- School of Biological Sciences, Victoria University of Wellington, Wellington, New Zealand
The global beekeeping industry faces an escalating challenge in the form of Varroa destructor. Synthetic chemicals serve as a cornerstone for varroa management, although they face a major challenge in the form of acaricide resistance. Here, I examine acaricide resistance in varroa under the framework of Insecticide Resistance Management (IRM). I assess the potential of diverse IRM strategies, such as pesticide rotation and mixtures, refuge utilization, synergists and the integration of non-persistent chemicals. The peculiar life history of varroa, characterized by its incestuous breeding system, challenges conventional IRM strategies. There is little published evidence that pesticide rotation is beneficial for resistance management in varroa, with several studies showing resistance is maintained despite rotation. Fitness costs associated with pesticide resistance are often an essential component for IRM strategies, but there are no current data from varroa demonstrating such specific fitness costs (e.g., a reduced relative oviposition rate) associated with resistance. The single published experimental study directly examining relative fitness found that here was little or no reproductive fitness cost associated with pyrethroid resistance. More work is needed on fitness effects of the key acaricides, which would better guide the use of rotation and refuge strategies. A key prospect for future work that has been identified through simulation modeling is offered by pesticide mixtures and the role of synergists to elevate acaricide efficacy. Additional tools for varroa IRM include ‘soft’ acaricides, including oxalic acid, and biopesticides such as dsRNA. In light of the widespread prevalence of acaricide resistance and an increasing varroa problem, there is an urgent need for nuanced, data-driven varroa IRM strategies.
1 Introduction
Varroa destructor, along with the associated Deformed wing virus (DWV) and other viruses associated with the mite, are key pests that are resulting in an ongoing decline in honey bee health around the globe (Rosenkranz et al., 2010; Traynor et al., 2020; Jack and Ellis, 2021; De Jong and Lester, 2023). Varroa was first reported on Apis mellifera outside its natural distribution area of Southeast Asia in 1949, spreading quickly to reach Europe in the 1970s, and then rapidly to North America, South America, Africa, and the Asia Pacific region (Nazzi and Le Conte, 2016). In 2022 it was first detected in Australia, which has been the last major landmass previously free of the parasite and DWV (Chapman et al., 2023). In many of these countries including New Zealand, for example, the combined effects of this parasite and virus have been consistently identified as the leading cause of overwintering honey bee hive mortality (Stahlmann-Brown et al., 2022; McGruddy et al., 2023). This parasite now fits the description of a ‘superpest’, or a species that has the capacity to invade and adapt to environments around the globe, causing significant damage, and evading control strategies (Whitfield and Rotenberg, 2023).
Throughout its global distribution, synthetic chemicals are and will likely continue to be an essential tool for beekeepers in managing varroa. Currently, only a small number of these acaricides are widely used for varroa control: pyrethroids (such as tau-fluvalinate and flumethrin), the organophosphate coumaphos, and the formamidine amitraz. Their ease of use and historical efficacy have led to a significant global reliance on these products (Mitton et al., 2022). A recent survey indicated that 23% of beekeepers in New Zealand used only amitraz or flumethrin, while another 31% used these two pesticides in rotation (Stahlmann-Brown et al., 2022). Similarly, in the UK, 18% of beekeepers depended solely on amitraz, and 20% utilized a rotation or mixed-method approach to mite treatments (Valentine and Martin, 2023). High concentrations of these chemicals or their metabolites are commonly found in bees, honey, wax, and other components of the hive (Mullin et al., 2010; Kiljanek et al., 2017; Vegh et al., 2023).
Pesticide resistance can be defined as a relative decrease in the susceptibility of a pest population to a particular pesticide (Figure 1). Due to the demonstrated ability of many other mite species to rapidly develop resistance to pesticides, it was considered inevitable that varroa would also become resistant (Martin, 2004). Resistance to the pyrethroid tau-fluvalinate was first observed in 1991 and spread quickly (Lodesani et al., 1995). For instance, in the UK, it was initially discovered in 2001 but had already spread over many hundreds of kilometres by 2004 (Martin, 2004). Similarly, resistance was first observed for amitraz in 1991, flumethrin in 1995, and coumaphos in 2001, and has since become globally widespread (Mitton et al., 2022). The development of resistance has historically been classified as mechanisms of decreased exposure (pharmacokinetic) such as through behavioral modification, increased metabolism, sequestration, and excretion, or mechanisms of decreased sensitivity (pharmacodynamic) that include target-site insensitivity (Van Leeuwen and Dermauw, 2016). Pyrethroid resistance in varroa has commonly been associated with amino acid changes in the voltage gated sodium channel (VGSC), although esterase activity has also been speculated to play a role (Mitton et al., 2022). Amitraz resistance has been correlated with the mutations in the receptor for the neuromodulator octopamine (Rinkevich et al., 2023). Coumaphos resistance has been linked to varroa down-regulating the expression of a P450 monooxygenase enzyme on the Greek island of Andros (Vlogiannitis et al., 2021b). It is likely that varroa have developed a diversity of resistance mechanisms that are only beginning to emerge, as mites have an array of gene families that can confer resistance and which are not well studied (Van Leeuwen and Dermauw, 2016).
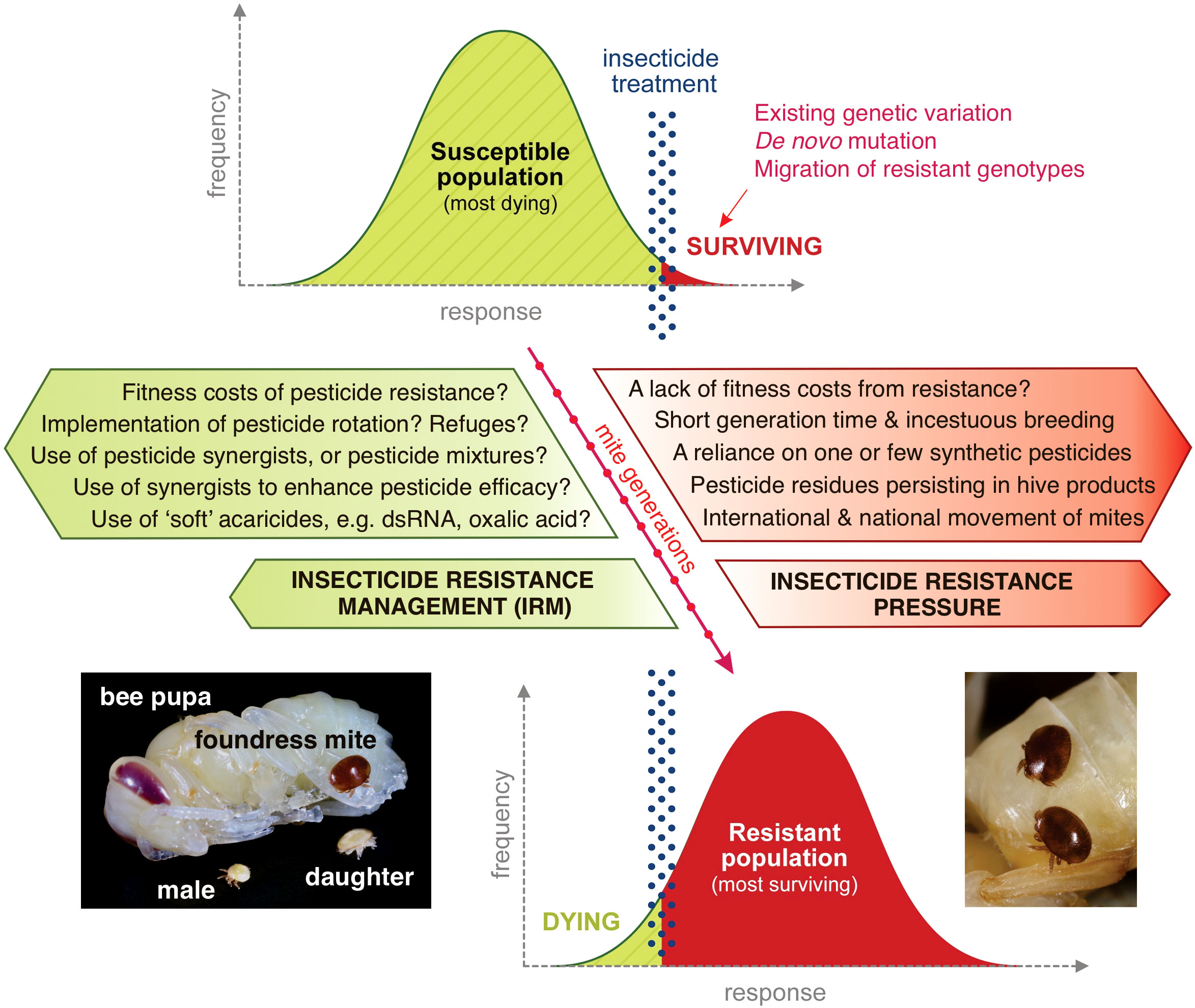
Figure 1 The development and factors affecting the selection of insecticide resistance in varroa. Pest populations are initially susceptible to synthetic chemicals. Resistance builds in these pests under selection pressure from pesticides over many generations. The factors favoring the selection of resistance are shown in red. The factors potentially impairing the selection of resistance and supporting IRM are shown in green. There is currently little published evidence to support the factors that could benefit resistance management: more research on these topics is needed. Adapted from Dusfour et al. (2019). Photographs are by Phil Lester.
The primary objectives of Insecticide Resistance Management (IRM) are to prevent the evolution or emergence of resistance in susceptible pest populations, slow down the development of resistance, or reverse it to a level that allows for the effective and efficient use of existing or new insecticides, all while minimizing adverse environmental impacts (Denholm and Rowland, 1992; Dusfour et al., 2019). IRM has been widely recognized as essential for maintaining effective pest control across various systems, including insect vectors of malaria and arboviruses (WHO, 2012; Mnzava et al., 2015; Dusfour et al., 2019). Georghiou (1994) categorized IRM approaches into three main groups: management through multiple attack, management through saturation, and management through moderation. Management through multiple attack involves the application of multi-directional selection pressures, often achieved by using pesticide mixtures or rotating unrelated insecticides. Management through saturation seeks to eliminate the selective advantage of resistant individuals, which can be accomplished by increasing insecticide uptake through attractants or by suppressing detoxification enzymes with synergists. Management through moderation focuses on reducing selection pressure while supplementing control with non-chemical measures (Georghiou, 1994).
To ensure that synthetic chemicals remain a viable tool for varroa control in beekeeping, the global honey bee industry must align its management and research efforts with the objectives of IRM. Our goals should include maintaining or extending the effectiveness of acaricides while minimizing their environmental and non-target impacts. Additionally, we should strive to integrate acaricides more effectively into holistic varroa control strategies. This article aims to examine varroa mite management via acaricides within the framework of IRM. I explore the key factors that could influence IRM in the context of varroa control, assess the utility of foundational IRM components like pesticide rotation, and identify the research needs required to enhance future varroa IRM. Below, I address these issues using the IRM framework proposed by Georghiou (1994).
2 Varroa management by multiple attack
Management by multiple attack involves the application of multi-directional selection pressures, including by the use of pesticide mixtures or rotations of unrelated insecticides that have different modes of action (Georghiou, 1994). This strategy operates on the premise that control can be achieved through the influence of multiple, independent stressors, which may encompass the use of insecticides. Each stressor imposes a selection pressure below the threshold required for the development and sustenance of resistance. It is an approach that can include the application of chemicals in rotations or in mixtures.
The rotation of acaricides with different modes of action has widely been considered an essential strategy for preventing or delaying resistance development in varroa (Jack and Ellis, 2021; Mitton et al., 2022; Morfin et al., 2022; Warner et al., 2024). In a simulation analysis, Sudo et al. (2018) illustrated how rotation represents the optimal strategy for IRM in many scenarios, particularly when pesticide efficacy is high in the presence of pre-mating selection and dispersal. Pesticide rotation, therefore, remains an indispensable tool for managing many pest species. However, the analysis by Sudo et al. (2018) also identified scenarios in which no known pesticide-use strategy could delay resistance development. An absence of conditions such as pre-mating dispersal and existing high levels of pesticide resistance rendered resistance management strategies unavailable for certain pest species, including the mite Tetranychus urticae (Sudo et al., 2018). Indeed, pesticide rotation has been shown to be ineffective for resistance management in this spider mite (Overmeer et al., 1975). Varroa shares several life-history attributes that theoretically limit the efficacy of pesticide rotation, and the literature contains several examples where rotation failed as a resistance management strategy. Maggi et al. (2011) documented coumaphos resistance in apiaries in Uruguay despite strict acaricide rotation. Subsequently, coumaphos resistance persisted in these apiaries even after 9 years of non-use (Mitton et al., 2018). Similarly, in Spanish apiaries, coumaphos resistance endured for at least 5 years in the absence of this pesticides use (Higes et al., 2020). It is possible that resistance was maintained in the absence of coumaphos. It is also possible that resistance was maintained due to selection pressure via coumaphos residues from contaminated beeswax or other hive matrices. Or alternatively, immigration of resistant mites may have contributed to this outcome. Genetic and dispersal data would be needed to discriminate between mechanisms of mite drift or genetic factors for resistance maintenance.
Despite simulation modeling approaches that have indicated resistance is predicted to be maintained in varroa even in the absence of pesticide use, there are some observations that pesticide resistance can be reduced when the use of a synthetic chemical has been halted. Milani (1999) cite unpublished observations that the proportion of varroa resistant to fluvalinate showed a slow decrease after the use of this pesticide was halted. In published work from this Italian region, they describe the decline of pyrethroid resistance to take many generations (Milani and Della Vedova, 2002). It was predicted that pesticide rotation could be useful but only if the pyrethroid was used once every 4-6 years and only then if it was promptly followed by an alternative treatment to eliminate as many remaining pyrethroid-resistant mites (Milani and Della Vedova, 2002). Of note from this work was that the authors considered toxicological parameters such the LD50 to be of limited use for resistance management because of co-occurring combinations of susceptible and resistant mites (Milani and Della Vedova, 2002). To my knowledge, there is only one other publication reporting an instance of varroa undergoing a reversion from a resistant to a susceptible population through pesticide rotation management. Elzen & Westervelt (2004) describe a ‘modest’ reversion over a relatively short 10-month period after discontinuing the use of fluvalinate. While it is possible that susceptible mite immigration contributed to these results, the authors considered it unlikely (Elzen and Westervelt, 2004).
Much more common in the scientific literature are reports of rotation being infective and which question the usefulness of this strategy for resistance management (Alissandrakis et al., 2017; Mitton et al., 2018; Higes et al., 2020).
For a pest population to revert from pesticide-resistant to susceptible genotypes, there needs to be a significant evolutionary fitness cost (Kliot and Ghanim, 2012). There is, however, limited experimental evidence specifically demonstrating any relative fitness effects for varroa. Relative fitness estimates need to compare both the survival and reproductive performance of susceptible and resistant genotypes (Orr, 2009). Martin et al. (2002) is the only published study that I am aware of to examine egg-laying and daughter mite production in a pyrethroid-resistant and susceptible population of mites. They found little or no reproductive fitness cost associated with pyrethroid resistance in varroa from Texas, which was concluded to be likely if the point mutations associated with resistance cause little or no metabolic cost to the mite (Martin et al., 2002).
An absence of relative fitness cost in resistant individuals would not be unique. Other pest species, including a blowfly and a flour beetle, have developed heritable modifiers that appear to have eliminated fitness costs and have resulted in resistance traits becoming fixed in pest populations (Kliot and Ghanim, 2012). Some authors have suggested point mutations in varroa can induce major fitness costs, though have not measured any life history parameters (González-Cabrera et al., 2018). Another example was a report of no disadvantage or only a small but consistent reduction in the fitness of pyrethroid-resistant mites in Italy (Martin, 2004), although the authors of the cited Italian study did not directly measure reproduction or fitness parameters (Milani and Della Vedova, 2002). A more comprehensive understanding of the selection pressure and fitness effects exerted by different pesticides on varroa is needed.
It is clear that pesticide rotation does not always work for resistance management in varroa. A more nuanced analysis is needed for this management approach (Rosenkranz et al., 2010; Mitton et al., 2021; Mitton et al., 2022). The “mainly non-professional structure of the beekeepers’ community” in many countries or regions has implied rotation may have only ever been a short-term solution for resistance management (Rosenkranz et al., 2010). Nevertheless, the few reports of resistance reversion and decline offer some hope for ongoing varroa management through the rotation of synthetic chemicals. These observations should be a top priority for further investigation: can we transform these exceptions into the norm? Have resistant genes genuinely disappeared from the population, or do resistance reservoirs persist? What are the fitness implications of various mutations? How quickly will resistance re-emerge when synthetic chemicals are reintroduced into a rotation schedule, whether through reservoirs or re-invasion? It is crucial to disentangle the impacts of resistance through the two potential mechanisms of genetic maintenance within populations and reinvasion. What is the ideal pesticide rotation schedule to maintain pesticide efficacy? A rotation schedule that involves using a pesticide only once every 4-6 years (Milani and Della Vedova, 2002) probably isn’t practical for a beekeepers toolbox. Other researchers have reached the same conclusion: there is an urgent need for a more sophisticated assessment of rotation as a resistance management tool, and how it might be best integrated varroa control approaches (Mitton et al., 2021).
A further complicating aspect to achieve rotation is that relatively high concentrations of acaricides or their metabolites persist in honey, wax, and other components of the hive (Mullin et al., 2010; Kiljanek et al., 2017; Vegh et al., 2023). Beekeepers often recycle and reuse wax and hive components from hives for many years. Varroa may thus be being exposed to low concentrations of different acaricides even when under rotation. This accumulation and management of miticides in beeswax is considered to be a widespread problem of increasing importance for resistance management (Le Conte et al., 2010).
An approach that has received little attention in the context of varroa IRM is the use of pesticide mixtures. Simulations, however, suggest that mixtures can be extremely beneficial. Helps et al. (2020) developed a model to explore the factors influencing optimal insecticide resistance management strategies. Their model incorporated variables such as fitness effects on the pest, the rotation of different insecticides, and the use of refuges. Their findings revealed that the primary determinant of the optimal strategy was the reproductive strategy of the pest. For pests with a sexual reproductive mode, employing a pesticide mixture emerged as the nearly always optimal strategy for IRM. Interestingly, a reduced application dose mixture often outperformed the label dose mixture, and regardless of the pests reproductive mode, a reduced dose mixture frequently represented the optimal strategy (Helps et al., 2020). There is reason to expect that mixtures might be beneficial for varroa IRM. For example, coumaphos resistance on the Greek the island of Andros has been linked to varroa down-regulating the activation of a P450 monooxygenase enzyme (Vlogiannitis et al., 2021b). Perhaps this resistance could be negated or exploited by using an alternative or a mixture of pesticides. Vlogiannitis et al. (2021b) discuss their results in regard to IRM, citing other instances of how the gene expression in the P450 pathway of other pests confers resistance to one pesticide but can increase the susceptibility to chemicals. Such negative cross-resistance between different insecticide or pro-insecticides caused by differential gene regulation in resistant insects could be exploited for varroa IRM via a push–pull strategy (Vlogiannitis et al., 2021b).
In summary, regarding varroa IRM through multiple attack:
● There is currently no empirical evidence indicating that pesticide resistance imposes a specific relative fitness cost on varroa. There is a pressing need for pesticide-specific, laboratory-based assessments of fitness costs associated with resistance. Such assessments would provide valuable insights into the implementation of various aspects of varroa IRM. It is possible that fitness costs may arise for some pesticides or specific genetic mutations linked to resistance but not for others.
● Pesticide rotation has been frequently suggested a key component of IRM for varroa, although there is limited current evidence that rotation is or could be effective. A simulation model suggests rotation is likely to be ineffective for varroa IRM (but would not be deleterious). There is an urgent need for a nuanced analysis or rotation and how it might be best integrated into varroa IRM strategies.
● Pesticide mixtures are projected to hold potential value for IRM based on simulation models, yet experimental investigations in this area are lacking and warrant further research before implementation can be considered.
3 Varroa management by saturation
Management by saturation requires an elimination of the selective advantage of resistant individuals, perhaps by increasing insecticide uptake through attractants or by suppressing of detoxication enzymes via synergists (Georghiou, 1994).
Semiochemical attractants or lures are used as a component of IRM in a range of pest management systems. The ‘lure and kill’ approach has been proposed as an effective method for pest control and even potential eradication (El-Sayed et al., 2006). These treatments of lure and kill can be further combined with additional pheromone disruption practices (Suckling et al., 2016). Varroa produce or respond to a wide variety of semiochemicals that influence their behavior and physiology (Plettner et al., 2016). Some semiochemicals can deter and repel varroa (Pernal et al., 2005), arrest their movement (Calderone and Lin, 2001), alter their host-selection behavior (Eliash et al., 2014), or even induce gravid adult females to reabsorb eggs (Frey et al., 2013). Despite these observations, no commercially available attractants that lure or attract varroa currently exist. Research in this area is ongoing, with one report of an experimental attractant capable of causing 35-50% of mites to disengage from bees (Suszkiw, 2009). An efficient attractant that could enhance varroa exposure to pesticides would be of significant value for IRM, as would non-pesticide control options such as mating disruption. In addition to ‘lure and kill’ approaches being used directly on the mites, an additional or alternative approach would be to investigate ways to selectively attract varroa-infested bees to acaricide treatments.
There is potential for the suppression of detoxification enzymes through synergists, which could in some situations enhance or sustain the effectiveness of synthetic chemicals in varroa IRM. For example, in pyrethroid-resistant mosquito populations the benefits of using a synergistic pesticide (synergist piperonyl butoxide, or PBO) are thought to be via the inhibition of the cytochrome P450 enzymes that catalyse pyrethroid detoxification (Farnahm, 1998). The degree of pyrethroid enhancement via the PBO synergist was found to be mosquito species-dependent and dependent on the level of pyrethroid resistance in the population (Churcher et al., 2016). PBO was similarly shown to restore pyrethroid efficacy in resistant populations of whitefly and cotton bollworm (Young et al., 2006). Similarly, to chemical synergists, dsRNA can silence P450 genes that confer resistance to pests against plant defenses (Mao et al., 2007). dsRNA can also knock-down host immune responses and augment the virulence of pathogens (Wang et al., 2021; Felden et al., 2023). The synergistic use of biopesticides has also been observed to substantially increase pyrethroid efficacy (Nishimatsu and Jackson, 1998). An important caveat in the use of synergists for varroa, however, is that synergists may only be of benefit in situations where resistance arises from metabolic mechanisms. Alternatively, if the major mechanism is the target site modification, as with the majority of know resistance cases for varroa to date (Mitton et al., 2022), it is unlikely that there will be significant benefits from the use of such compounds.
In summary, regarding varroa IRM by saturation:
● Varroa respond to an array of semiochemicals, which could be useful for IRM if mites could be attracted to pesticide exposure as in ‘lure and kill’ methods. Semiochemical use and development for varroa has been challenging and further research is needed in this area.
● The suppression of detoxication enzymes via synergists is also an under-studied area in varroa IRM, although may be of limited benefit for varroa IRM if the key resistant mechanisms are target site modifications. Results from other IRM systems demonstrate that enhanced efficacy and even the restoration of pesticide efficacy can be achieved by the use of synergists.
● The elimination of the selective advantage of resistant varroa might be achieved in some instances through the use of a wide variety of synergists including other pesticides, biopesticides including dsRNA.
4 Varroa IRM by moderation
Management by moderation acknowledges the value of pesticide-susceptible genes and individuals as a valuable resource. It entails reducing the selection pressure and complementing control efforts with non-chemical measures (Georghiou, 1994). These management actions may involve decreasing insecticide application rates, reducing the frequency of application, using non-persistent chemicals, or preserving susceptible genes and individuals through the utilization of untreated refuge populations.
Lowering or eliminating the selection pressure for a particular pesticide is a crucial component for successful IRM in many pest species. Low rates of application might be useful for IRM with many pests that are yet to develop resistance. Pesticide resistance in varroa, however, has developed and is already widespread (Mitton et al., 2022).
Could lowering rates or infrequent application of pesticides successfully cause a reversion of varroa populations to become dominated by susceptible individuals? The inbreeding behavior of varroa makes resistance development and management especially challengingly and very different from many other pests. This mite displays arrhenotokous parthenogenesis (Reams and Rangel, 2022). In hives with low density mite infestations bee pupal cells are typically occupied by single foundress mites. Inbreeding necessarily then results, with the first offspring being a male from an unfertilised egg. This male then mates with subsequent sister female offspring that develop from fertilized egg. Outbreeding between lineages only occurs in scenarios of high mite abundance when multiple foundress mites inhabit a single bee pupal cell. Low to moderate mite densities thus limit the opportunity for selection and outbreeding and genes for pesticide resistance to be lost from populations. It is the number of mites per brood cell in hives that will determine the genetic populations structure of varroa, with simulations demonstrating that inbreeding and low mite abundance will enhance the fixation of mite with homozygous pesticide resistance alleles (Beaurepaire et al., 2017). Resistant allele homozygosity in varroa does appear to enhance physiological pesticide resistance (Mitton et al., 2021; Vlogiannitis et al., 2021a).
Compounding this inbreeding outcome, low mite densities limit the propensity of varroa to move between hives. In low abundance, mites appear likely to remain within the bee colony by an ability to selectively chose nurse bees and quickly move to new cells for reproduction (Cervo et al., 2014). It is only when mites attain higher densities that they are more frequently found on older foraging bees that have a higher likelihood of movement outside the hive and between hives.
What implications does the varroa life history and inbreeding have for a beekeeper in an area where varroa have developed resistance to synthetic chemicals? These mites (and their genes) have been moved around between beekeeping operations due to management practices such as receiving packaged bees that may already have pesticide resistance (Strange et al., 2008). Natural mite movement behaviors can also contribute to the spread of resistance genotypes, including via bee drifting (Kulhanek et al., 2021), robbing (Peck and Seeley, 2019), or even by mites moving between bees while foraging on flowers (Peck et al., 2016). A beekeeper who acquires resistant mites but successfully maintains low varroa infestations, possibly even without relying on synthetic chemical pesticides and instead employing varroa-resistant bee strains and oxalic acid treatments, might inadvertently facilitate the persistence of resistance to synthetic chemicals. By sustaining low mite densities, this diligent beekeeper essentially perpetuates inbreeding and limits opportunities for outbreeding. Genetic drift alone cannot be counted on to eliminate genetic variation, and the inbreeding mating behavior of varroa will likely result in numerous inbred mite lineages, each harbouring different alleles in a homozygous state (Beaurepaire et al., 2017). Even if all beekeepers in the region cease using a particular pesticide for several years, those who successfully maintain low mite numbers during this period are essentially preserving reservoirs of resistance. Thus, management actions that involve decreasing insecticide application rates, reducing the frequency of application, or even using non-persistent chemicals may not contribute toward IRM in varroa.
The use of ‘refuges’ can be beneficial for the management other pests including with other mite species (Lester et al., 1998). However, refuges may offer limited utility in the context of varroa management. For varroa IRM, a requirement for refuges would be to maintain populations of homozygous, susceptible females. These susceptible females would need to migrate from refuges to cohabit bee pupal cells with resistant mites to enable the potential for crossbreeding between lineages. As noted previously, for cohabitation or co-occurrence to occur would generally require high mite densities. Deleterious fitness effects are also assumed as necessary for the selection of and reversion to susceptible genotypes. It is possible that beekeepers who use non-chemical means of controlling varroa could develop such refuge populations of acaricide-susceptible varroa. However, it is hard to be optimistic regarding the use of refuges for varroa IRM given the previously discussed, frequent long-term maintenance of resistance in varroa after the cessation of pesticide use. Further, the national and international movement of bees could negate any benefits of refuges. Beekeeping operations frequently entail the movement of bee colonies within and between regions, countries, and even across continents. Packages or nucleus colonies of bees, and even truckloads of entire hives, inadvertently carrying varroa mites and associated viruses, are transported within countries and globally (Martin, 2004; Strange et al., 2008; Budge et al., 2020). The high rate of varroa movement within and between countries via bee packages or the transport of entire hives makes resistance development in one region a global problem. Any benefits for resistance management that beekeepers may accrue through the use of refuges could be negated through hive and mite movement.
Additional, new synthetic chemical acaricides are being investigated including carbamates (Jack et al., 2022) and lithium salts including lithium formate (Ziegelmann et al., 2018; Sevin et al., 2022). Non-synthetic acaricide measures may be a key method to reducing varroa selection pressure and complementing control efforts. The ‘soft’ acaricides that include oxalic acid, thymol, and formic acid have become widespread, with oxalic acid often seen as most effective of the soft acaricides in terms of mite control and honey yield (Qadir et al., 2021). In 2020/2021 the most common acaricide treatment method in the UK was oxalic acid, followed by thymol and amitraz (Valentine and Martin, 2023). There has been no reported evidence of resistance to oxalic acid treatments. An additional emerging technology is gene silencing or dsRNA based biopesticide treatments, which can have be directly lethal to varroa (Garbian et al., 2012) or be used in the silencing of genes in resistance pathways (Vlogiannitis et al., 2021b). Honey bee bacterial symbionts have recently been produced to express dsRNA for both DWV and varroa control (Leonard et al., 2020). Another bee-safe approach might be through selective acaricides targeting the varroa mite‐specific neuropeptides and signaling systems (Jindal et al., 2022). These biopesticides and soft acaricides offer an opportunity for enhancing varroa IRM, as they could reduce acaricide selection pressure and complement synthetic pesticide control efforts (Georghiou, 1994).
In summary, regarding varroa IRM through moderation:
● Pesticide-susceptible genes and individuals represent a valuable resource. Currently, it remains unclear how IRM practices can be effectively employed to increase the frequency of susceptible genes and individuals within a population.
● Due to varroa’s inbreeding system, resistance is likely to persist in populations even after discontinuing the use of a particular pesticide. This is especially true in scenarios where mite populations are kept at low levels. Consequently, IRM strategies like infrequently applying different acaricides with diverse modes of action are expected to have limited efficacy in reversing resistance in varroa populations.
● Resistance is already widespread in varroa, likely implying that in IRM management actions such as lowering insecticide application rates having limited value. The value of refuges could be via supplying homozygous, susceptible females that could reduce or dilute resistance. However, the value of refuges is largely unknown for varroa IRM.
● The adoption of non-persistent soft acaricides like oxalic acid, as well as the introduction of new acaricides and emerging treatments such as biopesticides including varroa-specific double-stranded RNA (dsRNA), presents opportunities for IRM. The integration of these alternatives into varroa IRM strategies should be encouraged as well as resistance monitoring in mite populations to understand their benefit.
5 Conclusions
The global plan for managing insecticide resistance in malaria vectors was formulated by the World Health Organization Global Malaria Program (WHO, 2012). This plan has been recognized as one of the few comprehensive documents addressing the management of a worldwide insecticide resistance crisis. It places a strong emphasis on five interconnected “pillars” for a global strategy, namely: (i) devising and implementing insecticide resistance management strategies, (ii) ensuring ongoing resistance monitoring, (iii) developing novel control tools, (iv) addressing knowledge gaps, and (v) establishing essential enabling mechanisms, which encompass advocacy and the allocation of financial resources (WHO, 2012; Sternberg and Thomas, 2018; Dusfour et al., 2019). My assessment is that IRM is far less developed for varroa than it is for malaria vectors or than for other pests including lepidopteran species in horticulture (Walker et al., 2017). Varroa IRM needs a global strategy that could be modelled using the malaria vector approach.
Varroa management encompasses three fundamental, broad beekeeping practices: employing resistant bee strains, implementing apitechnical measures such as brood removal, and utilizing chemical control methods (Bubnič et al., 2021). Chemical control methods are likely to remain as one of these key pillars for the foreseeable future, and thus IRM should be a priority. Research is required across nearly all the facets of varroa IRM, including the evaluation of mixtures, synergists, assessing fitness costs associated with resistance, and even in exploring the advantages of acaricide rotation. Among the numerous avenues and approaches proposed, the examination of the effectiveness and advantages of pesticide mixtures and synergists stands out as particularly promising (Helps et al., 2020), as well as the continuing development and use of oxalic acid, new chemicals including lithium salts, and biopesticides such as dsRNA. New developments and long-term analyses in varroa IRM are desperately needed in order to help stem colony losses due to varroa infestations around the globe.
Author contributions
PL: Conceptualization, Project administration, Visualization, Writing – original draft, Writing – review & editing.
Funding
The author(s) declare financial support was received for the research, authorship, and/or publication of this article. Funding to support the publication of this article was provided by Victoria University of Wellington.
Acknowledgments
I would like to thank the two reviewers, the editor, and the social insect laboratory group at Victoria University of Wellington for their useful comments and suggestions on this manuscript.
Conflict of interest
The author declares that the research was conducted in the absence of any commercial or financial relationships that could be construed as a potential conflict of interest.
The author(s) declared that they were an editorial board member of Frontiers, at the time of submission. This had no impact on the peer review process and the final decision.
Publisher’s note
All claims expressed in this article are solely those of the authors and do not necessarily represent those of their affiliated organizations, or those of the publisher, the editors and the reviewers. Any product that may be evaluated in this article, or claim that may be made by its manufacturer, is not guaranteed or endorsed by the publisher.
References
Alissandrakis E., Ilias A., Tsagkarakou A. (2017). Pyrethroid target site resistance in Greek populations of the honey bee parasite Varroa destructor (Acari: Varroidae). J. Apicult. Res. 56 (5), 625–630. doi: 10.1080/00218839.2017.1368822
Beaurepaire A. L., Krieger K. J., Moritz R. F. A. (2017). Seasonal cycle of inbreeding and recombination of the parasitic mite Varroa destructor in honeybee colonies and its implications for the selection of acaricide resistance. Infect. Genet. Evol. 50, 49–54. doi: 10.1016/j.meegid.2017.02.011
Bubnič J., Moosbeckhofer R., Prešern J., Moškrič A., Formato G., Pietropaoli M., et al. (2021). Three pillars of Varroa control. Apidologie 52 (6), 1305–1333. doi: 10.1007/s13592-021-00903-4
Budge G. E., Simcock N. K., Holder P. J., Shirley M. D. F., Brown M. A., Van Weymers P. S. M., et al. (2020). Chronic bee paralysis as a serious emerging threat to honey bees. Nat. Commun. 11 (1), 2164. doi: 10.1038/s41467-020-15919-0
Calderone N. W., Lin S. (2001). Behavioural responses of Varroa destructor (Acari: Varroidae) to extracts of larvae, cocoons and brood food of worker and drone honey bees, Apis mellifera (Hymenoptera: Apidae). Physiol. Entomol. 26 (4), 341–350. doi: 10.1046/j.0307-6962.2001.00254.x
Cervo R., Bruschini C., Cappa F., Meconcelli S., Pieraccini G., Pradella D., et al. (2014). High Varroa mite abundance influences chemical profiles of worker bees and mite-host preferences. J. Exp. Biol. 217 (17), 2998–3001. doi: 10.1242/jeb.099978
Chapman N. C., Colin T., Cook J., da Silva C. R. B., Gloag R., Hogendoorn K., et al. (2023). The final frontier: ecological and evolutionary dynamics of a global parasite invasion. Biol. Lett. 19 (5), 20220589. doi: 10.1098/rsbl.2022.0589
Churcher T. S., Lissenden N., Griffin J. T., Worrall E., Ranson H. (2016). The impact of pyrethroid resistance on the efficacy and effectiveness of bednets for malaria control in Africa. Elife 5, e16090. doi: 10.7554/eLife.16090
De Jong D., Lester P. J. (2023). The global challenge of improving bee protection and health. Front. Bee. Sci. 1. doi: 10.3389/frbee.2023.1118292
Denholm I., Rowland M. W. (1992). Tactics for managing pesticide resistance in arthropods: theory and practice. Annu. Rev. Entomol. 37, 91–112. doi: 10.1146/annurev.en.37.010192.000515
Dusfour I., Vontas J., David J. P., Weetman D., Fonseca D. M., Corbel V., et al. (2019). Management of insecticide resistance in the major Aedes vectors of arboviruses: advances and challenges. PloS Negl. Trop. Dis. 13 (10), e0007615. doi: 10.1371/journal.pntd.0007615
Eliash N., Singh N. K., Kamer Y., Pinnelli G. R., Plettner E., Soroker V. (2014). Can we disrupt the sensing of honey bees by the bee parasite Varroa destructor? PloS One 9 (9), e106889. doi: 10.1371/journal.pone.0106889
El-Sayed A. M., Suckling D. M., Wearing C. H., Byers J. A. (2006). Potential of mass trapping for long-term pest management and eradication of invasive species. J. Econ. Entomol. 99 (5), 1550–1564. doi: 10.1093/jee/99.5.1550
Elzen P., Westervelt D. (2004). A scientific note on reversion of fluvalinate resistance to a degree of susceptibility in Varroa destructor. Apidologie 35 (5), 519–520. doi: 10.1051/apido:2004036
Farnahm A. W. (1998). “Chapter 12: The mode of action of piperonyl butoxide with reference to studying pesticide resistance,” in Piperonyl Butoxide: The Insecticide Synergist. Ed. Jones G. (London, England: Acadmeic Press), 199–213.
Felden A., Dobelmann J., Baty J. W., McCormick J., Haywood J., Lester P. J. (2023). Can immune gene silencing via dsRNA feeding promote pathogenic viruses to control the globally invasive Argentine ant? Ecol. Appl. 33 (2), e2755. doi: 10.1002/eap.2755
Frey E., Odemer R., Blum T., Rosenkranz P. (2013). Activation and interruption of the reproduction of Varroa destructor is triggered by host signals (Apis mellifera). J. Invertebrate. Pathol. 113 (1), 56–62. doi: 10.1016/j.jip.2013.01.007
Garbian Y., Maori E., Kalev H., Shafir S., Sela I. (2012). Bidirectional transfer of RNAi between honey bee and Varroa destructor: Varroa gene silencing reduces Varroa population. PloS Pathog. 8 (12), e1003035. doi: 10.1371/journal.ppat.1003035
Georghiou G. P. (1994). Principles of insecticide resistance management. Phytoprotection 75 (4), 51–59. doi: 10.7202/706071ar
González-Cabrera J., Bumann H., Rodríguez-Vargas S., Kennedy P. J., Krieger K., Altreuther G., et al. (2018). A single mutation is driving resistance to pyrethroids in European populations of the parasitic mite, Varroa destructor. J. Pest Sci. 91 (3), 1137–1144. doi: 10.1007/s10340-018-0968-y
Helps J. C., Paveley N. D., White S., van den Bosch F. (2020). Determinants of optimal insecticide resistance management strategies. J. Theor. Biol. 503, 110383. doi: 10.1016/j.jtbi.2020.110383
Higes M., Martin-Hernandez R., Hernandez-Rodriguez C. S., Gonzalez-Cabrera J. (2020). Assessing the resistance to acaricides in Varroa destructor from several Spanish locations. Parasitol. Res. 119 (11), 3595–3601. doi: 10.1007/s00436-020-06879-x
Jack C. J., Ellis J. D. (2021). Integrated pest management control of Varroa destructor (Acari: Varroidae), the most damaging pest of (Apis mellifera L. (Hymenoptera: Apidae)) colonies. J. Insect Sci. 21 (5), 1–32. doi: 10.1093/jisesa/ieab058
Jack C. J., Kleckner K., Demares F., Rault L. C., Anderson T. D., Carlier P. R., et al. (2022). Testing new compounds for efficacy against Varroa destructor and safety to honey bees (Apis mellifera). Pest Manage. Sci. 78 (1), 159–165. doi: 10.1002/ps.6617
Jindal V., Li D., Rault L. C., Fatehi S., Singh R., Mating M., et al. (2022). Bee-safe peptidomimetic acaricides achieved by comparative genomics. Sci. Rep. 12 (1), 17263. doi: 10.1038/s41598-022-20110-0
Kiljanek T., Niewiadowska A., Gawel M., Semeniuk S., Borzecka M., Posyniak A., et al. (2017). Multiple pesticide residues in live and poisoned honeybees - preliminary exposure assessment. Chemosphere 175, 36–44. doi: 10.1016/j.chemosphere.2017.02.028
Kliot A., Ghanim M. (2012). Fitness costs associated with insecticide resistance. Pest Manage. Sci. 68 (11), 1431–1437. doi: 10.1002/ps.3395
Kulhanek K., Garavito A., vanEngelsdorp D. (2021). Accelerated Varroa destructor population growth in honey bee (Apis mellifera) colonies is associated with visitation from non-natal bees. Sci. Rep. 11 (1), 7092. doi: 10.1038/s41598-021-86558-8
Le Conte Y., Ellis M., Ritter W. (2010). Varroa mites and honey bee health: can Varroa explain part of the colony losses? Apidologie 41 (3), 353–363. doi: 10.1051/apido/2010017
Leonard S. P., Powell J. E., Perutka J., Geng P., Heckmann L. C., Horak R. D., et al. (2020). Engineered symbionts activate honey bee immunity and limit pathogens. Science 367 (6477), 573–576. doi: 10.1126/science.aax9039
Lester P. J., Thistlewood H. M. A., Harmsen R. (1998). The effects of refuge size and number on acarine predator-prey dynamics in a pesticide-disturbed apple orchard. J. Appl. Ecol. 35 (2), 323–331. doi: 10.1046/j.1365-2664.1998.00304.x
Lodesani M., Colombo M., Spreafico M. (1995). Ineffectiveness of Apistan® treatment against the mite Varroa jacobsoni Oud in several districts of Lombardy (Italy). Apidologie 26 (1), 67–72. doi: 10.1051/apido:19950109
Mao Y. B., Cai W. J., Wang J. W., Hong G. J., Tao X. Y., Wang L. J., et al. (2007). Silencing a cotton bollworm P450 monooxygenase gene by plant-mediated RNAi impairs larval tolerance of gossypol. Nat. Biotechnol. 25 (11), 1307–1313. doi: 10.1038/nbt1352
Maggi M.D., Ruffinengo S.R., Mendoza Y., Ojeda P., Ramallo G., Floris I., et al. (2011). Susceptibility of Varroa destructor (Acari: Varroidae) to synthetic acaricides in Uruguay: Varroa mites' potential to develop acaricide resistance. Parasitol. Res. 108 (4), 815–821. doi: 10.1007/s00436-010-2122-5
Martin S. J. (2004). Acaricide (pyrethroid) resistance in Varroa destructor. Bee. World 85 (4), 67–69. doi: 10.1080/0005772x.2004.11099632
Martin S. J., Elzen P. J., Rubink W. R. (2002). Effect of acaricide resistance on reproductive ability of the honey bee mite Varroa destructor. Exp. Appl. Acarol. 27 (3), 195–207. doi: 10.1023/a:1021675614116
McGruddy R. A., Bulgarella M., Felden A., Baty J. W., Haywood J., Stahlmann-Brown P., et al. (2023). Are increasing honey bee colony losses attributed to Varroa destructor in New Zealand driven by miticide resistance? bioRxiv. preprint. March. 24, 2023. doi: 10.1101/2023.03.22.533871
Milani N. (1999). The resistance of Varroa jacobsoni Oud. to acaricides. Apidologie 30 (2-3), 229–234. doi: 10.1051/apido:19990211
Milani N., Della Vedova G. (2002). Decline in the proportion of mites resistant to fluvalinate in a population of Varroa destructor not treated with pyrethroids. Apidologie 33 (4), 417–422. doi: 10.1051/apido:2002028
Mitton G. A., Meroi Arcerito F., Cooley H., Fernández de Landa G., Eguaras M. J., Ruffinengo S. R., et al. (2022). More than sixty years living with Varroa destructor: a review of acaricide resistance. Int. J. Pest Manage., 1–18. doi: 10.1080/09670874.2022.2094489
Mitton G. A., Quintana S., Mendoza Y., Eguaras M., Maggi M. D., Ruffinengo S. R. (2021). L925V mutation in voltage-gated sodium channel of Varroa destructor populations from Argentina and Uruguay, with different degree of susceptibility to pyrethroids. Int. J. Acarol. 47 (5), 374–380. doi: 10.1080/01647954.2021.1914158
Mitton G. A., Szawarski N., Ramos F., Fuselli S., Meroi Arcerito F. R., Eguaras M. J., et al. (2018). Varroa destructor: when reversion to coumaphos resistance does not happen. J. Apicult. Res. 57 (4), 536–540. doi: 10.1080/00218839.2018.1475038
Mnzava A. P., Knox T. B., Temu E. A., Trett A., Fornadel C., Hemingway J., et al. (2015). Implementation of the global plan for insecticide resistance management in malaria vectors: progress, challenges and the way forward. Malaria J. 14, 173. doi: 10.1186/s12936-015-0693-4
Morfin N., Rawn D., Petukhova T., Kozak P., Eccles L., Chaput J., et al. (2022). Surveillance of synthetic acaricide efficacy against Varroa destructor in Ontario, Canada. Can. Entomol. 154 (1), e17. doi: 10.4039/tce.2022.4
Mullin C. A., Frazier M., Frazier J. L., Ashcraft S., Simonds R., Vanengelsdorp D., et al. (2010). High levels of miticides and agrochemicals in North American apiaries: implications for honey bee health. PloS One 5 (3), e9754. doi: 10.1371/journal.pone.0009754
Nazzi F., Le Conte Y. (2016). Ecology of Varroa destructor, the major ectoparasite of the western honey bee, Apis mellifera. Annu. Rev. Entomol. 61, 417–432. doi: 10.1146/annurev-ento-010715-023731
Nishimatsu T., Jackson J. J. (1998). Interaction of insecticides, entomopathogenic nematodes, and larvae of the western corn rootworm (Coleoptera: Chrysomelidae). J. Econ. Entomol. 91 (2), 410–418. doi: 10.1093/jee/91.2.410
Orr H. A. (2009). Fitness and its role in evolutionary genetics. Nat. Rev. Genet. 10 (8), 531–539. doi: 10.1038/nrg2603
Overmeer W. P. J., van Zon A. Q., Helle W. (1975). The stability of acaricide resistance in spider mites (Tetranychus urticae) populations from rose houses. Entomol. Experimentalis. Applicata. 16 (1), 68–74. doi: 10.1111/j.1570-7458.1975.tb00387.x
Peck D. T., Seeley T. D. (2019). Mite bombs or robber lures? The roles of drifting and robbing in Varroa destructor transmission from collapsing honey bee colonies to their neighbors. PloS One 14 (6), e0218392. doi: 10.1371/journal.pone.0218392
Peck D. T., Smith M. L., Seeley T. D. (2016). Varroa destructor mites can nimbly climb from flowers onto foraging honey bees. PloS One 11 (12), e0167798. doi: 10.1371/journal.pone.0167798
Pernal S. F., Baird D. S., Birmingham A. L., Higo H. A., Slessor K. N., Winston M. L. (2005). Semiochemicals influencing the host-finding behaviour of Varroa destructor. Exp. Appl. Acarol. 37 (1-2), 1–26. doi: 10.1007/s10493-005-1117-x
Plettner E., Eliash N., Singh N. K., Pinnelli G. R., Soroker V. (2016). The chemical ecology of host-parasite interaction as a target of Varroa destructor control agents. Apidologie 48 (1), 78–92. doi: 10.1007/s13592-016-0452-8
Qadir Z. A., Idrees A., Mahmood R., Sarwar G., Bakar M. A., Ahmad S., et al. (2021). Effectiveness of different soft acaricides against honey bee ectoparasitic mite Varroa destructor (Acari: Varroidae). Insects 12, 1032. doi: 10.3390/insects12111032
Reams T., Rangel J. (2022). Understanding the enemy: a review of the genetics, behavior and chemical ecology of Varroa destructor, the parasitic mite of Apis mellifera. J. Insect Sci. 22 (1), 1–10. doi: 10.1093/jisesa/ieab101
Rinkevich F. D., Moreno-Marti S., Hernandez-Rodriguez C. S., Gonzalez-Cabrera J. (2023). Confirmation of the Y215H mutation in the beta(2) -octopamine receptor in Varroa destructor is associated with contemporary cases of amitraz resistance in the United States. Pest Manage. Sci. 79 (8), 2840–2845. doi: 10.1002/ps.7461
Rosenkranz P., Aumeier P., Ziegelmann B. (2010). Biology and control of Varroa destructor. J. Invertebrate. Pathol. 103, S96–S119. doi: 10.1016/j.jip.2009.07.016
Sevin S., Bommuraj V., Chen Y., Afik O., Zarchin S., Barel S., et al. (2022). Lithium salts: Assessment of their chronic and acute toxicities to honey bees and their anti-Varroa field efficacy. Pest Manage. Sci. 78 (11), 4507–4516. doi: 10.1002/ps.7071
Stahlmann-Brown P., Hall R. J., Pragert H., Robertson T. (2022). Varroa appears to drive persistent increases in New Zealand colony losses. Insects 13 (7), 589. doi: 10.3390/insects13070589
Sternberg E. D., Thomas M. B. (2018). Insights from agriculture for the management of insecticide resistance in disease vectors. Evol. Appl. 11 (4), 404–414. doi: 10.1111/eva.12501
Strange J. P., Cicciarelli R. P., Calderone N. W. (2008). What’s in that package? An evaluation of quality of package honey bee (Hymenoptera: Apidae) shipments in the United States. J. Econ. Entomol. 101 (3), 668–673. doi: 10.1093/jee/101.3.668
Suckling D. M., Baker G., Salehi L., Woods B. (2016). Is the combination of insecticide and mating disruption synergistic or additive in lightbrown apple moth, Epiphyas postvittana? PloS One 11 (8), e0160710. doi: 10.1371/journal.pone.0160710
Sudo M., Takahashi D., Andow D. A., Suzuki Y., Yamanaka T. (2018). Optimal management strategy of insecticide resistance under various insect life histories: Heterogeneous timing of selection and interpatch dispersal. Evol. Appl. 11 (2), 271–283. doi: 10.1111/eva.12550
Traynor K. S., Mondet F., de Miranda J. R., Techer M., Kowallik V., Oddie M. A. Y., et al. (2020). Varroa destructor: a complex parasite, crippling honey bees worldwide. Trends Parasitol. 36 (7), 592–606. doi: 10.1016/j.pt.2020.04.004
Valentine A., Martin S. J. (2023). A survey of UK beekeeper's Varroa treatment habits. PloS One 18 (2), e0281130. doi: 10.1371/journal.pone.0281130
Van Leeuwen T., Dermauw W. (2016). The molecular evolution of xenobiotic metabolism and resistance in Chelicerate mites. Annu. Rev. Entomol. 61, 475–498. doi: 10.1146/annurev-ento-010715-023907
Vegh R., Csoka M., Mednyanszky Z., Sipos L. (2023). Pesticide residues in bee bread, propolis, beeswax and royal jelly - a review of the literature and dietary risk assessment. Food Chem. Toxicol. 176, 113806. doi: 10.1016/j.fct.2023.113806
Vlogiannitis S., Jonckheere W., Laget D., de Graaf D. C., Vontas J., Van Leeuwen T. (2021a). Pyrethroid target-site resistance mutations in populations of the honey bee parasite Varroa destructor (Acari: Varroidae) from Flanders, Belgium. Exp. Appl. Acarol. 85 (2-4), 205–221. doi: 10.1007/s10493-021-00665-9
Vlogiannitis S., Mavridis K., Dermauw W., Snoeck S., Katsavou E., Morou E., et al. (2021b). Reduced proinsecticide activation by cytochrome P450 confers coumaphos resistance in the major bee parasite Varroa destructor. Proc. Natl. Acad. Sci. 118 (6), e2020380118. doi: 10.1073/pnas.2020380118
Walker J. T., Suckling D. M., Wearing C. H. (2017). Past, present, and future of integrated control of apple pests: the New Zealand experience. Annu. Rev. Entomol. 62, 231–248. doi: 10.1146/annurev-ento-031616-035626
Wang Y., Xie X., Qin L., Yu D., Wang Z., Huang B. (2021). Integration of dsRNA against host immune response genes augments the virulence of transgenic Metarhizium robertsii strains in insect pest species. Microbial. Biotechnol. 14 (4), 1433–1444. doi: 10.1111/1751-7915.13748
Warner S., Pokhrel L. R., Akula S. M., Ubah C. S., Richards S. L., Jensen H., et al. (2024). A scoping review on the effects of Varroa mite (Varroa destructor) on global honey bee decline. Sci. Total. Environ. 906, 167492. doi: 10.1016/j.scitotenv.2023.167492
Whitfield A. E., Rotenberg D. (2023). Pests and resistance: The biology and control of supervectors and superpests. Curr. Opin. Insect Sci. 58, 101060. doi: 10.1016/j.cois.2023.101060
WHO. (2012). Global plan for insecticide resistance management in malaria vectors (GPIRM) (Geneva, Switzerland: World Health Organization).
Young S. J., Gunning R. V., Moores G. D. (2006). Effect of pretreatment with piperonyl butoxide on pyrethroid efficacy against insecticide-resistant Helicoverpa armigera (Lepidoptera: Noctuidae) and Bemisia tabaci (Sternorrhyncha: Aleyrodidae). Pest Manage. Sci. 62 (2), 114–119. doi: 10.1002/ps.1127
Keywords: honey bee (Apis mellifera) health, pesticide resistance, resistance fitness costs, acaricide rotation and mixtures, synergists, refuges, novel acaricide development
Citation: Lester PJ (2023) Integrated resistance management for acaricide use on Varroa destructor. Front. Bee Sci. 1:1297326. doi: 10.3389/frbee.2023.1297326
Received: 19 September 2023; Accepted: 07 December 2023;
Published: 21 December 2023.
Edited by:
Victoria Soroker, Agricultural Research Organization (ARO), IsraelReviewed by:
Joel González-Cabrera, University of Valencia, SpainEstela Santos, Universidad de la República, Uruguay
Copyright © 2023 Lester. This is an open-access article distributed under the terms of the Creative Commons Attribution License (CC BY). The use, distribution or reproduction in other forums is permitted, provided the original author(s) and the copyright owner(s) are credited and that the original publication in this journal is cited, in accordance with accepted academic practice. No use, distribution or reproduction is permitted which does not comply with these terms.
*Correspondence: Philip J. Lester, UGhpbC5MZXN0ZXJAdnV3LmFjLm56