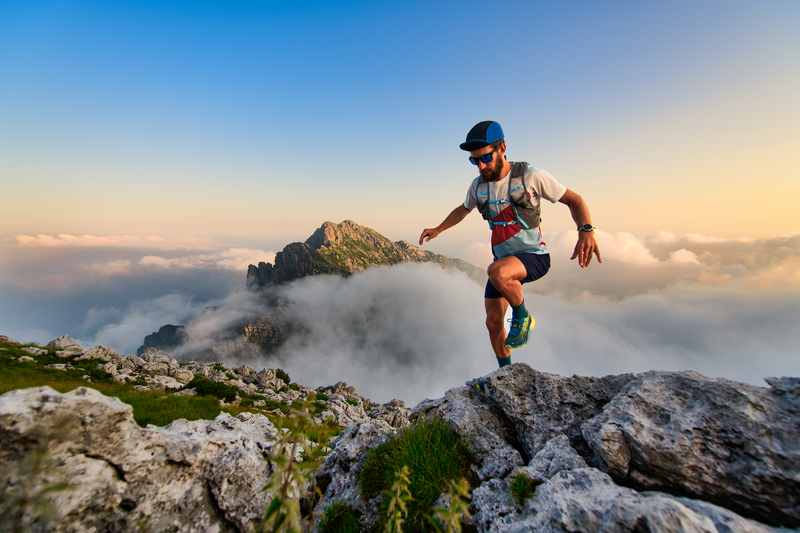
94% of researchers rate our articles as excellent or good
Learn more about the work of our research integrity team to safeguard the quality of each article we publish.
Find out more
ORIGINAL RESEARCH article
Front. Behav. Neurosci. , 29 December 2010
Sec. Individual and Social Behaviors
Volume 4 - 2010 | https://doi.org/10.3389/fnbeh.2010.00191
Although somatosensation in multiple whisker systems has been studied in considerable detail, relatively little information is available regarding whisker usage and movement patterns during natural behaviors. The Etruscan shrew, one of the smallest mammals, relies heavily on its whisker system to detect and kill its highly mobile insect prey. Here, we tracked whisker and body motion during prey capture. We found that shrews made periodic whisker movements (whisking) with frequencies ranging from 12 to 17 Hz. We compared shrew and rat whisking and found that shrew whisking was smaller amplitude and higher frequency than rat whisking, but that the shrew and rat whisking cycle were similar in that the velocity was higher during retraction than protraction. We were able to identify four phases during the shrew hunting behavior: (i) an immobile phase often preceding hunting, (ii) a search phase upon the initiation of hunting, (iii) a contact phase defined by whisker-to-cricket contact, and (iv) an attack phase, characterized by a rapid head movement directed toward the cricket. During the searching phase, whisking was generally rhythmic and whiskers were protracted forward. After prey contact, whisking amplitude decreased and became more variable. The final strike was associated with an abrupt head movement toward the prey with high head acceleration. Prey capture proceeded extremely fast and we obtained evidence that shrews can initiate corrective maneuvers with a minimal latency <30 ms. While the shrew’s rostrum is straight and elongated during most behaviors, we show for the first time that shrews bend their rostrum during the final strike and grip their prey with a parrot beak shaped snout.
Facial vibrissae, or whiskers, are used by almost all mammals as a tactile sensory organ (Vincent, 1912; Meyer and Meyer, 1992). Whisker systems are considered to be one of the primary channels used by nocturnal mammals to acquire information about their surrounding environment (Sokolov and Kulikov, 1987; Diamond et al., 2008). Particularly, it has been shown that some rodents actively move, or whisk, their vibrissae to gather tactile information (Welker, 1964; Lee and Woolsey, 1975; Wineski, 1985; Mitchinson et al., 2007; Wolfe et al., 2008). Whisker motion is predominantly in the horizontal plane, although it can have a significant vertical component (Bermejo et al., 2002). Whisking can be highly regular and periodic, and ranges in frequency from 5 to 25 Hz, depending on both the animal species and the behavior (Jin et al., 2004). Understanding how animals use their whiskers to gather information about their environment is crucial to understanding how that information is encoded by nuclei in the brain. For example, rats typically whisk at about 8 Hz with large amplitude during exploration, but after contact with an object, whisking amplitude may get smaller and the positioning of whiskers may change so as to maximize the number of contacts between the whisker array and the object (Grant et al., 2009). This suggests separate encoding schemes for object localization and object identification (Brecht et al., 1997).
More recently, work has been done to look at sensory coding in the shrew whisker system. The shrew is an attractive model for studying sensory coding due to its comparatively simple and small brain which has relatively few neurons compared to other mammals. The shrew has an impressive array of whiskers on its snout but very poor eyesight (Catania et al., 2008). Anjum et al. (2006) showed that Etruscan Shrews are highly dependent on their facial whiskers both for searching and directing attacks on their prey. More recently, it was found that the American Water Shrew uses tactile cues during underwater hunting which are likely whisker mediated (Catania et al., 2008). These behavioral observations are in agreement with physiological experiments, which have shown that large parts of sensory cortex are occupied by the S1 and S2 representations of the facial vibrissae (Catania, 2000; Roth-Alpermann et al., 2010). Currently, however, very little is known about how shrews use their whiskers during exploration and object recognition. Because of their small size, shrews make very quick movements and their whiskers are smaller and thinner than most rodents, making them difficult to visualize and track. However, in order to advance our understanding of tactile coding in the shrew whisker system, more detailed analysis of shrew whisker motion is needed.
Here we used very small, light reflective tags and high-speed videography to track whisker motion during cricket hunting and capture. We combined this with body tracking to address the following questions: (i) Are the shrew’s whiskers under active muscle control and do they periodically sweep their whiskers back and forth during searching (as in rats and mice)? (ii) Does the whisking behavior change over the course of pursuing and capturing their prey? (iii) How does head motion and orientation change to facilitate prey capture? We show that Etruscan shrews actively whisk at approximately 14 Hz while searching for their cricket prey. Upon contacting the cricket, whisking amplitude decreases and there is a small head acceleration. We found that shrews hold their whiskers more protracted as they begin to hunt and may be further protracted after making contact with the cricket. Additionally, we found that shrews attacked with very high head acceleration, reaching upwards of 1500 cm/s2, and were able to make very fast mid-attack changes in trajectory. Interestingly, we found that shrews bent their snout into a beak-like shape, likely in an effort to draw their prey closer to their mouth.
All observations refer to eight adult male Etruscan shrews. All shrews were offspring of captive breading, and bred and housed in the institute colony as previously described (Anjum et al., 2006).
Hunting movies used for whisker analysis were taken from above the arena (Figure 1A, left) under very low visible light conditions (Basler A504k camera, 1280 × 1024 pixels, light illuminance < 0.01 lux). Because all of our analysis was restricted to frequencies less than approximately 25 Hz, we filmed with a frame rate of 300 Hz which was adequate for our purposes. The arena was 9 × 9 cm and was illuminated from above with infrared light (880 nm, ABUS Mega-LED M). A background image with no shrew present was taken which was later subtracted from each frame. Before filming, the head was marked with non-toxic white marker for head tracking and whiskers were tagged with small reflective tags (see below). The cricket was then placed in the opposite corner of the arena shortly after filming began. No more than six hunting movies were filmed per day to ensure that the hunting behavior was not satiated.
Figure 1. Behavioral setup and whisker tagging. (A) Left: Behavioral setup for filming free hunting. Shrews were filmed in an arena (9 × 9 × 9 cm) from above (300-Hz frame rate). Movies were made under infrared (IR) illumination (λ = 880 nm). Right: in this setup the cricket was fixed in place and a mirror placed at a 45° angle allowed for simultaneous filming from the top and side (B) Left: diagram of whisker positions on shrew snout, red circles denote whiskers which were targeted for tagging. Middle: picture of a tag under visible light, circled in red. Right: tagged whisker under infrared illumination. (C) Variables used for quantifying shrew head and whisker motion.
For simultaneous top and side filming (Figure 1A, right), we altered the experimental setup such that the shrews had to walk down an elevated walkway. At the end of this walkway, a cricket was fixed on its legs. Movies were taken from above with a frame rate of 400 Hz and the field of view was reduced to 5 × 6.25 cm. A mirror (Kugler) was placed in parallel to the walkway at a 45° angle to yield a top as well as side view of the shrew during the attack. In this setup whiskers were not tracked and the shrews were not whisker tagged.
In order to track the whiskers we tagged one whisker on each side of the snout. During the tagging procedure, shrews were anesthetized using isoflurane (2–4%) vaporized in oxygen and breathing was monitored visually. The tag was constructed by forming a small drop of high viscosity UV glue (Dymax) approximately one-third of the distance from the whisker base to the tip. The glue was then illuminated with UV light (365 nm) for 5–10 s to facilitate the hardening process (Dymax Bluewave 50). After hardening, the bottom part of the tag was covered with silver paint. The tags were constructed to be as small as possible with a diameter of roughly 250 μm. We tagged whiskers in row B but varied the selected whisker column because whiskers were at times undergoing regrowth after having naturally fallen off. The positions of whiskers targeted for tagging are shown in Figure 1B, left. Tagged whiskers are shown under both visible and infrared illumination (red circles, Figure 1B, middle and right respectively). Shrews were put back in their home cage for at least 5 h before starting the experiment. Tags typically lasted 3–5 days before they either came off or the whisker came out, at which point shrews were retagged. Because we found whisking on the left and right sides to be largely coherent, only whisker motion from one side of the snout was included in data analyses, with the exception of coherence measurements. This was done in order to avoid using highly correlated motion as two independent trials. The whisker with the most clearly visible tag was chosen for analysis. No difference in behavior was detected comparing the day the shrew was tagged with consecutive days.
Whisker and head tracking were performed using custom written Matlab routines. The nose, head dot, whisker tag and whisker base positions were manually determined for the first frame of each video and then were automatically tracked on subsequent frames. The white dot which had been marked on the head was used to determine the head position. A midline drawn between the head position and the nose position (defined as a point of maximum curvature of the shrew head contour, Figure 1C) was used to estimate the head direction (0° was defined as pointing down in our images). A line drawn between the head position and the whisker base was used to determine the angular position of the whisker base relative to the head position in a shrew-centered coordinate frame (dashed black line Figure 1C). The intersection between a line drawn at this angle (α, Figure 1C) and the shrew contour was used to estimate the whisker base position on subsequent frames. The tracking algorithm was automated however, it was necessary to visually verify the calculated whisker base position. If the calculated whisker base position strayed more than the width of the whisker from the visually identified position, the program was interrupted and the base position was reset. Typically, the whisker base position had to be manually reset every 5–20 frames, depending on how much the shape of the head image was changing. To calculate whisker angle, a tangent along the face contour was drawn at the whisker’s base position. The line perpendicular to this tangent was defined as 0° (red line Figure 1C). Whisker angles anterior to 0° were positive and angles posterior were negative. This is a similar convention as (Hill et al., 2008) but with a 90° offset. We chose to measure whisker angle relative to the pad rather than the head midline in order to avoid including whisker motion which was due to movement of the snout or pad as a whole, such as could arise from sniffing. The average angle of the shrew body contour at the whisker base relative to the head midline was 20.4 ± 1.9° (mean ± SD, n = 1200 frames). Therefore, to use the whisker angles reported here to estimate the whisker angle relative to the midline one can use the equation φ = 90° – 20.4° – θ. Where φ is the angle relative to the midline and θ is measured as shown in Figure 1C. It should be noted that this is an approximation using the average contour angle, in order to be strictly precise one would need to know the exact angle of the contour at the whisker base for every frame. However, given the low SD of the contour angle (1.9°) this is a fairly good approximation.
Possible errors in our estimation of the whisker motion may come from errors in the measurement of the whisker base, or from vertical components of whisker motion which cannot be measured from above. We estimated the error in whisker angle that could arise from errors in the whisker base position by manually calculating the whisker angle based on the visually identified whisker base position in a subset of films and comparing to the algorithmically calculated whisker angle. We estimate that the maximum error in whisker position due to uncertainty in the whisker base position was no more than 4°. It is more difficult to quantify errors due to changes in the vertical orientation of the head. However we note that, qualitatively, during cricket hunting shrews did not tend to rotate or orient vertically very often, except at the moment of strike initiation. We therefore do not think that this would have a significant effect on the results reported here. It is also possible that, like rats, shrew whisking has an inherent dorsal–ventral component (Bermejo et al., 2002). While we did not see strong evidence of this in our videos, we did not track whiskers in the vertical direction and cannot rule out a vertical whisking component.
When the shrew and cricket were in contact we were able to manually block out the cricket from the image in order to estimate the true shrew body contour. While the presence of the tag likely has some effect on whisker motion, we are confident that these are small and do not significantly effect our analyses. Figure A1 in Appendix shows two whiskers’ motion. One was tagged and tracked using our automated algorithms (red trace). The second was a whisker from the adjacent column which was not tagged and was tracked manually (blue trace). As can be seen, the motion of the two is extremely similar. The initial angular offset between the two can be expected due to the fact that they come from different columns.
Head velocity and acceleration were calculated from the tracked head dot position. All head velocity and acceleration values are reported here as magnitudes (i.e., absolute values). Spectrograms of the whisker motion were calculated in windows (0.67, 0.5, or 0.233 s) that were slid along the trial by 3.3 ms. The whisker set angle was calculated by low-pass filtering the whisker position with a cutoff frequency of 2 Hz, using the “filtfilt” function in MATLAB. The average spectral power density of the whisker motion between 12 and 17 Hz was used to compare whisking power during different phases of hunting. All spectra and coherence measurements were calculated using the Chronux toolbox for Matlab. Thomson’s multitaper method was used for spectra estimation with a time-bandwidth parameter of 2 and using the first three tapers (Thomson, 1982; Mitra and Pesaran, 1999).
For comparing shrew and rat whisking, individual protraction and retraction events were defined as continuous movements lasting between 4 and 105° in the same direction. The lower bound for this range was calculated by subtracting 2 SD from the shrew average whisking amplitude. The upper range was calculated by adding 2 SD to the rat average whisking amplitude. This was done in order to span the full range of whisking of both shrews and rats. Rat whisking data was recorded from nine male and two female adult Wistar rats. All rats were greater than 3-months old at the time of recording and were housed two per cage on a reversed 12:12 night–day schedule. Rat whisking was recorded using the same high-speed video, tagging and tracking procedure as described above, however the frame rate for all rat videos was 250 Hz. Rat whisking videos were acquired in a separate set of experiments during which rats performed a searching behavior over an elevated gap. Amplitude of whisking was defined as the difference between the maximum and minimum protraction or retraction angles. Maximum velocity and acceleration were used to compute velocity and acceleration histograms during protraction and retraction.
Paired ranked sum tests were used to compare shrew whisking and head motion parameters before and after the transition from locomotion to searching and for the transition from searching to contact. A paired rank sum test was also used to compare head acceleration before and during attack. Two-tailed Welch’s t-tests were used for all comparisons between rat and shrew whisking. Error bars represent the SE.
All experimental procedures were performed according to German guidelines on animal welfare under the supervision of local ethics committees.
Previous studies have shown that whiskers are important for shrew predation (Anjum et al., 2006; Catania et al., 2008). However, to date, it is unknown whether shrews actively move their whiskers (as in rats), or if information is gathered through passive whisker contact (as in cats). As a first step toward understanding whisker use during shrew predatory behavior, we quantified shrew whisker motion from the time a cricket was introduced into the hunting arena until the time of attack. This comprises multiple types of behavior which we considered advantageous for quantifying the range of whisking amplitudes and frequencies employed by the shrews. We compare this to rat whisker use which is known to be under fine muscle control and include periodic whisking at approximately 8 Hz. Rat whisker motion was recorded in a separate set of experiments during which rats on an elevated platform used their whiskers to identify the location of another rat on a second platform. While these shrew and rat behaviors are not entirely similar, both include searching followed by contact with the desired object (crickets for the shrews and another rat for the rats). We use this data for comparison because it was recorded using the exact same whisker tagging and tracking procedure and, as with the shrew data, is a complex behavior which should include a range of types of whisker use. The average rat whisking peak-to-peak amplitude from our recordings was 54.7° ± 25.4° (mean ± SD). This value is similar to values reported in other studies (Gao et al., 2001; Berg and Kleinfeld, 2003; Jin et al., 2004) and slightly larger than others (Towal and Hartmann, 2008), however should be representative of exploratory whisking in the rat.
Figure 2 compares shrew (top) and rat (bottom) whisking (n = 159 protractions and 171 retractions for rats, and n = 61 protractions and 64 retractions for shrews). The average power spectrum shows a very clear peak in the shrew whisking at 13.8 Hz (Figure 2A, top) which can be compared to the 7.9 Hz peak in the rat power spectrum (Figure 2A, bottom), which is in agreement with the known rat whisking frequency of ∼8 Hz. The insets in Figure 2A show examples of periodic whisker motion by both shrews (top) and rats (bottom). Clearly shrews employ periodic whisking during their hunting behavior and the shrew whiskers are under active muscle control. Compared to rats, shrews had lower amplitude whisking (Figure 2B, defined as the protraction amplitude, p < 0.01). The peak velocity achieved during retraction was slightly lower for shrews than rats (dotted lines, Figure 2C, p < 0.01) although the peak acceleration during both protraction and retraction (Figure 2D) was slightly higher for shrews than rats (p < 0.01 for both). Interestingly, as in rats, retraction velocity was almost double protraction velocity (Figure 2C). In rats, protraction is controlled by a set of intrinsic muscles which move individual whiskers while retraction is controlled by a set of extrinsic muscles which move the whisker pad as a whole (Berg and Kleinfeld, 2003). The similar protraction and retraction dynamics in the shrew suggests that whisking may be under similar muscle control as in rats.
Figure 2. Comparison of shrew (top graphs) and rat whisking (bottom graphs). (A) Average spectral power of shrew (top) versus rat (bottom) whisking. The peak of the shrew whisking spectral power density occurs at 13.8 Hz compared to 7.9 Hz for rats. Insets show example whisking traces. (B) Shrew whisking amplitude (top) is smaller than rat whisking amplitude (bottom), (33.8° ± 15.0° versus 54.7° ± 25.4° respectively, p < 0.01). (C) Shrew and rat protraction velocities (blue bars) were not significantly different (1.662 ± 0.463 × 103 versus 1.717 ± 0.627 × 103 respectively, p = 0.4) however rats retracted their whiskers (red bars) with higher velocity than shrews (3.638 ± 1.301 × 103°/s versus 2.972 × 0.941 × 103°/s, respectively, p < 0.01). Both shrews and rats retracted their whiskers much faster than they were protracted. (D) Both protraction and retraction acceleration were higher in shrews than rats (protraction: 2.581 ± 1.107 × 105°/s2 versus 1.844 ± 0.985 × 105°/s2, respectively, p < 0.01, retraction: 3.665 ± 1.264 × 105°/s2 versus 3.276 ± 1.273 × 105°/s2, respectively, p < 0.01, two-tailed Welch’s t-test, all values are given as the mean ± 1 SD).
From our videos it appeared that whiskers from the more ventral rows in the shrew tended to drag along the ground. This appeared to be passive contact with the floor of the arena however, we cannot rule out the possibility that shrews have control of their whiskers in the vertical direction.
As in rats, shrew whisking on the left and right sides of the snout was largely coherent during exploratory whisking (Figure A2A in Appendix). This left–right symmetry was broken when shrews contacted and followed the wall of the arena with their whiskers (Figures A2B,C in Appendix and Video S5 in Supplementary Material). However we cannot say if this symmetry breaking is due to a change in muscle control or is just due to the force exerted unilaterally by the wall on the whiskers.
Based on head motion and proximity to the cricket we divided the shrew hunting behavior into four distinct phases (Figure 3):
Figure 3. An example hunting scene. (A) Sequence of pictures taken from a hunting scene in the 9 × 9 cm arena. The pictures have been filtered to increase contrast and brightness. In each frame, the names of the phases are given from which the pictures were taken. (B) Whisker motion for a hunting trial, classified according to behavior. Black line is the whisker set angle. Inset is the average power spectrum prior to the attack. (C) Spectrogram of whisker motion. During the search phase there is an increase in power centered at ∼14 Hz. (D) Head displacement from starting position. During the immobile phase, prior to the black dashed line, there is 0 displacement. The onset of motion can be seen most clearly in the expanded view of the first 1.5 s (inset). Search initiation coincides with the initial deviation of the head displacement from 0 at t = 0.8 s. (E) The sudden head acceleration increase can be clearly seen at attack onset following the contact period.
Prior to hunting shrews often showed very little head or whisker movement.
The search phase was characterized by the onset of locomotion while out of contact range with the cricket. An increase in head motion was used as the beginning of the search phase. This phase was followed by either contact with the cricket or another immobile phase.
The first whisker-to-cricket contact defined the transition from the search to the contact phase. This phase was kept very short by the shrew, as crickets tried to escape before the shrew was able to strike.
Attack was defined by a sudden increase in head acceleration directed toward the cricket.
Analysis of the whisker motion during a single hunting trial is shown in Figure 3. Single images from the four phases described above are show in Figure 3A (see also Video S1 in Supplementary Material) and the whisker motion, whisking spectrogram, head displacement and acceleration are shown in Figures 3B–E. In this example there was virtually no whisker motion during the immobile phase (Figures 3B,C first gray shaded region). The beginning of the search phase was determined by an increase in the head displacement (inset, Figure 3D). Concurrent with increased head motion the whisker set angle increased and whisker motion increased (Figures 3B,C). During the search phase there were periods of highly regular periodic whisking which can be seen by the power increase in the 10–20 Hz range in the spectrogram (Figure 3C). The inset in Figure 3B is the average spectral power density prior to attack. On average, whisking occurred at 14.2 Hz. In this example, after contact, the whisking magnitude decreased (Figure 3B, second gray shaded region). The contact phase lasted only briefly before the shrew struck with a sharp increase in head acceleration (Figure 3E).
In order to look at general changes in whisking strategy across the different hunting phases we first compared the average whisker motion during the 0.5 s preceding the beginning of searching to the 0.5 s following the beginning of searching (Figure 4). Here, we averaged a reduced set of whisker traces (n = 10). This was necessary as these were the only traces that included an immobile phase. Whisking power at approximately 14 Hz increased dramatically at the beginning of searching (blue versus red, Figure 4A, p < 0.01, n = 10 trials). The first harmonic of the whisker motion due to the non-sinusoidal nature of the whisking can also be seen at approximately 28 Hz. Concurrent with the increased whisking amplitude, shrews moved their whiskers to a more protracted position, shown by the increase in set angle at the beginning of searching (defined as t = 0, dotted line, Figure 4B, p < 0.01, n = 10 trials). The increase in head acceleration at search onset is shown in Figure 4C. Because all filming was done in the cricket hunting arena, we cannot say whether this increase in whisking is generally associated with locomotion or if it is specific to searching. However we did observe locomotion without whisking which argues that whisking is not required during locomotion and may be more specific to a searching task.
Figure 4. Averaged data on the transition between immobile and search phase. Averages triggered to onset of head motion, shown for 0.5s before and after trigger point (n = 10). Shaded regions represent the mean ± 1 SE. (A) The peak in the spectral power centered at approximately 14 Hz increases upon searching onset. (B) Whisker set angle significantly increases at the onset of searching. (C) The increase in head acceleration coincides with the searching onset. Double asterisks denote significant changes (paired ranked sum test, p < 0.01).
We next analyzed changes in whisker motion upon contact with the cricket (Figure 5). Because the time from first contact to attack was short we compared the whisker motion in shorter time windows of 0.23 s preceding and following whisker contact with the cricket. Contact time was determined by visual inspection of the video. We cannot absolutely rule out the possibility that there were whisker contacts prior to the determined first contact. However, the whiskers were generally clearly visible in the videos and it is safe to assume that the majority of the pre- and post-contact periods are correct. We included only those traces in which greater than 2 s had passed since the last contact, and we excluded any trials that contained an attack within 0.23 s after contact. With these criteria we had 22 contact trials. The searching data presented in this figure was taken from the end of the searching phase, whereas searching data presented in Figure 4 was from the beginning of the searching phase, at search onset. Therefore the searching results are similar but not precisely the same between the two figures. Following contact, whisking amplitude decreased (blue versus red, Figure 5A, p < 0.05, n = 22) and there was a small but non-significant increase in the whisker set angle (Figure 5B, p = 0.11, n = 22). There was also a small increase in the head acceleration magnitude (Figure 5C, p < 0.01, n = 22), indicating that the shrews responded to the whisker contact. It has been shown that rat whisking can become asymmetric after contact with an object (Mitchinson et al., 2007). However we found no change in the average coherence at 14 Hz between the left and right whisker motion after contact with the cricket (coherence = 0.82 ± 0.03 before contact and 0.73 ± 0.06 after, p = 0.38, n = 10 trials (there were 10 contact trials in which both whiskers were tracked).
Figure 5. Averaged data on the transition between search and contact phase. Averages triggered to whisker contact onto cricket, shown for 0.23 s before and after trigger point (n = 22). Shaded regions represent the mean ± 1 SE. (A) The peak in the spectral power centered at approximately 14 Hz decreases after whisker contact. (B) Average whisker set angle does not significantly change after whisker contact. (C) There was a small change in head acceleration magnitude after whisker contact. Single and double asterisks denote significant change (paired ranked sum test, p < 0.05, and <0.01 respectively).
Shrews generally attack by thrusting their heads toward the cricket’s thorax (Anjum et al., 2006). The average time between first contact and initiation of the attack was 179 ± 3.1 ms. However, this includes first whisker contact to the antenna of the cricket. When the shrew was already close enough to make first whisker contact onto the cricket body the time to attack could be as short as 53 ms (smallest measured duration). During the attack phase it appeared that the whiskers always stayed in contact with the cricket and whisker movements were disturbed by this contact. Because of this and due to the extremely fast head motions, we could not reliably track the whisker base position during the attack and therefore we restricted our analysis to the head motion after the beginning of the attack. During the attack head acceleration reached extremely high values (at times greater than 1500 cm/s2) which can be 10–20 times greater than head accelerations experienced during normal exploration. On average, the head acceleration increased from 239.1 ± 22.7 to 625.1 ± 59.4 cm/s2 during the attack and head velocity increased from 7.8 ± 0.8 to 15.2 ± 1.5 cm/s.
Interestingly, we found that shrews were able to react to cricket movements during the short duration of the attack. In Figure 6A (see also Videos S3 and S4 in Supplementary Material) we overlaid video images taken just before and at the end of an attack. The dots and circles show the head positions of the shrew and cricket, respectively, during the attack. The dots and circles are color coded to show simultaneous shrew and cricket head positions (note that the first four cricket head positions are nearly identical). A bright spot on the cricket’s head was used to track the head position manually. In this example the shrew is initially moving upward in the video and the cricket is still. When the cricket suddenly jumps backward the shrew reacts by adjusting its trajectory. In order to estimate the reaction time of the shrew we looked at the time delay between the cricket’s sudden speed increase, corresponding to its attempted flight, and the shrew’s increase in head speed as it adjusts its attack. In this example we found that it took the shrew only 29 ms to react to the cricket’s escape attempt (Figure 6B). We found six examples in which the shrew clearly responds to a sudden motion of the cricket. On average, the shrew’s increased head acceleration followed the cricket’s sudden acceleration by 26.9 ms (Figure 6C). It was previously reported that shrews react to underwater stimuli with a latency on the order of 20 ms, in good agreement with the values reported here (Catania et al., 2008).
Figure 6. Mid-attack change of direction. (A) Still frames from two different time points (time lapse between images = 0.23 s) before and at the end of the attack are overlaid on top of each other. Dots and circles are the head positions of the shrew and cricket, respectively, during the attack. Dots and circles are color coded so that head positions of the shrew and cricket at the same time point can be compared. (B) Head speed of the cricket (top) and shrew (bottom). A 29 ms lapse between the cricket’s speed increase and the shrew’s speed increase. Black dotted lines = 0 cm/s, red-dotted lines represent the thresholds used to determine time of speed increase (3 SD above mean speed preceding the increase). (C) Average of six attacks in which the shrew changed direction in reaction to the cricket. Black dashed lines are linear fits to the baseline acceleration prior to the sudden increase in cricket acceleration (defined as t = 0 ms). Shaded regions represent ± 1 SE. Acceleration was defined to have increased when it went at least one SE above the linear fit to the baseline (red and blue circles). The difference in time between the cricket and shrew acceleration increase was 26.9 ms.
During the final stages of the attack, in all the trials we analyzed, the shrews’ rostrum underwent a remarkable deformation. In order to better visualize this deformation we recorded simultaneously from the top and side under higher magnification and with a higher frame rate of 400 Hz (Figure 1A, right). The simultaneous top and side view was achieved by positioning a mirror at 45° on the side of the cricket, which was immobilized. Figure 7 shows an example of an attack on the cricket’s antenna (traced by the red-dotted line, see also Video S2 in Supplementary Material). At t2 = 50 ms, the shrew tries to bite the antenna of the cricket which is in contact with the shrew’s snout. As the shrew bites, the rostral part of the snout bends down to almost a right angle in what appears to be an attempt to draw the antenna further into its mouth (Figure 7, right). As it bends down the width of the snout increases from 5.6 mm (Figure 7, bottom, black dotted line marked by “x” at t1 = 0 ms) to 6.5 mm during the attack (“x” at t3 = 100 ms). However, in this scene the attack failed, and the antenna sprung loose from the mouth before the bite. This example shows that shrews use their snout similar to an elephant trunk in order to grasp at objects.
Figure 7. Split-screen high-speed videography of rostrum bending during the attack. Top row: side view, bottom row: top view. Left column: Shrew approaches cricket. Middle column: after contact with the cricket’s antenna the shrew attempts to bite. Right column: while trying to bite the antenna the snout is bent dramatically downward (clearly seen in the side view). This appears to be used to draw the antenna further into the shrew’s mouth. During attack the shrew’s snout becomes wider and rounder, increasing in width, x, from x = 5.6 mm at t1 = 0, to x = 6.5 mm at t3 = 100 ms. The cricket’s antenna is outlined in red. Scale tick mark spacing is 1 mm.
Many animals (e.g., rats and mice) actively whisk to gather information about their environment, but little is known about how they achieve this goal during natural behaviors (Brecht et al., 1997; Jin et al., 2004; Mehta et al., 2007). We found that shrews actively whisk while searching for their cricket prey. Shrew whisking frequency was around 14 Hz which lies between typical whisking frequencies for rats and mice. However, shrew whisking amplitude was around 34°, which is smaller than either rats or mice, both of which whisk with amplitudes around 55° (Jin et al., 2004). Shrews may whisk with smaller amplitude due to their extremely small size (shrews weigh approximately 10 times less than mice), which may make larger amplitude whisker movements unnecessary. Also, shrews seem to be well-suited for fitting through very small cracks and slits which probably requires less large-amplitude whisking. However the exact reasons for lower amplitude shrew whisking are unknown.
The focus of this study was to characterize whisking during a natural behavior that is both biologically relevant and demanding with respect to the acquisition of sensory information. We found that, during hunting, the shrews’ behavior could be divided into four distinct phases: immobile, searching, contact, and attack. These divisions were based on shrew head motion as well as proximity and whisker contact with the cricket. In order to better understand whisker use strategies during the complicated hunting task we looked for changes in whisker use across these different phases. We found that shrews did not whisk during an initial immobile phase, but whisked consistently around 14 Hz while searching. Additionally, upon transitioning from the immobile phase to searching, shrews held their whiskers in a more protracted position suggesting that both active whisking and scanning with static, protracted whiskers was important for hunting. Upon whisker contact with the cricket there was a small increase in the head acceleration magnitude in reaction to the contact. Whisking amplitude then decreased while the whiskers remained in the protracted position and may protract slightly more suggesting that whiskers were important in guiding behavior after the cricket was detected. Furthermore, the reduction in whisking amplitude upon contact suggests that periodic whisking was not critical for judging cricket shape and may suggest that the spatial distribution of whisker contacts across the entire pad is important for directing the shrews’ attack. The brief contact period was followed by extremely high head acceleration toward the cricket. As a consequence of their small size shrews are under immense metabolic pressure to obtain food and indeed hunting is very robust, easily elicited and may still continue even after six strikes. A major experimental benefit of the prey-capture paradigm studied here is that we did not have to train the shrews.
Several studies have been performed on shrew behavior (Anjum et al., 2006; Catania et al., 2008). All of these studies have shown that shrews are incredibly fast which is necessary for survival due to the very fast flight reflexes of their prey. Indeed, Camhi et al. (1978) determined the escape reaction time of a cockroach to be 58 ms, and Hoyle (1958) determined the startle response of the grasshopper to be 33 ms. This gives Etruscan shrews a very short time window between the detection and the escape of its prey. Here we showed that the shrews’ reaction time in response to the crickets’ escape attempt was, on average, 26.9 ms, which is comparable to the response times of the cockroach and grasshopper. During attacks, we found that the average head acceleration was 625 cm/s2 and on individual trials could be greater than 1500 cm/s2. This is comparable with the lion’s initial acceleration, which lies around 950 cm/s2 (McNeill, 2002). In this regard, it was surprising to see that shrews were able to react to the cricket while attacking mid-flight. This suggests that shrews have extraordinarily fast tactile sensing capabilities.
The fast movement of the shrew makes it technically challenging to both film and track the head and whisker positions. Moreover, the whiskers of the Etruscan shrew are very thin (<50 μm at the root of the whisker). To compensate for these problems, we marked the head as well as the whisker and made videos with a high frame rate (300–400 Hz). A limitation of this study might be that while marking the head likely does not lead to motion artifacts, the tagging of the whiskers might. To compensate for this possible confound, we developed the technique described above to make the whisker tags as small and light as possible. Visual inspection, and manual tracking of the whiskers showed no apparent differences between tagged and untagged whisker motion (Figure A1 in Appendix).
The shape of the snout did not change during the immobile or search phases. However during all of the attacks that were filmed, the shape of the shrews’ snout became wider as seen from above. Filming from a sagittal perspective revealed that this happens when shrews open their jaw and bend their snout. One could imagine that a widening of the snout helps tactile guidance during the attack (e.g., by increasing the distance between whisker base positions) moreover the shrew must integrate the change in snout shape during whisker use. Finally, we observed that shrews use their flexible snouts to grasp at their prey during the attack. This observation may have important implications for our understanding of the shrews’ hunting strategies.
The authors declare that the research was conducted in the absence of any commercial or financial relationships that could be construed as a potential conflict of interest.
We thank C. Roth-Alpermann and R. Naumann for useful discussion during manuscript preparation, and R. Grant for comments on the manuscript. This work was supported by Neurocure, the Bernstein Center for Computational Neuroscience (BMBF) and Humboldt University, the SFB 665, the EU Biotact-grant, and the Neuro-behavior ERC grant.
Anjum, F., Turni, H., Mulder, P. G., van der Burg, J., and Brecht, M. (2006). Tactile guidance of prey capture in Etruscan shrews. Proc. Natl. Acad. Sci. U.S.A. 103, 16544–16549.
Berg, R. W., and Kleinfeld, D. (2003). Rhythmic whisking by rat: retraction as well as protraction of the vibrissae is under active muscular control. J. Neurophysiol. 89, 104–117.
Bermejo, R., Vyas, A., and Zeigler, H. P. (2002). Topography of rodent whisking – I. Two-dimensional monitoring of whisker movements. Somatosens. Mot. Res. 19, 341–346.
Brecht, M., Preilowski, B., and Merzenich, M. M. (1997). Functional architecture of the mystacial vibrissae. Behav. Brain Res. 84, 81–97.
Camhi, J. M., Tom, W., and Volman, S. (1978). The escape behavior of the cockroach Periplaneta americana. J. Comp. Physiol. A 128, 203–212.
Catania, K. C. (2000). Cortical organization in insectivora: the parallel evolution of the sensory periphery and the brain. Brain Behav. Evol. 55, 311–321.
Catania, K. C., Hare, J. F., and Campbell, K. L. (2008). Water shrews detect movement, shape, and smell to find prey underwater. Proc. Natl. Acad. Sci. U.S.A. 105, 571–576.
Diamond, M. E., von Heimendahl, M., Knutsen, P. M., Kleinfeld, D., and Ahissar, E. (2008). ‘Where’ and ‘what’ in the whisker sensorimotor system. Nat. Rev. Neurosci. 9, 601–612.
Gao, P., Bermejo, R., and Zeigler, H. P. (2001). Whisker deafferentation and rodent whisking patterns: behavioral evidence for a central pattern generator. J. Neurosci. 21, 5374–5380.
Grant, R. A., Mitchinson, B., Fox, C. W., and Prescott, T. J. (2009). Active touch sensing in the rat: anticipatory and regulatory control of whisker movements during surface exploration. J. Neurophysiol. 101, 862–874.
Hill, D. N., Bermejo, R., Zeigler, H. P., and Kleinfeld, D. (2008). Biomechanics of the vibrissa motor plant in rat: rhythmic whisking consists of triphasic neuromuscular activity. J. Neurosci. 28, 3438–3455.
Jin, T. E., Witzemann, V., and Brecht, M. (2004). Fiber types of the intrinsic whisker muscle and whisking behavior. J. Neurosci. 24, 3386–3393.
Lee, K. J., and Woolsey, T. A. (1975). A proportional relationship between peripheral innervation density and cortical neuron number in the somatosensory system of the mouse. Brain Res. 99, 349–353.
Mehta, S. B., Whitmer, D., Figueroa, R., Williams, B. A., and Kleinfeld, D. (2007). Active spatial perception in the vibrissa scanning sensorimotor system. PLoS Biol. 5, e15. doi: 10.1371/journal.pbio.0050015.
Meyer, M. E., and Meyer, M. E. (1992). The effects of bilateral and unilateral vibrissotomy on behavior within aquatic and terrestrial environments. Physiol. Behav. 51, 877–880.
Mitchinson, B., Martin, C. J., Grant, R. A., and Prescott, T. J. (2007). Feedback control in active sensing: rat exploratory whisking is modulated by environmental contact. Proc. Biol. Sci. 274, 1035–1041.
Mitra, P. P., and Pesaran, B. (1999). Analysis of dynamic brain imaging data. Biophys. J. 76, 691–708.
Roth-Alpermann, C., Anjum, F., Naumann, R., and Brecht, M. (2010). Cortical organization in the Etruscan Shrew (Suncus etruscus). J. Neurophysiol. 104, 2389–2406.
Sokolov, V. E., and Kulikov, V. F. (1987). The structure and function of the vibrissal apparatus in some rodents. Mammalia 51, 125–138.
Towal, R. B., and Hartmann, M. J. (2008). Variability in velocity profiles during free-air whisking behavior of unrestrained rats. J. Neurophysiol. 100, 740–752.
Vincent, S. B. (1912). The function of the vibrissae in the behaviour of the white rat. Behav. Monographs 1, 7–81.
Wineski, L. E. (1985). Facial morphology and vibrissal movement in the golden hamster. J. Morphol. 183, 199–217.
Keywords: active touch, whisker, Etruscan shrew, barrel cortex, prey capture
Citation: Munz M, Brecht M and Wolfe J (2010) Active touch during shrew prey capture. Front. Behav. Neurosci. 4:191. doi: 10.3389/fnbeh.2010.00191
Received: 23 April 2010;
Accepted: 13 December 2010;
Published online: 29 December 2010.
Edited by:
Denise Manahan-Vaughan, Ruhr University Bochum, GermanyReviewed by:
Ehud Ahissar, Weizmann Institute of Science, IsraelCopyright: © 2010 Munz, Brecht and Wolfe. This is an open-access article subject to an exclusive license agreement between the authors and the Frontiers Research Foundation, which permits unrestricted use, distribution, and reproduction in any medium, provided the original authors and source are credited.
*Correspondence: Michael Brecht, Bernstein Center for Computational Neuroscience, Humboldt University of Berlin, Philippstr. 13, Haus 6, 10115 Berlin, Germany. e-mail:bWljaGFlbC5icmVjaHRAYmNjbi1iZXJsaW4uZGU=
Disclaimer: All claims expressed in this article are solely those of the authors and do not necessarily represent those of their affiliated organizations, or those of the publisher, the editors and the reviewers. Any product that may be evaluated in this article or claim that may be made by its manufacturer is not guaranteed or endorsed by the publisher.
Research integrity at Frontiers
Learn more about the work of our research integrity team to safeguard the quality of each article we publish.