponded to the caudal part of the LS and dorsolateral zone of the rostral part of the LS by Risold and Swanson(1996, 1997b), which receive afferents from the CA1 and CA3 subfields of the hippocampus as well as the subiculum. These results were consistent with a previous neurophysiological study in monkeys in which responsive septal neurons were located mainly in the same areas as those in the present study (Kita et al., 1995). Afferent fibers from the entorhinal cortex also terminate in the intermediate part of the LS (Alonso and Köhler, 1984). The bed nucleus of the stria terminalis projects to the intermediate and ventral part of the LS, and the medial amygdaloid nucleus projects to the ventral part of the LS (DeVries and Buijs, 1983; VanLeeuwen and Caffé, 1983; DeVries et al., 1985; Caffé et al., 1987; Mathieson et al., 1989; Staiger and Nürnberger, 1989; DeVries, 1990). The medial and orbital prefrontal cortex also project to both the LS and MS (Johnson et al., 1968; Sesack et al., 1989; Buchanan et al., 1994). These anatomical studies suggest that different information from the other limbic system noted above converges on the septal nuclei.
The major target of output fibers from the LS is the hypothalamus (Raisman, 1966a; Meibach and Siegel, 1977; Garris, 1979; Swanson and Cowan, 1979; Berk and Finkelstein, 1981; Sawchenko and Swanson, 1983; Wouterlood et al., 1988; Ferris et al., 1990; Staiger and Wouterlood, 1990; Staiger and Nürnberger, 1991; Risold and Swanson, 1996, 1997b). Electrically stimulating the septal nuclei may modulate motivated behaviors in which the hypothalamus plays an important role (Wishart and Mogenson, 1970; Altman and Wishart, 1971; Gordon and Johnson, 1981). Furthermore, lesions in the dorsal and intermediate parts of the septal nuclei, where most responsive neurons were located in the present study, induce anxiety and abnormal emotional behaviors in rats (Menard and Treit, 1995), and the antidepressant-like effects of allopregnanolone (GABAA receptor agonist) are mediated through its effects on the dorsal or intermediate part of the LS (Rodríguez-Landa et al., 2009). These behavioral changes after septal lesions and pharmacological stimulation may be related to a disconnection between the LS and the hypothalamus, and enhance LS control on the hypothalamus, respectively. Additionally, the LS projects to the VTA to regulate dopaminergic neuron firing rates (see review by Sheehan et al., 2004). Taken together, the results suggest that the LS neurons receive a variety of information related to cognition, memory, and emotion from other limbic areas such as the hippocampal formation, entorhinal cortex, and amygdala, and integrate this information to control the diencephalon and brainstem including the hypothalamus, which play an important role in ingestive, motivated, and emotional behaviors.
Conclusion
The present study analyzed rat septal neuronal activity during discrimination of elemental and configural stimuli associated with reward or non-reward, and septal neurons were grouped into five types; (1) CS+-related neurons responding selectively to the CSs predicting reward, (2) CS0-related neurons responding to the CSs predicting non-reward, (3) neurons responding to some CSs predicting reward or non-reward, (4) septal neurons responding non-differentially to all CSs, and (5) septal neurons responding mainly during the ingestion/ICSS phase.
Multivariate analysis of the septal neuronal responses indicated that septal neurons represented the CSs based on reward contingency regardless of the physical properties of the stimuli and categorized them into three groups; three CSs predicting the sucrose solution, three CSs predicting non-reward, and one CS predicting the ICSS. These results along with those of previous anatomical studies suggest that septal nuclei integrate convergent environmental stimuli to represent reward contingency, which is essential to manifest appropriate behaviors in response to changing environmental stimuli.
Conflict of Interest Statement
The authors declare that the research was conducted in the absence of any commercial or financial relationships that could be construed as a potential conflict of interest.
Acknowledgments
This work was supported, in part, by the JSPS Asian Core Program, and a Ministry of Education, Science, Sports, and Culture Grant-in-Aid for Scientific Research (A) (22240051).
References
Alonso, A., and Köhler, C. (1984). A study of the reciprocal connections between the septum and the entorhinal area using anterograde and retrograde axonal transport methods in the rat brain. J. Comp. Neurol. 225, 327–343.
Altman, J. L., and Wishart, T. B. (1971). Motivated feeding behavior elicited by electrical stimulation of the septum. Physiol. Behav. 6, 105–109.
Averback, P. (1981). Structural lesions of the brain in young schizophrenics. Can. J. Neurol. Sci. 8, 73–76.
Baldino, F. Jr., O’Kane, T. M., Fitzpatrick-McElligott, S., and Wolfson, B. (1988). Coordinate hormonal and synaptic regulation of vasopressin messenger RNA. Science 19, 978–981.
Baxter, M. G., Gaffan, D., Kyriazis, D. A., and Mitchell, A. S. (2007). Orbital prefrontal cortex is required for object-in-place scene memory but not performance of a strategy implementation task. J. Neurosci. 27, 11327–11333.
Berk, M. L., and Finkelstein, J. A. (1981). Afferent projections to the preoptic area and hypothalamic regions in the rat brain. Neuroscience 6, 1601–1624.
Brisch, R., Bernstein, H. G., Dobrowolny, H., Krell, D., Stauch, R., Trübner, K., Steiner, J., Ghabriel, M. N., Bielau, H., Wolf, R., Winter, J., Kropf, S., Gos, T., and Bogerts, B. (2011). A morphometric analysis of the septal nuclei in schizophrenia and affective disorders: reduced neuronal density in the lateral septal nucleus in bipolar disorder. Eur. Arch. Psychiatry Clin. Neurosci. 261, 47–58.
Buchanan, S. L., Thompson, R. H., Maxwell, B. L., and Powell, D. A. (1994). Efferent connections of the medial prefrontal cortex in the rabbit. Exp. Brain Res. 100, 469–483.
Butt, A. E., Noble, M. M., Rogers, J. L., and Rea, T. E. (2002). Impairments in negative patterning but not simple discrimination learning in rats with 192 IgG-saporin lesions of the nucleus basalis magnocellularis. Behav. Neurosci. 116, 241–255.
Caffé, A. R., VanLeeuwen, F. W., and Luiten, P. G. M. (1987). Vasopressin cells in the medial amygdala of the rat project to the lateral septum and the ventral hippocampus. J. Comp. Neurol. 261, 237–252.
Covian, M. R., Antunes-Rodriguez, J., and O’Flaherty, J. J. (1964). Effect of stimulation of the septal area upon blood pressure and respiration in the cat. J. Neurophysiol. 27, 394–407.
DeVries, G. J., and Buijs, R. M. (1983). The origin of the vasopressinergic and oxytocinergic innervation of the rat brain with special reference to the lateral septum. Brain Res. 273, 307–317.
DeVries, G. J., Buijs, R. M., VanLeeuwen, F. W., Caffé, A. R., and Swaab, D. F. (1985). The vasopressinergic innervation of the brain in normal and castrated rats. J. Comp. Neurol. 233, 236–254.
Ferris, C. F., Gold, L., DeVries, G. J., and Potegal, M. (1990). Evidence for a functional and anatomical relationship between the lateral septum and the hypothalamus in the control of flank marking behavior in golden hamsters. J. Comp. Neurol. 293, 476–485.
Ferry, A. T., Lu, X. C., and Price, J. L. (2000). Effects of excitotoxic lesions in the ventral striatopallidal–thalamocortical pathway on odor reversal learning: inability to extinguish an incorrect response. Exp. Brain Res. 131, 320–335.
Gaffan, E. A., Gaffan, D., and Hodges, J. R. (1991). Amnesia following damage to the left fornix and other sites. Brain 114, 1297–1313.
Gordon, F. J., and Johnson, A. K. (1981). Electrical stimulation of the septal area in the rat: prolonged suppression of water intake and correlation with self-stimulation. Brain Res. 206, 421–430.
Gray, J. A. (1987). The Neuropsychology of Anxiety: An Enquiry into the Functions of the Septo-Hippocampal System. New York: Oxford University Press.
Harvey, J. A., and Hunt, H. F. (1965). Effect of septal lesions on thirst in the rat as indicated by water consumption and operant responding for water reward. J. Comp. Physiol. Psychol. 59, 49–56.
Holdstock, T. L. (1967). Effects of septal stimulation in rats on heart rate, and galvanic skin response. Psychon. Sci. 9, 37–38.
Hsu, E., and Packard, M. G. (2008). Medial prefrontal cortex infusions of bupivacaine or AP-5 block extinction of amphetamine conditioned place preference. Neurobiol. Learn. Mem. 89, 504–512.
Jakab, R. L., and Leranth, C. (1995). “Septum,” in The Rat Nervous System, 2nd Edn, ed. G. Paxinos (San Diego: Academic Press), 405–442.
Johnson, T. N., Rosvold, H. E., and Mishkin, M. (1968). Projections from behaviorally-defined sectors of the prefrontal cortex to the basal ganglia, septum, and diencephalon of the monkey. Exp. Neurol. 21, 20–34.
Jones, B., and Mishkin, M. (1972). Limbic lesions and the problem of stimulus-reinforcement associations. Exp. Neurol. 36, 362–377.
Kita, T., Nishijo, H., Eifuku, S., Terasawa, K., and Ono, T. (1995). Place and contingency differential responses of monkey septal neurons during conditional place-object discrimination. J. Neurosci. 15, 1683–1703.
Kobayashi, S., Lauwereyns, J., Koizumi, M., Sakagami, M., and Hikosaka, O. (2002). Influence of reward expectation on visuospatial processing in macaque lateral prefrontal cortex. J. Neurophysiol. 87, 1488–1498.
Lamour, Y., Dutar, P., and Jobert, A. (1984). Cortical projections of the nucleus of the diagonal band of Broca and of the substantia innominata in the rat: an anatomical study using the anterograde transport of a conjugate of wheat germ agglutinin and horseradish peroxidase. Neuroscience 12, 395–408.
Lorens, S. A., and Kondo, C. Y. (1969). Effects of septal lesions on food and water intake and operant responding for food. Physiol. Behav. 4, 729–732.
Malmo, R. B. (1961). Slowing of heart rate following septal self-stimulation in rats. Science 133, 1128–1130.
Malmo, R. B. (1965). Classical and instrumental conditioning with septal stimulation as reinforcement. J. Comp. Physiol. Psychol. 60, 1–8.
Mathieson, W. B., Federico, P., Veale, W. L., and Pittman, Q. J. (1989). Single-unit activity in the bed nucleus of the stria terminalis during fever. Brain Res. 486, 49–55.
McAlonan, K., and Brown, V. J. (2003). Orbital prefrontal cortex mediates reversal learning and not attentional set shifting in the rat. Behav. Brain Res. 146, 97–103.
Meibach, R. C., and Siegel, A. (1977). Efferent connections of the hippocampal formation in the rat. Brain Res. 124, 197–224.
Menard, J., and Treit, D. (1995). Dissociations between the anxiolytic effects of septal and hippocampal lesions. Soc. Neurosci. Abstr. 21, 447.
Merrer, J. L., Gavello-Baudy, S., Galey, D., and Cazala, P. (2007). Morphine self-administration into the lateral septum depends on dopaminergic mechanisms: evidence from pharmacology and Fos neuroimaging. Behav. Brain Res. 180, 203–217.
Nieuwenhuys, R., Geeraedts, L. M. G., and Veening, J. G. (1982). The medial forebrain bundle of the rat. I. General introduction. J. Comp. Neurol. 206, 49–81.
Nishijo, H., Kita, T., Tamura, R., Eifuku, S., Terasawa, K., and Ono, T. (1997a). Motivation-related neuronal activity in the object discrimination task in monkey septal nuclei. Hippocampus 7, 536–548.
Nishijo, H., Yamamoto, Y., Ono, T., Uwano, T., Yamashita, J., and Yamashima, T. (1997b). Single neuron responses in the monkey anterior cingulate cortex during visual discrimination. Neurosci. Lett. 227, 79–82.
Nishijo, H., and Norgren, R. (1990). Responses from parabrachial gustatory neurons in behaving rats. J. Neurophysiol. 63, 707–724.
Nishijo, H., and Norgren, R. (1991). Parabrachial gustatory neural activity during licking by rats. J. Neurophysiol. 66, 974–985.
Nishijo, H., and Norgren, R. (1997). Parabrachial neural coding of taste stimuli in awake rats. J. Neurophysiol. 78, 2254–2268.
Nishijo, H., Ono, T., and Nishino, H. (1988a). Topographic distribution of modality-specific amygdalar neurons in alert monkey. J. Neurosci. 8, 3556–3569.
Nishijo, H., Ono, T., and Nishino, H. (1988b). Single neuron responses in amygdala of alert monkey during complex sensory stimulation with affective significance. J. Neurosci. 8, 3570–3583.
Nishijo, H., Uwano, T., Tamura, R., and Ono, T. (1998). Gustatory and multimodal neuronal responses in the amygdala during licking and discrimination of sensory stimuli in awake rats. J. Neurophysiol. 79, 21–36.
Nishino, H., Ono, T., Fukuda, M., and Sasaski, K. (1985a). Monkey substantia nigra (pars reticulata) neuron discharges during operant feeding. Brain Res. 334, 190–193.
Nishino, H., Ono, T., Muramoto, K., Fukuda, M., and Sasaki, K. (1985b). Movement and non-movement related pallidal unit activity during bar press feeding behavior in the monkey. Behav. Brain Res. 15, 27–42.
Nishino, H., Ono, T., Sasaki, K., Fukuda, M., and Muramoto, K. (1984). Caudate unit activity during operant feeding behavior in monkeys and modulation by cooling prefrontal cortex. Behav. Brain Res. 11, 21–33.
Numan, R., and Quaranta, J. R. Jr. (1990). Effects of medial septal lesions on operant delayed alternation in rats. Brain Res. 531, 232–241.
Olds, J., and Milner, P. (1954). Positive reinforcement produced by electrical stimulation of septal area and other regions of rat brain. J. Comp. Physiol. Psychol. 47, 419–427.
Ono, T., Nakamura, K., Nishijo, H., and Fukuda, M. (1986). Hypothalamic neuron involvement in integration of reward, aversion, and cue signals. J. Neurophysiol. 56, 63–79.
Ono, T., Sasaki, K., Nakamura, K., and Norgren, R. (1985). Integrated lateral hypothalamic neural responses to natural and artificial rewards and cue signals in the rat. Brain Res. 327, 303–306.
Oyoshi, T., Nishijo, H., Asakura, T., and Ono, T. (1996). Emotional and behavioral correlates of the mediodorsal thalamic neurons during associative learning in rats. J. Neurosci. 16, 5812–5829.
Panula, P., Revuelta, A. V., Cheney, D. L., Wu, J. Y., and Costa, E. (1984). An immunohistochemical study on the location of GABAergic neurons in rat septum. J. Comp. Neurol. 222, 69–80.
Paxinos, G., and Watson, C. (1986). The Rat Brain in Stereotaxic Coordinates, 2nd Edn. San Diego: Academic Press.
Raisman, G. (1966a). An experimental analysis of the efferent projection of the hippocampus. Brain 89, 83–108.
Raisman, G. (1969). A comparison of the mode of termination of the hippocampal and hypothalamic afferents to the septal nuclei as revealed by electron microscopy of degeneration. Exp. Brain Res. 7, 317–343.
Risold, P. Y., and Swanson, L. W. (1996). Structural evidence for functional domains in the rat hippocampus. Science 272, 1484–1486.
Risold, P. Y., and Swanson, L. W. (1997a). Chemoarchitecture of the rat lateral septal nucleus. Brain Res. Rev. 24, 91–113.
Risold, P. Y., and Swanson, L. W. (1997b). Connections of the rat lateral septal complex. Brain Res. Rev. 24, 115–195.
Rodríguez-Landa, J. F., Contreras, C. M., and García-Ríos, R. I. (2009). Allopregnanolone microinjected into the lateral septum or dorsal hippocampus reduces immobility in the forced swim test: participation of the GABAA receptor. Behav. Pharmacol. 20, 614–622.
Saper, C. B. (1987). “Diffuse cortical projection systems: anatomical organization and role in cortical function,” in Handbook of Physiology. Section 1: The Nervous System. Vol. 5, Higher Functions of the Brain, Part 1, eds V. B. Mountcastle, F. Plum, and S. R. Geiger (Bethesda, MD: American Physiological Society), 169–210.
Sawchenko, P. E., and Swanson, L. W. (1983). The organization of forebrain afferents to the paraventricular and supraoptic nuclei of the rat. J. Comp. Neurol. 218, 121–144.
Sesack, S. R., Deutch, A. Y., Roth, R. H., and Bunney, B. S. (1989). Topographical organization of the efferent projections of the medial prefrontal cortex in the rat: an anterograde tract-tracing study with Phaseolus vulgaris leucoagglutinin. J. Comp. Neurol. 290, 213–242.
Sheehan, T. P., Chambers, R. A., and Russell, D. S. (2004). Regulation of affect by the lateral septum: implications for neuropsychiatry. Brain Res. Rev. 46, 71–117.
Staiger, J. F., and Nürnberger, F. (1989). Pattern of afferents to the lateral septum in the guinea pig. Cell Tissue Res. 257, 471–490.
Staiger, J. F., and Nürnberger, F. (1991). The efferent connections of the lateral septal nucleus in the guinea pig: intrinsic connectivity of the septum and projections to other telencephalic areas. Cell Tissue Res. 264, 415–426.
Staiger, J. F., and Wouterlood, F. G. (1990). Efferent projections from the lateral septal nucleus to the anterior hypothalamus in the rat: a study combining Phaseolus vulgaris-leucoagglutinin tracing with vasopressin immunocytochemistry. Cell Tissue Res. 261, 17–23.
Stewart, M., and Steven, E. F. (1990). Do septal neurons pace the hippocampal Q-rhythm? Trends Neurosci. 13, 163–168.
Stewart, M., and Vanderwolf, C. H. (1987a). Hippocampal rhythmical slow activity following ibotenic acid lesions of the septal region. I. Relation to behavior and effect of atropine and urethane. Brain Res. 423, 88–100.
Stewart, M., and Vanderwolf, C. H. (1987b). Hippocampal rhythmical slow activity following ibotenic acid lesions of the septal region. II. Changes in hippocampal activity during sleep. Brain Res. 423, 101–108.
Stoller, W. L. (1972). Effects of septal and amygdaloid lesions on discrimination, eating and drinking. Physiol. Behav. 8, 823–828.
Swanson, L. W., and Cowan, W. M. (1977). An autoradiographic study of the organization of the efferent connections of the hippocampal formation in the rat. J. Comp. Neurol. 172, 49–84.
Swanson, L. W., and Cowan, W. M. (1979). The connections of the septal region in the rat. J. Comp. Neurol. 186, 621–656.
Swanson, L. W., Köhler, C., and Björklund, A. (1987). “The limbic region. I: the septohippocampal system,” in Handbook of Chemical Neuroanatomy. Vol. 5, Integrated Systems of the CNS, Part I, eds A. Björklund, T. Hökfelt, and L. W. Swanson (Amsterdam: Elsevier Science Publishers B.V.), 125–277.
Tait, D. S., and Brown, V. J. (2007). Difficulty overcoming learned non-reward during reversal learning in rats with ibotenic acid lesions of orbital prefrontal cortex. Ann. N. Y. Acad. Sci. 1121, 407–420.
Takenouchi, K., Nishijo, H., Uwano, T., Tamura, R., Takigawa, M., and Ono, T. (1999). Emotional and behavioral correlates of the anterior cingulate cortex during associative learning in rats. Neuroscience 93, 1271–1287.
Thomas, E., Yadin, E., and Strickland, C. E. (1991). Septal unit activity during classical conditioning: a regional comparison. Brain Res. 547, 303–308.
Thomas, G. J., and Gash, D. M. (1986). Differential effects of posterior septal lesions on dispositional and representational memory. Behav. Neurosci. 100, 712–719.
Toyomitsu, Y., Nishijo, H., Uwano, T., Kuratsu, J., and Ono, T. (2002). Neuronal responses of the rat amygdala during extinction and reassociation learning in the elementary and configural associative tasks. Eur. J. Neurosci. 15, 753–768.
Uwano, T., Nishijo, H., Ono, T., and Tamura, R. (1995). Neuronal responsiveness to various sensory stimuli, and associative learning in the rat amygdala. Neuroscience 68, 339–361.
VanLeeuwen, F., and Caffé, R. (1983). Vasopressin-immunoreactive cell bodies in the bed nucleus of the stria terminalis of the rat. Cell Tissue Res. 228, 525–534.
Watanabe, M., Hikosaka, K., Sakagami, M., and Shirakawa, S. (2002). Coding and monitoring of motivational context in the primate prefrontal cortex. J. Neurosci. 22, 2391–2400.
Wishart, T. B., and Mogenson, G. J. (1970). Reduction of water intake by electrical stimulation of the septal region of the rat brain. Physiol. Behav. 5, 1399–1404.
Wouterlood, F. G., Gaykema, R. P. A., Steinbusch, H. W. M., Watanabe, T., and Wada, H. (1988). The connections between the septum-diagonal band complex and histaminergic neurons in the posterior hypothalamus of the rat. Anterograde tracing with Phaseolus vulgaris-leucoagglutinin combined with immunocytochemistry of histidine decarboxylase. Neuroscience 26, 827–845.
Yadin, E. (1989). Unit activity in the medial septum during differential appetitive conditioning. Behav. Brain Res. 33, 45–50.
Yamatani, K., Ono, T., Nishijo, H., and Takaku, A. (1990). Activity and distribution of learning-related neurons in monkey (Macaca fuscata) prefrontal cortex. Behav. Neurosci. 104, 503–531.
Young, F. W. (1987). Multidimensional Scaling. History, Theory, and Applications. Hillsdale, NJ: Lawrence Erlbaum.
Keywords: conditioning, intracranial self-stimulation, motivation, non-reward, reward
Citation: Matsuyama N, Uwano T, Hori E, Ono T and Nishijo H (2011) Reward contingency modulates neuronal activity in rat septal nuclei during elemental and configural association tasks. Front. Behav. Neurosci. 5:26. doi: 10.3389/fnbeh.2011.00026
Received: 07 January 2011;
Paper pending published: 03 March 2011;
Accepted: 05 May 2011;
Published online: 19 May 2011.
Edited by:
Carlos Tomaz, University of Brasília, BrazilReviewed by:
Christa Mcintyre, University of Texas, USAGuillaume Poirier, Ecole Polytechnique Fédérale de Lausanne, Switzerland
Copyright: © 2011 Matsuyama, Uwano, Hori, Ono and Nishijo. This is an open-access article subject to a non-exclusive license between the authors and Frontiers Media SA, which permits use, distribution and reproduction in other forums, provided the original authors and source are credited and other Frontiers conditions are complied with.
*Correspondence: Hisao Nishijo, System Emotional Science, Graduate School of Medicine and Pharmaceutical Sciences, University of Toyama, Sugitani 2630, Toyama 930-0194, Japan. e-mail:bmlzaGlqb0BtZWQudS10b3lhbWEuYWMuanA=