- Aquitaine Institute of Cognitive and Integrative Neuroscience, Université de Bordeaux, CNRS UMR 5287, Bordeaux, France
Deficits in decision-making is a hallmark of several neuropsychiatric pathologies but is also observed in some healthy individuals that could be at risk to develop these pathologies. Poor decision-making can be revealed experimentally in humans using the Iowa gambling task, through the inability to select options that ensure long term gains over larger immediate gratification. We devised an analogous task in the rat, based on uncertainty and conflicting choices, the rat gambling task (RGT). It similarly reveals good and poor performers within a single session. Using this task, we investigated the role of three prefrontal cortical areas, the orbitofrontal, prelimbic, and cingulate cortices on decision-making, taking into account inter-individual variability in behavioral performances. Here, we show that these three distinct subregions are differentially engaged to solve the RGT. Cingulate cortex lesion mainly delayed good decision-making whereas prelimbic and orbitofrontal cortices induced different patterns of inadapted behaviors in the task, indicating varying degree of functional specialization of these three areas. Their contribution largely depended on the level of adaptability demonstrated by each individual to the constraint of the task. The inter-individual differences in the effect of prefrontal cortex area lesions on decision-making revealed in this study open new perspectives in the search for vulnerability markers to develop disorders related to executive dysfunctioning.
Introduction
Making a decision in complex and uncertain situations is a fundamental adaptive process resulting from the integration of several executive functions. These functions encompass a set of cognitive skills responsible for the planning, initiation, and monitoring of goal-directed behavior (Fellows, 2004; Ernst and Paulus, 2005; Rangel, 2008). Decision-making in humans can be accurately modeled in the laboratory using the Iowa gambling task (IGT; Bechara et al., 1994, 1997). This card-based task simulates real-life decision-making since the subjects deduce the best of several options trial by trial to maximize monetary gains. The contingencies are arranged so that some decks are associated with a higher immediate gain but are disadvantageous in the long run due to higher unpredictable penalties, in comparison with advantageous options associated with smaller immediate gain, but also smaller penalties. Most healthy individuals end up by making the best decision within a single session. In contrast, poor decision-making behavior characterizes patients suffering from psychiatric disorders such as attention deficit/hyperactivity disorder, or drug addiction (Dunn et al., 2006). Interestingly, poor decision-making is also observed in a significant proportion of healthy subjects that cannot refrain from choosing disadvantageous options (Bechara and Damasio, 2002; Bechara et al., 2002).
Significant progress has been made in relating poor decision-making in lesioned or psychiatric patients to specific prefrontal cortical areas, notably the orbitofrontal (OF) cortex, the dorsolateral prefrontal cortex (DL), and the anterior part of the cingulate cortex (AC; Bechara et al., 2000; Manes et al., 2002; Rushworth et al., 2007; Lawrence et al., 2009). The relative contribution of each of these brain areas in decision-making still remains poorly understood, due to several limitations. These limitations include the anatomical specificity of neuroimaging techniques, the localization of the lesions and also the symptom variability across psychiatric patients. Moreover, the inter-individual variability inherent to the control subjects is also a very important issue that has been poorly addressed in previous research (see Buelow and Suhr, 2009). Yet, recent works have shown that this behavioral variability can be reliably related to specific and differential patterns of frontal activations (Cazalis et al., 2003; Carlson et al., 2009; George and Koob, 2010; Mcguire and Botvinick, 2010).
In an attempt to resolve these issues, several tasks assessing decision-making in the rat were developed (Van Den Bos et al., 2006; Pais-Vieira et al., 2007; Zeeb et al., 2009). We recently devised a rat version of the IGT, the rat gambling task (RGT; Rivalan et al., 2009), based on the same principle. This task tracks, as it does for humans, the continuous and dynamic process of deduction and readjustment of choice in a complex and ambiguous situation, within a single session. A majority of good decision-makers can solve the RGT, following a rapid, experience-based evaluation and subsequent deduction of the best options. In contrast, some poor decision-makers prefer larger immediate rewards despite suffering large losses, whereas a minority of rats has no preference. The parallel between behaviors in humans and rodents suggests the recruitment of similar cognitive processes and resources to solve these tasks. As such, the RGT is particularly appropriate for an easy exploration of the neurobiological bases that account for good and poor decision-making. Therefore, we investigated the effects of specific prefrontal cortical area lesions in the anterior cingulate, OF, and prelimbic (PL) cortices, on the time-course of decision-making in the RGT, with a special focus on inter-individual differences in behavioral responding.
Materials and Methods
Apparatus
Twelve identical operant chambers (Imetronic, Pessac, France; adapted from five-choice serial reaction time chambers; Blondeau and Dellu-Hagedorn, 2007) were used for the RGT. Four circular holes were available on a curved wall, two on the left and two on the right side, and could be dimly illuminated with a white led located at their rear. A food magazine on the opposite wall was connected to an external dispenser delivering food pellets (45 mg, formula P, Bioserv, USA). A clear vertical Plexiglas partition (28 cm × 0.5 cm ×30 cm) with a central opening (7 cm × 7 cm) was placed across the middle of the chamber, parallel to the food wall. This partition allowed an equal distance to each nose-poke hole and avoids thigmotaxic behavior.
Subjects
Sixty-six male Wistar Han rats (Charles River, Lyon, France), weighing 200–250 g when received, were used in two identical replications of the experiment. The rats were housed in groups of four in a temperature-controlled room (23°C) on an inverted 12 h light/dark cycle (light on at 8:00 p.m.). Behavioral measures always began at least 1 h after the beginning of the dark phase of the cycle. Before behavioral training, rats were handled for a few minutes every day. During training and testing phases, rats were moderately food-deprived but had free access to water. Food rationing was adjusted to maintain their weight to approximately 95% of free-feeding weight. All experiments were performed in accordance with the European Communities Council Directive of November 24, 1986 (86/609/EEC).
Surgery
Rats were anesthetized using ketamine (i.p, 100–90 mg/kg) after analgesia with xylazine (i.p, 12–10 mg/kg), and then placed in a Kopf stereotaxic frame (Kopf Instrument, Tujunga, CA, USA) in a flat skull position. Local anesthetic (xylocaïne) was sprayed on the skin of the head before incision. The bone of the skull above the region to be lesioned was removed using a high-speed drill. N-methyl-D-aspartate (NMDA, Sigma-Aldrich) was dissolved in phosphate buffer saline (0.2 M) to provide a solution with a concentration of 20 mg/ml. This solution was injected into the brain through a glass pipette glued onto the end of the needle of a 5-μl Hamilton syringe held with a microinjector – micromanipulator (Imetronic, Pessac, France). Injections were made manually at a rate of 0.1 μl/30 s and the pipette was left in place for 3 min after the injection to allow diffusion of the solution into the tissue. PL cortex lesions were made with three bilateral infusions of NMDA at the following anterior–posterior (AP), mediolateral (ML), and dorsoventral (DV) coordinates: AP + 3.8, ML ± 0.6, DV − 3.8 (0.1 μl); AP + 3.2, ML ± 0.6, DV − 3.6 (0.1 μl); AP + 2.5, ML ± 0.6, DV − 3.4 (0.1 μl) (adapted from Killcross and Coutureau, 2003). Anterior–posterior and mediolateral coordinates were measured (in millimeters) relative to bregma, whereas dorsoventral coordinates were measured from brain surface. OF cortex lesions were made with three bilateral infusions of NMDA: AP + 4.2, ML ± 0.9, DV − 4.4 (0.1 μl); AP + 3.7, ML ± 2, DV − 4.5 (0.1 μl); AP + 3.2, ML ± 2.6, DV − 5.2 (0.1 μl) (adapted from Rudebeck et al., 2006). Anterior cingulate cortex (AC) lesions were made with four bilateral infusions of NMDA: AP + 2.3, ML ± 0.5, DV − 1.9 (0.1 μl); AP + 1.6, ML ± 0.5, DV − 2.4 (0.2 μl); AP + 0.9, ML ± 0.5, DV − 2.4 (0.2 μl); AP + 0.2, ML ± 0.5, DV − 2.2 (0.1 μl) (adapted from Rudebeck et al., 2006). Rats in the Sham group were given a similar surgical procedure, but the dura was simply breached with a needle tip, and no injection was given. All subjects recovered for a minimum period of 14 days after surgery with ad libitum access to food and water. Body weights were controlled daily.
Histology
After behavioral testing, animals received a lethal dose of sodium pentobarbital and were perfused transcardially with saline (0.9%) and then by 10% formaldehyde solution. The brains were then removed and post-fixed in 10% of formaldehyde solution at −4°C. After post-fixation, the brains were transferred in a 0.1-M phosphate-buffered 20% sucrose solution and remained at −4°C for 24 h. Coronal sections (50 μm thick) were cut using a freezing microtome (−20°C). The sections were collected onto gelatin-coated slides and dried at room temperature for 36 h before being stained with thionin. Histological analysis was performed by experimenters blind to the lesion condition. The extent of lesion was determined by microscopically examining slides for gross morphological changes, gliosis, and scarring. Reconstructions were drawn from sections with reference to the atlas of Paxinos and Watson (1997).
Behavioral Procedure
The training and test procedures were identical to that previously described (Rivalan et al., 2009). The effects of the lesions in the RGT were tested in naive animals, since previous experience in the task changes the behavior: rats remember the rules of the task and readily choose the options that they preferred (Rivalan et al., 2009). Rats were nevertheless accustomized to the experimental set up prior surgery.
Training
Prior to surgery, rats were initially trained to associate two consecutive nose-pokes in the same hole in order to obtain a reward, for 5 days. Rats could freely choose between the four holes during daily sessions (all rats sample the four holes), until they obtained 100 pellets within one session. When a nose-poke was made, only this hole remained illuminated until the pellet was collected. Afterward, the next trial immediately began with all the holes lighted-on. After recovery from surgery, rats were re-trained for two more days, to control the effects of the lesions on animal behavior in the task. The general ability to perform the task (activity and motivation levels) was controlled by the number of nose-pokes per minute, the mean duration of a session before reaching the criterion, as well as hole sampling (number of nose-pokes per option).
Rat gambling task
The test consisted in a single 1 h session (or cut-off when 250 pellets were obtained). Rats are faced to the four choice outcomes of the task during the 1-h unique test session, for the first time. In the RGT, rats had free access to the four nose-poke holes (A–D) but each choice was associated with different outcomes (Figure 1). Choice C or D allowed immediate delivery of one pellet, whereas choice A or B delivered two pellets simultaneously. Choices A and B were disadvantageous since they could be followed by unpredictable longer penalties consisting of time-outs during which no reward can be obtained (time-out of 222 and 444 s respectively) compared to advantageous choices (C and D, 12 and 6 s). Penalties occurred according to a low probability (¼) for B and C and to a high probability (¼) for A and D. During the penalty, the chosen hole remained illuminated to facilitate association between each choice and its consequences. A brief extinction of this light (1 s) signaled the end of the time-out. The four hole lights were then illuminated again to allow the rat to make a new choice. Trials not associated with a penalty had no time-out. The theoretical maximum gain was the same for choices (C and D) and was five times higher than for both choices (A and B; lower probabilities of a penalty are compensated by a longer duration of the penalty).
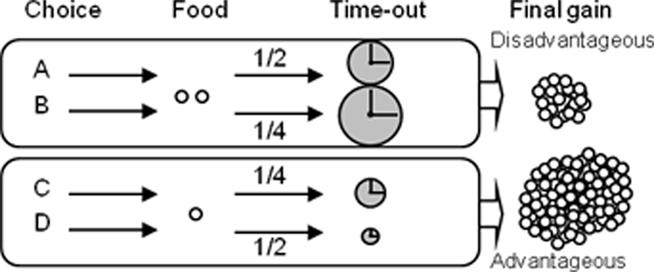
Figure 1. Principle of the rat gambling task. Rats can nose-poke among four different holes (A–D) to obtain food reward during a 1-h session. The selection of one option is immediately rewarded (food pellet) but can also be followed by a penalty (time-out) of variable intensity. Two options (C,D) are equally more advantageous than the two others (A,B), which are equally disadvantageous in the long term (see Rivalan et al., 2009 for more details).
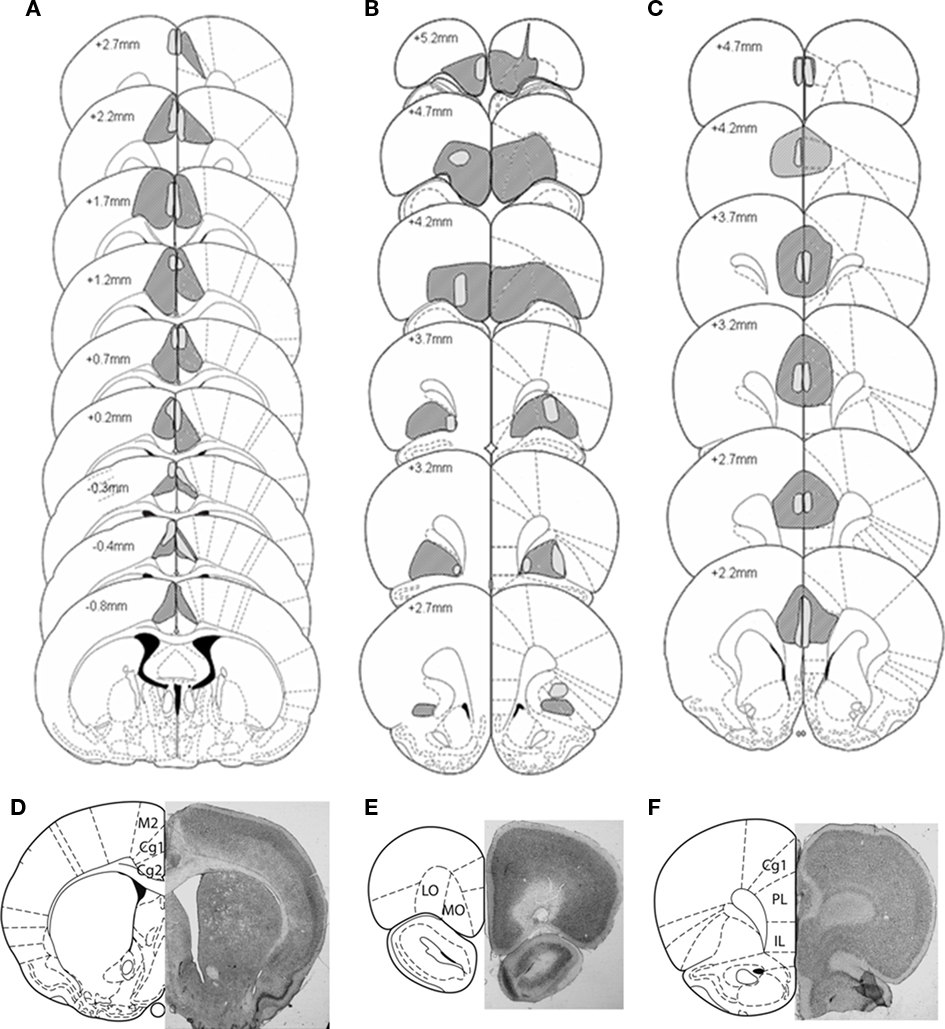
Figure 2. Representation of the largest (dark shading) and smallest (light shading) lesions at each level of the (A) Anterior Cingulate cortex (B) Orbitofrontal cortex, and (C) Prelimbic cortex lesion groups. Outlines are reproduced from Paxinos and Watson (1997), and represent sections ranging from −0.8 to 5.2 mm anterior to bregma. Microphotographs of representative lesions of the (D) Anterior Cingulate cortex, Orbitofrontal cortex (E), and Prelimbic cortex (F) lesion groups and a schematic representation of coronal sections where photographs were taken, located respectively at 4.7, 3.2, and 0.48 mm anterior to bregma.
RGT after learning of choice outcomes
We tested the possibility that inflexible and undecided behaviors of lesioned rats could have been modified in easier RGT conditions. Prior to a second test session, a month later, lesion-induced impaired rats were exposed to two consecutive daily sessions during which only advantageous or disadvantageous holes were accessible in each session and their respective outcomes identical to previous conditions. The order of presentation was counterbalanced for each group. These sessions ended when 130 pellets (advantageous holes accessible) or when 26 pellets (disadvantageous holes accessible) were obtained. The animals were then tested in the RGT the following day.
Statistical Data Analysis
Comparisons of scores (mean ± SEM) with random choice (50%) in the RGT were made using a two-tailed t-test for groups and for subgroups of good and poor decision-makers with homogenized scores. Comparisons of scores between groups were made with ANOVAs Comparisons of proportions of the subgroups were made using the Fisher exact test for 2 × 2 data (lesioned vs. shams comparisons), or the Pearson’s Chi-square exact test for independence (difference from chance level). Exact Chi-square and Fisher tests are considered as being the most appropriate analysis for comparing proportions of small samples (Agresti, 1992). Comparisons of choices during training and test sessions were made by measuring proportions of individuals with similar preferences [more than 60% preference for the same options (AB, or CD) or indifference in both cases]; or different preferences. These comparisons were made using the Chi-square exact goodness-of-fit-test.
Results
Impact of the Lesions on the General Ability to Perform the Task
Lesions of the prefrontal areas had no effect on the general ability to behave during the training sessions. Levels of activity, motivation, and sampling of the holes were unchanged:
Surgery effects on levels of activity and motivation were controlled by comparing the mean number of nose-poke per minute and the total duration of the task before reaching the criterion, during the last training session before surgery and, after surgery, during the last training session before testing. A small decrease in the number of nose-poke per minute was observed for all the rats after surgery [16.8 ± 0.5 vs. 15.0 ± 0.6 visits, n = 55; ANOVA, F(1,51) = 8.12; p < 0.01]. However, this decrease was observed similarly for the four groups [interaction effect of surgery × group, ANOVA, F(3,51) = 0.26, ns].
Surgery had no general effect on the mean duration of training sessions [before: 548 ± 18 s vs. after: 592 ± 21 s, n = 55; ANOVA, F(1,51) = 2.62, ns], whatever the group [interaction effect of surgery × group, ANOVA, F(3,51) = 0.48, ns]. Although a preference for a hole could be observed individually, no significant preference for any of the four options was observed in any group during training performed either before surgery [interaction number of visits for each option × group; ANOVA, F(9,156) = 0.73, ns] or after surgery [ANOVA, F(9,156) = 0.4, ns]. Surgery had no significant effect on the level of preference for one option during training whatever the group [interaction between largest percentage of choice for one option before and after surgery × group; ANOVA, F(3,52) = 0.31, ns].
During the testing day, there were no weight differences between each lesion group [Sham: 370 ± 7.0 g; AC: 363 ± 7.8 g; OF: 356 ± 7.0 g; PL: 375 ± 7.4 g; F(3.50) = 1.86, ns]. There was no lesion effect on the general activity [total number of nose-pokes in the four holes in the last training phase; F(3.51) = 1.98 ns] of each lesion group in training after lesion and before testing. There was no lesion effect on motivation to retrieve their reward as shown by the mean latency to collect food pellet during the test [14.5 ± 0.6 (sham); 13.6 ± 0.8 (AC); 15.4 ± 0.7 (OF); 14.8 ± 1.2 (PL) 1/10 s); ANOVA, F(1,3) = 0.79, ns]. These data indicate that none of these prefrontal cortex area is involved directly in primary motivational processes, nor in general motor activity.
Behaviors Measured in the RGT
Typically (as we observed in many distinct experiments so far), good decision-making was characterized by individuals that first chose randomly and then, progressively orientate their preference toward the more advantageous options by deduction, trials after trials. They end up making more than 70% of advantageous choices during the last 20 min of test (criterions for good decision-making). In contrast, poor decision-makers sample the different options before early developing a strong and stable preference for the disadvantageous options. They make less than 30% of advantageous choices during the last 30 min of test (criterions for poor decision-making). Undecided rats do not show any stable preference for either option all along the test. It is noteworthy that choices in the RGT of undecided, good and poor decision-making rat groups were never related to spatial preference sometimes developed during training, as previously described (Rivalan et al., 2009). In lesioned groups, a new inflexible behavior was characterized by rats that immediately preferred the pair of holes situated on the same side that they preferred during training (mean side preference during the last training day) and persisted in choosing them throughout the test without evaluating the others. This inflexible behavior could be distinguished from good and poor decision-making by a high percentage of choices (criterion: more than 85%) on the same options during the first 30 min of the test. Moreover, AC lesion delayed good decision-making compared to sham. Delayed good decision-making is considered when scores are below 70% at 30 min.
Lesion-Induced Behavioral Changes in Decision-Making
As previously described (Rivalan et al., 2009), good (56%), undecided (25%), and poor (19%) decision-makers could be identified in the RGT out of the sham lesioned rats. No preference was observed in any subgroup during the first 10 min after which the three subgroups differed on their within-session pattern of choice (Figures 3B and 4D). Good decision-makers earned significantly more food pellets across the session (174 ± 13) than undecided (92 ± 9) and poor decision-makers [72 ± 5; ANOVA, F(1,2) = 15.13, p < 0.001]. Proportion of individuals with analogous choices during training and testing did not significantly differ from chance level (Chi-square exact goodness-of-fit test, χ2 = 0.023; p = 1; ns).
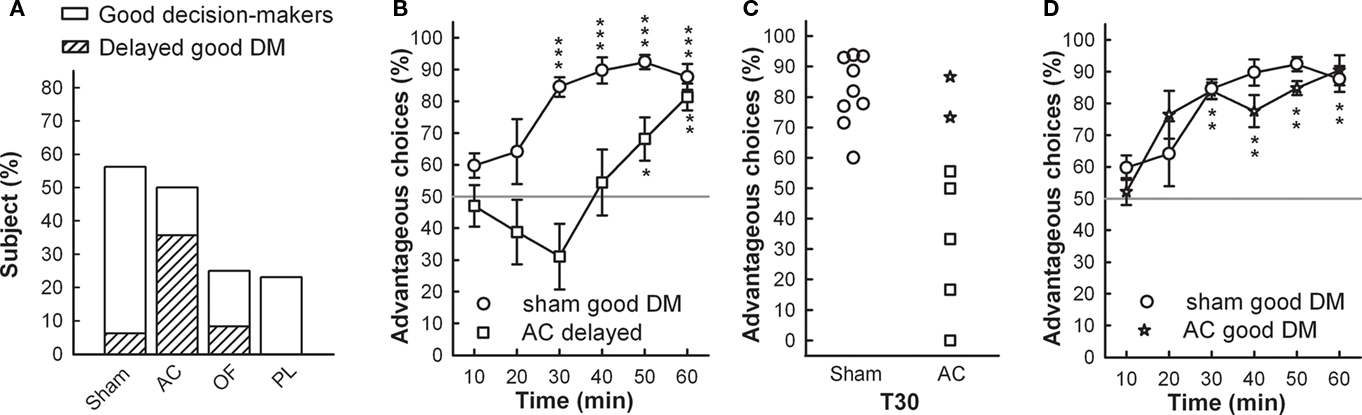
Figure 3. (A) Proportions of good decision-makers including proportion of delayed good performers (scattered bar) are represented for each group. (B) Sham and AC delayed good decision-makers’ mean time-course of advantageous choices during the RGT (mean ± SEM). (C) Individual percentage of advantageous choices at 30 min of test, in sham and AC good decision-makers. (D) Sham and AC good decision-makers’ (star symbol) mean time-course of advantageous choices during the RGT (mean ± SEM). Comparison with no preference level (gray line) t-test, *p < 0.05, **p < 0.01, ***p < 0.001.
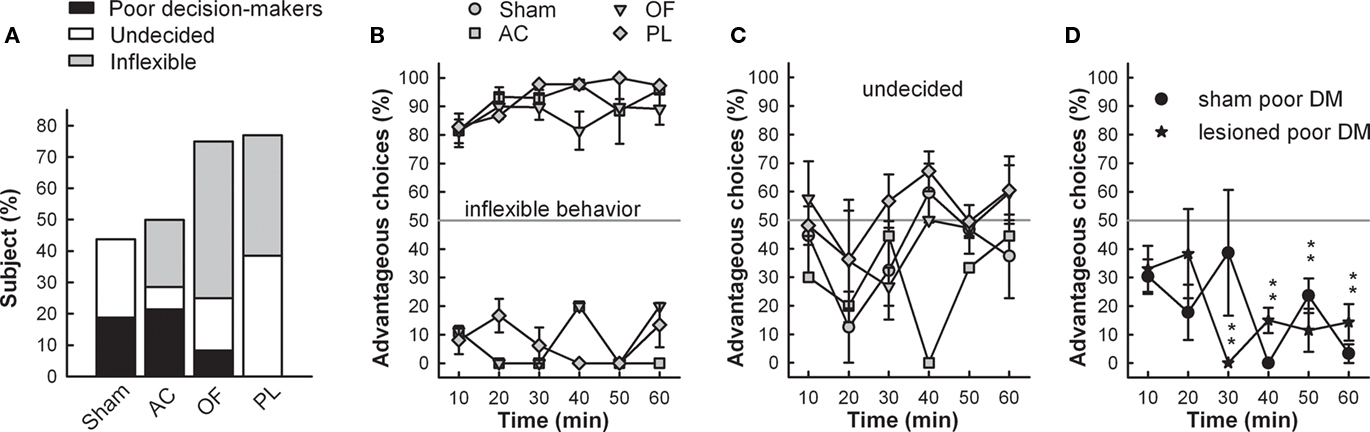
Figure 4. (A) Proportions of maladaptive behavior in RGT are represented for each group. Maladaptive behavior consists in poor decision-making (preference for disadvantageous options), undecided behavior (no preference for any option), and inflexible behavior (marked preference related to a spatial preference developed during training). Mean time-course of choices (mean ± SEM) of each group displaying inflexible (B), undecided behavior (C) as well as poor decision-makers (AC and OF lesioned rats pooled) (D). Gray line represents no preference level, 50%. Comparison of scores of inflexible lesioned rats with no preference level (gray line) t-test; ***p < 0.001.
The effects of the lesions revealed the critical role of the three prefrontal cortex areas in the resolution of the RGT. Overall, these three lesions impaired decision-making (Table 1), and induced new patterns of behavior in the RGT (detailed below). Detailed analysis revealed that the deficits induced by AC vs. PL and OF lesions induced different patterns of behaviors, in varying proportions. It is noteworthy that the proportion of side bias during training (before lesion) did not differ between sham and lesioned groups.
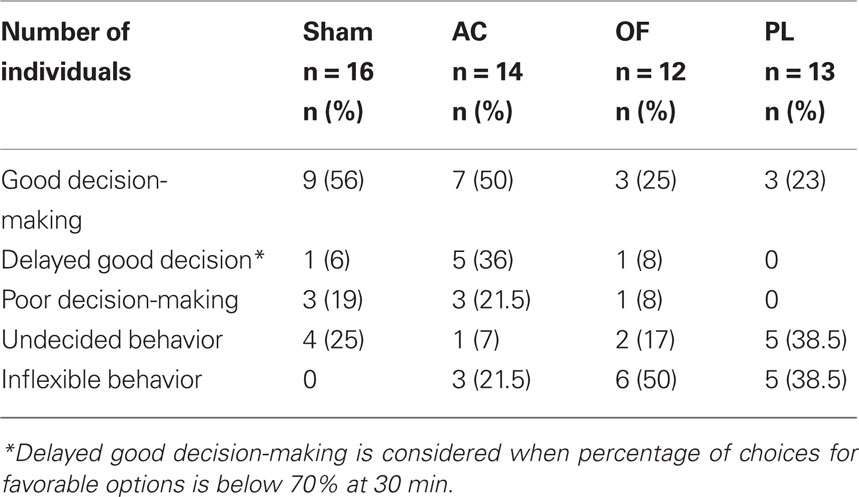
Table 1. Number and percentage of individuals exhibiting the behaviors observed in the RGT in the sham and lesioned groups in the anterior cingulate (AC), orbitofrontal (OF), prelimbic (PL) cortices.
The proportion of good decision-makers was similar after AC lesions (50%; Fisher’s exact test, p = 0.51) compared to the sham group (56%) but was reduced by half in OF and PL lesioned groups (25 and 23% respectively; p = 0.1 and p = 0.7 respectively; Figure 3A). However, although the AC good performers managed to select the advantageous options by the end of the task, they showed delayed decision-making compared to shams (Figure 3B). Whereas eight on nine good performers already had a score above 70% at 30 min, this was not yet the case for five on seven of AC good decision-makers: the proportion of those with delayed decision-making process among the good decision-makers was significantly higher than for the shams (71 vs. 11%; Fisher’s exact test, AC vs. sham, p = 0.024; Figure 3A). This particular form of behavior is shown in Figure 6B and clearly illustrated by the individual scores of the two groups at midcourse (Figure 3C). From 14 to 23% of lesioned good decision-makers behaving similarly to those observed in shams (see Figure 3D), remained in all lesioned groups.
Conversely, the proportion of maladapted decision-makers increased in OF and PL lesioned groups (Figure 4A). Among maladapted decision-makers of the three lesioned groups, inflexibility in behavior, emerged that was never observed in the sham rats. As opposed to shams, all rats with inflexible behavior (1) did not sample the four options at the beginning of the session but immediately demonstrated a preference as shown by their score during the first 10 min. (2) They remained on the holes situated on the side that they preferred during training (mean side preference during the last training day: 77.4 ± 2.9%) and persisted in choosing them throughout the test without evaluating the others (Figure 4B). This inflexible behavior resulted in more than 85% of choices on the same options during the first 30 min of the test, a score never reached by any of the good, poor, or undecided decision-makers in any group. A large proportion of rats exhibiting this behavior was observed in OF and PL lesion groups (50 and 38% respectively; Fisher’s exact test for comparison with the shams, p = 0.002 and p = 0.011) and to a lesser extent in AC rats (21%; p = 0.089; Figures 4A,B). The proportion of AC rats with analogous choice during training and testing did not significantly differ from chance level (Chi-square exact goodness-of-fit test = 1.2; p = 0.41; ns). However, the probabilities of this proportion differing from chance were much higher for OF and PL rats (χ2 = 3.86, p = 0.062 and χ2 = 4.8; p=0.05 respectively).
Beside these inflexible rats, 7 and 17% of undecided decision-makers were observed in the OF and AC lesion groups respectively, behaving like undecided shams and in similar proportions (Fisher’s exact test for comparison with the shams, p = 0.48 and p = 0.35, respectively; Figure 4C). This behavior was exhibited by the half of maladaptive PL (38%) that demonstrated similar undecided behavior during testing with large variations in their choices. These undecided rats had no marked preference for any hole even during training (mean side preference during the last training day: 63.8 ± 3.4%).
About 21.5% of poor decision-makers, behaving similarly to those observed in shams (Figure 4D), remained in the AC lesion group in identical proportion to that of shams (19%; Fisher’s exact test, AC vs. sham, p = 1), whereas they almost disappeared in PL (0%) and OF (8%) groups lesions (Figure 4A; Fisher’s exact test, PL and OF vs. sham, p = 0.23 and p = 0.60 respectively). These poor decision-makers sampled the four options during the five first minute and readily oriented their choices toward the disadvantageous options. Proportions of behaviors in the RGT for each group are summarized on Table 1.
Lesion-Induced Behavioral Changes in Decision-Making after Learning of Choice Outcomes
Acquiring information about the value of the options before choosing unchanged the decision-making process of the majority of inflexible and undecided lesioned rats in the RGT, since 12 rats on 14 remained inflexible (1 OF and 1 PL lesioned rats became undecided) and 6 on 9 remained undecided (2 OF oriented their choices toward the advantageous options and 1 PL toward the disadvantageous ones).
Histological Results
The largest and smallest lesions of each prefrontal cortex area, as well as photomicrographs of representative lesions, are represented in Figure 2. Histological control of the brains revealed that one sham rat had substantial damages located in the secondary motor cortex area M2 and was therefore excluded from the study. The relationships between the size of the anterior cingulate, OF, and PL cortex lesions and the behaviors in the RGT were evaluated (see Table A1 in Appendix). Among lesioned rats, two AC, four OF, and four PL were excluded from the analyses because the lesion size was not significant (less than 50% of the area on more than two consecutive histological 50 μm slices). The final group sizes were: 16 sham rats, 14 AC, 12 OF, and 13 PL. All AC lesions were more anterior to the PL area (maximum + 2.7). Each behavioral pattern in the RGT could be induced by any of the prefrontal cortex lesion size.
Discussion
Using our rat version of the human IGT, we show that the prefrontal cortex plays a critical role in the resolution of the task and that the effects of distinct prefrontal cortex area lesions vary according to the subject.
As previously shown (Rivalan et al., 2009), the RGT is efficient in revealing inter-individual differences since the majority of the control rats can evaluate and progressively deduce favorable options within a 1-h session, despite the complexity of the task, whereas other rats systematically choose the disadvantageous options.
We found that three key prefrontal cortex sub areas, namely the OF cortex, the PL cortex, and the cingulate cortex (AC) are solicited for decision-making in the RGT. Such a pattern of results indicates that decision processes might result from coordinated activity within the prefrontal areas, as suggested by recent evidence (Endepols et al., 2010).
It is likely that the coordination of decision-making within the prefrontal sub areas reflects the integration of different forms of cost-benefit decision processes. In fact, previous research suggests that time-related choice as assessed in delay-discounting tasks involves the integrity of the OF and the PL, but not of the AC (Cardinal et al., 2001; Mobini et al., 2002; Winstanley et al., 2004; Floresco et al., 2008). In contrast, various data suggests that decisions related to the effort required to get access to optimal reward depends on the integrity of the AC, but not OF or PL (Walton et al., 2003; Rudebeck et al., 2006). Risky decision-making, as assessed in tasks similar to the one used in the present study, has been shown to depend upon the OF (Pais-Vieira et al., 2007; Zeeb and Winstanley, 2011). It is important to note that decision-making in each of the abovementioned tasks additionally involves attentional and mnesic processes, which have also been shown to depend on the integrity of the medial prefrontal cortex (see Granon et al., 1994; Muir et al., 1996).
In addition to these prefrontal areas, recent data have demonstrated that the coordination of decision-making in relation to cost-benefit processes involves extended neural circuits (Cardinal, 2006). This research has demonstrated in particular that effort-related decision processes might depend upon the relationship between the AC and accumbens regions (Hauber and Sommer, 2009). This research has also provided evidence that the amygdala sends important information related to the integration of outcome representation (Balleine and Killcross, 2006).
In the present study, we found that the effects of the lesions were far from homogeneous, suggesting various levels of adaptability according to the individual. Some lesioned individuals behaved like controls whereas others were just less efficient, as shown by delayed good decision-making predominantly induced by AC lesion. Others were drastically impaired, as observed for PL and OF lesions that predominantly induced various patterns of inadapted behaviors, i.e., undecided and inflexible behaviors. Inflexible behavior in the RGT was largely induced by OF and PL, but also in a few AC. This behavior was characterized by choices similar to those preferred during training, reflecting an inability to detect changes in condition contingencies and to adapt preferences toward a specific option. The possibility that these impaired lesioned rats could have solved the RGT in easier conditions can be excluded since we failed to observe any improvement after previous learning of choice outcomes. It is therefore tempting to suggest that this variation in decision-making in the impact of sub area lesioning is reflected in individual variation in the neural circuit of basic cognitive processes.
Several studies in the literature highlight the role of OF, PL, and AC in behavioral flexibility, but their respective involvement in this process does not seem to rely on the same mechanisms. This functional differentiation is supported by differences in the anatomical connections to these regions (Moghaddam and Homayoun, 2008). Lesions or drug infusions in the OF impair reversal learning which requires a shift of a specific choice pattern, and induce perseverative responding (Bohn et al., 2003; Chudasama and Robbins, 2003; Mcalonan and Brown, 2003). The OF cortex receives prominent inputs from sensory associative cortices, as well as from the hypothalamus and amygdala, suggesting that it plays a role in integrating potentially salient information about environmental contingencies (Ongur and Price, 2000). Indeed, converging evidence indicates that the main role of the OF is to signal the value of an expected outcome (Stalnaker et al., 2009). Damage to the OF may therefore prevent the adequate integration of information about the consequences of responding for a signaled reward and the subjective value of that rewarding outcome (Schoenbaum et al., 1999; Ostlund and Balleine, 2007). Accordingly, OF lesioned rats impaired in the RGT could not perceive the change in the contingencies’ values between training and the test, inducing perseverative responding because OF lesioned rats continue to encode the old contingencies or fail to encode the new ones (Delamater, 2007).
The mPFC is crucial for coordinating actions and habits and current research suggests that the PL is a key component of the neural circuit regulating goal-directed responding both in rodents and primates (Balleine and O’doherty, 2010). PL lesions induce behavioral alterations in adaptation to both outcome value and reward contingency changes, that might have rendered behavior habit-based (Corbit and Balleine, 2003; Killcross and Coutureau, 2003; Ostlund and Balleine, 2005; Coutureau et al., 2009). Altered behavior of PL lesioned rats in the present ambiguous decision-making task might therefore be taken as a further argument of habit-based responding following PL lesions. It is worth noting that mPFC dopaminergic signal arising from the ventral tegmental area has recently been shown to trigger adaptation to reward contingency changes, likely through a prediction error signal mechanism (Naneix et al., 2009). As a consequence, perseverative responding in PL rats likely results from an inability to detect action-outcome contingency variations whether rats are good or bad decision-makers.
The AC is strongly interconnected with OF and PL (Paus et al., 2001; Heidbreder and Groenewegen, 2003; Wang et al., 2004), suggesting tight functional interactions. Data in humans showed that AC and dlPFC, that play similar roles to the rodent PL, are both engaged in task switching, so that the AC monitors and detects the presence of conflict related to actions, whereas the dlPFC engages a top-down process required to deal with it (Hyafil et al., 2009). A current modeling suggests that the AC signals error-likelihood (Sallet et al., 2007) since it predicts that the more cognitive control is required due to increased task demand, the more the AC is activated (Brown and Braver, 2005). This model is consistent with the proposed key role of the AC in choosing appropriate actions when the environment is uncertain or dynamic (Kennerley et al., 2006; Quilodran et al., 2008), and in combining information about the costs and benefits associated with alternative actions (Rudebeck et al., 2006). Indeed, several data in the rat suggest that the complexity of the task is a critical factor that engages the AC (Ragozzino and Rozman, 2007). In the RGT, the AC could be engaged specifically when the task difficulty is enhanced, by signaling the utilities associated with the different options. This could explain why AC lesioned good decision-makers are slower, as observed when decision-making had become more demanding by increased task difficulty (Rivalan et al., 2009). In contrast, the AC may not be engaged in the same manner in individuals that do not take into account the complexity of the task, leading to inflexible behavior or to an absence of effect in these individuals (AC lesioned poor decision-makers are in the same proportions as shams and behave similarly). Recently, findings in humans revealed that the AC is also involved in risky decision-making (De Martino et al., 2006; Brown and Braver, 2008), a characteristic of rat poor decision-makers (Rivalan et al., 2009). Indeed, choosing high-risk decisions is associated with different patterns of OF and AC activations (Cohen et al., 2005), and higher risk-proneness demonstrated by substance abusers correlates with hypoactivity of the AC (Brown and Braver, 2007, 2008). These results could explain the absence of effect of AC lesion in some individuals, an area which could be disengaged in these risk-prone poor decision-makers.
Strikingly, lesions had no effect in some individuals that exhibited good decision-making, suggesting that compensatory mechanisms could be involved. It is likely that the recruitment of alternative cognitive resources within the prefrontal network, combined with subcortical areas like the striatum, allows some individuals to perform the task. This area is involved in encoding S–R association and in selecting pertinent information related to the expected reward (Hassani et al., 2001; Stalnaker et al., 2009).
Conclusion
This study demonstrates that three distinct subregions of the rat prefrontal cortex are involved in resolution of a complex decision-making task and, more importantly, it reveals varying degrees of functional specialization of these subregions that largely depends on the individuals. This behavioral variability in prefrontal cortex area recruitment may depend on (1) the inter-individual capacities of the rat to solve the task, as shown by the AC lesion effects, probably because the prefrontal network is differentially engaged according to the rat’s natural ability to cope with the balance between risk and “wisdom” (Tobler et al., 2009); and (2) the ability to recruit alternative brain networks to compensate for the deficit. The inter-individual differences in lesion studies should be considered in the future since they could constitute an important bias that may explain contradictions in the literature.
Finally, our model should promote research on the psychobiological bases and brain networks involved in good and poor decision-making, as it allows a rapid and reliable assessment of executive functions, with a high degree of comparability to human performance. It provides a promising tool in the search for vulnerability markers of poor decision-making and related psychiatric disorders.
Conflict of Interest Statement
The authors declare that the research was conducted in the absence of any commercial or financial relationships that could be construed as a potential conflict of interest.
Acknowledgments
We thank R. Godfrey and Dr. K. Clemens for editorial assistance; Dr. M. Cador and A. Marchand for helpful comments; S. Lelgouach, T. Guégan, A. Fayoux, P. Gonzalez, C. Darracq, and M. H. Bruyères for technical assistance. This work was supported by the French Research Council (CNRS), the University Victor Segalen Bordeaux 2, the Conseil Régional Aquitaine, a fellowship from the French Government to Marion Rivalan, and by Fondation M. Bleustein-Blanchet pour la Vocation to Aurélie Fitoussi.
References
Balleine, B. W., and Killcross, S. (2006). Parallel incentive processing: an integrated view of amygdala function. Trends Neurosci. 29, 272–279.
Balleine, B. W., and O’doherty, J. P. (2010). Human and rodent homologies in action control: corticostriatal determinants of goal-directed and habitual action. Neuropsychopharmacology 35, 48–69.
Bechara, A., Damasio, A. R., Damasio, H., and Anderson, S. W. (1994). Insensitivity to future consequences following damage to human prefrontal cortex. Cognition 50, 7–15.
Bechara, A., and Damasio, H. (2002). Decision-making and addiction (part I): impaired activation of somatic states in substance dependent individuals when pondering decisions with negative future consequences. Neuropsychologia 40, 1675–1689.
Bechara, A., Damasio, H., Tranel, D., and Damasio, A. R. (1997). Deciding advantageously before knowing the advantageous strategy. Science 275, 1293–1295.
Bechara, A., Dolan, S., and Hindes, A. (2002). Decision-making and addiction (part II): myopia for the future or hypersensitivity to reward? Neuropsychologia 40, 1690–1705.
Bechara, A., Tranel, D., and Damasio, H. (2000). Characterization of the decision-making deficit of patients with ventromedial prefrontal cortex lesions. Brain 123(Pt 11), 2189–2202.
Blondeau, C., and Dellu-Hagedorn, F. (2007). Dimensional analysis of ADHD subtypes in rats. Biol. Psychiatry 61, 1340–1350.
Bohn, I., Giertler, C., and Hauber, W. (2003). Orbital prefrontal cortex and guidance of instrumental behaviour in rats under reversal conditions. Behav. Brain Res. 143, 49–56.
Brown, J. W., and Braver, T. S. (2005). Learned predictions of error likelihood in the anterior cingulate cortex. Science 307, 1118–1121.
Brown, J. W., and Braver, T. S. (2007). Risk prediction and aversion by anterior cingulate cortex. Cogn. Affect. Behav. Neurosci. 7, 266–277.
Brown, J. W., and Braver, T. S. (2008). A computational model of risk, conflict, and individual difference effects in the anterior cingulate cortex. Brain Res. 1202, 99–108.
Buelow, M. T., and Suhr, J. A. (2009). Construct validity of the Iowa gambling task. Neuropsychol. Rev. 19, 102–114.
Cardinal, R. N. (2006). Neural systems implicated in delayed and probabilistic reinforcement. Neural. Netw. 19, 1277–1301.
Cardinal, R. N., Pennicott, D. R., Sugathapala, C. L., Robbins, T. W., and Everitt, B. J. (2001). Impulsive choice induced in rats by lesions of the nucleus accumbens core. Science 292, 2499–2501.
Carlson, S. M., Zayas, V., and Guthormsen, A. (2009). Neural correlates of decision making on a gambling task. Child Dev. 80, 1076–1096.
Cazalis, F., Valabregue, R., Pelegrini-Issac, M., Asloun, S., Robbins, T. W., and Granon, S. (2003). Individual differences in prefrontal cortical activation on the tower of London planning task: implication for effortful processing. Eur. J. Neurosci. 17, 2219–2225.
Chudasama, Y., and Robbins, T. W. (2003). Dissociable contributions of the orbitofrontal and infralimbic cortex to pavlovian autoshaping and discrimination reversal learning: further evidence for the functional heterogeneity of the rodent frontal cortex. J. Neurosci. 23, 8771–8780.
Cohen, M. X., Heller, A. S., and Ranganath, C. (2005). Functional connectivity with anterior cingulate and orbitofrontal cortices during decision-making. Brain Res. Cogn. Brain Res. 23, 61–70.
Corbit, L. H., and Balleine, B. W. (2003). The role of prelimbic cortex in instrumental conditioning. Behav. Brain Res. 146, 145–157.
Coutureau, E., Marchand, A. R., and Di Scala, G. (2009). Goal-directed responding is sensitive to lesions to the prelimbic cortex or basolateral nucleus of the amygdala but not to their disconnection. Behav. Neurosci. 123, 443–448.
De Martino, B., Kumaran, D., Seymour, B., and Dolan, R. J. (2006). Frames, biases, and rational decision-making in the human brain. Science 313, 684–687.
Delamater, A. R. (2007). The role of the orbitofrontal cortex in sensory-specific encoding of associations in pavlovian and instrumental conditioning. Ann. N. Y. Acad. Sci. 1121, 152–173.
Dunn, B. D., Dalgleish, T., and Lawrence, A. D. (2006). The somatic marker hypothesis: a critical evaluation. Neurosci. Biobehav. Rev. 30, 239–271.
Endepols, H., Sommer, S., Backes, H., Wiedermann, D., Graf, R., and Hauber, W. (2010). Effort-based decision making in the rat: an [18F]fluorodeoxyglucose micro positron emission tomography study. J. Neurosci. 30, 9708–9714.
Ernst, M., and Paulus, M. P. (2005). Neurobiology of decision making: a selective review from a neurocognitive and clinical perspective. Biol. Psychiatry 58, 597–604.
Fellows, L. K. (2004). The cognitive neuroscience of human decision making: a review and conceptual framework. Behav. Cogn. Neurosci. Rev. 3, 159–172.
Floresco, S. B., Onge, J. R., Ghods-Sharifi, S., and Winstanley, C. A. (2008). Cortico-limbic-striatal circuits subserving different forms of cost-benefit decision making. Cogn. Affect. Behav. Neurosci. 8, 375–389.
George, O., and Koob, G. F. (2010). Individual differences in prefrontal cortex function and the transition from drug use to drug dependence. Neurosci. Biobehav. Rev. 35, 232–247.
Granon, S., Vidal, C., Thinus-Blanc, C., Changeux, J. P., and Poucet, B. (1994). Working memory, response selection, and effortful processing in rats with medial prefrontal lesions. Behav. Neurosci. 108, 883–891.
Hassani, O. K., Cromwell, H. C., and Schultz, W. (2001). Influence of expectation of different rewards on behavior-related neuronal activity in the striatum. J. Neurophysiol. 85, 2477–2489.
Hauber, W., and Sommer, S. (2009). Prefrontostriatal circuitry regulates effort-related decision making. Cereb. Cortex 19, 2240–2247.
Heidbreder, C. A., and Groenewegen, H. J. (2003). The medial prefrontal cortex in the rat: evidence for a dorso-ventral distinction based upon functional and anatomical characteristics. Neurosci. Biobehav. Rev. 27, 555–579.
Hyafil, A., Summerfield, C., and Koechlin, E. (2009). Two mechanisms for task switching in the prefrontal cortex. J. Neurosci. 29, 5135–5142.
Kennerley, S. W., Walton, M. E., Behrens, T. E., Buckley, M. J., and Rushworth, M. F. (2006). Optimal decision making and the anterior cingulate cortex. Nat. Neurosci. 9, 940–947.
Killcross, S., and Coutureau, E. (2003). Coordination of actions and habits in the medial prefrontal cortex of rats. Cereb. Cortex 13, 400–408.
Lawrence, N. S., Jollant, F., O’daly, O., Zelaya, F., and Phillips, M. L. (2009). Distinct roles of prefrontal cortical subregions in the Iowa Gambling Task. Cereb. Cortex 19, 1134–1143.
Manes, F., Sahakian, B., Clark, L., Rogers, R., Antoun, N., Aitken, M., and Robbins, T. (2002). Decision-making processes following damage to the prefrontal cortex. Brain 125, 624–639.
Mcalonan, K., and Brown, V. J. (2003). Orbital prefrontal cortex mediates reversal learning and not attentional set shifting in the rat. Behav. Brain Res. 146, 97–103.
Mcguire, J. T., and Botvinick, M. M. (2010). Prefrontal cortex, cognitive control, and the registration of decision costs. Proc. Natl. Acad. Sci. U.S.A. 107, 7922–7926.
Mobini, S., Body, S., Ho, M. Y., Bradshaw, C. M., Szabadi, E., Deakin, J. F., and Anderson, I. M. (2002). Effects of lesions of the orbitofrontal cortex on sensitivity to delayed and probabilistic reinforcement. Psychopharmacology (Berl.) 160, 290–298.
Moghaddam, B., and Homayoun, H. (2008). Divergent plasticity of prefrontal cortex networks. Neuropsychopharmacology 33, 42–55.
Muir, J. L., Everitt, B. J., and Robbins, T. W. (1996). The cerebral cortex of the rat and visual attentional function: dissociable effects of mediofrontal, cingulate, anterior dorsolateral, and parietal cortex lesions on a five-choice serial reaction time task. Cereb. Cortex 6, 470–481.
Naneix, F., Marchand, A. R., Di Scala, G., Pape, J. R., and Coutureau, E. (2009). A role for medial prefrontal dopaminergic innervation in instrumental conditioning. J. Neurosci. 29, 6599–6606.
Ongur, D., and Price, J. L. (2000). The organization of networks within the orbital and medial prefrontal cortex of rats, monkeys and humans. Cereb. Cortex 10, 206–219.
Ostlund, S. B., and Balleine, B. W. (2005). Lesions of medial prefrontal cortex disrupt the acquisition but not the expression of goal-directed learning. J. Neurosci. 25, 7763–7770.
Ostlund, S. B., and Balleine, B. W. (2007). Orbitofrontal cortex mediates outcome encoding in Pavlovian but not instrumental conditioning. J. Neurosci. 27, 4819–4825.
Pais-Vieira, M., Lima, D., and Galhardo, V. (2007). Orbitofrontal cortexr lesions disrupt risk assessment in a novel serial decision-making task for rats. Neuroscience 145, 225–231.
Paus, T., Castro-Alamancos, M. A., and Petrides, M. (2001). Cortico-cortical connectivity of the human mid-dorsolateral frontal cortex and its modulation by repetitive transcranial magnetic stimulation. Eur. J. Neurosci. 14, 1405–1411.
Paxinos, G., and Watson, C. (1997). The Rat Brain in Stereotaxis Coordinates. San Diego: Academic Press.
Quilodran, R., Rothe, M., and Procyk, E. (2008). Behavioral shifts and action valuation in the anterior cingulate cortex. Neuron 57, 314–325.
Ragozzino, M. E., and Rozman, S. (2007). The effect of rat anterior cingulate inactivation on cognitive flexibility. Behav. Neurosci. 121, 698–706.
Rangel, A. (2008). Consciousness meets neuroeconomics: what is the value of stimulus awareness in decision making? Neuron 59, 525–527.
Rivalan, M., Ahmed, S. H., and Dellu-Hagedorn, F. (2009). Risk-prone individuals prefer the wrong options on a rat version of the Iowa gambling task. Biol. Psychiatry 66, 743–749.
Rudebeck, P. H., Walton, M. E., Smyth, A. N., Bannerman, D. M., and Rushworth, M. F. (2006). Separate neural pathways process different decision costs. Nat. Neurosci. 9, 1161–1168.
Rushworth, M. F., Behrens, T. E., Rudebeck, P. H., and Walton, M. E. (2007). Contrasting roles for cingulate and orbitofrontal cortex in decisions and social behaviour. Trends Cogn. Sci. (Regul. Ed.) 11, 168–176.
Sallet, J., Quilodran, R., Rothe, M., Vezoli, J., Joseph, J. P., and Procyk, E. (2007). Expectations, gains, and losses in the anterior cingulate cortex. Cogn. Affect. Behav. Neurosci. 7, 327–336.
Schoenbaum, G., Chiba, A. A., and Gallagher, M. (1999). Neural encoding in orbitofrontal cortex and basolateral amygdala during olfactory discrimination learning. J. Neurosci. 19, 1876–1884.
Stalnaker, T. A., Takahashi, Y., Roesch, M. R., and Schoenbaum, G. (2009). Neural substrates of cognitive inflexibility after chronic cocaine exposure. Neuropharmacology 56(Suppl. 1), 63–72.
Tobler, P. N., Christopoulos, G. I., O’doherty, J. P., Dolan, R. J., and Schultz, W. (2009). Risk-dependent reward value signal in human prefrontal cortex. Proc. Natl. Acad. Sci. U.S.A. 106, 7185–7190.
Van Den Bos, R., Lasthuis, W., Den Heijer, E., Van Der Harst, J., and Spruijt, B. (2006). Toward a rodent model of the Iowa gambling task. Behav. Res. Methods 38, 470–478.
Walton, M. E., Bannerman, D. M., Alterescu, K., and Rushworth, M. F. (2003). Functional specialization within medial frontal cortex of the anterior cingulate for evaluating effort-related decisions. J. Neurosci. 23, 6475–6479.
Wang, Y., Matsuzaka, Y., Shima, K., and Tanji, J. (2004). Cingulate cortical cells projecting to monkey frontal eye field and primary motor cortex. Neuroreport 15, 1559–1563.
Winstanley, C. A., Theobald, D. E., Cardinal, R. N., and Robbins, T. W. (2004). Contrasting roles of basolateral amygdala and orbitofrontal cortex in impulsive choice. J. Neurosci. 24, 4718–4722.
Zeeb, F. D., Robbins, T. W., and Winstanley, C. A. (2009). Serotonergic and dopaminergic modulation of gambling behavior as assessed using a novel rat gambling task. Neuropsychopharmacology 34, 2329–2343.
Keywords: animal model, choice, executive functions, inflexible behavior, inter-individual differences, prefrontal cortex, lesion
Citation: Rivalan M, Coutureau E, Fitoussi A and Dellu-Hagedorn F (2011) Inter-individual decision-making differences in the effects of cingulate, orbitofrontal, and prelimbic cortex lesions in a rat gambling task. Front. Behav. Neurosci. 5:22. doi: 10.3389/fnbeh.2011.00022
Received: 15 February 2011; Accepted: 11 April 2011;
Published online: 27 April 2011.
Edited by:
Carmen Sandi, École Polytechnique Fédérale de Lausanne, SwitzerlandReviewed by:
Carmen Sandi, École Polytechnique Fédérale de Lausanne, SwitzerlandSylvie Granon, Université Paris Sud XI, France
Copyright: © 2011 Rivalan, Coutureau, Fitoussi and Dellu-Hagedorn. This is an open-access article subject to a non-exclusive license between the authors and Frontiers Media SA, which permits use, distribution and reproduction in other forums, provided the original authors and source are credited and other Frontiers conditions are complied with.
*Correspondence: Françoise Dellu-Hagedorn, Aquitaine Institute of Cognitive and Integrative Neuroscience, Université de Bordeaux, CNRS UMR 5287, BP 31, 146 rue Léo Saignat, 33076 Bordeaux Cedex, France. e-mail: francoise.dellu@u-bordeaux2.fr