- 1School of Environmental Sciences, University of Guelph, Guelph, ON, Canada
- 2Laboratoire de Zoologie, Research Institute of Biosciences, University of Mons, Mons, Belgium
- 3Biological Evolution and Ecology Research Unit, Université Libre de Bruxelles, Brussels, Belgium
Evidence is widespread that many species of Bombus are in population and biogeographical decline in response to adverse effects of global climate warming. The complex interactions of the mechanisms at the root of the declines are poorly understood. Among the numerous factors, we posit that heat stress in the nests could play a key role in the decline of bumblebee species. The similarity of the optimum temperature range in incubating nests is remarkable, about 28–32 °C regardless of species from the cold High Arctic to tropical environments indicates that the optimal temperature for rearing of brood in Bombus spp. is a characteristic common to bumblebees (perhaps a synapomorphy) and with limited evolutionary plasticity. We do note that higher brood rearing temperature for the boreal and Arctic species that have been tested is stressfully high when compared with that for B. terrestris. The Thermal Neutral Zone (TNZ), temperatures over which metabolic expenditure is minimal to maintain uniform nest temperatures, has not been studied in Bombus and may differ between species and biogeographic conditions. That heat stress is more serious than chilling is illustrated by the Thermal Performance Curve Relationship (TPC) (also sometimes considered as a Thermal Tolerance Relationship). The TPC indicates that development and activity increase more slowly as conditions become warmer until reaching a plateau of the range of temperatures over which rates of activity do not change markedly. After that, activity rates decline rapidly, and death ensues. The TPC has not been studied in eusocial bees except Apis dorsata but may differ between species and biogeographic conditions. The importance of the TPC and the TNZ indicates that environmental temperatures in and around bumblebee nests (which have been rarely studied especially in the contexts of nest architecture and substrate thermal characteristics) are factors central to understanding the adverse effects of heat stress and climatic warming on bumblebee populations, health, and biogeographical decline.
1 Introduction
Bumblebees are eusocial, large and conspicuous insects. More than 250 species are described throughout the world (Williams, 1998), but in some respects they are remarkably uniform in form and behaviour. Their colonies can be considered individually to be single “superorganisms” (i.e., a group of synergetically interacting organisms of the same species) because the entire colony, rather than the individual resident bees, is the reproductive unit subject to Darwinian selection. An important feature of the “superorganism” concept is the ability of the whole colony to maintain its temperature within a range over which metabolic rates are minimal and activity can be maintained. That range is the Thermal Neutral Zone (TNZ) (Figure 1) and was elucidated by Moritz and Southwick (1992) for the western honeybee (Apis mellifera). Thermal stress has been indicated to result in workers of smaller size and poorer condition through accelerated development and more energy devoted to respiration during development. Similarly, the reproductives, especially the gynes, may mature at a smaller size with concomitant adverse effects on overwintering success, spring founding of new colonies, fewer eggs in the ovaries, and overall reduced colony strength (see Vanderplanck et al., 2019).
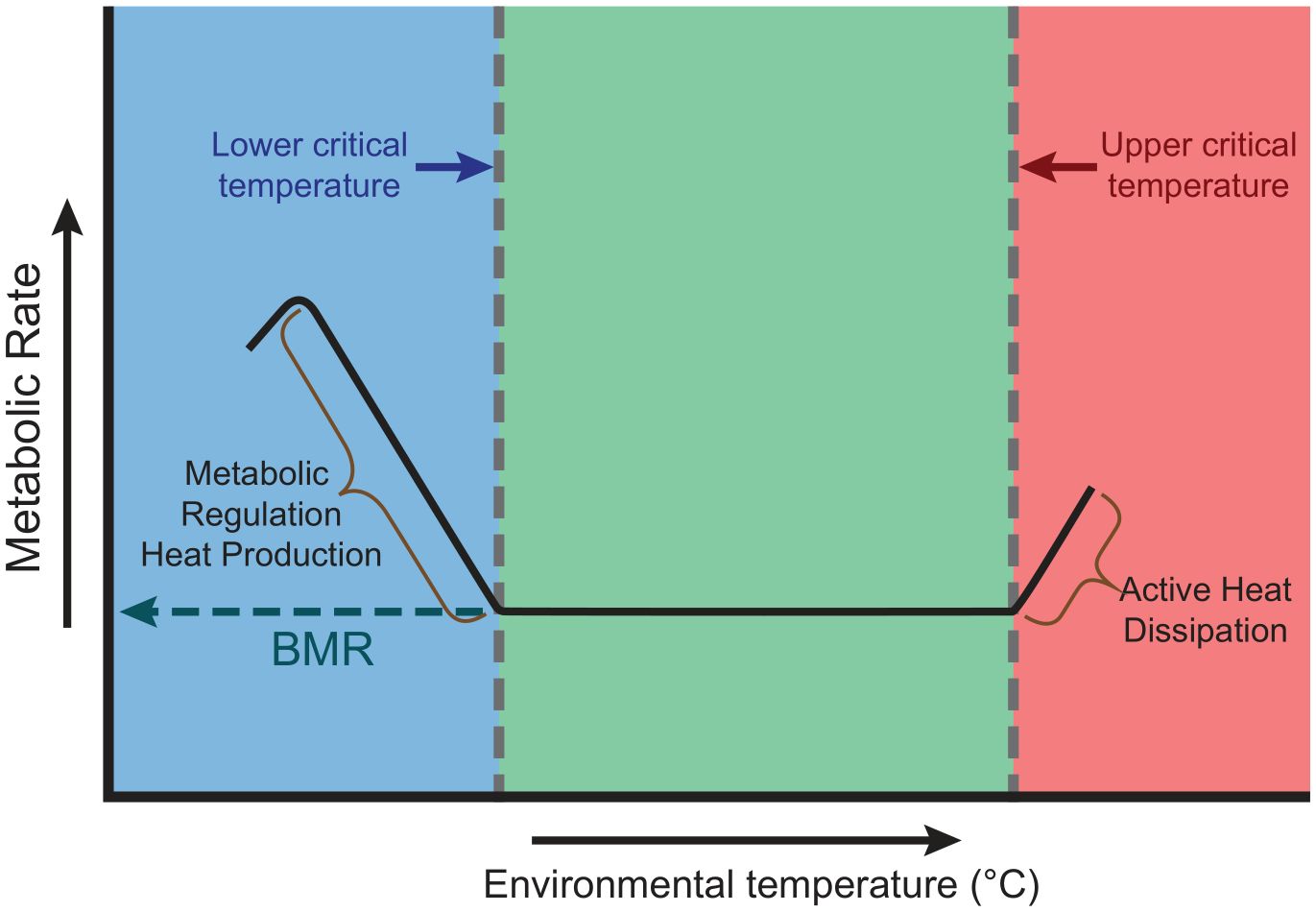
Figure 1 Generalised relationship between rate of performance (i.e. activity) and environmental temperature in animals, notably in those with capacity to thermoregulate as in the nests of eusocial insects (from Moritz and Southwick, 1992). As conditions become colder (in blue), thermoregulation requires more and more metabolic energy (e.g., through vibration of thoracic muscles) until cold torpor and then death set in (BMR). The Thermal Neutral Zone (TNZ) (in green) represents temperature conditions under which the metabolic rate required to thermoregulate remains minimal. If heat stress occurs (in red), the metabolic cost of thermoregulation for cooling increases (e.g., by fanning, evaporation, mass defecation, etc.) until thermoregulation becomes impossible and death ensues. In general, the slopes of metabolic and thermal stress are longer and shallower for warming than for cooling (i.e. overheating is more metabolically stressful than chilling) (see Figure 2). The TNZ has not been studied in Bombus and may differ between species and biogeographic conditions.
Bumblebees are recognised as important pollinators from the High Arctic to the tropics and are exploited in commercial agriculture (Cameron and Sadd, 2019). They form a monophyletic genus, Bombus, that includes the subgenus of socially parasitic Psithyrus, within the Family Apidea (Hymenoptera) (Cameron et al., 2007). Aspects of the bionomics of several species of bumblebees have contributed to their commercial value in pollination of crops (Velthuis and Van Doorn, 2006), assessing natural problems in pollination and associated issues in conservation (IPBES, 2016; Potts et al., 2016).
Bumblebees are widely considered to be in decline globally, with climate change (especially warming) being a major contributor (Vannote and Sweeney, 1980; Williams, 1986; Colla and Packer, 2008; Cameron et al., 2011; Bommarco et al., 2012; Rasmont and Iserbyt, 2012; Sunday et al., 2014; Kerr et al., 2015; Martins et al., 2015; Rasmont et al., 2015; Arbetman et al., 2017; Ogilvie et al., 2017; Woodard, 2017; Koch et al., 2018; Sirois-Delisle and Kerr, 2018; Françoso et al., 2019; Naeem et al., 2019; Vray et al., 2019; da Silva Krechemer and Marchioro, 2020; Jacquemin et al., 2020; Rollin et al., 2020; Soroye et al., 2020; Marshall et al., 2021; Martínez-López et al., 2021; Oyen et al., 2021). In the few examples when the diversity and abundance of bumblebees have been assessed by a standard method over several years, wide interannual fluctuations have not been explained (Turnock et al., 1997). The conclusions from most studies rely on inferences, circumstantial evidence, and correlations without invoking experimentally elucidated root physiological causes, or suggesting a suite of interacting ecological, behavioural to physiological causalities.
We suggest that assessment of the effects of stress at the superorganism level (i.e., colony) would help explain their plight under climate change. Conditions within nesting habitats are a rather neglected but probably important factor under conditions of heat stress, such as during heat waves (Vanderplanck et al., 2019; Martinet et al., 2021; Gradišek et al., 2023), in constraining and adversely affecting the health and survival of bumblebees as the effects of global warming become increasingly severe. The effects of stress may be well measured by whole colony respirometry which seems to have been rarely undertaken. The stress of parasitic tracheal mites adversely affects the respiratory rates of whole colonies of honeybees (Apis mellifera) and helped understand winter mortality (Skinner, 2000). Respirometry of honeybees in clusters held at differing temperatures has helped understand superorganism effects in colony thermoregulation (Moritz and Southwick, 1992; Stabentheiner et al., 2021).
The aim of this report is to stress the importance of nest thermoregulation and brood incubation, and their apparent consistency throughout the genus, as being highly sensitive to disruption, especially under heat stress. Then, by returning to classic understandings of thermal performance, we point out that more and comparative studies on this common character could explain the recorded sensitivities of various Bombus spp. to the effects of climate change.
Coupled with the remarkable uniformity in the nest incubation temperatures across the genus (Table 1), other studies on nesting ecology are needed, such as the ambient heat load (soil temperature), nature of the substrate, architecture of the nests, thermal buffering properties of the substrate, considerations of aspect to insolation, shading, and other environmental factors. The extent of behavioural plasticity is probably greater than noted in published studies but may be constrained in the face of other risks, such as flooding, predation and potential for disease.
2 Uniformity of nest incubation temperatures
Seeley and Heinrich (1981), Heinrich (1993), and Jones and Oldroyd (2007) review what is known about temperature regulation in the nests of various social insects. It is widely known that honeybees [Apis spp. (Hymenoptera: Apidae)] control the environmental conditions in their colonies (Huber, 1814; Milum, 1929; Seeley and Heinrich, 1981; Moritz and Southwick, 1992; Sudarsan et al., 2012; Stabentheiner et al., 2021), but the extent and mechanisms by which other eusocial insects, such as ants (Roeder et al., 2021; Nascimento et al., 2022) and bumblebees [Bombus spp. (Hymenoptera: Apidae)] do so is less understood (Goulson, 2010).
Newport (1837) presented probably the first detailed accounts of the temperatures generated for the incubation of the brood by bumblebees, as later pointed out by Hoffer (1882) as being similar to birds incubating their eggs and chicks. The informative books by Heinrich (1979, 1993, 2004) summarise the scientific information on both cooling and heating in terms of temperature control in individual bumblebees and of the nest.
Table 1 presents information on the species of Bombus for which nest incubation and body temperature data are published. From the first publication by Newport (1837), it has become increasingly accepted that the general optimum temperature within the brood of various species bumblebee is much the same, at about 28–32 °C, regardless of species. That narrow temperature range applies when the colony is well developed, but the brood nest may be much cooler when a lone queen needs to leave her nest to forage at the early phase of nest establishment. In small, queenless, colonies of B. terrestris thermoregulation is also tightly controlled, notably under conditions of cold stress. More recent research (e.g., Vogt, 1986; Weidenmüller et al., 2002; Weidenmüller, 2004; Gardner et al., 2007; Vanderplanck et al., 2019) has stressed behavioural responses, especially fanning, in response to high temperatures, cooling, and preventing the overheating of brood in developed colonies.
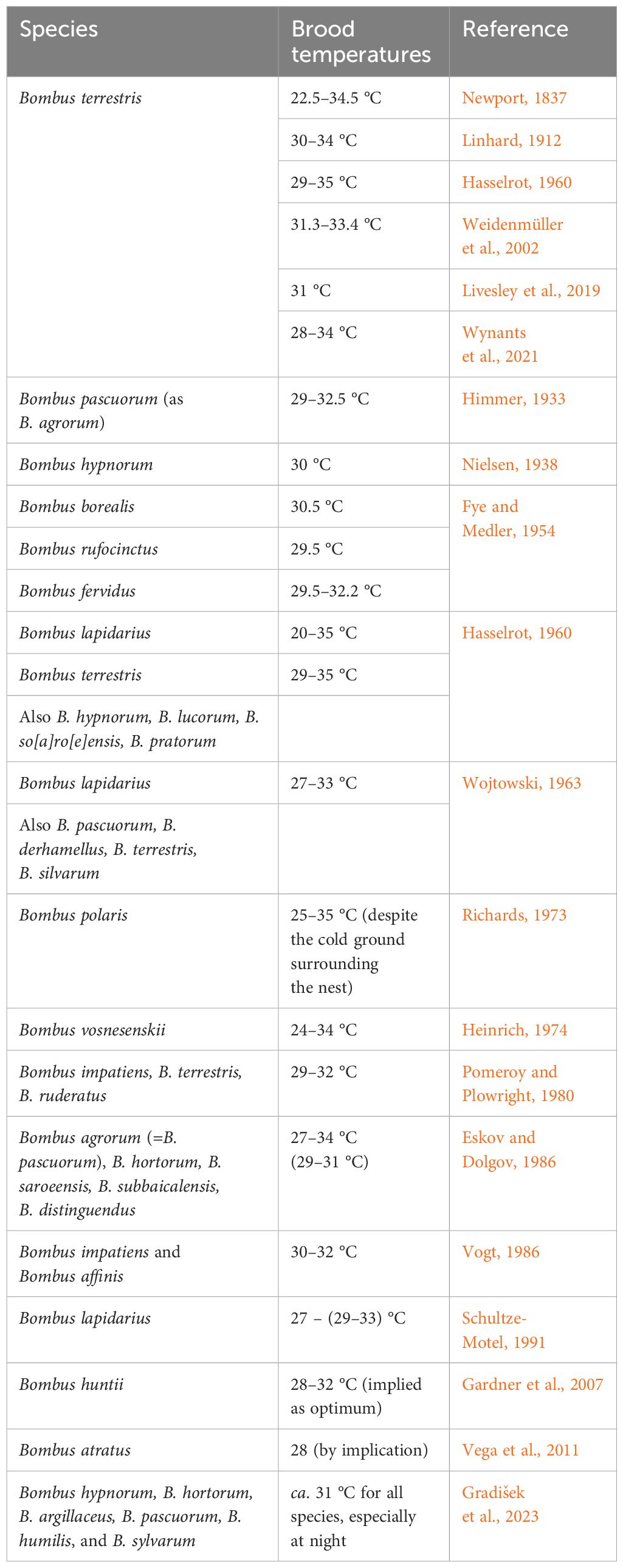
Table 1 Generalised brood temperatures of various species of Bombus arranged primarily in chronological order of studies made.
The temperature regime at the periphery of the cluster and within the cavity occupied by the nest varies depending on the ambient temperature outside the cavity or domicile in which the colony lives. The ambient temperature immediately outside the cavity is, of course, similarly influenced but the surrounding materials (e.g., wood, straw, animal nest residues, soil and so on) and their thermal properties, especially conductance (see below).
Our review of the published literature over the past 180 years indicates that bumblebees of all species so far studied maintain constant brood nest temperatures of 30–35 °C at the core of the colony (Table 1). This range seems to be wider at lower temperatures than for honeybees, however comparative studies have not been made. As with honeybees, the narrow temperature range of incubation and thermoregulation seems not to have been studied in bumblebees in relation to brood survival. It can be assumed that bumblebee brood would not survive at temperatures above 36 °C, and at temperatures at about 30–32 °C the brood survives and develops normally, prolonged chilling can be assumed to be detrimental.
3 Thermal performance & tolerance
In general, temperature affects rates of biological activity (A), from enzymatic reactions, bacterial population growth rates, various metabolic functions, organ development and maturation, to organismal growth and development. Together and in combination temperatures and rates of biological activity (also metabolic rates) constitute the Thermal Performance Curve (TPC) (Schulte et al., 2011; Rezende and Bozinovic, 2019) (Figure 2), sometimes referred to as the Thermal Tolerance Relationship (TTR). TPC is highly diverse across life forms, from organisms that thrive at near freezing temperatures to extreme thermophiles. Nevertheless, for all life forms, TPC may be modelled as the product of two functions, an exponentially increasing rate of the forward reaction to the optimum and a steeper exponential decay (till death) presumably resulting from enzyme denaturation, membrane dysfunction, deceleration of mitochondrial activity as temperatures increase beyond the optimum, and perhaps effects on DNA-methylation. The optimum, where the change in activity remains nil (homeostatic) as temperature increases (dA/dT = 0 for TTR) may be extended by biochemical plasticity, acclimation, thermoregulatory behaviour, and evolutionary adaptation.
In classical ecology it is generally recognised that the ranges of most terrestrial organisms, especially animals, can be explained ecophysiologically by temperature and moisture (Uvarov, 1931; Andrewartha and Birch, 1954; Lutterschmidt and Hutchison, 1997; Kinsolver and Umbanhower, 2018). In particular, the relationship between temperatures for development and survival of all organisms shares the common feature of being negatively skewed (i.e., the TPC, which indicates that once the optimal temperature for development, activity, or survival is exceeded, the decline is steep with death ensuing after a few more degrees of heat) (bacteria: Rezende and Bozinovic, 2019; plants: Rezende and Bozinovic, 2019; fungi: Rezende and Bozinovic, 2019; insects: Uvarov, 1931; Wigglesworth, 1972; Kinsolver and Umbanhower, 2018; Rezende and Bozinovic, 2019; vertebrates: Lutterschmidt and Hutchison, 1997; Rezende and Bozinovic, 2019).
Surprisingly, it seems that few experimental studies have been made to elucidate the thermal performance and tolerances (TT, see discussion below) of bees and their brood together (but see Vogt, 1986; Vanderplanck et al., 2019). It may be assumed that brood temperatures lower than optimal are more tolerable and less damaging over a greater range than temperatures above optimal. That is because temperatures lower than the optimum just slows down metabolism but going higher causes irreversible damage. In general, one may assume that heat stress may be lethal even if slightly elevated above optimum. Himmer (1932) suggested that the vital upper limit for temperature of honeybee brood (Apis mellifera) is 36 °C and Mardan and Kevan (2002) showed that for Apis dorsata brood does not survive when incubated at 36 °C or above. For adult bumblebees it is known that this curve may have different positions on the temperature continuum, with some species able to function at lower or higher temperatures and some species that can acclimate in response to ambient and local conditions (Oyen et al., 2016; Oyen and Dillon, 2018; Oyen et al., 2021; Gonzalez et al., 2022).
Food is the source of energy for thermoregulation and colony maintenance and can be influenced by climate change (e.g., Miller-Struttmann et al., 2015). Vanderplanck et al. (2019) studied the interactive effects of heat stress reduction of dietary resource quality, and colony size in bumblebee colonies. Using 117 colonies of B. terrestris, they applied a fully cross-treatment experiment to test the effects of three dietary quality levels under three levels of heat stress with two colony sizes. Both nutritional and heat stress reduced colony development and caused lower investment in offspring production. Small colonies were much more sensitive to heat and nutritional stresses than were large ones, possibly because a higher proportion of workers are needed to maintain social homeostasis in the former. From a nutritional viewpoint, the effects of heat stress were far less pronounced for small colonies which received relatively good diets than for those that received inferior diets.
4 Bumblebee nest architecture and soil temperature profiles
We suggest that the thermal environments in which bumblebee nest (mostly underground, some in cavities, and few on the surface of the ground) are a neglected area in bumblebee ecology. We have not found any reports that link experimentally the various factors involved.
Bumblebee nests take on many forms but are generally enclosed. The most well-known form is ovoid enclosed in a protective cavity. Those bumblebees using vacated rodent nests may be as much as 20 cm beneath the soil surface (Free and Butler, 1959; Goulson 2010; Martinet et al., 2022). The nest itself includes the central complex of brood cells within an involucre of waxy material adhering to an outer layer of insulation. That insulation may be a centimetre or so thick. Outside the cavity is the substrate in which the nest is enclosed is soil, wood (as in cavity nesters), or rarely vegetation (living and/or dead) for surface nesters (Figure 3) (for examples, see Free and Butler, 1959; Sakagami et al., 1967; Hines et al., 2007; Goulson, 2010; De Meulemeester et al., 2011; Martinet et al., 2022).
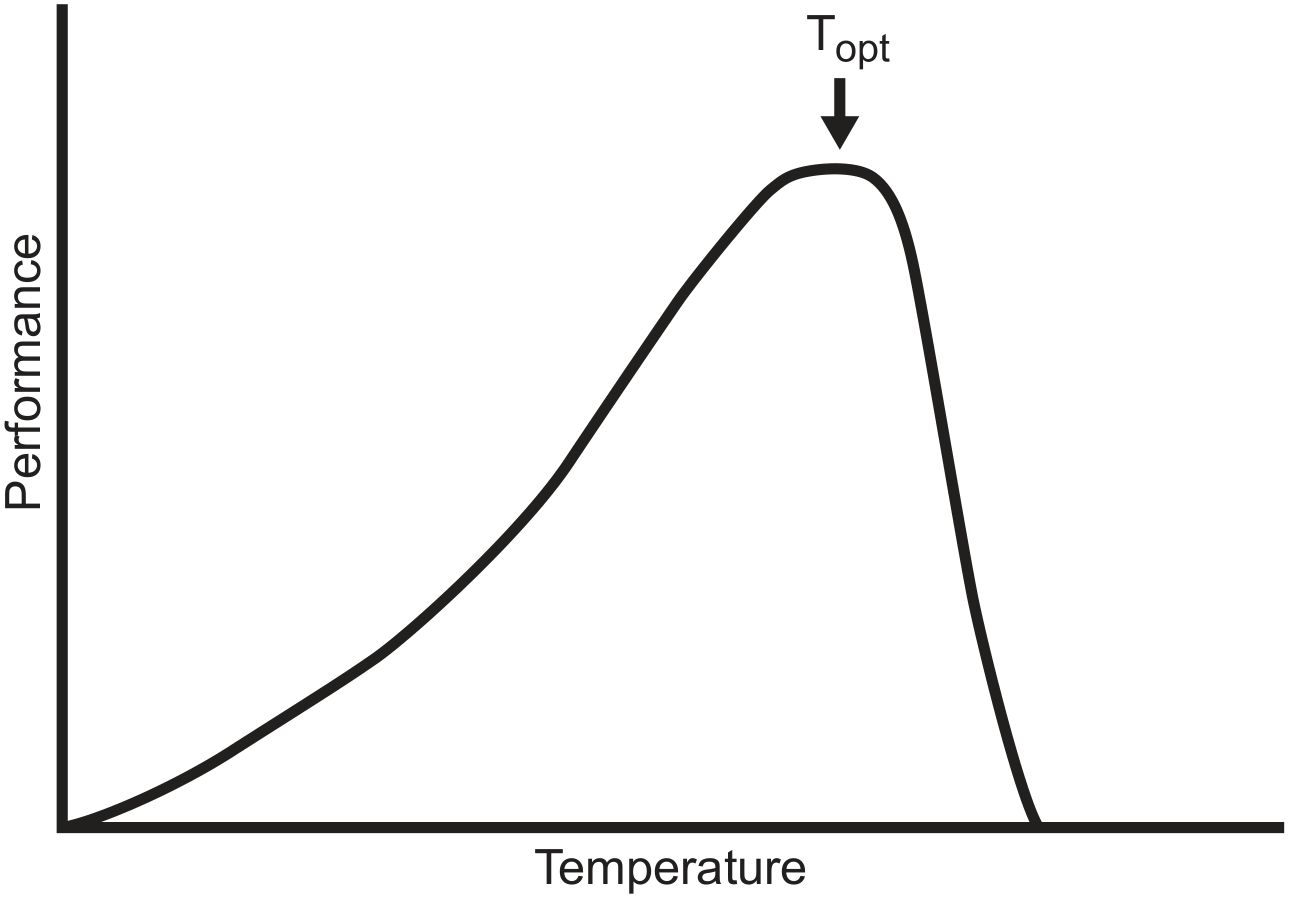
Figure 2 Generalised relationship between temperature and activity (Thermal Performance Curve; TPC) showing the more pronounced impact of heat stress (steep decline in activity after optimal temperatures are exceeded) than of chilling (left-hand tail). The position and breadth of the curve differs between organisms, being transposed to the right for thermophiles and to the left for organisms adapted to cooler conditions. The optimal temperature range, at which activity can be the greatest, may broader or narrower depending on adaptation and/or acclimation. For poikilotherms the relationship is generally broader, especially at cooler temperatures, but for homoeotherms the relationship is tight at across the range of tolerable temperatures as is well-known for incubation and successful development of birds’ eggs. The general form of the relationship is recognised by biologists who have studied temperatures and activity/development from bacteria to mammals (see Rezende and Bozinovic, 2019).
From a thermodynamic viewpoint, nest temperatures are influenced by thermoregulation by the colony (metabolic or behavioural) (Figure 1) (Vogt, 1986). The buffering of the temperature within the colony must be influenced by the insulation afforded by the involucre and insulation; that is, the capacity of the materials together to resist the flow of conductive heat (R- or RSI values and U-units) (see Figure 3). The involucre of the nest is likely to have high insulational value with RSI at about 20 (https://en.wikipedia.org/wiki/R-value_(insulation)) based on data for sheep fleece, straw, etc. Outside the involucre, the soil or wood substrate likely has a much lower RSI at less than half that of the involucre but its conductivity presumably varies widely depending on water content, compaction, and composition. We know of no measurements of RSI values for materials that make up bumblebee nests.
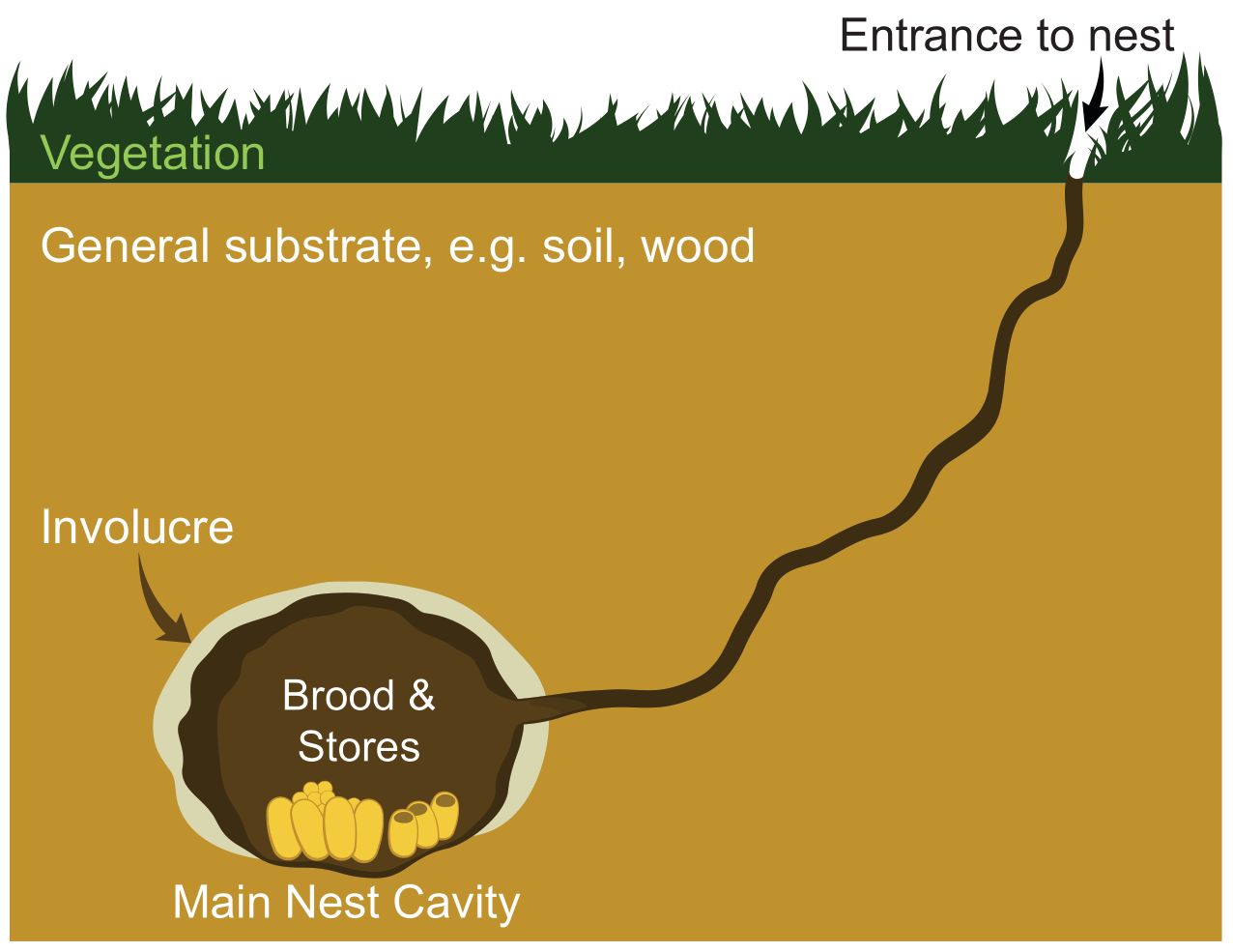
Figure 3 Diagram of the main components of the subterranean nest of Bombus species (see Martinet et al., 2022). Such nests are usually about 15 cm below the surface.
The actual temperature profile from the outside of the nest’s involucre to the surface presumably and typically would show the highest heat loads at the surface, especially if insolated with temperatures exceeding lethal temperatures for many organisms. Under shaded, night-time and rainy conditions the surface temperature of the soil is known to be close to that of the ambient air (Geiger et al., 2003; Holmes et al., 2008). We know of no records in which the soil temperature profile over bumblebee nests is given but Linhard (1912) provided data on ambient temperatures associated with wooden-cavity nests (bird nesting boxes) inhabited by B. hypnorum.
5 Discussion
This essay brings to readers’ attention that the issue of climate change and ensuing range reductions in bumblebees is complex. We hope that our readers recognise that we have attempted to provide a vista of the “Horizon” by presenting this “Frontier” for contemporary “Bee Science”.
Climate change and especially increases in global temperatures seem to affect bumblebees in various and interrelated ways. Anatomical adaptations have been recorded and include changes is size, body part dimensions (notably mouthparts) and possibly pilosity (Miller-Struttmann et al., 2015; but see de Keyzer et al., 2016 and, more recently, Gérard et al., 2018, 2021, 2022a, 2022b). Other adaptive morphological and physiological changes in bee species have been proposed for bees living in urban heat islands (Polidori et al., 2023). Flight activity is influenced by temperature, insolation, and other weather conditions in nest-seeking queens, foraging workers, and the next generation of sexuals. Thermoregulation in nests and incubation of brood are in themselves thermodynamically and behaviourally complex and presumably part of species and colony differences in nest site choice, nest design and construction, and use of materials. The nest differences must also reflect strategies and adaptations for overcoming periods of adversity (e.g., heat, cold, damp, dearth of resources and even hibernation/diapause/metamorphosis).
Excessive heat is clearly a major issue for perhaps most species of bumblebees and it is the factor considered in most detail for the effects of climatic warming. Most contemporary research has focussed on standard meteorological data and coincidental or circumstantial changes in the ranges of various species on bumblebees. Experimental explanations of cause and effect need more detailed and integrated studies with special attention paid to colonies as “superorganisms” (Moritz and Southwick, 1992).
Thermal performance and tolerance (TT) studies in perhaps all organisms show that heat is more hazardous than cold [see Figure 2 (TPC) with its negatively skewed shape]. After a certain ambient temperature is reached, the effects of increasing heat are more pronounced and deadly than are the effects of cooling. The relationship between temperature and larval death has been studies in only a few species of social insects and not in bumblebees. The maximum for the level of activity with temperature can be presumed to reflect a preferred range and the temperature at which social insects, including bumblebees, would try to keep their nests. That range lends to the general relationship (Figures 1, 2) but the variability in that feature seems not to have been assessed in insects, neither physiologically nor in the broader contexts of evolutionary ecology and behaviour. We suggest that such studies, specific and comparative, could elucidate explanatory aspects to climate change and biogeographical range shifts as well as providing insights into possible variability in the TNZ (Figure 1).
The remarkable similarity of nest temperatures recorded from numerous species of bumblebee (Table 1) needs to be seriously considered as a possible limitation on their abilities to withstand not just cool conditions but heat as well. The idea of the TNZ (Figure 1) provides insights as to the possible nature of ecological and evolutionary constraints. Few species have been studied for nesting TNZ. We suggest that various species differ in that respect, with cool-climate species possibly having broader TNZ than others from subtropical and tropic environments. Tropical and subtropical bumblebees may have narrow TNZs reflecting that thermoregulation for cooling is more energetically expensive than in temperate zone species. Thus, the latter with their abilities to live over a broad range of temperatures may represent greater variability in potential survival under relatively limited hot and cold conditions. That aspect of comparative energetics and thermoregulation remains unexplored but may suggest reasons for some changes in bumblebee ranges. The apparent and approximate inverse relationship between TPC (Figure 2) and TNZ (Figure 1) reflects mostly the general shape of the TPC curve with its extended tail at the cold end of the temperature range coupled with the preferred temperature range as maintained by thermoregulatory processes (TNZ, Figure 1).
Thermoregulatory behaviours are various. Air exchange is the most commonly considered reaction to temperature changes in the nests of various bees. The thermodynamics and biophysics of colony clustering within the nest have been elucidate by Sudarsan et al. (2012) for western honeybees (A. mellifera) and those principals likely apply to other cavity nesting social insects. Fanning to expel excess heat from the nest is well known in honeybees and bumblebees. That behaviour can be coupled with the evaporation of water (the swamp cooler effect) within the nesting cavity (e.g. hive) or on the surface of open nests, such as in A. dorsata which indulge in gobetting and mass defecatory flights related to cooling the colony under conditions of heat stress (Mardan and Kevan, 1989). Those behaviours seem not to have been recorded in bumblebees but may be important.
The placement, construction, and architecture, of nests of social bees and wasps have been described to greater or lesser extents for several species but not especially so for thermoregulatory considerations. Subterranean nesting is well-known in bumblebees. They use various substrates and materials, but nests are usually only a few centimetres below the surface (Figure 3). Less seems to be known about the nesting structures of bumblebees that nest in above ground cavities (e.g., hollow trees or bird-nesting boxes). Some may nest above ground in available cavities (even discarded household furniture) or, as the tropical Amazonian B. transversalis which even forages for nest-building thatch materials along its established walking trails (Cameron and Whitfield, 1996). Human beings have domesticated a few species so that they inhabit artificial domiciles (Velthuis and Van Doorn, 2006). Interspecific and intraspecific comparative studies on the nature of the nests and their locations, together with considerations on thermoregulation, may elucidate factors that pertain to physiological ecology and biogeographic limitations. A major problem confronting bumblebee studies is finding nests in the wild. For locating nests of subterranean species, perhaps non-invasive studies by ground-penetrating radar (GPR) could be useful. Sherrod et al. (2019) record nesting burrows of the ground-nesting, solitary bee, Colletes inaequalis at about 0.5 m depth. We know of no published studies using that technique for bumblebees.
We suggest that studies using flow-through respirometry (Skinner, 2000; Stabentheiner et al., 2021) of whole colonies whereby the temperature of the atmosphere, its saturation deficit, and possibly partial pressures of gases can be manipulated would provide more comprehensive and comparative ecophysiological understandings of the TNZ and TPC. Such studies could also involve measurements of the insulation properties of materials from which nests are constructed and of their surrounding matrix. We know of no measurements of heat loss through bee nesting materials. Skowron and Kern (1980) used basic principles of thermodynamics and heat flux to measure the insulation afforded by birds’ nests. Their approach could be followed with modifications possible by modernisation of instrumentation. We suggest that measuring heat flow (i.e., thermal resistance or conductance) across bee nest walls may be instructive and can be done when the temperatures inside and outside are known so as to calculate the temperature difference from inside to the outside of the nest. Then, by measuring the area or volume of the nesting material or matrix being considered and the amount of time taken for the temperature difference to stabilise the insulational properties of the nest can be measured (R-value or U-value for heat transmittance). We are not aware of any such measurements for materials comprising bumblebee nests, either artificial or natural. Calculation could be adjusted to account for the thickness and nature of the wall materials. Readers are referred to Çengel and Afshin (2015) and Tritt (2004) for principles and methods.
By linking the various ecophysiological and behavioural factors, with environmental concerns and constraints on evolutionary plasticity through the superorganism approach to bumblebee nesting, several novel approaches suggest themselves for understanding how climate change asserts its influence on bumblebees’ population dynamics, changing biogeography, and possibly conservation. We suggest that the same principles should be applied to the effects of climate change on other eusocial and other insects (e.g., ants, termites, other Hymenoptera, community gall-formers, etc.).
Author contributions
PK: Writing – original draft, Writing – review & editing. PR: Writing – original draft, Writing – review & editing. BM: Writing – original draft, Writing – review & editing.
Funding
The author(s) declare financial support was received for the research, authorship, and/or publication of this article. PK acknowledges funding from the Natural Sciences and Engineering Research Council (NSERC Individual Discovery Grant RGPIN-2018-0482) has been used to finance the reported research. It is also part of the Accelerating Green Plant Innovation for Environmental and Economic Benefit Cluster funded by the Canadian Ornamental Horticulture Alliance (COHA-ACHO) and by the Government of Canada under the Canadian Agricultural Partnership’s AgriScience Program. BM is supported by the Belgian National Fund for Scientific Research (FRS-FNRS; postdoctoral fellowship).
Acknowledgments
We thank Dr. T. Woodcock for critical review of the manuscript.
Conflict of interest
The authors declare that the research was conducted in the absence of any commercial or financial relationships that could be construed as a potential conflict of interest.
Publisher’s note
All claims expressed in this article are solely those of the authors and do not necessarily represent those of their affiliated organizations, or those of the publisher, the editors and the reviewers. Any product that may be evaluated in this article, or claim that may be made by its manufacturer, is not guaranteed or endorsed by the publisher.
References
Andrewartha H. G., Birch L. C. (1954). The distribution and abundance of animals. Chicago, IL: University of Chicago Press.
Arbetman M. P., Gleiser G., Morales C. L., Williams P., Aizen M. A. (2017). Global decline of bumblebees is phylogenetically structured and inversely related to species range size and pathogen. Proc. R. Soc. B-Biological Sci. 284, 20170204. doi: 10.1098/rspb.2017.0204
Bommarco R., Lundin O., Smith H. G., Rundlof M. (2012). Drastic historic shifts in bumble-bee community composition in Sweden. Proc. R. Soc. B-Biological Sci. 279, 309–315. doi: 10.1098/rspb.2011.0647
Cameron S. A., Hines H. M., Williams P. H. (2007). A comprehensive phylogeny of the bumble bees (Bombus). Biol. J. Linn. Soc. 91, 161–188. doi: 10.1111/j.1095-8312.2007.00784.x
Cameron S. A., Lozier J. D., Strange J. P., Koch J. B., Cordes N., Solter L. F., et al. (2011). Patterns of widespread decline in North American bumble bees. Proc. Natl. Acad. Sci. United States America 108, 662–667. doi: 10.1073/pnas.1014743108
Cameron S. A., Sadd B. M. (2019). Global trends in bumble bee health. Annu. Rev. Entomology 65, 209–232. doi: 10.1146/annurev-ento-011118-111847
Cameron S. A., Whitfield J. B. (1996). Use of walking trails by bees. Nature 379, 125. doi: 10.1038/379125a0
Çengel Y. A., Afshin J. G. (2015). Heat and mass transfer: fundamentals & applications. 5th edition. New York, NY: McGraw-Hill Education, 10121.
Colla S. R., Packer L. (2008). Evidence for decline in eastern North American bumblebees (Hymenoptera: Apidae), with special focus on Bombus affinis Cresson. Biodiversity Conserv. 17, 1379–1391. doi: 10.1007/s10531-008-9340-5
da Silva Krechemer K. F., Marchioro C. A. (2020). Past, present, and future distributions of bumble bees in South America: identifying priority species and areas for conservation. J. Appl. Ecol. 57, 1829–1839. doi: 10.1111/1365-2664.13650
de Keyzer C. W., Colla S. R., Kent C. F., Rafferty N. E., Richardson L. L., Thomson J. D. (2016). Delving deeper: questioning the decline of long-tongued bumble bees, long-tubed flowers and their mutualisms with climate change. J. Pollination Ecol. 18, 36–42. doi: 10.26786/1920-7603(2016)15
De Meulemeester T., Aytekin A. M., Cameron S., Rasmont P. (2011). Nest architecture and species status of the bumble bee Bombus (Mendacibombus) shaposhnikovi (Hymenoptera: Apidae: Bombini). Apidologie 42, 301–306. doi: 10.1007/s13592-011-0022-z
Eskov E. K., Dolgov L. A. (1986). Temperature regulation in the nest and its importance in the bumblebee family life. Zoologicheskii Zhurnal 65, 1500–1507.
Françoso E., Zuntini A. R., Arias M. C. (2019). Combining phylogeography and future climate change for conservation of Bombus morio and B. pauloensis (Hymenoptera: Apidae). J. Insect Conserv. 23, 63–73. doi: 10.1007/s10841-018-0114-4
Fye R. E., Medler J. T. (1954). Temperature studies in bumblebee domiciles. J. Economic Entomology 47, 847–852. doi: 10.1093/jee/47.5.847
Gardner K. E., Foster R. L., O’Donnell S. (2007). Experimental analysis of worker division of labor in bumblebee nest thermoregulation (Bombus huntii, Hymenoptera: Apidae). Behav. Ecol. Sociobiology. 61, 783–792. doi: 10.1007/s00265-006-0309-7
Geiger R., Aron R. H., Todhunter P. (2003). The climate near the ground (various editions). New York: Springer Science & Business Media. (2012). Lanham, MD: Rowman and Littlefield.
Gérard M., Michez D., Debat V., Fullgrabe L., Meeus I., Piot N., et al (2018). Stressful conditions reveal decrease in size, modification of shape but relatively stable asymmetry in bumblebee wings. Sci Rep. 8, 15169. doi: 10.1038/s41598-018-33429-4
Gérard M., Marshall L., Martinet B., Michez D. (2021). Impact of landscape fragmentation and climate change on body size variation of bumblebees during the last century. Ecography 44, 255–264. doi: 10.1111/ecog.05310
Gérard M., Amiri A., Cariou B., Baird E. (2022a). Short-term exposure to heatwave-like temperatures affects learning and memory in bumblebees. Glob. Change Biol 28, 4251–4259. doi: 10.1111/gcb.16196
Gérard M., Cariou B., Henrion M., Descamps C., Baird E. (2022b). Exposure to elevated temperature during development affects bumblebee foraging behavior. Behav. Ecol. 33, 816–824. doi: 10.1093/beheco/arac045
Gonzalez V. H., Oyen K., Ávila O., Ospina R. (2022). Thermal limits of Africanized honey bees are influenced by temperature ramping rate but not by other experimental conditions. J. Thermal Biol. 110, 103369. doi: 10.1016/j.jtherbio.2022.103369
Goulson D. (2010). Bumblebees: behaviour, ecology, and conservation. 2nd edition. Oxford, UK: Oxford University Press.
Gradišek A., Bizjak J., Popovski A., Grad J. (2023). Bumble bee nest thermoregulation: a field study. J. Apicultural Res. 62, 634–642. doi: 10.1080/00218839.2022.2164651
Hasselrot T. B. (1960). Studies on Swedish bumblebees (genus Bombus Latr.): their domestication and biology. Opusc. Entomol. Suppl. 17, 1–192.
Heinrich B. (1974). Thermoregulation in bumblebees. I. Brood incubation by Bombus vosnesenskii queens. J. Comp. Physiol. 88, 129–140. doi: 10.1007/BF00695404
Heinrich B. (1993). The hot-blooded insects: strategies and mechanisms of thermoregulation. Cambridge, MA: Harvard University Press.
Heinrich B. (2004). Bumblebee economics: with a new preface. Cambridge, MA: Harvard University Press.
Himmer A. (1932). Die Temperaturverhältnisse bei den sozialen Hymenopteren. Biol. Rev. 7, 224–253. doi: 10.1111/j.1469-185X.1962.tb01042.x
Hines H. M., Cameron S. A., Deans A. R. (2007). Nest architecture and foraging behavior in Bombus pullatus (Hymenoptera: Apidae), with comparisons to other tropical bumble bees. J. Kansas Entomological Soc. 80, 1–15. doi: 10.2317/0022-8567(2007)80[1:NAAFBI]2.0.CO;2
Holmes T. R. H., Owe M., De Jeu R. A. M., Kooi H. (2008). Estimating the soil temperature profile from a single depth observation: a simple empirical heatflow solution. Water Resour. Res. 44, W02412. doi: 10.1029/2007WR005994
Huber F. (1814). New observations upon bees. (Various editions and translations of this book or books exists: version used is 1926, translated from French by C. P. Dadant. Am. Bee Journal Hamilton Illinois II, 183–198.)
IPBES (2016). The assessment report of the intergovernmental science-policy platform on biodiversity and ecosystem services on pollinators, pollination and food production. Bonn, Germany: Secretariat of the Intergovernmental Science-Policy Platform on Biodiversity and Ecosystem Services. Available at: https://www.ipbes.net/sites/default/files/downloads/pdf/individual_chapters_pollination_20170305.pdf.
Jacquemin F., Violle C., Munoz F., Mahy G., Rasmont P., Roberts S. P., et al. (2020). Loss of pollinator specialization revealed by historical opportunistic data: insights from network-based analysis. PLoS One 15, e0235890. doi: 10.1371/journal.pone.0235890
Jones J. C., Oldroyd B. P. (2007). Nest thermoregulation in social insects. Adv. Insect Physiol. 33, 153–191. doi: 10.1016/S0065-2806(06)33003-2
Kerr J. T., Pindar A., Galpern P., Packer L., Potts S. G., Roberts S. M., et al. (2015). Climate change impacts on bumblebees converge across continents. Science 349, 177–180. doi: 10.1126/science.aaa7031
Kinsolver J. G., Umbanhower J. (2018). The analysis and interpretation of critical temperatures. J. Exp. Biol. 221, jeb167858. doi: 10.1242/jeb.167858
Koch J. B., Vandame R., Mérida-Rivas J., Sagot P., Strange J. (2018). Quaternary climate instability is correlated with patterns of population genetic variability in Bombus huntii. Ecol. Evol. 8, 7849–7864. doi: 10.1002/ece3.42949
Linhard E. (1912). “Humlebien som Husdyr. Spredte Træk af nogle danske Humlebiarters Biologi,” in Tidsskrift for Landbrugets Planteavl, vol. 19. (Organ for Statens Forsøgsvirksomhed i Plantekultur. Udgivet af Statens Planteavlsudvalg), 335–352.
Livesley J. S., Constable C., Rawlinson W. G., Robotham A. M., Wright C., Hampshire A. E., et al. (2019). The power and efficiency of brood incubation in queenless microcolonies of bumble bees (Bombus terrestris L.). Ecol. Entomol. doi: 10.1111/een.12736
Lutterschmidt W. I., Hutchison V. H. (1997). The critical thermal maximum: history and critique. Can. J. Zoology 75, 1561–1574. doi: 10.1139/z97-783
Mardan M., Kevan P. G. (2002). Critical temperatures for survival of brood and adult workers of the giant honeybee, Apis dorsata (Hymenoptera : Apidae). Apidologie 33, 295–301. doi: 10.1051/apido:2002017
Marshall L., Beckers V., Vray S., Rasmont P., Vereecken N. J., Dendoncker N. (2021). High thematic resolution land use change models refine biodiversity scenarios: a case study with Belgian bumblebees. J. Biogeography 48, 345–358. doi: 10.1111/jbi.14000
Martinet B., Dellicour S., Ghisbain G., Przybyla K., Zambra E., Lecocq T., et al. (2021). Global effects of extreme temperatures on wild bumblebees. Conserv. Biol. 1507–1518. doi: 10.1111/cobi.13685
Martinet B., Przybyla K., Atkins J., Bosiger Y., Evrard D., Gill P., et al. (2022). Description of nest architecture and ecological notes on the bumblebee Bombus (Pyrobombus) lapponicus (Hymenoptera: Apidae: Bombini). Insectes Sociaux 69, 131–135. doi: 10.1007/s00040-022-00849-5
Martínez-López O., Koch J. B., Martínez-Morales M. A., Navarrete-Gutiérrez D., Enríquez E., Vandame R. (2021). Reduction in the potential distribution of bumble bees (Apidae: Bombus) in Mesoamerica under different climate change scenarios: conservation implications. Global Change Biol. 27, 1772–1787. doi: 10.1111/gcb.15559.2015
Martins A. C., Silva D. P., De Marco P., Melo G. A. R. (2015). Species conservation under future climate change: the case of Bombus bellicosus, a potentially threatened South American bumblebee species. J. Insect Conserv. 19, 33–43. doi: 10.1007/s10841-014-9740-7
Miller-Struttmann N. E., Geib J. C., Franklin J. D., Kevan P. G., Holdo R. M., Ebert-May D., et al. (2015). Functional mismatch in a bumble bee pollination mutualism under climate change. Science 349, 1541–1544. doi: 10.1126/science.aab0868
Milum V. G. (1929). Brood rearing temperature and variations in developmental periods of the honey bee. Annu. Rep. Illinois State Beekeepers’ Assoc. 29, 72–95.
Moritz R. F. A., Southwick E. E. (1992). Bees as superorganisms: an evolutionary reality. Berlin etc: Springer-Verlag.
Naeem M., Liu M., Huang J., Ding G., Potapov G., Jung C., et al. (2019). Vulnerability of East Asian bumblebee species to future climate and land cover changes. Agriculture Ecosyst. Environ. 277, 11–20. doi: 10.1016/j.agee.2019.03.002
Nascimento G., Câmara T., Arnan X. (2022). Critical thermal limits in ants and their implications under climate change. Biol. Rev. 97, 1287–1305. doi: 10.1111/brv.12843
Newport G. (1837). On the temperature of insects, and its connexion with the functions of respiration and circulation in this class of invertebrated animals. Philos. Trans. R. Soc. London 127, 259–2338. doi: 10.1098/rstl.1837.0019
Nielsen E. T. (1938). Temperatures in a nest of Bombus hypnorum L. Videnskabelige. Meddelelser Naturhistorisk Forening København Naturhistorisk 102, 1–6.
Ogilvie J. E., Griffin S. R., Gezon Z. J., Inouye B. D., Underwood N., Inouye D. W., et al. (2017). Interannual bumble bee abundance is driven by indirect climate effects on floral resource phenology. Ecol. Lett. 20, 1507–1515. doi: 10.1111/ele.12854
Oyen K. J., Dillon M. E. (2018). Critical thermal limits of bumblebees (Bombus impatiens) are marked by stereotypical behaviors and are unchanged by acclimation, age or feeding status. J. Exp. Biol. 221, jeb165589. doi: 10.1242/jeb.165589
Oyen K. J., Giri S., Dillon M. E. (2016). Altitudinal variation in bumble bee (Bombus) critical thermal limits. J. Therm. Biol. 59, 52–57. doi: 10.1016/j.jtherbio.2016.04.015
Oyen K. J., Jardine L. E., Parsons Z. M., Herndon J. D., Strange J. P., Lozier J. D., et al. (2021). Body mass and sex, not local climate, drive differences in chill coma recovery times in common garden reared bumble bees. J. Comp. Physiol. B 191, 843–854. doi: 10.1007/s00360-021-01385-7
Polidori C., Ferrari A., Ronchetti F., Tommasi N., Nalini E. (2023). Warming up through buildings and roads: what we know and should know about the Urban Heat Island effect on bees. Front. Bee Sci. 1, 1269600. doi: 10.3389/frbee.2023.1269600
Pomeroy N., Plowright R. C. (1980). Maintenance of bumble bee colonies in observation hives (Hymenoptera, Apidae). Can. Entomologist 112, 321–326. doi: 10.4039/Ent112321-3
Potts S. G., Imperatriz-Fonseca V., Ngo H. T., Aizen M. A., Biesmeijer J. C., Breeze T. D., et al. (2016). Safeguarding pollinators and their values to human well-being. Nature 540, 220–229. doi: 10.1038/nature20588
Rasmont P., Franzén M., Lecocq T., Harpke A., Roberts S., Biesmeijer J. C., et al. (2015). Climatic risk and distribution atlas of European bumblebees. Sofia, Bulgaria: Pensoft Publishers, 48, 275–280. doi: 10.3897/biorisk.10.4749
Rasmont P., Iserbyt S. (2012). The bumblebee scarcity syndrome: are heat waves leading to local extinctions of bumblebees (Hymenoptera: Apidae: Bombus)? Annales la Societé Entomologique France 48, 275–280. doi: 10.1080/00379271.2012.10697776
Rezende E. L., Bozinovic F. (2019). Thermal performance across levels of biological organization. Philos. Trans. R. Soc. B 374, 20180549. doi: 10.1098/rstb.2018.0549
Richards K. W. (1973). Biology of Bombus polaris Curtis and B. hyperboreus Schönherr at Lake Hazen, Northwest Territories (Hymenoptera: Bombini). Quaestiones Entomologicae 9, 115–157.
Roeder K. A., Roeder D. V., Bujan J. (2021). Ant thermal tolerance: a review of methods, hypotheses, and sources of variation. Ann. Entomological Soc. America 114, 459–469. doi: 10.1093/aesa/saab018
Rollin O., Vray S., Dendoncker N., Michez D., Dufrêne M., Rasmont P. (2020). Drastic shifts in the Belgian bumblebee community over the last century. Biodiversity Conserv. 29, 2553–2573. doi: 10.1007/s10531-020-01988-6
Sakagami S. F., Akahira Y., Zucchi R. (1967). Nest architecture and brood development in a neotropical bumblebee, Bombus atratus. Insectes sociaux 14, 389–413. doi: 10.1007/BF02223686
Schulte P. M., Healy T. M., Fangue N. A. (2011). Thermal performance curves, phenotypic plasticity, and the time scales of temperature exposure. Integr. Comp. Biol. 51, 691–702. doi: 10.1093/icb/icr097
Schultze-Motel P. (1991). Heat loss and thermoregulation in a nest of the bumblebee Bombus lapidarius (Hymenoptera, Apidae). Thermochimica Acta 193, 57–66. doi: 10.1016/0040-6031(91)80174-H
Seeley T., Heinrich B. (1981). “Regulation of temperature in the nests of social insects,” in Insect Thermoregulation. Ed. Heinrich B. (New York: J. Wiley-Interscience Publication, John Wiley & Sons), 159–234.
Sherrod L., Sauck W., Simpson E., Werkema D., Swiontek J. (2019). Case histories of GPR for animal burrows mapping and geometry. J. Environ. Eng. Geophysics. 24, 1–17. doi: 10.2113/JEEG24.1.1
Sirois-Delisle C., Kerr J. T. (2018). Climate change-driven range losses among bumblebee species are poised to accelerate. Sci. Rep. 8, 14464. doi: 10.1038/s41598-018-32665-y
Skinner A. J. (2000). Impacts of tracheal mites (Acarapis woodii (Rennie)) on respiration and thermoregulation of overwintering honeybees in a temperate climate. Ontario, Canada: University of Guelph.
Skowron C., Kern M. (1980). The insulation in nests of selected North American songbirds. Auk 97, 816–824. doi: 10.1093/auk/97.4.816
Soroye P., Newbold T., Kerr J. (2020). Climate change contributes to widespread declines among bumble bees across continents. Science 367, 685–688. doi: 10.1126/science.aax8591
Stabentheiner A., Kovac H., Mandl M., Kafer. H. (2021). Coping with the cold and fighting the heat: thermal homeostasis of a superorganism, the honeybee colony. J. Comp. Physiol. A 207, 337–351. doi: 10.1007/s00359-021-01464-8
Sudarsan R., Thompson C., Kevan P. G., Eberl H. J. (2012). Flow currents and ventilation in Langstroth beehives due to brood thermoregulation efforts of honeybees. J. Theor. Biol. 295, 168–193. doi: 10.1016/j.jtbi.2011.11.007
Sunday J. M., Bates A. E., Kearney M. R., Colwell R. K., Dulvy N. K., Longino J. T., et al. (2014). Thermal-safety margins and the necessity of thermoregulatory behavior across latitude and elevation. Proc. Natl. Acad. Sci. U.S.A 111, 5610–5615. doi: 10.1073/pnas.1316145111
Tritt T. M. (Ed.) (2004). Thermal conductivity: theory, properties, and applications. Berlin, Germany: Springer Science & Business Media.
Turnock W. J., Kevan P. G., Lavertyy T. M., Dumouchel L. (1997). Abundance and species of bumble bees (Hymenoptera: Apoidea: Bombinae) in fields of canola, Brassica rapa L., in Manitoba: an 8–year record. J. Entomol. Soc. Ont. 137, 31–40.
Uvarov B. P. (1931). “Insects and climate,” in Transactions of the Royal Entomological Society of London 79, 1–232.
Vanderplanck M., Martinet B., Carvalheiro L. G., Rasmont P., Barraud A., Renaudeau C., et al. (2019). Ensuring access to high-quality resources reduces the impacts of heat stress on bees. Sci. Rep. 9, 1–10. doi: 10.1038/s41598-019-49025-z
Vannote R. L., Sweeney B. W. (1980). Geographic analysis of thermal equilibria: a conceptual model for evaluating the effect of natural and modified thermal regimes on aquatic insect communities. Am. Nat. 115, 667–695. doi: 10.1086/283591
Vega L., Torres A., Hoffmann W., Lamprecht I. (2011). Thermal investigations associated with the behaviour patterns of resting workers of Bombus atratus (Hymenoptera: Apidae). J. Thermal Anal. Calorimetry 104, 233–237. doi: 10.1007/s10973-011-1373-4
Velthuis H. H., Van Doorn A. (2006). A century of advances in bumblebee domestication and the economic and environmental aspects of its commercialization for pollination. Apidologie 37, 421–451. doi: 10.1051/apido:2006019
Vogt D. F. (1986). Thermoregulation in bumblebee colonies. I. Thermoregulatory versus brood-maintenance behaviors during acute changes in ambient temperature. Physiol. Zoology 59, 55–59. doi: 10.1086/physzool.59.1.30156090
Vray S., Rollin O., Rasmont P., Dufrêne M., Michez D., Dendoncker N. (2019). A century of local changes in bumblebee communities and landscape composition in Belgium. J. Insect Conserv. 23, 489–501. doi: 10.1007/s10841-019-00139-9
Weidenmüller A. (2004). The control of nest climate in bumblebee (Bombus terrestris) colonies: individual variability and self reinforecement in fanning response. Behav. Ecol. 15, 120–128. doi: 10.1093/beheco/arg101
Weidenmüller A., Kleineidam C., Tautz J. (2002). Collective control of nest climate parameters in bumblebee colonies. Anim. Behav. 63, 1065–1071. doi: 10.1006/anbe.2002.3020
Williams P. H. (1986). Environmental change and the distributions of British bumble bees (Bombus Latr.). Bee World 67, 50–61. doi: 10.1080/0005772X.1986.11098871
Williams P. H. (1998). An annotated checklist of bumble bees with an analysis of patterns of description (Hymenoptera: Apidae, Bombini). Bull. Nat. Hist. Mus. Lond. (Ent.) 67, 79–152.
Wojtowski F. (1963). Studies on heat and water economy in bumble-bee nests (Bombinae). Zoologicae Poloniae 13, 19–36.
Woodard S. H. (2017). Bumble bee ecophysiology: integrating the changing environment and the organism. Curr. Opin. Insect Sci. 22, 101–108. doi: 10.1016/j.cois.2017.06.001
Keywords: thermoregulation, Thermal Neutral Zone, thermal performance, thermal tolerance, nest architecture, incubation, nest insulation, diet
Citation: Kevan PG, Rasmont P and Martinet B (2024) Thermodynamics, thermal performance and climate change: temperature regimes for bumblebee (Bombus spp.) colonies as examples of superorganisms. Front. Bee Sci. 2:1351616. doi: 10.3389/frbee.2024.1351616
Received: 06 December 2023; Accepted: 06 March 2024;
Published: 03 May 2024.
Edited by:
Franco Mutinelli, Experimental Zooprophylactic Institute of the Venezie (IZSVe), ItalyReviewed by:
Jiandong An, Chinese Academy of Agricultural Sciences, ChinaEduardo E. Zattara, National University of Comahue, Argentina
Copyright © 2024 Kevan, Rasmont and Martinet. This is an open-access article distributed under the terms of the Creative Commons Attribution License (CC BY). The use, distribution or reproduction in other forums is permitted, provided the original author(s) and the copyright owner(s) are credited and that the original publication in this journal is cited, in accordance with accepted academic practice. No use, distribution or reproduction is permitted which does not comply with these terms.
*Correspondence: Peter G. Kevan, cGtldmFuQHVvZ3VlbHBoLmNh